- 1Aquatic Systems Biology Unit, TUM School of Life Sciences, Technical University of Munich, Freising, Germany
- 2Department of Infrastructure Engineering, School of Engineering, University of Melbourne, Parkville, VIC, Australia
An increase in river water temperatures is being detected worldwide, with some predictions of an up to 4°C rise by 2050. Such stream temperature increase is likely to affect aquatic communities, with predicted declines and range shifts of cold stenothermic species and a facilitated dispersal and population development in temperature-tolerant species, including invasive ones. This study analyzed how thermal changes affect macroinvertebrate community compositions in three differentiated thermal regions within a single stream system. In each thermal region, we used standard surber sampling in combination with in-stream cross-exposure experiments, comparing the thermal response of native vs. non-native gammarids (Gammarus roeselii and Dikerogammarus villosus). Macroinvertebrate species composition was highly dependent on temperature, with indigenous gammarids preferring colder sites and invasive ones such as D. villosus being dominant at higher temperatures. Species composition was also strongly affected by season, substratum and the presence of macrophytes. In light of climate change, consideration of shifts in community composition highly driven by temperature needs to become integrated with biological response patterns related to morphological and flow degradation, chemical pollution and fine sediment. Such an approach is crucial for the effective conservation and restoration of native biodiversity and for a realistic prediction of the ability to reach policy targets for aquatic ecosystems.
1 Introduction
An increase in stream water temperatures is being detected worldwide because of global warming. Mean increases of at least 0.5°C per decade have been observed (Daufresne et al., 2004; Durance and Ormerod, 2009; O’Reilly et al., 2015; Liu et al., 2020; Niedrist and Füreder, 2020). As global warming continues, stream temperature predictions suggest rises of 1.5°C by 2030, and, in some regions of the world, up to 4°C by 2050 (Wanders et al., 2019). Increased water temperatures in rivers can be accelerated by anthropogenic structures like weirs and dams, which slow the flow and promote warming of the upper water layers (Schneider et al., 2013; Zaidel et al., 2021). Water temperature is directly related to oxygen concentrations which are crucial for biota in these systems (Piatka et al., 2021). Greater seasonal variation in water temperature and higher temperature extremes in summer can result in severe stress on riverine organisms (Durance and Ormerod, 2009; van Vliet et al., 2013), with cold stenothermic species being most heavily affected (Fullerton et al., 2018). Consequences include the decline of cold-water adapted native species and a potential increase of opportunistic invasive species (Daufresne et al., 2004; Haidekker and Hering, 2008; Rahel and Olden, 2008). While cold-stenothermic fishes such as salmonids can quickly move to more oxygen-rich cold-water patches (reviewed in Smialek et al., 2021), these suitable thermal habitats likely become scarcer, more distant and less accessible in future climatic scenarios (Kuhn et al., 2021). For other organisms such as macroinvertebrates (MIV) during the aquatic phases of their life cycles, reaching potentially cooler water may even be harder given their limited mobility compared to fish, which may leave them more exposed to short-term extremes in water temperature. Especially in hot and dry summers, when the predicted maximum temperatures have the potential to exceed the upper tolerance levels of many MIV species (Leigh et al., 2015), pronounced changes in MIV community composition can be expected (Lento et al., 2021). MIV play a key role in regulating functional processes in streams and are an essential food source for higher trophic levels (Wallace and Webster, 1996; Huryn, 1998; Ruetz et al., 2002). Therefore, MIV community changes may have consequences for the entire food web and the resilience of riverine ecosystems.
The effects of thermal-induced seasonal changes in stream community composition are not fully clear. Still, shifts driven by species-specific thermal tolerances, especially in those having their entire life cycle in aquatic environments—such as gammarids—(Kolding and Fenchel, 1979), should be expected. Gammarids are ideal indicators to study stream temperature effects in aquatic habitats given their functional importance for these systems (Boeker and Geist, 2015) as well as their sensitivity to habitat structure and temperature, with clearly different thermal thresholds between species (Wijnhoven et al., 2003; Cottin et al., 2012; Halle et al., 2016).
The aim of this study was to analyze how spatio-temporal thermal changes may affect MIV communities, placing special emphasis on the comparison of native vs. non-native gammarids (Gammarus roeselii and Dikerogammarus villosus, respectively). We carried out sampling in combination with a cross-experiment (Figure 1) in a single restored stream system with three differentiated thermal regions [TR: cold (C), intermixed (I) and warm (W)] during spring and summer, to test the following hypotheses:
1) Differences in temperature between the three thermal regions will result in pronounced differences in MIV abundance and community composition.
2) Such differences in community composition will be most pronounced during the warmest season when maximum water temperatures and upper temperature tolerance thresholds of certain species are reached. During these periods, we expect 1) a decrease of temperature-sensitive taxa as water temperatures approach their upper thermal limits; 2) an increase of species with greater temperature tolerances (often non-indigenous species) as they will be able to colonize warmer habitats.
3) Specifically, native vs. non-native gammarid species (with more and less sensitivity to upper thermal limits, respectively) will show differentiated mortality rates under different thermal regions based on their thermal tolerances.
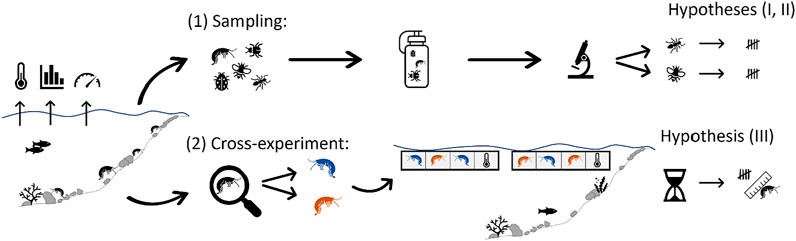
FIGURE 1. Schematic view of the study concept including the dual experiments related to (A) the sampling of macroinvertebrates, and (B) cross-exposure of selected MIV, and the hypotheses they each addressed. Colored pictograms of gammarids (orange = Dikerogammarus villosus and blue = Gammarus roeselii) represent the species used in the cross-exposure experiment.
In order to test our three hypotheses, we designed a dual experiment (Figure 1) to differentiate spatial vs. seasonal thermal effects on overall macroinvertebrate community composition and the targeted gammarids. The dual experiment consisted of 1) the characterization of the in-situ macroinvertebrate community during two seasons in three thermal regions (TR) of an interconnected stream system (hypotheses I and II), and 2) a cross-exposure of two gammarid species typically found in that stream system to investigate their survival in the 3 TR (hypothesis III), using standardized exposure to ambient stream water in the so-called “salmonid-egg floating boxes” (SEFLOBs, Pander and Geist, 2010).
2 Materials and Methods
2.1 Study Sites
The study area was located in a restored floodplain of the upper River Danube near Ingolstadt, southern Germany (Figure 2; River Danube, river km 2,472; Stammel et al., 2012; Fischer and Cyffka, 2014; Pander et al., 2018). The three interconnected TRs consisted of a cold (C), warm (W), and intermixed (I) region located in close proximity (less than 300 m to each other) without physical barriers in between, and had a similar river morphology that can be described as run without deeper pools or shallow riffles (Table 1). Current velocities range from 0.26 ms−1 to 0.59 ms−1 (Table 2), and substratum is mainly gravel with fine sediment patches in combination with macrophytes. Macrophyte coverage ranges between 30 and 77% and the most dominant species are Potamogeton pectinatus, Myriophyllum spicatum, Elodea sp., Callitriche sp., and Ranunculus fluitans. The three sites are similar in riparian vegetation with mostly alders (Alnus glutinosa), willows (Salix alba and Salix fragilis) and poplar trees (Populus x canadensis) at the river banks, the understory is dominated by nitrophilic shrubs such as Urtica dioica, Filipendula ulmaria, and Solidago canadensis. The trees are 15–20 m high, approximately 40 years old, and grow as a sparse riparian woody fringe partly shading the river corridor (Figure 2).
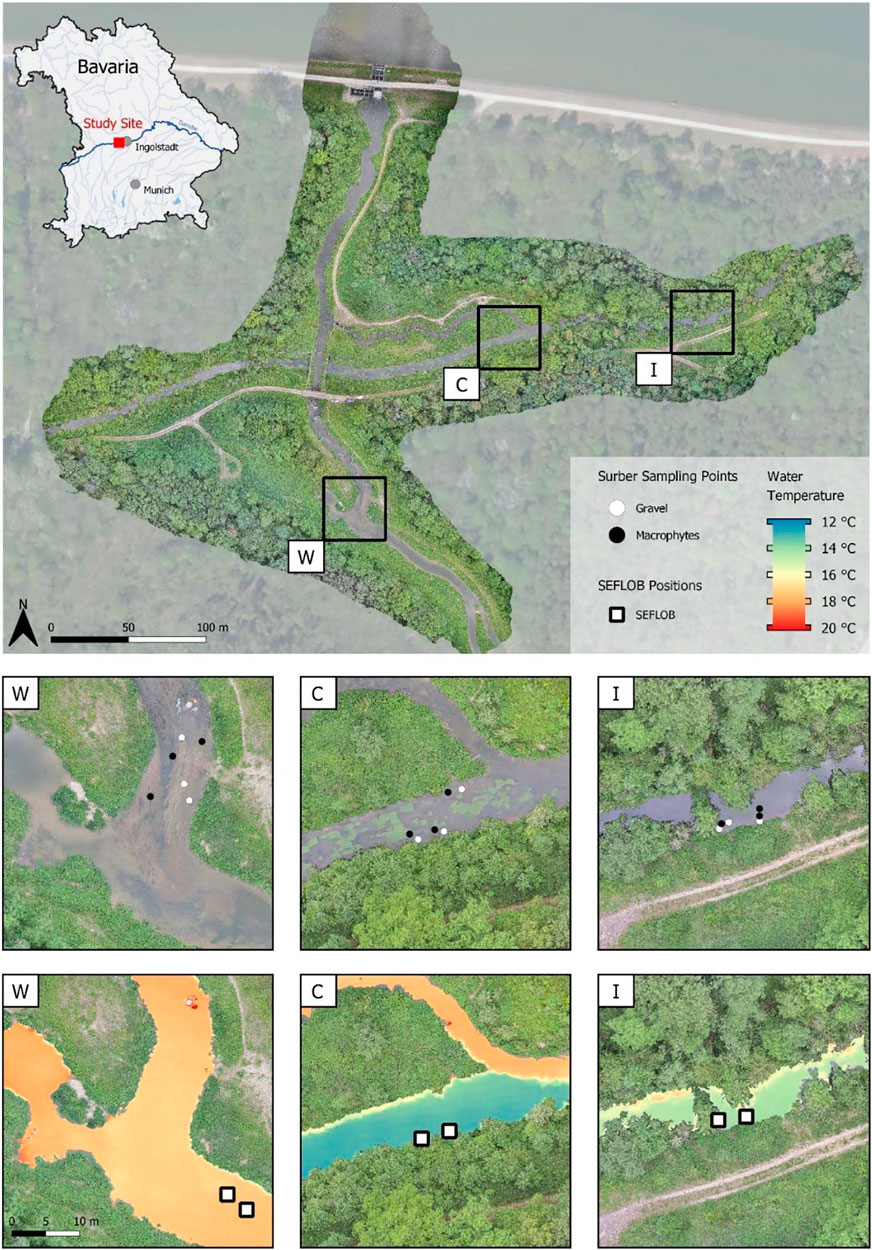
FIGURE 2. Overview of the study area with referenced thermal regions and habitats. Surber samples were taken at marked white and black dots (GR- and MP-dominated habitats, respectively), displayed in the top warm = W, C = cold and I = intermixed panels. The second row of W, C, and I panels displays the thermal regime assessed in spring using UAV thermal imaging before the sampling started (26 May 2020) with noticeable differences in water temperature between habitats. Base orthophoto: Landesamt für Digitalisierung, Breitband und Vermessung (2020).
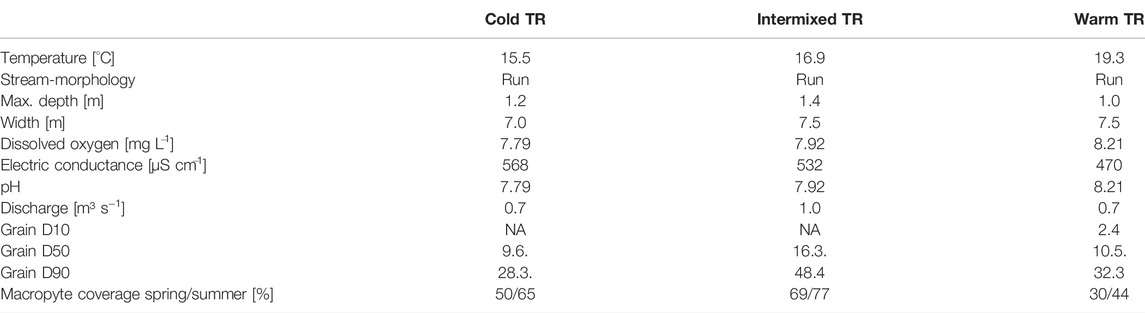
TABLE 1. Characterisation of the three different thermal regions (TR) cold, intermixed and warm. Temperature, dissolved oxygen, pH and electric conductance are given as means.
2.2 Abiotic Characterization of Thermal Regions and Habitats
During the study period, abiotic and hydraulic habitat characteristics were monitored to relate them to changes in MIV community and mortality of gammarids exposed (Tables 1,2). Sediment composition was determined using a box substratum sampler described in Pander et al. (2015a). Dissolved oxygen, O2 [mg l−1 and %], electric conductance, EC [µS cm−1, corrected to 20°C], and pH were measured with a hand-held WTW® Multimeter 340i (WTW GmbH, Weilheim, Germany), and current speed [ms−1] was recorded using Seba Flow-Sens (SEBA Hydrometrie, Kaufbeuren, Germany). Water temperature [°C] was measured in a resolution of 10 min using HOBO Pendant® Temperature/Light 8 K Data Loggers with three devices each installed in the 3 TRs (Figure 3).
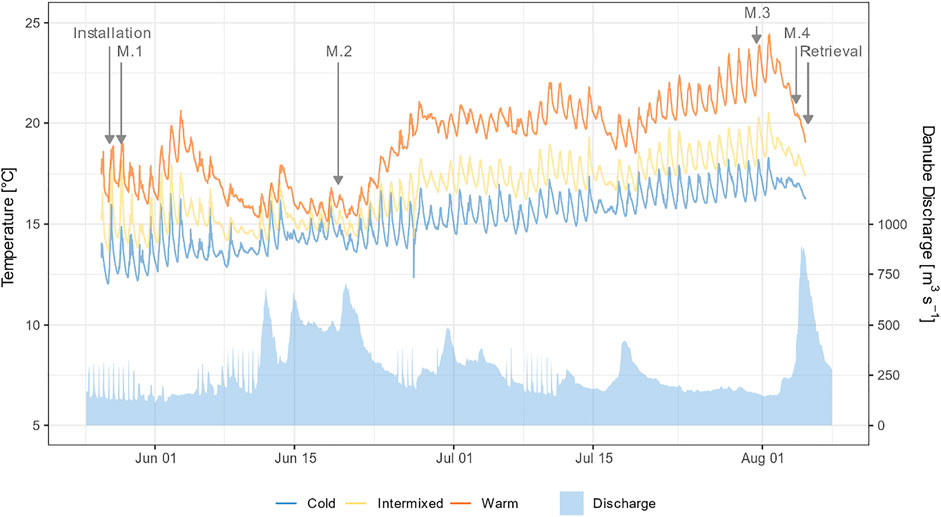
FIGURE 3. Mean water temperature of the three thermal regions over the study period generated from logger data. Hydrograph of the Danube measured at the nearest water gauge Neuburg a.d. Donau (Bayerisches Landesamt für Umwelt, 2020).
2.3 Experimental Setup of the Study Concept
2.3.1 Community Composition in Different Thermal Regions and Habitats
Macroinvertebrates were collected at the 3 TRs in spring and summer using a standardized surber sampler (Surber, 1937; Hauer and Lamberti, 2017). Within each TR, two sets of samples (three replicates in each) were collected at gravel (GR)—as well as macrophytes (MP)—dominated habitats (Figure 2). These sampling locations in each TR are further referred to as habitats. The sampling was repeated in two different seasons in spring and summer, adding up to 36 surber-samples, 18 taken in spring and another 18 in summer. The surber sampler was evenly placed on the corresponding habitat, and a metal fork was used to rework a defined area (0.096 m2) in front of the sampler to a depth of 10 cm, for 2 min. The MIV were then collected in a mesh net (mesh size 250 µM) and preserved in 70% ethanol following the procedure described in Mueller et al. (2011). Macroinvertebrates were pre-sorted in the laboratory according to Haase and Sundermann (2004) and assigned to the orders of Crustacea, Ephemeroptera, Plecoptera and Trichoptera (EPT-Taxa). Orders were then classified to the finest taxonomic resolution possible according to keys by Schmedtje and Kohmann (1992), Eggers and Martens (2001), Lechthaler (2009), and Eiseler (2010) using a binocular microscope (Stemi 305, Carl Zeiss Microscopy Deutschland GmbH, Oberkochen, Germany).
2.3.2 Thermal Cross-Exposure With Gammarids
2.3.2.1 Species Selection
For the thermal cross-exposure experiment, Dikerogammarus villosus and Gammarus roeselii were identified as the most suitable gammarid indicator species, representative of non-native and native species, respectively. Species selection was based on the following criteria: 1) robust enough to survive the handling; 2) easily distinguishable for life sorting; 3) expected different thermal optima; 4) part of the MIV community in the study streams and readily available in sufficient numbers (Pander et al., 2016); and 5) comprising one native and one non-native species. Although other gammarid species may be perceived as ‘more’ native in contrast to the naturalized G. roeselii, we selected this species given its easiness of determination in the field and its typical distribution in colder areas. Gammarids have been described to distinctly affect the physico-chemical and microbiological properties of freshwater substrates (Boeker and Geist, 2015), increasing the relevance of understanding changes in their community structure under climate change conditions.
The Ponto-Caspian invasive D. villosus has populated rivers all over Europe and with a mean reported optimum temperature of 20.2–25.8°C under optimal environmental conditions (Dick and Platvoet, 2000; Bruijs et al., 2001; Müller, 2001; Wijnhoven et al., 2003; Rewicz et al., 2015; Halle et al., 2016). This species tolerates more than 20% higher mean temperatures than G. roeselii. D. villosus has in general a larger brood size, higher partial fecundity and earlier maturation compared to G. roeselii (Grabowski et al., 2007a; Pöckl, 2007). G. roeselii is considered naturalised, meanwhile co-existing with native amphipods (Karaman and Pinkster, 1977; Müller, 2001; Grabowski et al., 2007b; Grabowski et al., 2017; Kuçi et al., 2017; Mauchart et al., 2017). It is easy to distinguish from any other gammarids by its dorsal spines and, therefore, clearly differs from D. villosus. (Karaman and Pinkster, 1977; Eggers and Martens, 2001; Lechthaler, 2009). The mean optimum temperature of G. roeselii has been determined to range between 17.45 and 17.88°C, with the ability to cope with temperatures above 20°C, however, resulting in increased stress and reduced egg survival rates (Pöckl, 1992; Sutcliffe, 1992; Wijnhoven et al., 2003; Halle et al., 2016).
2.3.2.2 Experimental Setup, Installation, Maintenance, and Retrieval
Salmonid-egg floating boxes were used to house the gammarids during the cross-exposure experiment ensuring the same physical environment for both MIV indicators. Each of the SEFLOBs (56 cm × 56 cm width and 15 cm depth, Pander and Geist, 2010) was subdivided into four compartments by sealed plastic plates. Each of the four compartments was 9.5 cm × 38 cm. Thirty individuals of each species were placed alternating by species in three compartments and a temperature logger was placed in the remaining compartment. Overall, this resulted in three compartments per species (G. roeselii and D. villosus) and thermal region (cold, warm and intermixed) resulting overall in 18 exposed compartments (Figure 1). Before installation, each of the perforated aluminum openings in the SEFLOBs were covered with a fine mesh gaze of 500 µM to prevent the gammarids from escaping and changing compartments. The fine mesh gaze also hindered other species that could harm or compete with the experimental subjects from entering. Two SEFLOBs were installed in each of the 3 TRs and were anchored within the riverbed close to the shore in moderate flow velocities (Pander and Geist, 2010) by attaching them to steel poles. Onset® HOBO Pendant® Temperature/Light 8 K data loggers were placed in each box to monitor potential temperature differences in the respective TRs. The two gammarid species had previously been collected within the study area using a modified kick-sampling method (Hauer and Lamberti, 2017, sampler opening 35 × 35 cm, mesh size 500 µM). The contents of the kick sample were placed in aerated water containers and immediately transported to the laboratory, where they were identified and sorted. A total of 270 individuals of each gammarid species were identified and used to populate the compartments in the SEFLOBS. Back in the field, three SEFLOB compartments were filled with 30 individuals each (Figure 1 (D-G-D; G-D-G)). After 3 days of acclimatization, gammarids were fed with a weekly dose of 1.5 g of fresh alder leaves (Alnus glutinosa) per compartment, according to Maltby et al. (2002). The functionality of the SEFLOBs was checked weekly to ensure no damage until their recovery 71 days of exposure (May 27–August 5). The exposure time of 71 days was to allow a heatwave to occur and retrieve. The SEFLOBs were then placed in large plastic trays, carried to shore, and gammarid individuals were collected from each compartment using large pipettes and subsequently preserved in 70% ethanol following the procedure described in Mueller et al. (2011). Species determination was carried out in the laboratory using the binocular microscope OLYMPUS SZX10 with mount camera module and computer software cellSens® (Olympus Europa SE & Co. KG, Hamburg, Germany). Only individuals with a body size >10 mm were considered.
2.4 Data Analysis
2.4.1 Community Composition in Different Thermal Habitats
Macroinvertebrates community composition was analyzed using multivariate statistics with R (R Core Team, 2020). In order to understand distribution patterns of macroinvertebrates, a non-metric multi-dimensional scaling (NMDS) on family- or species level combined with environmental variables was processed based on species abundance data and Bray-Curtis similarity using the R-package vegan. A permutational analysis of variance (PERMANOVA, R-package vegan; Anderson, 2001) based on Bray-Curtis similarities was applied to test for significant differences between the three different thermal regions (C, W, and I), and GR- and MP-dominated habitats. We used the same test to assess the differences in macroinvertebrate community composition during the two sampled seasons, spring and summer. In addition, a multilevel pairwise comparison was applied to identify species which contributed significantly to the differences of community composition between TRs using the R package pairwise Adonis (Martinez Arbizu, 2020). A multilevel pattern analysis of the library indicspecies was further used to identify those species that were significantly abundant for a particular habitat grouping parameter (Cáceres and Legendre, 2009). Species and environmental variables that showed a significant relation to the ordination of the NMDS were fitted to the plot with vectors (p ≤ 0.05 based on 999 permutations).
Diversity indices were calculated for species abundance, species richness, Shannon-index and Evenness based on TR and season and tested univariately in R. In addition, all recorded habitat variables such as water temperature, dissolved oxygen, electric conductance, pH and current speed were tested univariately between TRs. Each dataset was tested for normal distribution (Shapiro-Wilk test) and homoscedasticity (Levene test). Since data did not fulfil the criteria for parametric testing, the non-parametric Kruskal Wallis test was applied to test for significant differences. A subsequent post-hoc Wilcoxon test with Bonferroni correction for multiple comparisons was used to determine whether values differed significantly.
2.4.2 Thermal Cross-Exposure Experiment
Gammarid data of the cross-exposure experiment were analyzed using univariate statistics in R. Gammarid mortality was tested for normal distribution using the Shapiro-Wilk test and homogeneity of variance across groups using the Levene-test. Since data fulfilled the criteria for parametric testing, a univariate analysis of variance (ANOVA) was computed, followed by a Post-Hoc-Tukey-Test with Bonferroni correction for multiple comparisons.
3 Results
3.1 Abiotic Habitat Variables
The three thermal regions W, C, and I differed significantly in mean temperature (Figure 3., Wilcoxon post-hoc test, p < 0.001), whereas other habitat variables such as pH, EC, O2, V, water depth, substratum composition and macrophytes coverage did not differ significantly between the three sites (Wilcoxon post-hoc test, p > 0.05). However, MP coverage in MP-dominated habitats was significantly higher in summer in all 3 TRs (Wilcoxon post-hoc test, p < 0.05). In addition, MP-dominated habitats comprised a higher share of sand and fines than GR-dominated habitats (Wilcoxon post-hoc test, p < 0.05).
3.2 Community Composition in Different Thermal Habitats
In total 10,956 individuals of 75 MIV taxa were identified, of which 43.7% individuals were collected in spring and 56.3% in summer. In the cold thermal region C (42.3%), the highest number of MIV-individuals was detected, followed by the warm thermal region W (32.8%) and the intermixed thermal region I (24.8%) (Table 3). In thermal region W, species richness was highest (overall 56 species) both in summer and spring, with 42 species detected in each season. In thermal region I, species richness was overall 54 species, with 36 species detected in each season. Thermal region C had the lowest species richness (overall 39 species) with 26 species detected in spring and 25 species in summer, resulting in significant differences between C and W in summer, and significant differences between all TR in spring (Wilcoxon post-hoc test, p < 0.05). Individual numbers of aquatic species which complete their full life cycle in aquatic habitats were detected with 39% in spring and 61% in summer with a 2.3 fold higher density in cold TR in summer compared to spring. In the warm TR only a slight increase of 1.4 fold in individual numbers was detectable. Individuals of semi-aquatic species such as Ephemeroptera, Trichoptera, and Plecoptera were caught 51% in spring and 49% in summer with 40% fewer individuals detected in the warm TR in summer compared to spring. This depletion was not evident in the cold TR where in summer 2.7 fold more individuals could be detected.
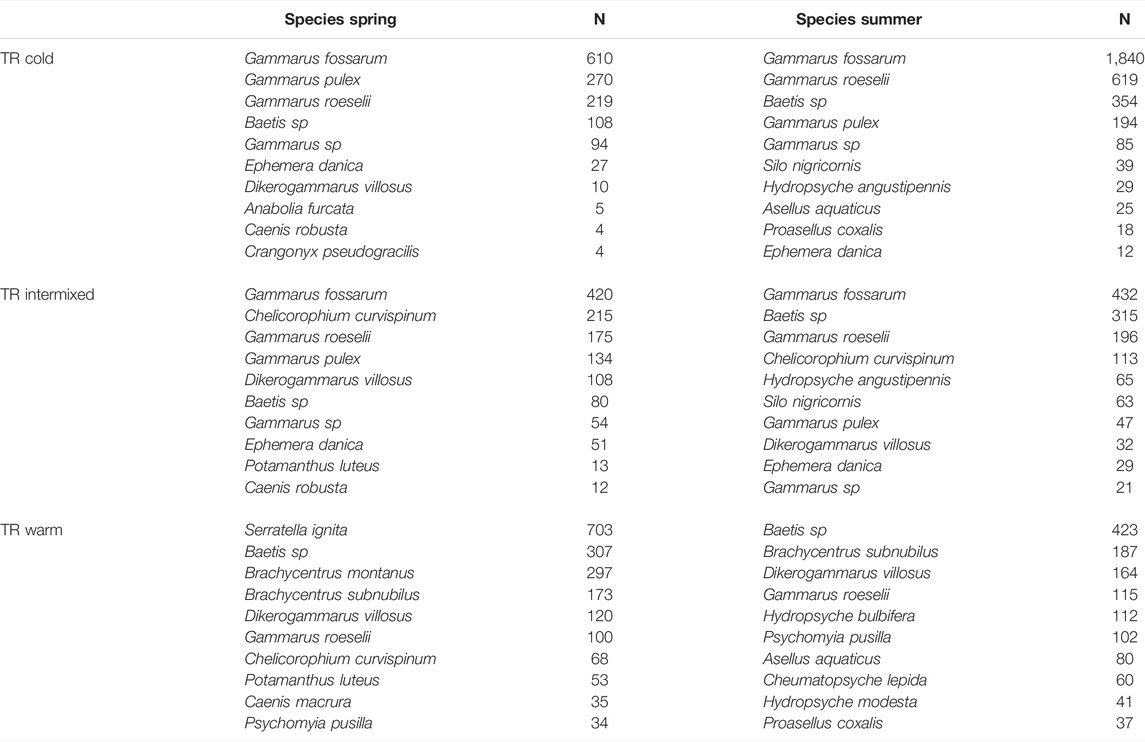
TABLE 3. Most abundant Species determined by thermal region (TR), and season spring and summer. N = number of detected indiciduals.
The most abundant taxa in the samples were amphipoda such as Gammarus fossarum (n total = 3329, In C n = 2450, in I n = 852, in W n = 27), Gammarus roeselii (n total = 1424, in C n = 838, in I 371, in W n = 215), Gammarus pulex (n total = 648, in C n = 464, in I n = 181, in W n = 3), Dikerogammarus villosus (n total = 445, in C n = 21, in I n = 140, in W n = 284) and mayflies such as Baetis spec. (n = 1587) and Serratella ignita (n = 718). Only Serratella ignita had a strong lifecycle induced seasonal occurrence with almost all individuals (703) found in the thermal region W in spring. However, amphipods at the thermal region C, such as Gammarus fossarum and Gammarus roeselii were more abundant (by a factor of 3) in summer than in spring (Table 3).
Shannon diversity was highest in the thermal regions W and I and lowest at C. Significant differences in Shannon diversity were found between the cold thermal region C and the other two thermal regions I and W, which was true for spring and summer. At thermal region C, significantly lower Eveness-values could be detected only in summer compared to I and W (Wilcoxon post-hoc test, p < 0.05, Figure 4).
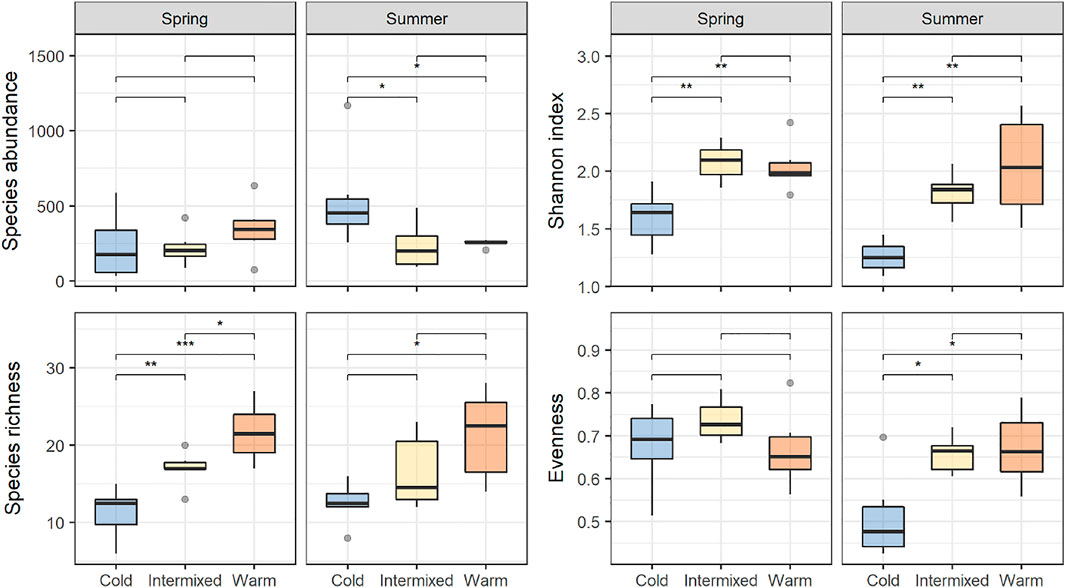
FIGURE 4. Species abundance and -richness, Shannon index and Evenness for each of the thermal regions, and their comparison between seasons. Asterisks indicate significant differences between treatments detected with Wilcoxson signed-ranks post hoc test as indicated by the bracket, * = p < 0.05, ** = p < 0.01, *** = p < 0.001.
Multivariate comparison of species community composition as illustrated in the NMDS (Figure 5) revealed significant differences between TRs, seasons and GR- or MP-dominated habitats (Table 4). The higher R-value detected by PERMANOVA for the between TR-comparison (R 0.26, p < 0.001) indicates a stronger separation of the cold thermal region C, intermixed I and warm W compared to the seasonal separation of TR (R 0.13, p < 0.001) and the MP- or GR-dominated habitats (R 0.05, p < 0.01) within TR. The ordination of TR was largely driven by a distinct set of species. The two gammarid species Gammarus fossarum and Gammarus pulex were more characteristic of the cold thermal region C, while the warm W was characterised by Dikerogammarus villosus and trichopterean species e.g., of the genus Hydropsyche or Brachycentrus (Figure 5, Table 5).
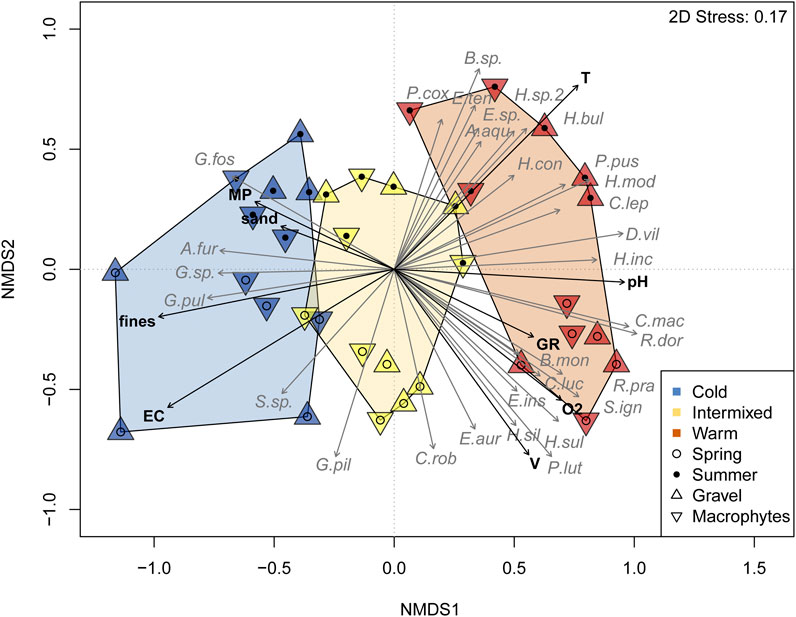
FIGURE 5. Non-metric multidimensional scaling of spring and summer sampling based on macroinvertebrate abundance data and Bray-Curtis similarity. 2D Stress = non-metric stress value after Kruskal. Thermal regions are displayed by color-filled polygons determined by grouping of sampling spots per habitat. Significant environmental variables (T = Temperature, pH, O2 = Oxygen concentration, V = flow velocity, EC= Electric conductivity, MP = Macrophyte habitat, GR = Gravel habitat, fines = fine sediment <0.85 mm, Sand 0.85–2 mm) and species (macroinvertebrates, abbreviations in Appx.1) are displayed as vectors.
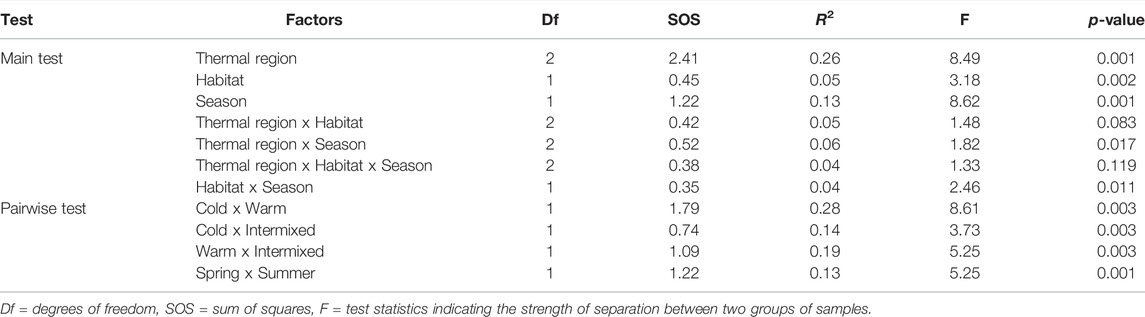
TABLE 4. Results of the multivariate analyses of overall macroinvertebrate community composition using PERMANOVA with pairwise comparisons.
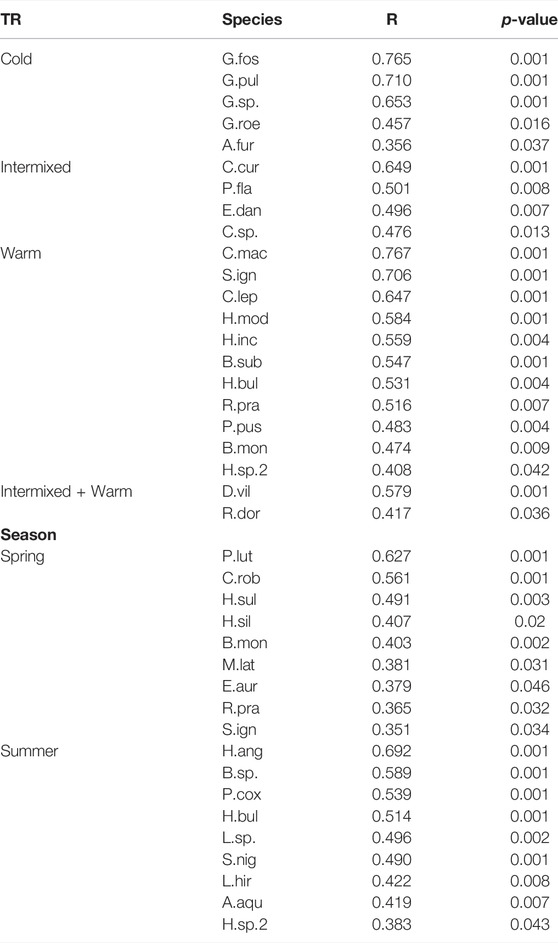
TABLE 5. Results of multilevel pattern analysis with species of significant abundances for a specific Thermal region (TR), habitat (macrophytes or gravel dominated) and season (spring or summer).
In addition to the differentiation of thermal regions by water temperature, the PERMANOVA also revealed a correlation for species abundances to spatial (habitat) and temporal (season) grouping by other environmental variables such as macrophytes, substratum composition (expressed as fines, sand or gravel) and chemical variables such as O2, pH, and EC. MP-dominated habitats were found to support gammarid populations in summer, particularly in C, whilst in less MP-dominated habitats Ephemeroptera and Trichoptera species were more abundant.
Furthermore, a different niche separation of species within families was detected. This was evident for Hydropsyche species, which, in addition to the differentiation of GR- and MP-dominated habitats, also showed an approximation to different environmental factors and seasons within the warm thermal region (Figure 5, Table 5).
All habitat variables correlated significantly with the arrangement of the data points representing the community composition in the NMDS (Figure 5). Positive correlations were found for EC, fines, sand and MP with the community composition in the thermal region C and Temperature, pH, GR, and O2 were positively correlated with the thermal region W.
3.3 Gammarids Survival During Thermal Cross-Exposure
After 71 days of exposure in the SEFLOBs, a total of 470 gammarid individuals were recovered from the initially placed 540 individuals in the cross-exposure experiment. After species determination and measurement of all individuals, a total of 157 individuals were analyzed, with 83 individuals of G. roeselii (overall mortality in treatments 71%) and 74 individuals of D. villosus (overall mortality in treatments 81%) (Post-Hoc-Tukey-Test, p < 0.05). Thermal region-specific patterns of survival were observed in general (both species pooled) with a significantly higher survival rate in thermal region C, compared to W (Figure 6, Post-Hoc-Tukey-Test, p < 0.05). At the species level, differences in survival were found in I and W compared to C. However, this result was only significant between TR, with the decrease of the number of individuals of D. villosus being more pronounced at the 10%-level (Post-Hoc-Tukey-Test, C:I p = 0.073; C:W p = 0.060). No significant differences between TR were found for G. roeselii, but a survival trend was found with most survivors in C, followed by I, and the least number of survivors in the warm thermal region W (Figure 6).
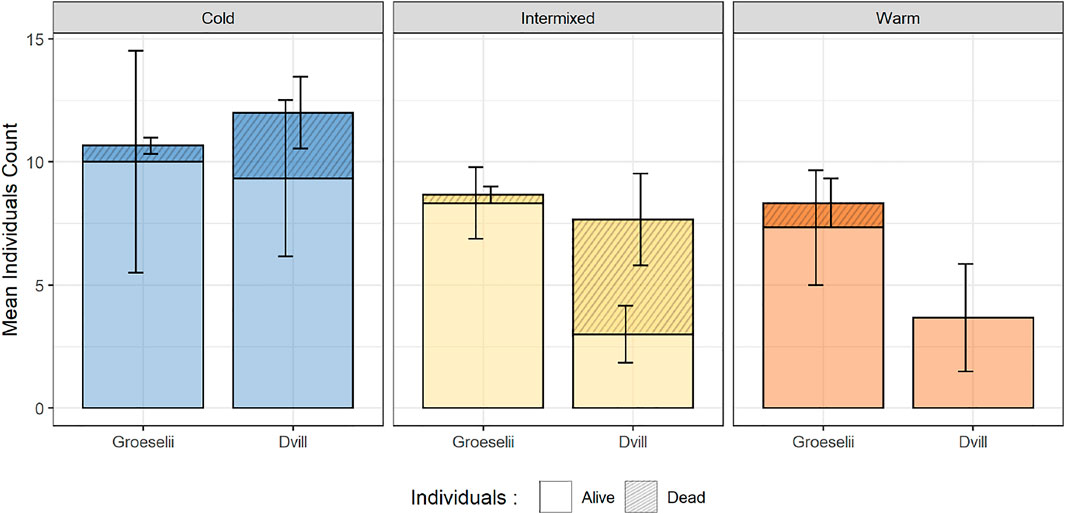
FIGURE 6. Alive (non-shaded) vs. dead (shaded) of each Groeselii = Gammarus roeselii and Dvill = Dikerogammarus villosus found at the end of the cross-exposure experiment in the exposed compartments of each thermal region.
4 Discussion
This study examined the influence of water temperature and other important habitat variables in warm, cold and intermixed thermal regions on the composition of the MIV communities and the presence and survival of native versus non-native gammarids. Although very much influenced by season and the presence of macrophytes or gravel as substratum, temperature most strongly affected MIV species composition. Indigenous gammarids preferred colder thermal regions, whilst the invasive one, D. villosus, was dominant in warmer thermal regions. These results indicate that during rising water temperatures, community composition can change irrespective of other factors which is in line with our hypothesis I. It is well known that macroinvertebrate community composition can widely be predicted based on structural habitat (Poff, 1997; Gore et al., 2001; Cortes et al., 2011) and that a high structural habitat heterogeneity, particularly characteristic of the study system, explains high species diversity (Pander et al., 2016; Pander et al., 2018). Our findings provide evidence that temperature is also a strong driver often impairing structural habitat quality, particularly affecting cold-stenothermic species negatively. This is indicated by the highest R-value detected by PERMANOVA compared to the seasonal and habitat (GR-vs. MP-dominated habitats) factors tested in each of the thermal regions. Additionally, the comparatively low individual numbers of indigenous gammarids and high numbers of the invasive D. villosus in warm thermal region W compared to cold C (where indigenous gammarids were more dominant) clearly mirror the temperature effect. Other factors such as O2, current speed, water depth, or substratum composition were less important in this study since they exerted similar effects throughout all thermal regions and were overall differentiated on a relatively low level.
We hypothesized (hypothesis II) that such differences in community composition will be most pronounced during the warmest season when maximum water temperatures and upper temperature tolerance thresholds of certain species are reached. Due to their evolutionary adaptation, species driving community changes at increased temperatures are often Mediterranean and Ponto-Caspian species which have already spread throughout the middle and northern regions of Europe (Hesselschwerdt and Wantzen, 2018). Some of them are potentially invasive, as evident for D. villosus in this study. D. villosus is known to have colonized all of the studied TRs since 2010, when a large-scale floodplain restoration project connected all aquatic habitats (Pander et al., 2016). In 2010, the species was detected in higher densities close to the source (main River Danube) in GR-dominated habitats with low-to-medium current speed. In contrast, currently, the species prefer MP-dominated habitats in the W thermal regions of the system. However, these habitats are also located close to the Danube River - from where this species originally invaded the system - excluding a potential distance to source effect as a driver of spatial distribution patterns (Pander et al., 2016; Pander et al., 2018). The MP-dominated habitats are characterized by less current speed resulting from the denser vegetation compared to habitats without macrophytes. The slower current results in increased fine sediment accumulation, which seem particularly attractive for D. villosus combined with high temperatures. However, since all TRs comprised habitats with and without macrophytes, this specific habitat structure does not play a major role in the composition of the gammarid community among the different TRs.
Either other gammarid species such as G. pulex or G. roeselii, which are known to also colonize MP-dominated habitats, are already above their preferred temperature range or unable to compete with D. villosus when temperatures are high and were therefore only recorded at low densities in warm TR. This explanation is not fully supported by the cross-exposure experiment that was used to test hypothesis III. Here, D. villosus as well as the tested indigenous gammarid species had highest survival rates in SEFLOBs exposed in the cold thermal region C. This indicates favorable conditions for both species there and it contrasts the situation found in the TR habitats where probably additional factors such as competition for food, microhabitat and predation play a role. Predation can be excluded in our study for both G. roeselii and D. villosus as well since they were locked in their compartments not affecting each other nor being affected from invaders outside of the compartments. We cannot exclude cannibalism, which is reported for D. villosus, however, we have no indication for this effect in our study. We neither could observe direct cannibalism during the SEFLOBs maintenance, nor did we find gnawed carcasses of D. villosus in the compartments. Alternatively, the mortality rate in cold water could be related to the fact that the specimens are less active and so their predatory behaviour is weak. Also, cold water has higher levels of oxygen saturation which may positively affect gammarid survival. Preference of D. villosus for cold water was to some extend also found in other studies, suggesting that this species can tolerate a wide range of temperatures (Wijnhoven et al., 2003) and that it can cope better with colder temperatures compared to other gammarids (e.g., Maazouzi et al., 2011). In contrast to these laboratory studies, both exposed gammarids herein seemed to persisted better in the SEFLOBs exposed in the cold thermal region, contrasting the results found in the natural habitats of the TRs. However, we have to state that overall mortality in the compartments was relatively high for both species. Findings generated in laboratory studies do not seem to be directly transferable to the natural environment where other factors such as direct and indirect competition, the availability of oxygen and shelter act together and may influence principle physiological tolerances of the competing species, potentially pushing indigenous species out of their preferred habitats. MP-dominated habitats in the thermal region C had a higher presence of indigenous gammarids as also detected in a study carried out at Lake Constance (Hesselschwerdt and Wantzen, 2018). This is in contrast with a laboratory study about the effects of direct interactions of invasive and non-invasive gammarids in combination with a fish predator. Beggel et al. (2016) demonstrated that D. villosus is more assertive when it comes to hiding in niches to avoid predation and we would therefore have expected that D. villosus would preferably colonize the macrophytic habitats since a high predation risk due to the rich fish population in the thermal regions is likely (Pander et al., 2015b). In the study herein, temperature in combination with structure may have affected competition between species, influencing indigenous species’ assertiveness in terms of greater competitive strength in cold and MP-dominated habitats.
Reaching thermal limits for macroinvertebrate communities can depend on different factors such as the initial species community, source habitats from which permanent colonization can be driven and the degree of temperature-related alteration these communities already experienced over time. Since it is known that the Danube system has been particularly affected by rising water temperatures for decades (Bonacci et al., 2008; Markovic et al., 2013), a warm-water adapted macroinvertebrate community could already be found in all TRs. This is evident from the absence of species favoring cold water conditions such as plecopterans of the genera Isoperla, which were quite regularly detected in that system until a decade ago (Pander et al., 2016; Pander et al., 2018). Such effects are potentially strongest during time periods when temperatures repeatedly exceed the thermal limits of cold-stenothermic species. This effect may be disguised in our dataset because several of those species have now disappeared from the system without the possibility of recolonization due to the general warming of the source habitats. However, our dataset still indicates a shift in the community composition in the warm TR where temperatures reached the detected maxima and resistant species of the genus Assellus and Proassellus along with D. villosus occurred in higher numbers compared to cooler time periods.
In light of the ongoing climate change with increasing mean temperatures and increasing numbers of extremes, it becomes obvious that novel MIV communities will arise, largely driven by species with higher temperature optima. In a globalized world, this can be dramatically boosted by the translocation of species from warmer regions, often happening accidentally and unnoticed (Walther et al., 2009; Brandner et al., 2015; Ricciardi et al., 2021), resulting in novel communities being permanently invaded and fast-changing (Rodriguez, 2006; Walther et al., 2009; Belnap et al., 2012). Up to now, it is not clear to which extent indigenous highly specialized species can cope with these temperature changes and ongoing invasions.
5 Conclusion
This study provides evidence that regional thermal effects on MIV community composition may exceed seasonal or meso-habitat effects, commonly known to govern such composition. Specifically, rising water temperatures have the potential to favour invasive D. villosus, with higher thermal tolerances. In light of climate change, our findings indicate that an impairment of habitat quality by increased temperatures may provide competitive advantage for invasive macroinvertebrate species. Consideration of shifts in community composition related to temperature needs to become integrated with biological response patterns related to other, better-characterized stressors such as morphological and flow degradation, chemical pollution and fine sediment. Such an approach is crucial for effective conservation and restoration of native biodiversity and for a realistic prediction of the ability to reach policy target settings for aquatic ecosystems.
Data Availability Statement
The original contributions presented in the study are included in the article/Supplementary Material, further inquiries can be directed to the corresponding author.
Author Contributions
All authors have read and agreed to the published version of the manuscript. Individual contributions are as follows: Conceptualization, JP, LH, RC-M and JG, methodology, JP, LH and RC-M; formal analysis, JP and LH; writing—original draft preparation, JP, LH and RC-M; editing and artwork JP and LH, review JG; project administration, JP and JG; resources, JG and RC-M; funding acquisition, RC-M, JG.
Funding
This research was partly funded by the Alexander von Humboldt Foundation through a fellowship awarded to RC‐M, carried out at TUM, and a grant financed by the HIT-Umweltstiftung. This study was also partly supported by the framework of the project AquaKlif in the bayklif network for investigation of regional climate change funded by the Bavarian State Ministry of Science and the Arts. The funders did not have any influence on the study design and the interpretation of results.
Conflict of Interest
The authors declare that the research was conducted in the absence of any commercial or financial relationships that could be construed as a potential conflict of interest.
Publisher’s Note
All claims expressed in this article are solely those of the authors and do not necessarily represent those of their affiliated organizations, or those of the publisher, the editors and the reviewers. Any product that may be evaluated in this article, or claim that may be made by its manufacturer, is not guaranteed or endorsed by the publisher.
Acknowledgments
We thank the Auen Zentrum Neuburg-Ingolstadt for coordinating the permissions with the land owner and the local authorities to carry out the study.
Supplementary Material
The Supplementary Material for this article can be found online at: https://www.frontiersin.org/articles/10.3389/fenvs.2022.869396/full#supplementary-material
References
Anderson, M. J. (2001). A New Method for Non-parametric Multivariate Analysis of Variance. Austral Ecol. 26 (1), 32–46. doi:10.1111/j.1442-9993.2001.01070.pp.x
Bayerisches Landesamt für Umwelt (2020). Abfluss Neuburg/Donau: Hochwassernachrichtendienst Bayern. Available at: https://www.hnd.bayern.de/pegel/donau_bis_kelheim/neuburg-10043708/abfluss?begin=25.05.2020&end=07.08.2020&addhr=false (Accessed February 2nd, 2022).
Beggel, S., Brandner, J., Cerwenka, A. F., and Geist, J. (2016). Synergistic Impacts by an Invasive Amphipod and an Invasive Fish Explain Native Gammarid Extinction. BMC Ecol. 16 (1), 1–13. doi:10.1186/s12898-016-0088-6
Belnap, J. y. e., Ludwig, J. A., Wilcox, B. P., Betancourt, J. L., Dean, W. R. J., Hoffmann, B. D., et al. (2012). Introduced and Invasive Species in Novel Rangeland Ecosystems: Friends or Foes? Rangeland Ecol. Manage. 65 (6), 569–578. doi:10.2111/REM-D-11-00157.1
Boeker, C., and Geist, J. (2015). Effects of Invasive and Indigenous Amphipods on Physico-Chemical and Microbial Properties in Freshwater Substrates. Aquat. Ecol. 49, 467–480. doi:10.1007/s10452-015-9539-y
Bonacci, O., Trninić, D., and Roje-Bonacci, T. (2008). Analysis of the Water Temperature Regime of the Danube and its Tributaries in Croatia. Hydrol. Process. 22 (7), 1014–1021. doi:10.1002/hyp.6975
Brandner, J., Auerswald, K., Schäufele, R., Cerwenka, A. F., and Geist, J. (2015). Isotope Evidence for Preferential Dispersal of Fast-Spreading Invasive Gobies along Man-Made River Bank Structures. Isotopes Environ. Health Stud. 51 (1), 80–92. doi:10.1080/10256016.2014.993978
Bruijs, M. C. M., Kelleher, B., van der Velde, G., and Bij de Vaate, A. (2001). Oxygen Consumption, Temperature and Salinity Tolerance of the Invasive Amphipod Dikerogammarus Villosus: Indicators of Further Dispersal via Ballast Water Transport. Archiv fur Hydrobiol. 152, 633–646. doi:10.1127/archiv-hydrobiol/152/2001/633
Cáceres, M. D., and Legendre, P. (2009). Associations between Species and Groups of Sites: Indices and Statistical Inference. Ecology 90 (12), 3566–3574. doi:10.1890/08-1823.1
Cortes, R. M., Varandas, S., Teixeira, A., Hughes, S. J., Magalhães, M., Barquín, J., et al. (2011). Effects of Landscape Metrics and Land-Use Variables on Macroinvertebrate Communities and Habitat Characteristics. Limnetica 30 (2), 347–362. doi:10.23818/limn.30.25
Cottin, D., Roussel, D., Foucreau, N., Hervant, F., and Piscart, C. (2012). Disentangling the Effects of Local and Regional Factors on the thermal Tolerance of Freshwater Crustaceans. Naturwissenschaften 99 (4), 259–264. doi:10.1007/s00114-012-0894-4
Daufresne, M., Roger, M. C., Capra, H., and Lamouroux, N. (2004). Long-term Changes within the Invertebrate and Fish Communities of the Upper Rhône River: Effects of Climatic Factors. Glob. Change Biol. 10 (1), 124–140. doi:10.1046/j.1529-8817.2003.00720.x
Dick, J. T. A., and Platvoet, D. (2000). Invading Predatory Crustacean Dikerogammarus Villosus Eliminates Both Native and Exotic Species. Proc. R. Soc. Lond. B 267 (1447), 977–983. doi:10.1098/rspb.2000.1099
Durance, I., and Ormerod, S. J. (2009). Trends in Water Quality and Discharge Confound Long-Term Warming Effects on River Macroinvertebrates. Freshw. Biol. 54 (2), 388–405. doi:10.1111/j.1365-2427.2008.02112.x
Eggers, T. O., and Martens, A. (2001). Bestimmungsschlüssel der Süßwasser-Amphipoda (Crustacea) Deutschlands: A key to the freshwater Amphipoda (Crustacea) of Germany. Lauterbornia 42, 1–68. Available at: https://www.zobodat.at/pdf/Lauterbornia_2001_42_0001-0068.pdf (Accessed February 2nd, 2022).
Eiseler, B. (2010). Bestimmungshilfen‐Makrozoobenthos (1). Taxonomie für die Praxis, LANUV-Arbeitsblatt 14. Recklinghausen, Germany. Available at: https://www.lanuv.nrw.de/fileadmin/lanuvpubl/4_arbeitsblaetter/40014.pdf (Accessed February 2nd, 2022).
Fischer, P., and Cyffka, B. (2014). Floodplain Restoration on the Upper Danube by Re-establishing Back Water Dynamics: First Results of the Hydrological Monitoring. Erdkunde 68 (1), 3–18. doi:10.3112/erdkunde.2014.01.02
Fullerton, A. H., Torgersen, C. E., Lawler, J. J., Steel, E. A., Ebersole, J. L., and Lee, S. Y. (2018). Longitudinal thermal Heterogeneity in Rivers and Refugia for coldwater Species: Effects of Scale and Climate Change. Aquat. Sci. 80 (3), 1–15. doi:10.1007/s00027-017-0557-9
Gore, J. A., Layzer, J. B., and Mead, J. (2001). Macroinvertebrate Instream Flow Studies after 20 years: a Role in Stream Management and Restoration. Regul. Rivers: Res. Mgmt. 17 (4‐5), 527–542. doi:10.1002/rrr.650
Grabowski, M., Bacela, K., and Konopacka, A. (2007a). How to Be an Invasive Gammarid (Amphipoda: Gammaroidea)-comparison of Life History Traits. Hydrobiologia 590 (1), 75–84. doi:10.1007/s10750-007-0759-6
Grabowski, M., Jazdzewski, K., and Konopacka, A. (2007b). Alien Crustacea in Polish Waters - Amphipoda. Aquat. Inv. 2 (1), 25–38. doi:10.3391/ai.2007.2.1.3
Grabowski, M., Mamos, T., Bącela-Spychalska, K., Rewicz, T., and Wattier, R. A. (2017). Neogene Paleogeography Provides Context for Understanding the Origin and Spatial Distribution of Cryptic Diversity in a Widespread Balkan Freshwater Amphipod. PeerJ 5, e3016. doi:10.7717/peerj.3016
Haase, P., and Sundermann, A. (2004). Standardisierung der Erfassungs-und Auswertungsmethoden von Makrozoobenthosuntersuchungen in Fließgewässern: Abschlussbericht 2. Projektjahr. Forschungsinstitut Senckenberg: Biebergmünd.
Haidekker, A., and Hering, D. (2008). Relationship between Benthic Insects (Ephemeroptera, Plecoptera, Coleoptera, Trichoptera) and Temperature in Small and Medium-Sized Streams in Germany: A Multivariate Study. Aqua. Ecol. 42 (3), 463–481. doi:10.1007/s10452-007-9097-z
Halle, M., Müller, A., and Sundermann, A. (2016). Ableitung von Temperaturpräferenzen des Makrozoobenthos für die Entwicklung eines Verfahrens zur Indikation biozönotischer Wirkungen des Klimawandels in Fließgewässern. KLIWA-Berichte: Heft 20. Karlsruhe, Germany: Arbeitskreis KLIWA. Available at: https://www.kliwa.de/_download/KLIWAHeft20.pdf (Accessed February 2nd, 2022).
Hauer, F. R., and Lamberti, G. A. (2017). “Macroinvertebrates,” in Methods in Stream Ecology: Volume 1: Ecosystem Structure. Editors F. R. Hauer, and G. A. Lamberti (Vienna, Austria: Academic Press), 297–319.
Hesselschwerdt, J., and Wantzen, K. M. (2018). Global Warming May Lower thermal Barriers against Invasive Species in Freshwater Ecosystems - A Study from Lake Constance. Sci. Total Environ. 645, 44–50. doi:10.1016/j.scitotenv.2018.07.078
Huryn, A. D. (1998). Ecosystem-level Evidence for Top-Down and Bottom-Up Control of Production in a Grassland Stream System. Oecologia 115 (1-2), 173–183. doi:10.1007/s004420050505
Karaman, G. S., and Pinkster, S. (1977). Freshwater Gammarus Species from Europe, North Africa and Adjacent Regions of Asia (Crustacea-Amphipoda). Bijdragen Tot De Dierkunde 47, 165–196. doi:10.1163/26660644-04702003
Kolding, S., and Fenchel, T. M. (1979). Coexistence and Life Cycle Characteristics of Five Species of the Amphipod Genus Gammarus. Oikos 33, 323–327. doi:10.2307/3544009
Kuçi, R., Ibrahimi, H., and Gashi, A. (2017). First Record of Gammarus Roeselii Gervais, 1835 (Amphipoda: Gammaridae) from Kosovo with Ecological Notes. Natura Croatica 26 (2), 215–223. doi:10.20302/NC.2017.26.18
Kuhn, J., Casas-Mulet, R., Pander, J., and Geist, J. (2021). Assessing Stream Thermal Heterogeneity and Cold-Water Patches from UAV-Based Imagery: A Matter of Classification Methods and Metrics. Remote Sens. 13, 1379. doi:10.3390/rs13071379
Landesamt für Digitalisierung, Breitband und Vermessung (2020). GeodatenOnline: Digitales Orthophoto DOP80 (WMS). Available at: https://geoservices.bayern.de/wms/v2/ogc_dop80_oa.cgi? (Accessed February 2, 2022).
Lechthaler, W. (2009). Macrozoobenthos - Key to Families of Macroinvertebrates in European Freshwaters. Computer Software. Vienna, Austria. Available at: www.eutaxa.com (Accessed February 2nd, 2022).
Leigh, C., Bush, A., Harrison, E. T., Ho, S. S., Luke, L., Rolls, R. J., et al. (2015). Ecological Effects of Extreme Climatic Events on Riverine Ecosystems: Insights from Australia. Freshw. Biol. 60 (12), 2620–2638. doi:10.1111/fwb.12515
Lento, J., Culp, J. M., Levenstein, B., Aroviita, J., Baturina, M. A., Bogan, D., et al. (2021). Temperature and Spatial Connectivity Drive Patterns in Freshwater Macroinvertebrate Diversity across the Arctic. Freshw. Biol. 67, 159–175. doi:10.1111/fwb.13805
Liu, S., Xie, Z., Liu, B., Wang, Y., Gao, J., Zeng, Y., et al. (2020). Global River Water Warming Due to Climate Change and Anthropogenic Heat Emission. Glob. Planet. Change 193, 103289. doi:10.1016/j.gloplacha.2020.103289
Maazouzi, C., Piscart, C., Legier, F., and Hervant, F. (2011). Ecophysiological Responses to Temperature of the "killer Shrimp" Dikerogammarus Villosus: Is the Invader Really Stronger Than the Native Gammarus Pulex? Comp. Biochem. Physiol. A: Mol. Integr. Physiol. 159 (3), 268–274. doi:10.1016/j.cbpa.2011.03.019
Maltby, L., Clayton, S. A., Wood, R. M., and McLoughlin, N. (2002). Evaluation of the Gammarus Pulex In Situ Feeding Assay as a Biomonitor of Water Quality: Robustness, Responsiveness, and Relevance. Environ. Toxicol. Chem. 21 (2), 361–368. doi:10.1897/1551-5028(2002)021<0361:eotgpi>2.0.co;2
Markovic, D., Scharfenberger, U., Schmutz, S., Pletterbauer, F., and Wolter, C. (2013). Variability and Alterations of Water Temperatures across the Elbe and Danube River Basins. Climatic Change 119 (2), 375–389. doi:10.1007/s10584-013-0725-4
Martinez Arbizu, P. (2020). pairwiseAdonis (Version R Package Version 0.4. Computer Software. Available at: https://github.com/pmartinezarbizu/pairwiseAdonis (Accessed February 2nd, 2022).
Mauchart, P., Czirok, A., Horvai, V., Herczeg, R., Móra, A., and Csabai, Z. (2017). Effects of Meso- and Microhabitat Characteristics on the Coexistence of Two Native Gammarid Species (Crustacea, Gammaridae). Int. Rev. Hydrobiol. 102 (1-2), 38–46. doi:10.1002/iroh.201601855
Müller, J. (2001). Invasion History and Genetic Population Structure of Riverine Macroinvertebrates. Zoology 104 (3-4), 346–355. doi:10.1078/0944-2006-00040
Mueller, M., Pander, J., and Geist, J. (2011). The Effects of Weirs on Structural Stream Habitat and Biological Communities. J. Appl.Ecol. 48 (6), 1450–1461. doi:10.1111/J.1365-2664.2011.02035.X
Niedrist, G. H., and Füreder, L. (2020). Real‐time Warming of Alpine Streams: (Re)defining Invertebrates' Temperature Preferences. River Res. Applic. 37 (2), 283–293. doi:10.1002/rra.3638
O'Reilly, C. M., Sharma, S., Gray, D. K., Hampton, S. E., Read, J. S., Rowley, R. J., et al. (2015). Rapid and Highly Variable Warming of lake Surface Waters Around the globe. Geophys. Res. Lett. 42 (24), 10773. doi:10.1002/2015gl066235
Pander, J., and Geist, J. (2010). Salmonid-egg Floating Boxes as Bioindication for Riverine Water Quality and Stocking success. J. Fish. Biol. 76 (10), 2584–2590. doi:10.1111/j.1095-8649.2010.02645.x
Pander, J., Mueller, M., and Geist, J. (2015a). A Comparison of Four Stream Substratum Restoration Techniques Concerning Interstitial Conditions and Downstream Effects. River Res. Applic. 31 (2), 239–255. doi:10.1002/rra.2732
Pander, J., Mueller, M., and Geist, J. (2015b). Succession of Fish Diversity after Reconnecting a Large Floodplain to the Upper Danube River. Ecol. Eng. 75, 41–50. doi:10.1016/j.ecoleng.2014.11.011
Pander, J., Mueller, M., Sacher, M., and Geist, J. (2016). The Role of Life History Traits and Habitat Characteristics in the Colonisation of a Secondary Floodplain by Neobiota and Indigenous Macroinvertebrate Species. Hydrobiologia 772 (1), 229–245. doi:10.1007/s10750-016-2667-0
Pander, J., Mueller, M., and Geist, J. (2018). Habitat Diversity and Connectivity Govern the Conservation Value of Restored Aquatic Floodplain Habitats. Biol. Conserv. 217, 1–10. doi:10.1016/j.biocon.2017.10.024
Piatka, D. R., Wild, R., Hartmann, J., Kaule, R., Kaule, L., Gilfedder, B., et al. (2021). Transfer and Transformations of Oxygen in Rivers as Catchment Reflectors of continental Landscapes: A Review. Earth-Sci. Rev. 220, 103729. doi:10.1016/j.earscirev.2021.103729
Pöckl, M. (1992). Effects of Temperature, Age and Body Size on Moulting and Growth in the Freshwater Amphipods Gammarus Fossarum and G. Roeseli. Freshw. Biol. 27 (2), 211–225. doi:10.1111/j.1365-2427.1992.tb00534.x
Pöckl, M. (2007). Strategies of a Successful New Invader in European Fresh Waters: Fecundity and Reproductive Potential of the Ponto-Caspian Amphipod Dikerogammarus Villosus in the Austrian Danube, Compared with the Indigenous Gammarus Fossarum and G. Roeseli. Freshw. Biol. 52 (1), 50–63. doi:10.1111/j.1365-2427.2006.01671.x
Poff, N. L. (1997). Landscape Filters and Species Traits: towards Mechanistic Understanding and Prediction in Stream Ecology. J. North Am. Benthol. Soc. 16 (2), 391–409. doi:10.2307/1468026
R Core Team (2020). R: A Language and Environment for Statistical Computing (Version 4.0.3) [Computer Software]. Wien: R Foundation for Statistical Computing. Available at: http://www.r-project.org/
Rahel, F. J., and Olden, J. D. (2008). Assessing the Effects of Climate Change on Aquatic Invasive Species. Conserv. Biol. 22 (3), 521–533. doi:10.1111/j.1523-1739.2008.00950.x
Rewicz, T., Wattier, R., Grabowski, M., Rigaud, T., and Bącela-Spychalska, K. (2015). Out of the Black Sea: Phylogeography of the Invasive Killer Shrimp Dikerogammarus Villosus across Europe. PLoS One 10 (2), e0118121. doi:10.1371/journal.pone.0118121
Ricciardi, A., Iacarella, J. C., Aldridge, D. C., Blackburn, T. M., Carlton, J. T., Catford, J. A., et al. (2021). Four Priority Areas to advance Invasion Science in the Face of Rapid Environmental Change. Environ. Rev. 29 (2), 119–141. doi:10.1139/er-2020-0088
Rodriguez, L. F. (2006). Can Invasive Species Facilitate Native Species? Evidence of How, when, and Why These Impacts Occur. Biol. Invasions 8 (4), 927–939. doi:10.1007/s10530-005-5103-3
Ruetz, C. R., Newman, R. M., and Vondracek, B. (2002). Top-Down Control in a Detritus-Based Food Web: Fish, Shredders, and Leaf Breakdown. Oecologia 132 (2), 307–315. doi:10.1007/s00442-002-0953-1
Schmedtje, U., and Kohmann, F. (1992). Bestimmungsschlüssel für die Saprobier-DIN-Arten (Makroorganismen). Informationsberichte des Bayerischen Landesamtes für Wasserwirtschaft 2, 88.
Schneider, C., Laizé, C. L. R., Acreman, M. C., and Flörke, M. (2013). How Will Climate Change Modify River Flow Regimes in Europe? Hydrol. Earth Syst. Sci. 17 (1), 325–339. doi:10.5194/hess-17-325-2013
Smialek, N., Pander, J., and Geist, J. (2021). Environmental Threats and Conservation Implications for Atlantic salmon and Brown trout during Their Critical Freshwater Phases of Spawning, Egg Development and Juvenile Emergence. Fish. Manag. Ecol. 28, 437–467. doi:10.1111/fme.12507
Stammel, B., Cyffka, B., Geist, J., Müller, M., Pander, J., Blasch, G., et al. (2012). Floodplain Restoration on the Upper Danube (Germany) by Re-establishing Water and Sediment Dynamics: a Scientific Monitoring as Part of the Implementation. River Sys. 20 (1-2), 55–70. doi:10.1127/1868-5749/2011/020-0033
Surber, E. W. (1937). Rainbow Trout and Bottom Fauna Production in One Mile of Stream. Trans. Am. Fish. Soc. 66 (1), 193–202. doi:10.1577/1548-8659(1936)66[193:rtabfp]2.0.co;2
Sutcliffe, D. W. (1992). Reproduction in Gammarus (Crustacea, Amphipoda): Basic Processes. Freshw. Forum 2 (2), 102–128.
van Vliet, M. T. H., Franssen, W. H. P., Yearsley, J. R., Ludwig, F., Haddeland, I., Lettenmaier, D. P., et al. (2013). Global River Discharge and Water Temperature under Climate Change. Glob. Environ. Change 23, 450–464. doi:10.1016/j.gloenvcha.2012.11.002
Wallace, J. B., and Webster, J. R. (1996). The Role of Macroinvertebrates in Stream Ecosystem Function. Annu. Rev. Entomol. 41 (1), 115–139. doi:10.1146/annurev.en.41.010196.000555
Walther, G.-R., Roques, A., Hulme, P. E., Sykes, M. T., Pyšek, P., Kühn, I., et al. (2009). Alien Species in a Warmer World: Risks and Opportunities. Trends Ecol. Evol. 24 (12), 686–693. doi:10.1016/j.tree.2009.06.008
Wanders, N., Vliet, M. T. H., Wada, Y., Bierkens, M. F. P., and Beek, L. P. H. (2019). High‐Resolution Global Water Temperature Modeling. Water Resour. Res. 55, 2760–2778. doi:10.1029/2018WR023250
Wijnhoven, S., van Riel, M., and van der Velde, G. (2003). Exotic and Indigenous Freshwater Gammarid Species: Physiological Tolerance to Water Temperature in Relation to Ionic Content of the Water. Aquat. Ecol. 37 (2), 151–158. doi:10.1023/A:1023982200529
Keywords: climate change, spatio-temporal thermal variability, water temperature, invasive species, gammarids, thermal heterogeneity
Citation: Pander J, Habersetzer L, Casas-Mulet R and Geist J (2022) Effects of Stream Thermal Variability on Macroinvertebrate Community: Emphasis on Native Versus Non-Native Gammarid Species. Front. Environ. Sci. 10:869396. doi: 10.3389/fenvs.2022.869396
Received: 04 February 2022; Accepted: 21 March 2022;
Published: 13 April 2022.
Edited by:
Iseult Lynch, University of Birmingham, United KingdomReviewed by:
Daniele Paganelli, University of Pavia, ItalyŁukasz Jermacz, Nicolaus Copernicus University in Toruń, Poland
Yixin Zhang, Soochow University, China
Copyright © 2022 Pander, Habersetzer, Casas-Mulet and Geist. This is an open-access article distributed under the terms of the Creative Commons Attribution License (CC BY). The use, distribution or reproduction in other forums is permitted, provided the original author(s) and the copyright owner(s) are credited and that the original publication in this journal is cited, in accordance with accepted academic practice. No use, distribution or reproduction is permitted which does not comply with these terms.
*Correspondence: Juergen Geist, Z2Vpc3RAdHVtLmRl
†These authors share first authorship