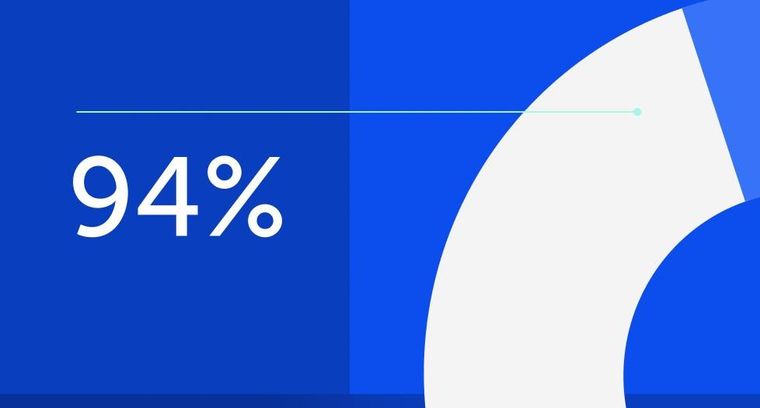
94% of researchers rate our articles as excellent or good
Learn more about the work of our research integrity team to safeguard the quality of each article we publish.
Find out more
REVIEW article
Front. Environ. Sci., 29 April 2022
Sec. Water and Wastewater Management
Volume 10 - 2022 | https://doi.org/10.3389/fenvs.2022.864894
This article is part of the Research TopicThe Detection of Emerging Pollutants by New or Alternative Analytical MethodsView all 4 articles
PFAS are a very diverse group of anthropogenic chemicals used in various consumer and industrial products. The properties that characterize are their low degradability as well as their resistance to water, oil and heat. This results in their high persistence in the environment and bioaccumulation in different organisms, causing many adverse effects on the environment as well as in human health. Some of their effects remain unknown to this day. As there are thousands of registered PFAS, it is difficult to apply traditional technologies for an efficient removal and detection for all. This has made it difficult for wastewater treatment plants to remove or degrade PFAS before discharging the effluents into the environment. Also, monitoring these contaminants depends mostly on chromatography-based methods, which require expensive equipment and consumables, making it difficult to detect PFAS in the environment. The detection of PFAS in the environment, and the development of technologies to be implemented in tertiary treatment of wastewater treatment plants are topics of high concern. This study focuses on analyzing and discussing the mechanisms of occurrence, migration, transformation, and fate of PFAS in the environment, as well the main adverse effects in the environment and human health. The following work reviews the recent advances in the development of PFAS detection technologies (biosensors, electrochemical sensors, microfluidic devices), and removal/degradation methods (electrochemical degradation, enzymatic transformation, advanced oxidation, photocatalytic degradation). Understanding the risks to public health and identifying the routes of production, transportation, exposure to PFAS is extremely important to implement regulations for the detection and removal of PFAS in wastewater and the environment.
Polyfluoroalkyl and perfluoroalkyl substances (PFAS) are large family of organofluorinated compounds that contain carbon chains with stable carbon-fluorine (C-F) bonds, and they have been gaining attention in recent years due to their toxicity. The PFAS family is estimated to exceed 4,000 synthetic fluorinated compounds (Bolan et al., 2021) with Perfluorooctanesulfonate (PFOS) and perfluorooctanoic acid (PFOA) being the most frequently encountered PFAS (Ateia et al., 2019; Singh et al., 2019; Cui et al., 2020; Stoiber et al., 2020). PFAS can be classified by their polar group, a group that gives PFAS high solubility in water and is non-volatile. PFAS contain at least one perfluoroalkyl moiety in the chemical structure, including groups of perfluorinated phosphonic acids (PFPA), perfluorinated carboxylic acids (PFCA) and perfluorinated carboxylic acids (PFCA), while polyfluorinated groups include sulfonic acids, fluorotelomers (FTS), polyfluorinated alkyl phosphates (PAP), fluorotelomer alcohols (FTOH), perfluorooctane sulfonamine (PFOSA) and their derivatives, Figure 1. Anionic PFAS with sulfonate or carboxylate polar groups are the group with the greatest focus from public and regulatory entities, however, PFAS with cationic and zwitterionic polar groups are also beginning to generate environmental concern.
FIGURE 1. Polyfluoroalkyl and perfluoroalkyl substances (PFAS), divided into polymeric and nonpolymeric compounds. Polymers include polytetrafluoroethylene (PTFE) and Viton. Non polymers include 8:2 Fluorotelomer alcohol (FTOH), Perfluorooctanoic acid (PFOA), Perfluorooctanesulfonic acid (PFOS), 6:2 Flourotelomer sulfonate (FTSA), Chlorinated polyfluorinated ether sulfonate (F53-B), and 6:2 Flourotelomer sulfonamide alkyletaine (FTAB). Created with BioRender.com.
They have a high thermal and chemical stability due to their carbon fluorine bonds and may possess hydrophobic or lipophobic moieties making them impermeable to water and oil and resistant to friction. Thanks to these advantageous properties, they have an extended life and durability (Lenka et al., 2021). PFAS have been widely used in industrial and commercial applications since the 1940s with some of their uses including fire-fighting foams as surfactants, waterproofing treatment for textiles, personal care products, cleaning products, food packaging, photos films, and many automotive, medical, and aerospace products.
Their strong C-F bonds, their possession of a fluoroalkyl tail, high chemical, and thermal stability, as well as their resistance to chemical and biological degradation also makes them hard to break down and extremely persistent in the environment (Ateia et al., 2019; Gagliano et al., 2020; Zhang et al., 2022). PFAS high persistence, widespread use and bioaccumulation potential has resulted in an environmental problem of high global concern, derived from the rapid distribution of these compounds in surface and groundwater, and many drinking water supplies in concentrations reported as highly toxic for different ecosystems and human health (Singh et al., 2019). The United States Environmental Protection Agency (EPA) recommends that PFOS and PFOA do not exceed 70 parts per trillion (70 ng L−1), while the European Union introduced drinking water regulations at parts per billion levels (Gobelius et al., 2018). The monitoring of PFAS in the environment is essential to protect human health and safety. In recent years biotechnology has made exponential progress in the development of new technologies for PFAS sensors, allowing the effective in field monitoring at lower costs in comparison to standard technologies such as HPLC (Menger et al., 2021). Conventional wastewater treatment processes in wastewater treatment plants (WWTPs) are ineffective at degrading emerging pollutants such as PFAS, mainly due to their low concentrations and high hydrophilicity. The mostly commonly used processes are anaerobic or aerobic biological treatments only break carbon chains (C-C), producing short-chain PFAS. This often results in higher concentrations of PFAS in treated water than untreated water (Gagliano et al., 2020). PFAS are also resistant to other common WWTP processes such as ultraviolet radiation, disinfection with free chlorine, oxidation by ozone and hydroxyl free radicals. They show some degradation with advanced oxidation but often degrade into shorter chain PFAS (Nzeribe et al., 2019). Adsorption, high pressure nanofiltration and reverse osmosis membrane processes have shown substantial effectiveness in removing PFAS (Kucharzyk et al., 2017). However, there are other problems inherent to the removal of PFAS by the most advanced techniques, which are the influence of the water matrix and its frequent regeneration, and the removal of concentrates with a high content of PFAS (Gagliano et al., 2020).
The review highlights the environmental problem posed by PFAS, focusing on their sources, occurrence and transport in water, their negative effects on human health as well as in the environment, the most recent technologies for the detection and degradation of PFAS, as well as their regulations of use, release, and monitoring in the environment.
PFAS are a class of synthetic organic chemicals containing fluorinated carbon chains with different functional groups initially long chain and more recently, short chain PFAS have been developed (Kwiatkowski et al., 2020). Some of the functional groups include alcohols (-OH), amines (-NH2), halogens (-F, -Cl, -Br, -I), esters (-CO2R), ethers (-O-), thiols (-SH), carboxylic acids (-CO2H), sulfonic acids (-SO3H), sulfinic acids (-SO2H), sulfones (-SO2R), phosphate acids (-PO(OH)2), phosphinic acids (-PO(OH)), polymers, salts and derivatives. In the past decade, the number of PFAS has increased considerably due to its extensive applications. From 2015 to 2018 the number increased from 3,000 to 4,700 registered PFAS (KemI, 2015; OECD, 2018). Most PFAS substances have only one identified application or have been patented for only one use. Many potential uses of different PFAS have not been explored yet. Contrary to single-use substances, some PFAS have many applications associated with them (Glüge et al., 2020). Figure 2 shows 12 PFAS that are assigned 10 or more uses.
FIGURE 2. Structures of the PFAS with more than 10 assigned uses: 1 CAS No. 3825–26–1, 2 CAS No. 2795–39–3, 3 CAS No. 2991–51–7, 4 CAS No. 38006–74–5, 5 CAS No. 29117–08–6, 6 CAS No. 163702–07–6, 7 CAS No. 364–33–6, 8 CAS No. 138495–42–8, 9 CAS No. 375–03–1, 10 CAS No. 24937–79–9, 11 CAS No. 9002–84–0 and 12 CAS No. 25–038–71–5. Adapted from (Glüge et al., 2020).
The PFAS lifecycle consists of four steps: PFAS production, product manufacture, product use and waste management. In each step PFAS are released to the environment (Wahlström et al., 2021). Recent works have highlighted the mechanisms of environmental occurrence and transport by which they reach different water bodies causing pollution and health associated risks. PFAS reach most freshwater bodies, such as rivers, lakes and groundwater (Guelfo et al., 2018; Chambers et al., 2021).
The production of PFAS starts with the mining of Fluorspar. In 2017, 63% of this material was extracted from China and 26% from Mexico (Raichl and Schatz, 2019) causing associated problems elsewhere (Jha and Tripathi, 2021; Solanki et al., 2022). The mineral processing needed to generate PFAS is a Global Warming Potential and Ozone Depletion Potential as it emits unquantified amounts of halogenated substances into the environment. PFAS production generates monomers of volatile and ionic compounds which also generate unquantified emissions that contaminate environment surrounding the production facilities and affect local communities (Kwiatkowski et al., 2020; Wahlström et al., 2021). Some of the activities of production include tetrafluoroethylene synthesis, polymerization, initiators, solvents and carriers, dispersants, and chain transfer agents. Moreover, some PFAS form part of products such as coatings, textiles, biomedical utensils, implants and orthopedic devices, foams and additives. Once the products have been used, they generate waste and mainly make their way to landfills or get treated thermally, by wastewater treatment plants, and less often, by recycling (Wahlström et al., 2021).
Due to their characteristics, PFAS have been suitable for multiple applications such as fire-fighting foams, defoaming additives, adhesives, cosmetics, cleaners, coating, among others, due to their properties such as surface activity and repellence to water, oil, and stains (Guo et al., 2020). The concern about PFAS is associated with their persistence, bio accumulative potential, and possible adverse effects on human health which are difficult to determine due to the complexity of environmental exposure. Animal models do not always reflect human toxicokinetics accurately, in addition to the fact that exposure to PFAS often occurs from mixtures which might act synergistically. Public attention has increased in the last years, positioning perfluorooctane sulfonate (PFOS) and its precursors in the list of persistent organic pollutants (POPs) from the Stockholm convention in 2009 (Boone et al., 2019; Guo et al., 2020).
Studies have associated the presence of PFAS in water to specific sources and daily activities, such as fire training facilities (Ahrens et al., 2014), the textile industry, domestic sewage and household garbage (Guo et al., 2020), as shown in Table 1. The presence of these compounds in water even after regulation does not show significant changes. Furthermore, their persistence has been detected up to 60 km away from an associated source (Boiteux et al., 2017). There is an association between seasons and PFAS water concentrations, with an elevated PFAS concentration observed during summer, mainly attributed to droughts. Nevertheless, during a specific study by Chen et al. (2017) no clear association was made between seasons and PFAS concentrations in different points of a river. At some sampling points PFAS concentrations increase with water flow, in others the opposite effect was observed. A possible explanation for this can be the different contamination sources, while for some the source could constantly discharge some untreated wastewater with a high concentration of PFAS, in other cases the water flow could affect the dilution factor, and in other cases the contamination source could be PFAS accumulated in soil where water flow increases compounds dragged to the river (Chen et al., 2017).
Due to PFAS transport phenomena, Figure 3, the presence of these substances in soil poses a long-term threat to water sources. The migration of PFAS from soil to groundwater could take from 3 to 20 years depending on the transport phenomena (Brusseau and Guo, 2021). These results are interesting as they suggest that even if regulation decreases the amount of PFAS discharged, the actual accumulation of these substances in soil would still impact water quality over the next 20 years. The major problem with PFAS and their transport to water is that conventional drinking water treatments did not efficiently remove it. In fact, different studies have shown the persistence of different PFAS in water, reporting no significant difference in the concentration before and after the treatment, even finding that some advanced treatment as ozonation could increase PFAS concentrations (Boiteux et al., 2017; Boone et al., 2019).
FIGURE 3. PFAS source, persistence, and transport in the environment to humans, and possible mitigation. Created with BioRender.com.
PFAS persist in a variety of different matrices, increasing the possibility of interaction with humans. PFAS contact mainly derives from activities during their production or contact with their subproducts. Diverse studies demonstrate that regardless of if people have been directly exposed or not, most people end up with PFAS in their system (Kato et al., 2018; Calafat et al., 2019; Li et al., 2021; Peng et al., 2022). The United States developed the National Health and Nutrition Examination Survey (NHANES) in the early 2000’s which included information of the presence of PFAS paired samples in serum and urine in people above 6 years of age. The study found a presence of long chain PFAS in urine and short chain PFAS in serum. These findings were not associated to specific activities or a clear exposure to PFAS (Kato et al., 2018; Calafat et al., 2019). On the other hand, the association between demographic factors and PFAS exposure was assessed in waste recycling workers. The study found concentrations up to 66 ngL−1 in urine samples and 33 ngL−1 in serum (Peng et al., 2022). Finally, Li et al. (2021) associated the presence of PFAS not only to carrying out activities that favor contact with these substances, but also to environments where they had detected PFAS in air and drinking water samples. The study was carried out on children 4–6 years old and detected the presence of PFAS in hair and urine samples. These studies show that the correct management of PFAS and the development of technologies that allow for their removal are needed.
Due to their chemical nature, characterized by strong C-F bonds along their backbone, PFAS are highly resistant to thermal, and biochemical degradation which leads to their accumulation in the environment and in human tissues (Buck et al., 2011). The physicochemical properties of PFAS are strictly related to the size of the chain and functional groups, resulting in two theories about their bioaccumulation and/or biomagnification mechanisms: PFAS bind to proteins such as serum albumin, fatty acid binding proteins and organic anion carriers; PFAS are divided into membrane phospholipids due to their greater affinity to charged species, which could result in greater bioaccumulation and distribution, as well as greater difficulty in elimination (Chen et al., 2021). The main way of exposure to PFAS is through polluted drinking water or food, which could be contaminated by cookware, food packing or from remnant sources of PFAS in the environment (Domingo and Nadal, 2019). Although there is still controversy about the degree of toxicity in the human body, several PFAS have been associated to diabetes, obesity, high cholesterol, certain types of cancer, and some effects during pregnancy such as neurodevelopment, and immunomodulation (Corsini et al., 2014; Sunderland et al., 2019). Figure 4 remarks the principal effects on human health.
FIGURE 4. Main effects of per- and polyfluoroalkyl substances (PFAS) on human health. Through contaminated sources such as drinking water or food, PFAS mainly cause endocrine disruption that could promote metabolic diseases, organ damage, fetal distress, neurodevelopment alterations and immunotoxicity. Created with BioRender.com.
Since their implementation was suspended in the early 2000s, the toxicity of PFAS has been rigorously tested. The U.S. Department of Health and Human Services, through the National Toxicology Program, has issued a series of technical reports about the toxicological effects caused by chronic exposure to perfluorinated sulfonated and carboxylated substances in rodents (National Toxicology Program (NTP), 2020). The results showed that the exposure to perfluorooctanoic acid (PFOA) has been associated with toxicity in liver, kidney, and thyroid gland, and with certain cancerogenic activity. After gavage administration the time of PFAS clearance increases with chain length (Huang et al., 2019a) from a half-life time of a few hours for perfluorobutane sulfonate (PFBS) to nearly 20 days for perfluorooctane sulfonate (PFOS), which increases their toxicity. Similar analyses were performed, with similar results, for 8:2 fluorotelomer alcohol (8:2-FTOH), perfluorohexane-1 sulfonic acid (PFHxS), perfluorohexanoic acid (PFHxA), perfluorooctanoic acid (PFOA), and perfluorodecanoic acid (PFDA)) (Huang et al., 2019a, 2019b; Dzierlenga et al., 2020). Toxicity studies of perfluorobutane sulfonic acid, perfluorohexane sulfonate potassium salt, perfluorooctane sulfonic acid, perfluorohexanoic acid, perfluorononanoic acid, and perfluorodecanoic acid have also been performed (National Toxicology Program (NTP), 2021a; National Toxicology Program (NTP), 2021b).
Several PFAS act as endocrine disrupting chemicals, increasing the risk of neurodevelopmental disorders, and obesity (Braun, 2017). PFOA and PFOS promote the activity of peroxisome proliferator-activated receptor-α (PPARα), and -γ (PPARγ) in humans, mice, and rats (vanden Heuvel et al., 2006). This impairment in PPARα activity affects adipocytic differentiation, increasing body fat, and the immunotoxicity of PFOA, presumably through modifications in antibody production (Bastos Sales et al., 2013; Corsini et al., 2014; Sunderland et al., 2019). PPARα impairment directly alters the cytokine production and human immune cell activation, throughout the inhibition of NF-κB activity. PFOA and PFOS toxicity reduces p65 phosphorylation which is crucial for the genetic transcription induced by NF-κB (Corsini et al., 2011, 2012).
It is still too soon to understand the effects of PFAS exposition completely. PFAS can also promote hepatocyte necrosis, hepatomegaly, splenic atrophy (Frawley et al., 2018), and interfere with mitochondrial respiration (Wallace et al., 2013). Some PFAs move evenly across the placental passage which exposes the fetus to PFAS (Gützkow et al., 2012). Maternal exposition to PFOS, PFOA and PFNA have been associated to lower birth weight (Verner et al., 2015), an impaired immune response in early childhood (Pennings et al., 2016), the risk of miscarriage and preeclampsia (Gao et al., 2021).
The findings of PFAS exposure during pregnancy have been inconsistent. Gao et al. (2021) carried out a statistical study of recent reports where the direct link between the effects of PFAS in pregnancy at concentrations greater than 1 ng/ml, showing that exposure to PFOS was directly associated with the risk of premature birth, exposure to PFNA and PFOA showed a relationship in the inverted U-shaped with risk of preterm birth, and exposure to PFDA and PFOS were directly associated with risks of miscarriage and preeclampsia, respectively. In vitro and in vivo studies have shown that exposure to PFAS has negative effects essential biological processes of the ovary, such as folliculogenesis and steroidogenesis which are the development of oocytes and follicles, as well as the consequent decrease in ovarian reserve, which are also linked to irregular and longer menstrual cycles, late menarche, and early menopause. These effects are associated with some alterations such as the activation of the peroxisome proliferator-activated receptor (PPAR), oxidative stress, interruption of thyroid hormone and interruption of gap junction intercellular communication. These studies were limited to ranges of human exposure to PFAS, PFOA from 3 to 220 ng/kg and PFOS from 1 to 130 ng/kg of body weight (Ding et al., 2020).
Grandjean et al. (2012) studied the effect of PFAS on the production of antibodies in children after the tetanus and diphtheria vaccines. The doubling of serum concentrations of PFAS such as PFOA, PFOS and PFHxS in children aged 5, led to a 50% loss of serum antibody concentrations by the time the children turned 7 years old.
PFAS exposition is not only a problem for human health. Poly- and per-fluorinated substances have are of great concern to the environment too. Several PFAS have been detected in many organisms across the globe and their toxic response varies in a species dependent manner (Fitzgerald et al., 2019; Hitchcock et al., 2019; Niu et al., 2019; Gaballah et al., 2020; Abercrombie et al., 2021; Ask et al., 2021; Death et al., 2021; Liu et al., 2022). Ankley et al. (2021) made an exhaustive revision of the myriad of PFAS-induced alterations on relevant members of animalia kingdom such as invertebrates, fish, amphibians, birds, reptiles, and mammalian wildlife. Invertebrate organisms only have an innate immune system that is based on protection by hemocytes or coelomocytes, which have an important role in non-specific cellular immunity, which, contrary to vertebrate organisms, have an adaptive immune system that offers them greater protection. Several PFAS have been shown to have negative effects on the cell viability of the innate immune system of earthworms, bivalves, and crustaceans (Ankley et al., 2021).
An extensive study on the effect of PFAS on zebrafish included the in vivo analysis of 139 PFAS at 10 concentrations in water between 0.015 and 100 µM from 6 to 120 h after fertilization, analyzing the larval photomotor response (LPR), the embryonic photomotor response (EPR) and 13 morphological endpoints as study response variables. The study showed that 49 PFAS exhibited bioactivity in one or more assays, with 25 induced altered LPR, 11 altered EPR, and 31 altered morphologies. Perfluorooctanesulfonamide (FOSA) was the only substance with activity in the 3 assays and perfluorodecanoic acid (PFDA) was the one that showed the highest bioactivity (Truong et al., 2022).
Similarly, the study carried out by Rericha et al. (2021) showed that 21 PFAS out of 58 studied induced aberrant larval behavior in zebrafish, without significant mortality. Already in larval development studies with concentrations of 0–100 μM of different PFCA with chain sizes between 4 and 12 carbons, they demonstrated an abnormal LPR, without significant alterations of the EPR or significant mortality.
The bioassay on the effect of PFOA and PFOS on Daphnia carinata carried out by Logeshwaran et al. (2021) demonstrated an LD50 at 48 h of 78.2 (54.9–105) mg L−1and 8.8 (6.4–11.6) mg L−1, respectively. Chronic exposure for 21 days showed effects on reproduction, with an increase in days to generate the first offspring and a reduction in the number of offspring and an increase in offspring mortality at concentrations from 0.001 mg L−1. The genotoxicity study using the comet assay at concentrations of 1–10 g L−1 showed significant damage to the genetic composition of D. carinata.
This environmental crisis urges the development of public policies, methodologies and technologies focused on the proper management and disposal of these highly durable substances that are having a great impact on ecosystems and human health. Despite the existence of several data regarding the neurotoxicity and its effects of PFAS, there is still a lack of research and development of new tests to evaluate all the possible effects of PFAS.
The inherent risks of PFA pollution to human health and environmental integrity has raised concerns over potential exposure pathways of biological agents. Drinking water remains a major route of human PFA consumption. Wastewater treatment plants are well-known sources of such substances (Banzhaf et al., 2017). Conventional WWTPs remediation processes have limited effectiveness for PFA removal and may even increase their concentration through breakdown of related precursors and byproducts; thus, WWTPs act as a crucial intermediary of PFA environmental cycling (Winchell et al., 2022).
Regulation worldwide has targeted the lowering of PFA concentrations in water resources as well as their impact. The United States Environmental Agency and US Geological Survey have released a report in conjunction that highlights the identification of PFA concentrations before and after drinking water treatment (Andrews et al., 2021). Furthermore, EPA Unregulated Contaminant Monitoring Rule 3 (UCMR3) targeted six PFA substances and recommended a Lifetime Health Advisory level of 70 parts per trillion for PFOS and PFAS present in drinking water (Kurwadkar et al., 2022). A similar measure by the European Commission limits total PFA concentration at 0.5 μg/L “pending the development of a suitable method for measuring total PFAS” (Dasu et al., 2022). These directives highlight the need for sensitive and specific PFA detection methods in water treatment effluents.
Current strategies for PFA detection with widespread implementation rely on liquid chromatography techniques coupled with a mass spectrometry detector (HPLC-MS). The United States Environmental Protection Agency describes three methods for the specific detection of PFAS, namely, methods 533, 537, and 537.1 (Winchell et al., 2021). The overview for these strategies requires the concentration of the sample followed by LC separation and has varying limits of detection ranging from 0.71 to 16 ppt and for a total combined detection of 29 compounds, limiting its application for drinking water (Menger et al., 2021).
Nevertheless, the chromatographic approach for PFA analysis poses several disadvantages. In a wastewater context, quality monitoring is a complex endeavor due to the high variability of the analyzed matrix and the low concentrations in which PFAs may be present (Choi et al., 2018). HPLC-MS analyses are also time consuming (35 min approximately per sample), have a high inherent cost, and require specialized equipment that impedes the in-situ assessment of samples (Fang, 2017). Sample preparation for analysis occasionally carries interference, samples may be contaminated in their handling through exposure to contaminated solvents and reagents, adsorption of PFA particles in glassware, and bacterial activity in non-refrigerated samples (Winchell et al., 2021). For these reasons, the development of fast, non-destructive PFA assessment techniques with minimal sample preparation for WWTP decision making has been an emergent topic of research in recent years (Figure 5).
FIGURE 5. Trends in PFA detection for wastewater treatment systems. Created with BioRender.com.
An electrochemical biosensor can be defined as an analytical device, which is based on the selective interaction between the target analyte and the biological recognition element (e.g., enzyme, DNA, antibodies, virus, cells, or microorganisms) coupled to an electrode in which reaction of oxidation/reduction takes place (Rodríguez-Delgado et al., 2015; Uniyal and Sharma 2018). Electrochemical biosensors are classified depending on their range of detection, which includes potentiometric biosensors that measure changes in the ion-selective membrane potential (mV) (Karimi-Maleh et al., 2021), conductometric biosensors that measure changes in conductance (G, Ω−1) (Jaffrezic-Renault and Dzyadevych et al., 2008), impedimetric biosensors that measure changes in the impedance (Z) over a range of frequencies (Hz) (Rodriguez et al., 2020) and voltammetric/amperometric biosensors which are characterized by measure the change in current (A) that occur during the oxidation/reduction reactions induced by an applied voltage (mV) (Rodríguez-Delgado et al., 2015).
Among these different approaches that can be adapted to develop an electrochemical biosensor, each have important advantages according to their different properties (Menger et al., 2021). For example, electrochemical biosensors allow for automated analysis due to their ability to combine high analytical selectivity and sensitivity with low cost, portability, and short analysis time (Karimian et al., 2018). Moreover, electrochemical transducers have other added advantages, conductometric transducers are light insensitive, do not need reference electrodes and can be miniaturized (Soldatkina et al., 2018), potentiometric transducers’ main advantage is their possibility to make portable kits by their fast and wide dynamic range of response (Karimi-Maleh et al., 2021), and amperometric/voltammetric transducers commonly have lower detection limits due to their high sensitivity to the analyte which usually is greater than those for potentiometric transducers, thus making amperometric/voltammetric biosensors most commonly applied in general (Grieshaber et al., 2008).
Different electrochemical biosensors have been applied for a variety of PFAs detection. For example, a selective perfluorooctane sulfonate (PFOS) amperometric biosensor was designed by the modification of a glassy carbon electrode with multiwalled carbon nanohorns as carriers of glutamic dehydrogenase (GLDH) and bilirubin oxidase (BOD) (Zhang et al., 2014). The PFOS biosensor presented a wide range of linear detection between 5 and 500 nmol/L with a lowest detection limit of LOD = 1.6 nmol/L and high selectivity over different chemicals that co-exist with PFOS in micro-polluted water, which suggested that this biosensor might be implemented in real water monitoring. Moreover, an impedimetric biosensor for the detection of perfluorooctanoic acid (PFOA) was designed by the modification of a graphite screen printed electrodes (G-SPE) (Moro et al., 2020). This electrochemical biosensor was developed by the modification of a G-SPE with a covalently immobilized human serum albumin (hSA) as bioreceptor into pyrrole-2-carboxylic acid (Py-2-COOH) and PFOA was detected in range between 100 nmol/L and 5 μmol/L. In addition, some other bioreceptors have been applied for the detection and monitoring of PFAs, such as DNA immobilized into Au/(Peroxide polypyrrole) electrode, which was applied for the detection of PFOA (Lu et al., 2011). Moreover, molecular imprinted polymers (MIPs) are mimetic biosensors due to their similarity to natural antibodies and antigens, which allows them to implement this kind of material as a synthetic receptor for a targeted molecule (Belbruno, 2019). Owing to important characteristic such as thermal and pH stability, reusability, and low-cost synthesis (Menger et al., 2021), MIPs based electrochemical biosensors have been applied for the detection of PFAs, including PFOS sensors (Karimian et al., 2018; Moro et al., 2019) and PFOA (Gong et al., 2015; Fang et al., 2016).
The fast analysis times and low costs of colorimetric assays, coupled to an acceptable sensitivity towards targeted contaminants, has placed this category as a trending topic of research for emerging contaminant analysis (Wongniramaikul et al., 2018; Wu et al., 2020). When applied to wastewater monitoring, colorimetric assays can offer real-time, in-situ monitoring of effluents in miniaturized kits and fast interpretation by the user (Awual, 2017).
PFA substance sensing by colorimetry has been attempted and reported through several mechanisms of chromophore generation. A novel method for this type of sensing involves the destabilization of gold or silver nanoparticles, generating surface plasmons that can be monitored optically; such an approach can be incorporated into paper-based assays (Vikesland, 2018). In a study by Takayose et al. (2012), perfluorooctanoic acid was focused as an analytic substance using a detection system based on the application of gold nanoparticles (AuNPs); the study is based on the aggregation of fluorine-fluorine groups and the resulting interactions between modifying groups caused a color shift from red and purple. The study concluded that PFOA could be detected by the naked eye after 10 min of reaction at concentrations as low as 250 µm. This report highlights the emerging approach of applying gold or silver nanoparticles as a platform for PFA substance detection, owing to features such as large surface area and high adsorption capabilities that enable the development of nano-based sensors. Gold nanoparticles have also been reported as a sensing method for perfluorinated compounds (PFC) in water sources by Niu et al. (2014) by causing an aggregation of AuNPs by adsorption and a consequent precipitation that changes the color response of the assay up to a PFC concentration of 10 μg L−1.
Colorimetric assessment can also be achieved through the complexation of PFA ions with cationic dyes to create hydrophobic phases that can be extracted and compared to a standardized chart to determine substance concentration using an analysis akin to a pH strip (Fang et al., 2018). To diminish the subjectivity of the user, assessed samples can be analyzed to a paired smartphone app that provides an objective evaluation through the introduction of a calibration step (Menger et al., 2021). To enhance resolution from such comparatively high concentrations, samples may be previously pre-concentrated using solid-liquid extraction (Zheng et al., 2019); the usage of a magnetic agent such as iron oxide nanoparticles can also for complexation can also increase detection capabilities by concentrating the sample (Liu et al., 2019; Menger et al., 2021).
Assays and kits that rely on colorimetric techniques can be seen as complementary tools to analytical detection methods, as they trade off convenience and ease of use for diminished detection capabilities, higher detection limits and assay subjectivity. Product allocation is also dependent on the development of market-viable kits and regulation of sensing platforms and parameters by pertinent agencies (Menger et al., 2021). Nano-based sensors are also still restricted to a narrow group of PFA pollutants and water applications, and the high adsorption capabilities of nanoparticles and potential cell membrane penetration raises concerns over the proper environmental interaction of AuNPs used in potential environmental biosensors (Liu et al., 2014).
Spectroscopy has become a tool with enough potential to provide the means for enhanced sensitive detection of plastic microparticles and related environmental contaminants (Chen et al., 2020). This approach also provides the potential of smaller probes, allowing an in-situ, real-time and non-destructive monitoring with positive implications for the decision making at the economical and risk-assessment of operation (Peng et al., 2019). The selection of the appropriate spectrum band for the detection of interest compounds is crucial as molecules may absorb different spectrum bands with varied intensity (Mesquita et al., 2017). Care must be taken when pondering the need for spectral resolution, available equipment’s power, spectrum quality and technique specificity.
Infrared spectroscopy comprises three energetic wave regions: far-infrared, mid-infrared, and near-infrared (Zulkifli et al., 2018). Assays determine the qualitative and quantitative nature of a sample through its changes in the vibrational state of the molecular bonds that compose the material (Gowen et al., 2015). Due to its quick and straightforward features, infrared spectroscopy has potential for the real-time monitoring and characterization of pollutants in wastewater (Park et al., 2021). The gradual development of IR spectrometers has enabled this technology to perform in-situ tests with minimal preparation.
The application of infrared spectroscopy for the detection of PFASs and related contaminants in drinking water have been explored recently. A mixed qualitative and quantitative approach is provided as an example by Park et al. (2021), using regression models to correlate obtained spectrums with PFAS as well as assessing the effect of salinity, pH and other water quality parameters in a multivariate model. The obtention of PFA spectra is often not possible by conventional readings, and a mathematical treatment of spectra is needed to overcome the complications in the reduced detection rate of non-treated wastewater samples (Carreres-Prieto et al., 2020). Thus, sample preparation may also be a concern for the researcher proposing the testing methodology, as absorbance and dispersive properties of the testing sample may be inadvertently changed (S. Tagg et al., 2017; Hong et al., 2021). Minimal sample preparation is preferred to ensure reproducibility in test procedures and obtained results.
Challenges and issues remain for the full integration of IR spectroscopy in the workflow of the on-site WWTP monitoring. Developments in systems and methods have been largely impaired as there is still not enough validation for established tests for quantification (Li et al., 2018). As PFA detection using infrared spectroscopy is still an infant field, a database for corresponding IR fingerprints is still not well established and is limited to theoretical spectra calculated for a battery of PFA compounds (Wallace et al., 2021). Available molecular information is still limited and the effect of the complex interaction that particles sustain in a complex matrix such as those found in a wastewater sample are still not well studied (Hernández et al., 2019). Water interference is usually regarded as the main challenge for the spectroscopic analysis in the infrared spectrum and is preferably to be removed in a pretreatment step (Xu et al., 2019).
Raman spectroscopy (RS) relies on inelastic scattering from reflecting light in visible wavelengths into target molecules (Silver et al., 2019). Substances each have their unique inelastic scattering pattern that distinguishes it from one another. This fingerprint allows the easy and fast identification of compounds in complex solutions (Sobhani et al., 2019). As IR, Raman spectra have proven useful for water quality monitoring, as it is a fast, reliable, and cheap tool for the characterization of molecules suspended in an aqueous solvent (Kniggendorf et al., 2019). Compared to IR, the main advantage of Raman spectroscopy for its use on biological samples is the minimal interference caused by water molecules, the most common solvent present in test solutions (Araujo et al., 2018). This minimizes the required sample preparation and poses RS as an enticing tool for on-site WWTP monitoring.
Affinity of the test substrate may be improved using complementary particles that enhance light interaction with the target PFAS molecule in the water sample. Surface-Enhanced Raman Spectroscopy (SERS) is used as the main way to enhance the weak inelastic Raman scattering by two means: electromagnetic and chemical enhancement. The optical properties of metallic nanoparticles that integrate elements such as gold and silver generate electromagnetic fields with a size comparable to the wavelength of the light being used generating a phenomenon known as localized surface plasmon resonance (LSPR) (Jia et al., 2021). The generated electromagnetic field amplifies the Raman signal of the molecule that is in proximity to the nanoparticle. This phenomenon can be controlled by changing the parameters of the nanoparticle such as size, composition, structure, and adjacent environment (Vikesland, 2018). Further research allowed the introduction of more complex shapes such as rods, cubes, and stars as well as 2D arrangements (Wei et al., 2015). The main result of the introduction of new morphologies is the overall enhancement of the Raman effect, by creating hotspots in the physical gaps between particles (Ong et al., 2020).
The specific application of RS and SERS to detect PFAs in aqueous solutions is limited. Fang et al. (2016) reported the detection of fluoro-surfactants such as perfluorooctanesulfonic acid using silver nanoparticles to enhance Raman activity. The overall low sensitivity of RS has remained an issue for the collection and processing of meaningful data (Bell et al., 2021). Further improvements on spectrum collection must ponder the ability to purify the target pollutant molecule on the studied matrix and to optimize the resolution of the obtained spectrum through enhanced mathematical manipulation of obtained data. Another recommended solution is the coupling of chemometric methods such as Principal Components analysis or Least-Square regression to characterize process variables and set an acceptable limit of detection, as discussed in the RS-based monitoring of polyfluoroalkyl ethers in aqueous matrixes by Marchetti et al. (2020).
While the feasibility of pollutant detection through spectroscopic methods has been already established, most if not all studies have stagnated at the proof-of-concept stage due to limitations of the methodology, reduced testing equipment availability or lack of procedural optimization (Chen et al., 2020; Zhang et al., 2020). Spectroscopy diagnostic methods have matured to a good enough level where taking the next step towards commercial applications must be considered. As breakthroughs are achieved on the fabrication techniques of sensors that enables higher sensitivities, lower sizes and cheaper prices, the use of this approach for the WWTP implementation of PFA monitoring may become practical.
As the detection methods show, the concentration of PFAS in the environment has increased with the human activities. One common migration pathway of the contaminants to the environment is through the sewage, as most of the WWTPs are not capable of removing efficiently PFAS. Traditional WWTPs have primary and secondary treatments focused on the removal of solids and disinfection to eliminate biological pathogens, and in some cases a tertiary treatment for the removal of other contaminants (Rout et al., 2021). However, persistent contaminants like PFAS are not efficiently removed during these treatments (Lenka et al., 2021).
In this way several approaches have been evaluated to reduce the leakage of these contaminants to the environment. Nevertheless, some of the technologies employed with other contaminants, like chlorination, flocculation or precipitation, may produce toxic by-products or are less efficient with PFAS (Mazhar et al., 2020; McCord et al., 2020; Rout et al., 2021). Due to this, most of the studies have been focused on the application of adsorption, advanced filtration, advanced oxidation and biodegradation processes (Phong Vo et al., 2020).
Adsorption is a technology widely employed in the removal of contaminants, especially for water remediation. It consists in the transfer and further adhesion of atoms or molecules of the contaminants, or adsorbate, from the solution the surface of a solid material, named adsorbent. This technology has been extensively used mainly because it is relatively simple and cheap. Also, adsorption is a versatile technology, as there is a wide range of adsorbents that can be employed such as carbon-based materials, zeolites, polymeric materials, metal-organic frameworks (MOFs), nanoparticles, ceramic materials, composites, and natural adsorbents (Phong Vo et al., 2020; Militao et al., 2021; Vu and Wu, 2022).
Due to this advantages, different adsorbents have been employed to evaluate their potential in the removal of PFAS from wastewater. The use of carbon-based materials is a common approach in adsorption, especially activated carbon and biochars, due to its low cost and accessibility and high adsorption capacity. Activated biochars from spent coffee grounds (SCG) were evaluated for the removal of perfluorooctanesulfonate (PFOS) from a simulated wastewater effluent (Steigerwald and Ray, 2021), and the results showed up to 99.6% of removal. The adsorption capacity of the SCG biochars was 43.4 mg/g, compared to 55.7 mg/g of a commercial activated carbon, and 79.5 mg/g of a fly ash char. Another activated biochar produced from sewage sludge (SBAC) was evaluated using a mixture of nine of the most common PFAS and compared to a commercial activated carbon (Mohamed et al., 2022). The results showed that the SBAC removed more than 91% of the PFAS, which was higher than granular activated carbon.
Another study evaluated the removal from water of several PFAS, mainly present in groundwaters affected by aqueous film-forming foams (AFFF), by commercial biochar, granular activated carbon (GAC) and biochar synthesized from pine needle (Xiao et al., 2017). The authors included 30 different PFAS and compared their removal by the proposed adsorbents, evaluating their sorption in batch experiments. Also, this data was employed to adjust an intraparticle diffusion-limited sorption kinetic model to predict the quantity of the pollutants that could be retained by the adsorbents. The results showed that even though GAC could adsorb PFASs, the adsorption of PFOS-like PFASs was less effective than PFOS and PFOA.
A research evaluated the adsorption of PFAS by biochars produced by the conversion of biosolids from wastewater treatment plants at three different temperatures (500, 550, and 600°C), and compared their performance with a sawdust biomass biochar (Kundu et al., 2021). The 600°C biochar (BSBC-600) had higher surface area, thus it was selected for the adsorption tests, where the results showed that for long-chain PFASs the adsorption was above 80%, while for short-chain PFASs was in the range of 19–27%. Compared to the sawdust biochar, BSBC-600 had a lower removal efficiency for most of the PFAS, however due to its low production cost, biosolids biochars remain as a promising alternative.
Despite the great potential showed by carbon materials, there are still some drawbacks that are needed to overcome, especially to improve the selectivity of PFAS in solutions with coexisting species. The studies have shown that in most cases their removal efficiency is decreased as the presence of dissolved organic matter or other organic compounds increases (Gagliano et al., 2020; Vu and Wu, 2022). In this way, a major challenge to enhance the application of adsorption in the wastewater treatment is to develop new low-cost materials with higher selectivity towards specific PFAS.
Membrane filtration is a process where the molecules of the contaminants are retained in membranes by a pressure-driven and separated from water, mainly due to size exclusion, but also by electrostatic repulsion and diffusion processes (Speed, 2016; Franke et al., 2019). Membranes can be classified according to their pore size, but the most common for the removal of PFAS from water are nanofiltration (NF) and reverse osmosis (RO) (Phong Vo et al., 2020). These membrane technologies have high removal efficiencies of PFAS that can be above 99% (Soriano et al., 2017; Franke et al., 2019; Phong Vo et al., 2020).
A study evaluated the removal efficiency of both nanofiltration and reverse osmosis for the removal of 42 different PFAS from water affected with aqueous film-forming foam (AFFF) (Liu et al., 2021). The results showed that the rejection efficiency for the PFAS was above 97% for both NF and RO. The authors also found that changing the matrix negatively impacted the removal efficiency. Another research employed reverse osmosis membranes to remove perfluorooctane sulfonate (PFOS) from wastewater (Tang et al., 2006). The authors reported that the membrane rejected 99% of the PFOS within a concentration range of 0.5–1,500 ppm. Also, the presence of isopropyl alcohol affected the removal efficiency, and the authors recommended the application of pretreatments to wastewater to remove it before the RO.
Membrane technologies have a great potential in the removal of PFAS from wastewater. Their efficiencies are higher than most of the other technologies, however there are still some problems that need to be assessed to promote the implementation of this technology. One of them is related to membrane fouling, as other organic contaminants and inorganic ions may reduce considerably their efficiency. Another major issue is the cost of operation, as it requires high pressures, and the membranes are expensive to produce.
Different technologies have been explored for PFAS removal from water resources and soil samples; however, the high energy C–F presented in PFAS’ structure make them resistant to many of such treatment methods (hydrolysis, biodegradation, photolysis, among others) (Wanninayake, 2021). In recent times, advanced oxidation processes (AOPs) have emerged as simple, clean, and efficient alternatives for water treatments. They are defined as chemical oxidation processes capable of degrading pollutants because of the in-situ generation of oxidizing agents, such as hydroxyl radicals
The mechanism in which heterogeneous photocatalysis degrade PFAS initiates with hv absorption to generate electrons (eˉ) and holes (h⁺), which in the surface of the photocatalysts are able of degrading PFAS pollutants (Xu et al., 2017). A wide diversity of photocatalysts with efficient PFAS removal efficiencies can be found in the literature, such as bismuth-based (Bacha et al., 2019), gallium-based (Zhao et al., 2015; Zhu et al., 2021), titanium dioxide-based (Li et al., 2016), and zinc oxide-based (Wu et al., 2017, 2018) photocatalysts. TiO2 as a photocatalyst has been widely used to remove a vast diversity of pollutants (Bouyarmane et al., 2021; Paumo et al., 2021). In fact, TiO2 doped with transition metals has been employed to remove perfluorooctanoic acid (PFOA) by photocatalytic decomposition. Li et al. (2016) reported that Pt-, Pd-, and Ag-doped TiO2 presented 12.5, 7.5-, and 2.2-times higher degradation than pure TiO2, respectively. The remarkably better performances were attributed to the capacity of the noble metallic nanoparticles to capture the electrons accumulated in the conduction band (Li et al., 2016). In comparison to pure TiO2, gallium-based photocatalysts have also demonstrated superior photocatalytic degradation which has been attributed to its higher oxidation-reduction potential (Zhao et al., 2015; Xu et al., 2017). In this regard, Zhao et al. (2015) reported an almost complete degradation of PFOA within 90 min of UV light irradiation.
On the other hand, sonochemical treatment has also shown the potential to efficiently degrade PFAS from water samples. This process belongs to AOPs since the organic pollutants are oxidized by hydroxyl radicals produced during the treatment (Cao et al., 2020). A low frequency (20 kHz) sonochemical method achieved a complete degradation of perfluorooctane sulfonate (PFOS) and PFOA (at trace levels) within 80 min of process time (Panda et al., 2019). Sonochemical treatments possess advantageous features such as energy-saving, high cleanliness, and non-secondary pollution. Interestingly, Lei et al. (2020) combined the use of ultrasound with persulfate-based AOPs to synergistically degrade different PFAS. Among the tested PFAS compounds, PFOA presented the highest degradation percentage (∼100%) in water samples. Sonochemical treatment was notably enhanced by persulfate since it can be activated by ultrasound generating sulfate radicals improving the degradation (Lei et al., 2020).
Electrochemical oxidation has gained increased research attention due to its simplicity, high energy efficiency, easy operation, eco-friendliness, and effectiveness for the degradation of different organic pollutants (Barisci and Suri, 2020, 2021). Like other AOPs methods, pollutants subjected to this process are degraded due to the reaction with hydroxyl radicals. However, such hydroxyl radicals are formed at the surface of an anode from water oxidation (Barisci and Suri, 2021). Therefore, the anode material is a highly relevant parameter to reach a complete degradation of PFAS. Different anode materials have been tested according to their electron transfer abilities, hydroxyl radical generation capacities, and oxygen evolution potential. Boron-doped diamond (BDD) electrodes have been widely explored to degrade different PFAS. As representative work, Sukeesan et al. (2021) reported a PFOA degradation efficiency of 94% using Si/BDD anode and titanium cathode under the optimal conditions (applied current density of 40 mA cm−2 and potential voltage at 25 V). The effect of different parameters during electrochemical oxidation have been explored, such as electrolyte, initial pH, initial pollutant concentration, applied current density, among others. Interestingly, it has been reported a remarkable effect on the degradation rate by the chain length of PFAS compound; increasing chain length leads to higher degradation ratios because of higher hydrophobicity (Barisci and Suri, 2020, 2021).
Other anode materials like Ti/RuO2 (Barisci and Suri, 2021), 3D graphene-lead dioxide (Duan et al., 2020), and graphene oxide-titanium dioxide anode coated on fluorine-doped tin oxide glass (Yang J. S. et al., 2020) have been evaluated to avoid the disadvantages presented by BDD electrodes such as its high cost and toxicity potential if leaching occurs (Barisci and Suri, 2021). Table 2 presents the most recent advances in the degradation of different types of PFAS through AOPs methods.
Research on PFAS biodegradation was discouraging for many years, once it was considered ineffective, and only physicochemical treatments for PFAS degradation were sought due to high stability and resistance to biodegradation, however, recent research with various microbial species, certain pure strains and some mixed cultures have been reported to degrade certain PFAS species such as PFOS, PFOA and some PFAA precursors. These successful results in biodegrading PFAS have led to increased scientific interest in this degradation strategy. However, there are still very important challenges in the biodegradation of PFAS such as the complexity of the PFAS species, the environmental conditions, and the composition of the microorganisms (Zhang et al., 2022).
Biodegradation of PFAS requires a hydrogen atom in the alkyl chain for primary attack and molecular cleavage. Another factor that also limits biodegradation is the oxidative replacement of fluorine atoms, since these could form a dense hydrophobic layer that surrounds the C-C bonds, inhibiting oxidative degradation. For this reason, it is believed that the results obtained in the initial studies of aerobic and anaerobic biodegradation of PFOS and PFOA can be attributed to sorption processes (Kucharzyk et al., 2017).
Within all the microbial species studied, Pseudomonas sp. has shown greater potential in the biodegradation of PFAS. The HJ4 strain of Pseudomonas aeruginosa was the first report of effective biodegradation and quantification of intermediates resulting from the degradation of PFOS to PFBS and perfluorohexane sulfonate (PFHxS), with 67% efficiency (Kwon et al., 2014).
Pseudomonas plecoglossicida 2.4-D strain was reported with high PFOS biodegradation rates, an efficiency of 75% in soil in 90 days, and 100% of efficiency in laboratory using Raymond’s mineral medium as carbon source in 6 days (Chetverikov et al., 2017).
Recent reports demonstrate the high effectiveness of the biodegradation of the aerobic strain Gordonia sp. aerobic NB4-1Y, which showed efficiencies of 85 and 88% in 1 week, in the degradation of alkylbetaine 6:2 fluorotelomer sulfonamide and 6:2 sulfonate fluorotelomer, respectively (Shaw et al., 2019).
Several reports suggest a variety of advanced processes for remediation, Ion exchange resins, carbon-based absorption, electrochemical degradation, and nanofiltration has shown a removal efficiency from 90 to 100% than conventional processes. However, more attention has been posed to short-chain PFAs, PFOA, and PFOS, some of these processes can be less efficient for this kind of compound (Lenka et al., 2021; Mukhopadhyay et al., 2021), due to their use as an alternative to long-chain ones, and to their high mobility in the water bodies, therefore short-chain PFAs removal is more challenging. For implementations to conventional treatment systems, nanofiltration and carbon-based absorption could be a more viable option (Gagliano et al., 2020; Dixit et al., 2021).
In a recent study, authors propose that regenerable organic scavenger resins can operate for longer durations when compared to PFAs-specific resins in single-use mode. However, regenerable resins demand higher resin contact times when compared to single-use resins (Coyle et al., 2021). Remediation based on absorption is the most common process for PFAs removal. It is important to consider a key factor for process implementation, ion exchange resin selectivity, which are classified by fluorinated groups, cationic groups, and cavitary groups. To improve the remediation process, their synergy effects have been probed (Wang et al., 2021). Alternative methods such as enhanced photolysis, and sonochemical destruction can conduct reduction of PFAs at some level. Also, new technologies like nanofiltration, electrochemical anodic oxidation, and electro-Fenton degradation have been proposed to maximize economic and environmental benefits of PFAs remedial measures avoiding the high energy consumption and extreme operating conditions from other methods that made the implementation process unviable for scaling up (Lu et al., 2020).
Nevertheless, the high harmful impacts of PFAS on human health, the international treaties to reduce the production of PFAS were signed in the early 2000 s. The implemented regulations have specific restrictions based on national, state and territory-level, even though there are differences within countries. The US EPA released limits for the use of PFOS and PFOA in 2016. After that, a rapid increase in the number of states following the policies to prevent the PFAS pollution and their effects (Brennan, et al., 2021).
Some PFAS have been regulated in Europe. PFOA and PFOS and another long chain (PFHxS) were listed in the Stockholm Convention on Persistent Organic Pollutants (POPs) and restricted under the EU. Alternatives were developed to substitute long-chain PFAS, however they still trigger adverse effects in human health and the environment. Due to these concerns the short-chains PFAS are starting to be regulated under EU (European Commission, 2020).
The PFOA regulations in Asian countries are moving to strengthen the decision of the use of chemical substances. In April 2021, PFOA was added to the list of Chemical Substances Control Act, but related substances are excluded from that. Also, PFOA use was restricted in China in 2020. Other countries such as Taiwan and Singapore the use of PFOA is partially regulated (Enviliance ASIA, 2021).
Challenges derived from PFAS are complex, making it difficult to develop policies. The lack of funding supporting PFAS legislation, the variable monitoring of their impact on the environmental and human health worldwide, the limited release of scientific evidence, and restricted cultural understanding of the prioritization of environmental issues. Furthermore, substitutes need to keep their advantageous properties while avoiding their negative properties in order to propel their widespread adoption.
PFAS are very stable chemical substances of low biodegradability that do not hydrolyze or photolyze under ambient conditions, resulting in persistent and bioaccumulative substances in the environment that are extremely difficult to remedy. The wide distribution in aquatic environments derived from its physicochemical properties and high solubility in water, which have generated concern about possible negative effects on human and animal health. The increasing concentration of PFAS in the environment, especially in water, has become an important problem gaining the scientific communities’ attention particularly with a focus on the detection and removal of these contaminants.
The study of the distribution and ecological risks of PFAS in the environment is a multidisciplinary challenge that requires collaboration between experts from many areas from the field of chemistry, biotechnology, environmental toxicology, health, among many others to solve lack of information and problems associated with the distribution of PFAS. The PFAS family is made up of chemical compounds with highly variable physicochemical properties, which depending on the destination or exposure to these compounds, their effects may vary.
The real time analysis of influents and effluents of WWTPs is needed to ensure the quality of the water treatment to avoid the discharge of PFAS back to the environment. This will be a challenge, particularly when coupled with traditional detection methods. In this way, spectroscopic methods for detection are a promising alternative that requires more attention to go beyond the proof-of-concept stage.
Regarding the removal technologies, membrane technologies have shown high efficiency of removal compared to other methods, however their operational costs hinder their applications in most WWTPs, making technologies like RO more suitable for its application in drinking water treatment. Due to this, adsorption and advanced oxidation processes represent an attractive alternative for wastewater treatment, especially for their implementation in developing countries. Adsorption has already showed high potential in the removal of several emerging contaminants, mainly due to the wide variety of adsorbents, its relative low cost, ease of operation and competitive removal rates. However, for its application for PFASs removal it is necessary further research to understand better the adsorption mechanisms and to improve the selectivity, the major challenges of this technology. On the other hand, diverse AOPs have been already tested at pilot scale with good PFASs removal rate, being photocatalytic degradation and Fenton-based the ones with better results. Despite the great performance of AOPs, it is necessary to improve their energetic efficiency and selectivity for being applied in real urban and industrial effluents.
As knowledge of the different types and physicochemical behavior of PFAS grows, more efficient and economic strategies as well as technologies for detection and degradation are created. These advances aim to fulfill current global regulations as well as inspire new ones to control the excessive use of products with PFAS as well as generate public knowledge about PFAS environmental and human health impact.
Current reports on the study of PFAS continue to generate new information on damage to health and the environment, however, more information on compounds as well as their combinations are needed, as it difficult to obtain all information on each PFAS. In recent years, different effective bio-based technologies have been developed that remove PFAS. As they because they still lack information regarding efficacy in complex samples, different WWTP flows, and generation studies. degradation compounds and their effects approval and implementation. Some effective actions to manage the risks surrounding PFAS and their continuous accumulation in the environment would be to regulate the production and use of these compounds, emphasizing the importance of eliminating non-essential uses of PFAS, as well as developing of biodegradable compounds with similar properties, as well as the continuous study of strategies and technologies for the detection and safe elimination of PFAS in the environment.
RGA, JR-H, RG-G, RM-G, MM-R, HR-P, SM, LP-A, EM-M, JS-H, KC-A, SV, DB, HNI, RP-S participated in the preparation and revision of the manuscript. All authors listed have made substantial, direct, and intellectual contribution to the work and approved it for publication.
This work is part of the project entitled “Contaminantes emergentes y prioritarios en las aguas reutilizadas en agricultura: riesgos y efectos en suelos, producción agrícola y entorno ambiental” funded by CSIC-Tecnológico de Monterrey under i-Link + program (LINKB20030).
The authors declare that the research was conducted in the absence of any commercial or financial relationships that could be construed as a potential conflict of interest.
All claims expressed in this article are solely those of the authors and do not necessarily represent those of their affiliated organizations, or those of the publisher, the editors and the reviewers. Any product that may be evaluated in this article, or claim that may be made by its manufacturer, is not guaranteed or endorsed by the publisher.
The authors acknowledge Tecnológico de Monterrey for the financial support for the publication. All listed authors are also grateful to their representative universities/institutes for providing literature facilities. Consejo Nacional de Ciencia y Tecnología (CONACYT) is thankfully acknowledged for partially supporting this work under Sistema Nacional de Investigadores (SNI) program awarded to RA (CVU: 714118), MM-R (CVU: 418151), EM-M (CVU: 230784), JS-H (CVU: 375202), HNI (CVU: 735340) and RP-S (CVU: 35753).
Abercrombie, S. A., Perre, C., Iacchetta, M., Flynn, R. W., Sepúlveda, M. S., Lee, L. S., et al. (2021). Sublethal Effects of Dermal Exposure to Poly and Perfluoroalkyl Substances on Postmetamorphic Amphibians. Environ. Toxicol. Chem. 40, 717–726. doi:10.1002/etc.4711
Ahrens, L., Norström, K., Viktor, T., Cousins, A. P., and Josefsson, S. (2014). Stockholm Arlanda Airport as a Source of Per- and Polyfluoroalkyl Substances to Water, Sediment and Fish. Chemosphere 129, 33–38. doi:10.1016/jchemosphere.2014.03.13610.1016/j.chemosphere.2014.03.136
Andrews, D. Q., Hayes, J., Stoiber, T., Brewer, B., Campbell, C., and Naidenko, O. V. (2021). Identification of Point Source Dischargers of Per and Polyfluoroalkyl Substances in the United States. AWWA Water Sci. 3, e1252. doi:10.1002/aws2.1252
Ankley, G. T., Cureton, P., Hoke, R. A., Houde, M., Kumar, A., Kurias, J., et al. (2021). Assessing the Ecological Risks of Per and Polyfluoroalkyl Substances: Current State of the Science and a Proposed Path Forward. Environ. Toxicol. Chem. 40, 564–605. doi:10.1002/etc.4869
Araujo, C. F., Nolasco, M. M., Ribeiro, A. M. P., and Ribeiro-Claro, P. J. A. (2018). Identification of Microplastics Using Raman Spectroscopy: Latest Developments and Future Prospects. Water Res. 142, 426–440. doi:10.1016/j.watres.2018.05.060
Ask, A. V., Jenssen, B. M., Tartu, S., Angelier, F., Chastel, O., and Gabrielsen, G. W. (2021). Per and Polyfluoroalkyl Substances Are Positively Associated with Thyroid Hormones in an Arctic Seabird. Environ. Toxicol. Chem. 40, 820–831. doi:10.1002/etc.4978
Ateia, M., Alsbaiee, A., Karanfil, T., and Dichtel, W. (2019). Efficient PFAS Removal by Amine-Functionalized Sorbents: Critical Review of the Current Literature. Environ. Sci. Technol. Lett. 6, 688–695. doi:10.1021/acs.estlett.9b00659
Awual, M. R. (2017). New Type Mesoporous Conjugate Material for Selective Optical Copper(II) Ions Monitoring & Removal from Polluted Waters. Chem. Eng. J. 307, 85–94. doi:10.1016/j.cej.2016.07.110
Bacha, A.-U. -R., Nabi, I., Fu, Z., Li, K., Cheng, H., and Zhang, L. (2019). A Comparative Study of Bismuth-Based Photocatalysts with Titanium Dioxide for Perfluorooctanoic Acid Degradation. Chin. Chem. Lett. 30 (12), 2225–2230. doi:10.1016/j.cclet.2019.07.058
Banzhaf, S., Filipovic, M., Lewis, J., Sparrenbom, C. J., and Barthel, R. (2017). A Review of Contamination of Surface-, Ground-, and Drinking Water in Sweden by Perfluoroalkyl and Polyfluoroalkyl Substances (PFASs). Ambio 46, 335–346. doi:10.1007/s13280-016-0848-8
Barisci, S., and Suri, R. (2020). Electrooxidation of Short and Long Chain Perfluorocarboxylic Acids Using Boron Doped Diamond Electrodes. Chemosphere 243, 125349. doi:10.1016/j.chemosphere.2019.125349
Barisci, S., and Suri, R. (2021). Electrooxidation of Short- and Long-Chain Perfluoroalkyl Substances (PFASs) under Different Process Conditions. J. Environ. Chem. Eng. 9 (4), 105323. doi:10.1016/j.jece.2021.105323
Bastos Sales, L., Kamstra, J. H., Cenijn, P. H., van Rijt, L. S., Hamers, T., and Legler, J. (2013). Effects of Endocrine Disrupting Chemicals on In Vitro Global DNA Methylation and Adipocyte Differentiation. Toxicol. Vitro 27, 1634–1643. doi:10.1016/j.tiv.2013.04.005
Belbruno, J. J. (2019). Molecularly Imprinted Polymers. Chem. Rev. 119, 94–119. doi:10.1021/acs.chemrev.8b00171
Bell, E. M., De Guise, S., McCutcheon, J. R., Lei, Y., Levin, M., Li, B., et al. (2021). Exposure, Health Effects, Sensing, and Remediation of the Emerging PFAS Contaminants - Scientific Challenges and Potential Research Directions. Sci. Total Environ. 780, 146399. doi:10.1016/j.scitotenv.2021.146399
Boiteux, V., Dauchy, X., Bach, C., Colin, A., Hemard, J., Sagres, V., et al. (2017). Concentrations and Patterns of Perfluoroalkyl and Polyfluoroalkyl Substances in a River and Three Drinking Water Treatment Plants Near and Far from a Major Production Source. Sci. Total Environ. 583, 393–400. doi:10.1016/j.scitotenv.2017.01.079
Bolan, N., Sarkar, B., Yan, Y., Li, Q., Wijesekara, H., Kannan, K., et al. (2021). Remediation of Poly- and Perfluoroalkyl Substances (PFAS) Contaminated Soils - to Mobilize or to Immobilize or to Degrade? J. Hazard. Mater. 401, 123892. doi:10.1016/j.jhazmat.2020.123892
Boone, J. S., Vigo, C., Boone, T., Byrne, C., Ferrario, J., Benson, R., et al. (2019). Per- and Polyfluoroalkyl Substances in Source and Treated Drinking Waters of the United States. Sci. Total Environ. 653, 359–369. doi:10.1016/j.scitotenv.2018.10.245
Bouyarmane, H., El Bekkali, C., Labrag, J., Es-saidi, I., Bouhnik, O., Abdelmoumen, H., et al. (2021). Photocatalytic Degradation of Emerging Antibiotic Pollutants in Waters by TiO2/Hydroxyapatite Nanocomposite Materials. Surfaces Interfaces 24 (3), 101155. doi:10.1016/j.surfin.2021.101155
Braun, J. M. (2017). Early-life Exposure to EDCs: Role in Childhood Obesity and Neurodevelopment. Nat. Rev. Endocrinol. 13, 161–173. doi:10.1038/nrendo.2016.186
Brennan, N. M., Evans, A. T., Fritz, M. K., Peak, S. A., and von Holst, H. E. (2021). Trends in the Regulation of Per- and Polyfluoroalkyl Substances (PFAS): A Scoping Review. Ijerph 18, 10900. doi:10.3390/ijerph182010900
Brusseau, M. L., and Guo, B. (2021). Air-water Interfacial Areas Relevant for Transport of Per and Poly-Fluoroalkyl Substances. Water Res. 207, 117785. doi:10.1016/j.watres.2021.117785
Buck, R. C., Franklin, J., Berger, U., Conder, J. M., Cousins, I. T., de Voogt, P., et al. (2011). Perfluoroalkyl and Polyfluoroalkyl Substances in the Environment: Terminology, Classification, and Origins. Integr. Environ. Assess. Manag. 7, 513–541. doi:10.1002/ieam.258
Calafat, A. M., Kato, K., Hubbard, K., Jia, T., Botelho, J. C., and Wong, L.-Y. (2019). Legacy and Alternative Per- and Polyfluoroalkyl Substances in the U.S. General Population: Paired Serum-Urine Data from the 2013-2014 National Health and Nutrition Examination Survey. Environ. Int. 131, 105048. doi:10.1016/j.envint.2019.105048
Cao, H., Zhang, W., Wang, C., and Liang, Y. (2020). Sonochemical Degradation of Poly- and Perfluoroalkyl Substances - A Review. Ultrason. Sonochemistry 69 (April), 105245. doi:10.1016/j.ultsonch.2020.105245
Carreres-Prieto, D., García, J. T., Cerdán-Cartagena, F., and Suardiaz-Muro, J. (2020). Wastewater Quality Estimation through Spectrophotometry-Based Statistical Models. Sensors 20, 5631. doi:10.3390/s20195631
Chambers, W. S., Hopkins, J. G., and Richards, S. M. (2021). A Review of Per- and Polyfluorinated Alkyl Substance Impairment of Reproduction. Front. Toxicol. 3, 1–9. doi:10.3389/ftox.2021.732436
Chen, H., Han, J., Zhang, C., Cheng, J., Sun, R., Wang, X., et al. (2017). Occurrence and Seasonal Variations of Per- and Polyfluoroalkyl Substances (PFASs) Including Fluorinated Alternatives in Rivers, Drain Outlets and the Receiving Bohai Sea of China. Environ. Pollut. 231, 1223–1231. doi:10.1016/j.envpol.2017.08.068
Chen, Y., Fu, J., Ye, T., Li, X., Gao, K., Xue, Q., et al. (2021). Occurrence, Profiles, and Ecotoxicity of Poly- and Perfluoroalkyl Substances and Their Alternatives in Global Apex Predators: A Critical Review. J. Environ. Sci. 109, 219–236. doi:10.1016/j.jes.2021.03.036
Chen, Y., Wen, D., Pei, J., Fei, Y., Ouyang, D., Zhang, H., et al. (2020). Identification and Quantification of Microplastics Using Fourier-Transform Infrared Spectroscopy: Current Status and Future Prospects. Curr. Opin. Environ. Sci. Health 18, 14–19. doi:10.1016/j.coesh.2020.05.004
Chetverikov, S. P., Sharipov, D. A., Korshunova, T. Y., and Loginov, O. N. (2017). Degradation of Perfluorooctanyl Sulfonate by Strain Pseudomonas Plecoglossicida 2.4-D. Appl. Biochem. Microbiol. 53, 533–538. doi:10.1134/S0003683817050027
Choi, P. M., Tscharke, B. J., Donner, E., O'Brien, J. W., Grant, S. C., Kaserzon, S. L., et al. (2018). Wastewater-based Epidemiology Biomarkers: Past, Present and Future. TrAC Trends Anal. Chem. 105, 453–469. doi:10.1016/j.trac.2018.06.004
Corsini, E., Avogadro, A., Galbiati, V., dell'Agli, M., Marinovich, M., Galli, C. L., et al. (2011). In Vitro evaluation of the Immunotoxic Potential of Perfluorinated Compounds (PFCs). Toxicol. Appl. Pharmacol. 250, 108–116. doi:10.1016/j.taap.2010.11.004
Corsini, E., Luebke, R. W., Germolec, D. R., and DeWitt, J. C. (2014). Perfluorinated Compounds: Emerging POPs with Potential Immunotoxicity. Toxicol. Lett. 230, 263–270. doi:10.1016/j.toxlet.2014.01.038
Corsini, E., Sangiovanni, E., Avogadro, A., Galbiati, V., Viviani, B., Marinovich, M., et al. (2012). In Vitro characterization of the Immunotoxic Potential of Several Perfluorinated Compounds (PFCs). Toxicol. Appl. Pharmacol. 258, 248–255. doi:10.1016/j.taap.2011.11.004
Coyle, C., Ghosh, R., Leeson, A., and Thompson, T. (2021). US Department of Defense-Funded Research on Treatment of Per and Polyfluoroalkyl Substance-Laden Materials. Environ. Toxicol. Chem. 40 (1), 44–56. doi:10.1002/etc.4836
Cui, J., Gao, P., and Deng, Y. (2020). Destruction of Per- and Polyfluoroalkyl Substances (PFAS) with Advanced Reduction Processes (ARPs): A Critical Review. Environ. Sci. Technol. 54, 3752–3766. doi:10.1021/acs.est.9b05565
Dasu, K., Xia, X., Siriwardena, D., Klupinski, T. P., and Seay, B. (2022). Concentration Profiles of Per- and Polyfluoroalkyl Substances in Major Sources to the Environment. J. Environ. Manag. 301, 113879. doi:10.1016/j.jenvman.2021.113879
Death, C., Bell, C., Champness, D., Milne, C., Reichman, S., and Hagen, T. (2021). Per- and Polyfluoroalkyl Substances (PFAS) in Livestock and Game Species: A Review. Sci. Total Environ. 774, 144795. doi:10.1016/j.scitotenv.2020.144795
Ding, N., Harlow, S. D., Randolph Jr, J. F., Loch-Caruso, R., and Park, S. K. (2020). Perfluoroalkyl and Polyfluoroalkyl Substances (PFAS) and Their Effects on the Ovary. Hum. Reprod. Update 26, 724–752. doi:10.1093/humupd/dmaa018
Dixit, F., Dutta, R., Barbeau, B., Berube, P., and Mohseni, M. (2021). PFAS Removal by Ion Exchange Resins: A Review. Chemosphere 272, 129777. doi:10.1016/j.chemosphere.2021.129777
Domingo, J. L., and Nadal, M. (2019). Human Exposure to Per- and Polyfluoroalkyl Substances (PFAS) through Drinking Water: A Review of the Recent Scientific Literature. Environ. Res. 177, 108648. doi:10.1016/j.envres.2019.108648
Duan, X., Wang, W., Wang, Q., Sui, X., Li, N., and Chang, L. (2020). Electrocatalytic Degradation of Perfluoroocatane Sulfonate (PFOS) on a 3D Graphene-Lead Dioxide (3DG-PbO2) Composite Anode: Electrode Characterization, Degradation Mechanism and Toxicity. Chemosphere 260, 127587. doi:10.1016/j.chemosphere.2020.127587
Dzierlenga, A. L., Robinson, V. G., Waidyanatha, S., DeVito, M. J., Eifrid, M. A., Gibbs, S. T., et al. (2020). Toxicokinetics of Perfluorohexanoic Acid (PFHxA), Perfluorooctanoic Acid (PFOA) and Perfluorodecanoic Acid (PFDA) in Male and Female Hsd:Sprague Dawley SD Rats Following Intravenous or Gavage Administration. Xenobiotica 50, 722–732. doi:10.1080/00498254.2019.1683776
Enviliance Asia (2021). PFOA Regulations in Asian Countries. Available at: https://enviliance.com/regions/asia-pfoa (Accessed January 28, 2022).
European Commission (2020). Poly- and Perfluoroalkyl Substances (PFAS). Available at: https://ec.europa.eu/environment/pdf/chemicals/2020/10/SWD_PFAS.pdf (Accessed January 28, 2022).
Fang, C., Chen, Z., Megharaj, M., and Naidu, R. (2016). Potentiometric Detection of AFFFs Based on MIP. Environ. Technol. Innovation 5, 52–59. doi:10.1016/j.eti.2015.12.003
Fang, C. (2017). Using Smartphones to Detect PFAS in Aqueous Film-Forming Foam Using Smartphones to Detect PFAS in Aqueous Film-Forming Foam. Callaghan, NSW, Australia: CRC Care.
Fang, C., Zhang, X., Dong, Z., Wang, L., Megharaj, M., and Naidu, R. (2018). Smartphone App-Based/portable Sensor for the Detection of Fluoro-Surfactant PFOA. Chemosphere 191, 381–388. doi:10.1016/j.chemosphere.2017.10.057
Fitzgerald, N. J. M., Temme, H. R., Simcik, M. F., and Novak, P. J. (2019). Aqueous Film Forming Foam and Associated Perfluoroalkyl Substances Inhibit Methane Production and Co-contaminant Degradation in an Anaerobic Microbial Community. Environ. Sci. Process. Impacts 21, 1915–1925. doi:10.1039/c9em00241c
Franke, V., McCleaf, P., Lindegren, K., and Ahrens, L. (2019). Efficient Removal of Per- and Polyfluoroalkyl Substances (PFASs) in Drinking Water Treatment: Nanofiltration Combined with Active Carbon or Anion Exchange. Environ. Sci. Water Res. Technol. 5, 1836–1843. doi:10.1039/c9ew00286c
Frawley, R. P., Smith, M., Cesta, M. F., Hayes-Bouknight, S., Blystone, C., Kissling, G. E., et al. (2018). Immunotoxic and Hepatotoxic Effects of Perfluoro-N-Decanoic Acid (PFDA) on Female Harlan Sprague-Dawley Rats and B6C3F1/N Mice when Administered by Oral Gavage for 28 Days. J. Immunotoxicol. 15, 41–52. doi:10.1080/1547691X.2018.1445145
Gaballah, S., Swank, A., Sobus, J. R., Howey, X. M., Schmid, J., Catron, T., et al. (2020). Evaluation of Developmental Toxicity, Developmental Neurotoxicity, and Tissue Dose in Zebrafish Exposed to genX and Other PFAS. Environ. Health Perspect. 128, 047005–047022. doi:10.1289/EHP5843
Gagliano, E., Sgroi, M., Falciglia, P. P., Vagliasindi, F. G. A., and Roccaro, P. (2020). Removal of Poly- and Perfluoroalkyl Substances (PFAS) from Water by Adsorption: Role of PFAS Chain Length, Effect of Organic Matter and Challenges in Adsorbent Regeneration. Water Res. 171, 115381. doi:10.1016/j.watres.2019.115381
Gao, X., Ni, W., Zhu, S., Wu, Y., Cui, Y., Ma, J., et al. (2021). Per- and Polyfluoroalkyl Substances Exposure during Pregnancy and Adverse Pregnancy and Birth Outcomes: A Systematic Review and Meta-Analysis. Environ. Res. 201, 111632. doi:10.1016/j.envres.2021.111632
Glüge, J., Scheringer, M., Cousins, I. T., Dewitt, J. C., Goldenman, G., Herzke, D., et al. (2020). An Overview of the Uses of Per- and Polyfluoroalkyl Substances (PFAS). Environ. Sci. Process. Impacts 22, 2345–2373. doi:10.1039/d0em00291g
Gobelius, L., Hedlund, J., Dürig, W., Tröger, R., Lilja, K., Wiberg, K., et al. (2018). Per- and Polyfluoroalkyl Substances in Swedish Groundwater and Surface Water: Implications for Environmental Quality Standards and Drinking Water Guidelines. Environ. Sci. Technol. 52, 4340–4349. doi:10.1021/acs.est.7b05718
Gole, V. L., Fishgold, A., Sierra-Alvarez, R., Deymier, P., and Keswani, M. (2018). Treatment of Perfluorooctane Sulfonic Acid (PFOS) Using a Large-Scale Sonochemical Reactor. Sep. Purif. Technol. 194 (7), 104–110. doi:10.1016/j.seppur.2017.11.009
Gong, J., Fang, T., Peng, D., Li, A., and Zhang, L. (2015). A Highly Sensitive Photoelectrochemical Detection of Perfluorooctanic Acid with Molecularly Imprined Polymer-Functionalized Nanoarchitectured Hybrid of AgI-BiOI Composite. Biosens. Bioelectron. 73, 256–263. doi:10.1016/j.bios.2015.06.008
Gowen, A. A., Marini, F., Tsuchisaka, Y., De Luca, S., Bevilacqua, M., O’Donnell, C., et al. (2015). On the Feasibility of Near Infrared Spectroscopy to Detect Contaminants in Water Using Single Salt Solutions as Model Systems. Talanta 131, 609–618. doi:10.1016/j.talanta.2014.08.049
Grandjean, P., Andersen, E. W., Budtz-Jørgensen, E., Nielsen, F., Mølbak, K., Weihe, P., et al. (2012). Serum Vaccine Antibody Concentrations in Children Exposed to Perfluorinated Compounds. Jama 307, 391–397. doi:10.1001/jama.2011.2034
Grieshaber, D., MacKenzie, R., Vörös, J., and Reimhult, E. (2008). Electrochemical Biosensors - Sensor Principles and Architectures. Sensors 8, 1400–1458. doi:10.3390/s8031400
Guelfo, J. L., Marlow, T., Klein, D. M., Savitz, D. A., Frickel, S., Crimi, M., et al. (2018). Evaluation and Management Strategies for Per- and Polyfluoroalkyl Substances (PFASs) in Drinking Water Aquifers: Perspectives from Impacted U.S. Northeast Communities. Environ. Health Perspect. 126 (6), 065001. doi:10.1289/EHP2727
Guo, R., Liu, X., Liu, J., Liu, Y., Qiao, X., Ma, M., et al. (2020). Occurrence, Partition and Environmental Risk Assessment of Per- and Polyfluoroalkyl Substances in Water and Sediment from the Baiyangdian Lake, China. Sci. Rep. 10, 1–11. doi:10.1038/s41598-020-61651-6
Gützkow, K. B., Haug, L. S., Thomsen, C., Sabaredzovic, A., Becher, G., and Brunborg, G. (2012). Placental Transfer of Perfluorinated Compounds Is Selective - A Norwegian Mother and Child Sub-cohort Study. Int. J. Hyg. Environ. Health 215, 216–219. doi:10.1016/j.ijheh.2011.08.011
Hernández, F., Bakker, J., Bijlsma, L., de Boer, J., Botero-Coy, A. M., Bruinen de Bruin, Y., et al. (2019). The Role of Analytical Chemistry in Exposure Science: Focus on the Aquatic Environment. Chemosphere 222, 564–583. doi:10.1016/j.chemosphere.2019.01.118
Hitchcock, D. J., Andersen, T., Varpe, Ø., Loonen, M. J. J. E., Warner, N. A., Herzke, D., et al. (2019). Potential Effect of Migration Strategy on Pollutant Occurrence in Eggs of Arctic Breeding Barnacle Geese (Branta leucopsis). Environ. Sci. Technol. 53, 5427–5435. doi:10.1021/acs.est.9b00014
Hong, Y., Oh, J., Lee, I., Fan, C., Pan, S.-Y., Jang, M., et al. (2021). Total-organic-carbon-based Quantitative Estimation of Microplastics in Sewage. Chem. Eng. J. 423, 130182. doi:10.1016/j.cej.2021.130182
Huang, M. C., Dzierlenga, A. L., Robinson, V. G., Waidyanatha, S., DeVito, M. J., Eifrid, M. A., et al. (2019a). Toxicokinetics of Perfluorobutane Sulfonate (PFBS), Perfluorohexane-1-Sulphonic Acid (PFHxS), and Perfluorooctane Sulfonic Acid (PFOS) in Male and Female Hsd:Sprague Dawley SD Rats after Intravenous and Gavage Administration. Toxicol. Rep. 6, 645–655. doi:10.1016/j.toxrep.2019.06.016
Huang, M. C., Robinson, V. G., Waidyanatha, S., Dzierlenga, A. L., DeVito, M. J., Eifrid, M. A., et al. (2019b). Toxicokinetics of 8:2 Fluorotelomer Alcohol (8:2-FTOH) in Male and Female Hsd:Sprague Dawley SD Rats after Intravenous and Gavage Administration. Toxicol. Rep. 6, 924–932. doi:10.1016/j.toxrep.2019.08.009
Jaffrezic-Renault, N., and Dzyadevych, S. (2008). Conductometric Microbiosensors for Environmental Monitoring. Sensors 8, 2569–2588. doi:10.3390/s8042569
Jha, P. K., and Tripathi, P. (2021). Arsenic and Fluoride Contamination in Groundwater: a Review of Global Scenarios with Special Reference to India. Groundw. Sustain. Dev. 13 (13), 100576. doi:10.1016/j.gsd.2021.100576
Jia, X.-X., Li, S., Han, D.-P., Chen, R., Yao, Z.-Y., Ning, B., et al. (2021). Development and Perspectives of Rapid Detection Technology in Food and Environment. Crit. Rev. Food Sci. Nutr. 0, 1–20. doi:10.1080/10408398.2021.1878101
Karimi-Maleh, H., Orooji, Y., Karimi, F., Alizadeh, M., Baghayeri, M., Rouhi, J., et al. (2021). A Critical Review on the Use of Potentiometric Based Biosensors for Biomarkers Detection. Biosens. Bioelectron. 184, 113252. doi:10.1016/j.bios.2021.113252
Karimian, N., Stortini, A. M., Moretto, L. M., Costantino, C., Bogialli, S., and Ugo, P. (2018). Electrochemosensor for Trace Analysis of Perfluorooctanesulfonate in Water Based on a Molecularly Imprinted Poly(o-Phenylenediamine) Polymer. ACS Sens. 3, 1291–1298. doi:10.1021/acssensors.8b00154
Kato, K., Kalathil, A. A., Patel, A. M., Ye, X., and Calafat, A. M. (2018). Per- and Polyfluoroalkyl Substances and Fluorinated Alternatives in Urine and Serum by On-Line Solid Phase Extraction-Liquid Chromatography-Tandem Mass Spectrometry. Chemosphere 209, 338–345. doi:10.1016/j.chemosphere.2018.06.085
KemI, O. 2015. "Studies Have Associated the Presence," in Occurrence and Use of Highly 717 Fluorinated Substances and Alternatives. Swedish Chemicals Agency.
Kniggendorf, A.-K., Wetzel, C., and Roth, B. (2019). Microplastics Detection in Streaming Tap Water with Raman Spectroscopy. Sensors 19, 1839. doi:10.3390/s19081839
Kucharzyk, K. H., Darlington, R., Benotti, M., Deeb, R., and Hawley, E. (2017). Novel Treatment Technologies for PFAS Compounds: A Critical Review. J. Environ. Manag. 204, 757–764. doi:10.1016/j.jenvman.2017.08.016
Kundu, S., Patel, S., Halder, P., Patel, T., Hedayati Marzbali, M., Pramanik, B. K., et al. (2021). Removal of PFASs from Biosolids Using a Semi-pilot Scale Pyrolysis Reactor and the Application of Biosolids Derived Biochar for the Removal of PFASs from Contaminated Water. Environ. Sci. Water Res. Technol. 7, 638–649. doi:10.1039/D0EW00763C
Kurwadkar, S., Dane, J., Kanel, S. R., Nadagouda, M. N., Cawdrey, R. W., Ambade, B., et al. (2022). Per- and Polyfluoroalkyl Substances in Water and Wastewater: A Critical Review of Their Global Occurrence and Distribution. Sci. Total Environ. 809, 151003. doi:10.1016/j.scitotenv.2021.151003
Kwiatkowski, C. F., Andrews, D. Q., Birnbaum, L. S., Bruton, T. A., DeWitt, J. C., Knappe, D. R. U., et al. (2020). Scientific Basis for Managing PFAS as A Chemical Class. Environ. Sci. Technol. Lett. 7 (8), 532–543. doi:10.1021/acs.estlett.0c00255
Kwon, B. G., Lim, H.-J., Na, S.-H., Choi, B.-I., Shin, D.-S., and Chung, S.-Y. (2014). Biodegradation of Perfluorooctanesulfonate (PFOS) as an Emerging Contaminant. Chemosphere 109, 221–225. doi:10.1016/j.chemosphere.2014.01.072
Lei, Y.-J., Tian, Y., Sobhani, Z., Naidu, R., and Fang, C. (2020). Synergistic Degradation of PFAS in Water and Soil by Dual-Frequency Ultrasonic Activated Persulfate. Chem. Eng. J. 388 (1), 124215. doi:10.1016/j.cej.2020.124215
Lenka, S. P., Kah, M., and Padhye, L. P. (2021). A Review of the Occurrence, Transformation, and Removal of Poly- and Perfluoroalkyl Substances (PFAS) in Wastewater Treatment Plants. Water Res. 199, 117187. doi:10.1016/j.watres.2021.117187
Li, J., Liu, H., and Paul Chen, J. (2018). Microplastics in Freshwater Systems: A Review on Occurrence, Environmental Effects, and Methods for Microplastics Detection. Water Res. 137, 362–374. doi:10.1016/j.watres.2017.12.056
Li, M., Yu, Z., Liu, Q., Sun, L., and Huang, W. (2016). Photocatalytic Decomposition of Perfluorooctanoic Acid by Noble Metallic Nanoparticles Modified TiO 2. Chem. Eng. J. 286, 232–238. doi:10.1016/j.cej.2015.10.037
Li, N., Ying, G.-G., Hong, H., and Deng, W.-J. (2021). Perfluoroalkyl Substances in the Urine and Hair of Preschool Children, Airborne Particles in Kindergartens, and Drinking Water in Hong Kong. Environ. Pollut. 270, 116219. doi:10.1016/j.envpol.2020.116219
Liu, C. J., Strathmann, T. J., and Bellona, C. (2021). Rejection of Per- and Polyfluoroalkyl Substances (PFASs) in Aqueous Film-Forming Foam by High-Pressure Membranes. Water Res. 188, 116546. doi:10.1016/J.WATRES.2020.116546
Liu, H., Hu, W., Li, X., Hu, F., Liu, Y., Xie, T., et al. (2022). Effects of Perfluoroalkyl Substances on Root and Rhizosphere Bacteria: Phytotoxicity, Phyto-Microbial Remediation, Risk Assessment. Chemosphere 289, 133137. doi:10.1016/j.chemosphere.2021.133137
Liu, J., Du, J., Su, Y., and Zhao, H. (2019). A Facile Solvothermal Synthesis of 3D Magnetic MoS2/Fe3O4 Nanocomposites with Enhanced Peroxidase-Mimicking Activity and Colorimetric Detection of Perfluorooctane Sulfonate. Microchem. J. 149, 104019. doi:10.1016/j.microc.2019.104019
Liu, Q., Zhou, Q., and Jiang, G. (2014). Nanomaterials for Analysis and Monitoring of Emerging Chemical Pollutants. TrAC Trends Anal. Chem. 58, 10–22. doi:10.1016/j.trac.2014.02.014
Logeshwaran, P., Sivaram, A. K., Surapaneni, A., Kannan, K., Naidu, R., and Megharaj, M. (2021). Exposure to Perfluorooctanesulfonate (PFOS) but Not Perflurorooctanoic Acid (PFOA) at Ppb Concentration Induces Chronic Toxicity in Daphnia Carinata. Sci. Total Environ. 769, 144577. doi:10.1016/j.scitotenv.2020.144577
Lu, D., Sha, S., Luo, J., Huang, Z., and Zhang Jackie, X. (2020). Treatment Train Approaches for the Remediation of Per- and Polyfluoroalkyl Substances (PFAS): A Critical Review. J. Hazard. Mater. 386, 121963. doi:10.1016/j.jhazmat.2019.121963
Lu, L.-P., Wang, F., Kang, T.-F., Cheng, S.-Y., and Wang, Y.-T. (2011). Electrochemical Deoxyribonucleic Acid Damage Treated with Perfluorinated Compounds on Peroxide Polypyrrole Modified Gold Electrode. Chin. J. Anal. Chem. 39, 392–396. doi:10.1016/S1872-2040(10)60426-4
Marchetti, M., Offroy, M., Abdat, F., Branchu, P., Bourson, P., Jobard, C., et al. (2020). Chemometrics-Assisted Monitoring in Raman Spectroscopy for the Biodegradation Process of an Aqueous Polyfluoroalkyl Ether from a Fire-Fighting Foam in an Environmental Matrix. Environments 7, 4. doi:10.3390/environments7010004
Mazhar, M. A., Khan, N. A., Ahmed, S., Khan, A. H., Hussain, A., Rahisuddin, , et al. (2020). Chlorination Disinfection By-Products in Municipal Drinking Water - A Review. J. Clean. Prod. 273, 123159. doi:10.1016/j.jclepro.2020.123159 https://www.sciencedirect.com/science/article/abs/pii/S0959652620332042.
McCord, J. P., Strynar, M. J., Washington, J. W., Bergman, E. L., and Goodrow, S. M. (2020). Emerging Chlorinated Polyfluorinated Polyether Compounds Impacting the Waters of Southwestern New Jersey Identified by Use of Nontargeted Analysis. Environ. Sci. Technol. Lett. 7, 903–908. doi:10.1021/ACS.ESTLETT.0C00640/SUPPL_FILE/EZ0C00640_SI_001.PDF
Menger, R. F., Funk, E., Henry, C. S., and Borch, T. (2021). Sensors for Detecting Per- and Polyfluoroalkyl Substances (PFAS): A Critical Review of Development Challenges, Current Sensors, and Commercialization Obstacles. Chem. Eng. J. 417, 129133. doi:10.1016/j.cej.2021.129133
Mesquita, D. P., Quintelas, C., Amaral, A. L., and Ferreira, E. C. (2017). Monitoring Biological Wastewater Treatment Processes: Recent Advances in Spectroscopy Applications. Rev. Environ. Sci. Biotechnol. 16, 395–424. doi:10.1007/s11157-017-9439-9
Militao, I. M., Roddick, F. A., Bergamasco, R., and Fan, L. (2021). Removing PFAS from Aquatic Systems Using Natural and Renewable Material-Based Adsorbents: A Review. J. Environ. Chem. Eng. 9, 105271. doi:10.1016/J.JECE.2021.105271
Mohamed, B. A., Li, L. Y., Hamid, H., and Jeronimo, M. (2022). Sludge-based Activated Carbon and its Application in the Removal of Perfluoroalkyl Substances: A Feasible Approach towards a Circular Economy. Chemosphere 294, 133707. doi:10.1016/J.CHEMOSPHERE.2022.133707
Moro, G., Bottari, F., Liberi, S., Covaceuszach, S., Cassetta, A., Angelini, A., et al. (2020). Covalent Immobilization of Delipidated Human Serum Albumin on Poly(pyrrole-2-Carboxylic) Acid Film for the Impedimetric Detection of Perfluorooctanoic Acid. Bioelectrochemistry 134, 107540. doi:10.1016/j.bioelechem.2020.107540
Moro, G., Cristofori, D., Bottari, F., Cattaruzza, E., De Wael, K., and Moretto, L. M. (2019). Redesigning an Electrochemical MIP Sensor for PFOS: Practicalities and Pitfalls. Sensors 19, 4433. doi:10.3390/s19204433
Mukhopadhyay, R., Sarkar, B., Palansooriya, K. N., Dar, J. Y., Bolan, N. S., Parikh, S. J., et al. (2021). Natural and Engineered Clays and Clay Minerals for the Removal of Poly- and Perfluoroalkyl Substances from Water: State-Of-The-Art and Future Perspectives. Adv. Colloid Interface Sci. 297, 102537. doi:10.1016/j.cis.2021.102537
National Toxicology Program (NTP) (2020). NTP Technical Report on the Toxicology and Carcinogenesis Studies of Perfluorooctanoic Acid (CASRN 335-67-1) Administered in Feed to Sprague Dawley (Hsd:Sprague Dawley® SD®) Rats. Durham, NC 27709: NTP Technical Report. 111 TW Alexander Dr. doi:10.22427/NTP-TR-598
National Toxicology Program (NTP) (2021a). TOX-96: 1-Perfluorobutanesulfonic Acid (375-73-5), Potassium Perfluorohexanesulfonate (3871-99-6), Perfluorooctane Sulfonate (1763-23-1), WY-14643 (50892-23-4). Research Triangle Park, NC (USA): National Institute of Environmental Health Sciences. Available at: https://cebs.niehs.nih.gov/cebs/publication/TOX-96 (Accessed January 19, 2022).
National Toxicology Program (NTP) (2021b). TOX-97: Perfluorohexanoic Acid (307-24-4), Perfluorooctanoic Acid (335-67-1), Perfluorononanoic Acid (375-95-1), Perfluorodecanoic Acid (335-76-2), WY-14643 (50892-23-4). Research Triangle Park, NC (USA): National Institute of Environmental Health Sciences. Available at: https://cebs.niehs.nih.gov/cebs/publication/TOX-97 (Accessed January 19, 2022).
Niu, H., Wang, S., Zhou, Z., Ma, Y., Ma, X., and Cai, Y. (2014). Sensitive Colorimetric Visualization of Perfluorinated Compounds Using Poly(ethylene Glycol) and Perfluorinated Thiols Modified Gold Nanoparticles. Anal. Chem. 86, 4170–4177. doi:10.1021/ac403406d
Niu, Z., Na, J., Xu, W. a., Wu, N., and Zhang, Y. (2019). The Effect of Environmentally Relevant Emerging Per- and Polyfluoroalkyl Substances on the Growth and Antioxidant Response in Marine Chlorella Sp. Environ. Pollut. 252, 103–109. doi:10.1016/j.envpol.2019.05.103
Nzeribe, B. N., Crimi, M., Mededovic Thagard, S., and Holsen, T. M. (2019). Physico-Chemical Processes for the Treatment of Per- and Polyfluoroalkyl Substances (PFAS): A Review. Crit. Rev. Environ. Sci. Technol. 49, 866–915. doi:10.1080/10643389.2018.1542916
OECD (2018). Toward a New Comprehensive Global Database of Per-And Polyfluoroalkyl Substances (PFASs): Summary Report on Updating the OECD 2007 List of Per-And Polyfluoroalkyl Substances (PFASs). Available at: https://www.oecd.org/officialdocuments/publicdisplaydocumentpdf/?cote=ENV-JM-MONO(2018)7anddoclanguage=en (Accessed January 26, 2022).
Ong, T. T. X., Blanch, E. W., and Jones, O. A. H. (2020). Surface Enhanced Raman Spectroscopy in Environmental Analysis, Monitoring and Assessment. Sci. Total Environ. 720, 137601. doi:10.1016/j.scitotenv.2020.137601
Panda, D., Sethu, V., and Manickam, S. (2019). Kinetics and Mechanism of Low-Frequency Ultrasound Driven Elimination of Trace Level Aqueous Perfluorooctanesulfonic Acid and Perfluorooctanoic Acid. Chem. Eng. Process. - Process Intensif. 142 (11), 107542. doi:10.1016/j.cep.2019.107542
Park, J., Hodges, K. L., Tumuluri, U., Zaki, A. A., Dussor, J. C., Daunis, T., et al. (2021). “Infrared Sensors for Environmental and Biomedical Applications,” in Optical Fibers and Sensors for Medical Diagnostics, Treatment and Environmental Applications XXI (Washington: SPIE), 157–165. doi:10.1117/12.2583757
Paumo, H. K., Dalhatou, S., Katata-Seru, L. M., Kamdem, B. P., Tijani, J. O., Vishwanathan, V., et al. (2021). TiO2 Assisted Photocatalysts for Degradation of Emerging Organic Pollutants in Water and Wastewater. J. Mol. Liq. 331, 115458. doi:10.1016/j.molliq.2021.115458
Peng, L., Xu, W., Zeng, Q., Sun, F., Guo, Y., Zhong, S., et al. (2022). Exposure to Perfluoroalkyl Substances in Waste Recycling Workers: Distributions in Paired Human Serum and Urine. Environ. Int. 158, 106963. doi:10.1016/j.envint.2021.106963
Peng, M.-W., Wei, X.-Y., Yu, Q., Yan, P., Chen, Y.-P., and Guo, J.-S. (2019). Identification of Ceftazidime Interaction with Bacteria in Wastewater Treatment by Raman Spectroscopic Mapping. RSC Adv. 9, 32744–32752. doi:10.1039/C9RA06006E
Pennings, J. L. A., Jennen, D. G. J., Nygaard, U. C., Namork, E., Haug, L. S., van Loveren, H., et al. (2016). Cord Blood Gene Expression Supports that Prenatal Exposure to Perfluoroalkyl Substances Causes Depressed Immune Functionality in Early Childhood. J. Immunotoxicol. 13, 173–180. doi:10.3109/1547691X.2015.1029147
Phong Vo, H. N., Ngo, H. H., Guo, W., Hong Nguyen, T. M., Li, J., Liang, H., et al. (2020). Poly and Perfluoroalkyl Substances in Water and Wastewater: A Comprehensive Review from Sources to Remediation. J. Water Process Eng. 36, 101393. doi:10.1016/J.JWPE.2020.101393
Pierpaoli, M., Szopińska, M., Wilk, B. K., Sobaszek, M., Łuczkiewicz, A., Bogdanowicz, R., et al. (2021). Electrochemical Oxidation of PFOA and PFOS in Landfill Leachates at Low and Highly Boron-Doped Diamond Electrodes. J. Hazard. Mater. 403, 123606. doi:10.1016/j.jhazmat.2020.123606
Rericha, Y., Cao, D., Truong, L., Simonich, M., Field, J. A., and Tanguay, R. L. (2021). Behavior Effects of Structurally Diverse Per- and Polyfluoroalkyl Substances in Zebrafish. Chem. Res. Toxicol. 34, 1409–1416. doi:10.1021/acs.chemrestox.1c00101
Reichl, C., and Schatz, M. (2019). World Mining Data 2019. Int. Organ. Comm. World Min. Congr. 34, 1–262.
Rodriguez, K. L., Hwang, J.-H., Esfahani, A. R., Sadmani, A. H. M. A., and Lee, W. H. (2020). Recent Developments of PFAS-Detecting Sensors and Future Direction: A Review. Micromachines 11, 667. doi:10.3390/mi11070667
Rodríguez-Delgado, M. M., Alemán-Nava, G. S., Rodríguez-Delgado, J. M., Dieck-Assad, G., Martínez-Chapa, S. O., Barceló, D., et al. (2015). Laccase-based Biosensors for Detection of Phenolic Compounds. TrAC Trends Anal. Chem. 74, 21–45. doi:10.1016/j.trac.2015.05.008
Rout, P. R., Zhang, T. C., Bhunia, P., and Surampalli, R. Y. (2021). Treatment Technologies for Emerging Contaminants in Wastewater Treatment Plants: A Review. Sci. Total Environ. 753, 141990. doi:10.1016/J.SCITOTENV.2020.141990
Shaw, D. M. J., Munoz, G., Bottos, E. M., Duy, S. V., Sauvé, S., Liu, J., et al. (2019). Degradation and Defluorination of 6:2 Fluorotelomer Sulfonamidoalkyl Betaine and 6:2 Fluorotelomer Sulfonate by Gordonia Sp. Strain NB4-1Y under Sulfur-Limiting Conditions. Sci. Total Environ. 647, 690–698. doi:10.1016/j.scitotenv.2018.08.012
Silver, A., Kitadai, H., Liu, H., Granzier-Nakajima, T., Terrones, M., Ling, X., et al. (2019). Chemical and Bio Sensing Using Graphene-Enhanced Raman Spectroscopy. Nanomaterials 9, 516. doi:10.3390/nano9040516
Singh, R. K., Fernando, S., Baygi, S. F., Multari, N., Thagard, S. M., and Holsen, T. M. (2019). Breakdown Products from Perfluorinated Alkyl Substances (PFAS) Degradation in a Plasma-Based Water Treatment Process. Environ. Sci. Technol. 53, 2731–2738. doi:10.1021/acs.est.8b07031
Sobhani, Z., Al Amin, M., Naidu, R., Megharaj, M., and Fang, C. (2019). Identification and Visualisation of Microplastics by Raman Mapping. Anal. Chim. Acta 1077, 191–199. doi:10.1016/j.aca.2019.05.021
Solanki, Y. S., Agarwal, M., Gupta, A. B., Gupta, S., and Shukla, P. (2022). Fluoride Occurrences, Health Problems, Detection, and Remediation Methods for Drinking Water: A Comprehensive Review. Sci. Total Environ. 807 (1), 150601. doi:10.1016/j.scitotenv.2021.150601
Soldatkina, O. V., Soldatkin, O. O., Velychko, T. P., Prilipko, V. O., Kuibida, M. A., and Dzyadevych, S. V. (2018). Conductometric Biosensor for Arginine Determination in Pharmaceutics. Bioelectrochemistry 124, 40–46. doi:10.1016/j.bioelechem.2018.07.002
Soriano, Á., Gorri, D., and Urtiaga, A. (2017). Efficient Treatment of Perfluorohexanoic Acid by Nanofiltration Followed by Electrochemical Degradation of the NF Concentrate. Water Res. 112, 147–156. doi:10.1016/J.WATRES.2017.01.043
Speed, D. E. (2016). “Environmental Aspects of Planarization Processes,” in Advances in Chemical Mechanical Planarization (CMP) (Elsevier), 229–269. doi:10.1016/B978-0-08-100165-3.00010-3
Steigerwald, J. M., and Ray, J. R. (2021). Adsorption Behavior of Perfluorooctanesulfonate (PFOS) onto Activated Spent Coffee Grounds Biochar in Synthetic Wastewater Effluent. J. Hazard. Mater. Lett. 2, 100025. doi:10.1016/J.HAZL.2021.100025
Stoiber, T., Evans, S., and Naidenko, O. V. (2020). Disposal of Products and Materials Containing Per- and Polyfluoroalkyl Substances (PFAS): A Cyclical Problem. Chemosphere 260, 127659. doi:10.1016/j.chemosphere.2020.127659
Sukeesan, S., Boontanon, N., and Boontanon, S. K. (2021). Improved Electrical Driving Current of Electrochemical Treatment of Per- and Polyfluoroalkyl Substances (PFAS) in Water Using Boron-Doped Diamond Anode. Environ. Technol. Innovation 23, 101655. doi:10.1016/j.eti.2021.101655
Sunderland, E. M., Hu, X. C., Dassuncao, C., Tokranov, A. K., Wagner, C. C., and Allen, J. G. (2019). A Review of the Pathways of Human Exposure to Poly- and Perfluoroalkyl Substances (PFASs) and Present Understanding of Health Effects. J. Expo. Sci. Environ. Epidemiol. 29, 131–147. doi:10.1038/s41370-018-0094-1
Tagg, A. S., Harrison, J. P., Ju-Nam, Y., Sapp, M., Bradley, E. L., Sinclair, C. J., et al. (2017). Fenton's Reagent for the Rapid and Efficient Isolation of Microplastics from Wastewater. Chem. Commun. 53, 372–375. doi:10.1039/C6CC08798A
Takayose, M., Akamatsu, K., Nawafune, H., Murashima, T., and Matsui, J. (2012). Colorimetric Detection of Perfluorooctanoic Acid (PFOA) Utilizing Polystyrene-Modified Gold Nanoparticles. Anal. Lett. 45, 2856–2864. doi:10.1080/00032719.2012.696225
Tang, C. Y., Fu, Q. S., Robertson, A. P., Criddle, C. S., and Leckie, J. O. (2006). Use of Reverse Osmosis Membranes to Remove Perfluorooctane Sulfonate (PFOS) from Semiconductor Wastewater. Environ. Sci. Technol. 40, 7343–7349. doi:10.1021/ES060831Q/SUPPL_FILE/ES060831QSI20060801_013646.PDF
Tran, T., Abrell, L., Brusseau, M. L., and Chorover, J. (2021). Iron-activated Persulfate Oxidation Degrades Aqueous Perfluorooctanoic Acid (PFOA) at Ambient Temperature. Chemosphere 281 (5), 130824. doi:10.1016/j.chemosphere.2021.130824
Truong, L., Rericha, Y., Thunga, P., Marvel, S., Wallis, D., Simonich, M. T., et al. (2022). Systematic Developmental Toxicity Assessment of a Structurally Diverse Library of PFAS in Zebrafish. J. Hazard. Mater. 431, 128615. doi:10.1016/j.jhazmat.2022.128615
Uniyal, S., and Sharma, R. K. (2018). Technological Advancement in Electrochemical Biosensor Based Detection of Organophosphate Pesticide Chlorpyrifos in the Environment: A Review of Status and Prospects. Biosens. Bioelectron. 116, 37–50. doi:10.1016/j.bios.2018.05.039
vanden Heuvel, J. P., Thompson, J. T., Frame, S. R., and Gillies, P. J. (2006). Differential Activation of Nuclear Receptors by Perfluorinated Fatty Acid Analogs and Natural Fatty Acids: A Comparison of Human, Mouse, and Rat Peroxisome Proliferator-Activated Receptor-α, -β, and -γ, Liver X Receptor-β, and Retinoid X Receptor-α. Toxicol. Sci. 92, 476–489. doi:10.1093/toxsci/kfl014
Verner, M.-A., Loccisano, A. E., Morken, N.-H., Yoon, M., Wu, H., McDougall, R., et al. (2015). Associations of Perfluoroalkyl Substances (PFAS) with Lower Birth Weight: An Evaluation of Potential Confounding by Glomerular Filtration Rate Using a Physiologically Based Pharmacokinetic Model (PBPK). Environ. Health Perspect. 123, 1317–1324. doi:10.1289/ehp.1408837
Vikesland, P. J. (2018). Nanosensors for Water Quality Monitoring. Nat. Nanotech 13, 651–660. doi:10.1038/s41565-018-0209-9
Vu, C. T., and Wu, T. (2022). Recent Progress in Adsorptive Removal of Per- and Poly-Fluoroalkyl Substances (PFAS) from Water/wastewater. Crit. Rev. Environ. Sci. Technol. 52, 90–129. doi:10.1080/10643389.2020.1816125
Wahlström, M., Pohjalainen, E., Yli-Rantala, E., Behringer, D., Herzke, D., Mudge, S. M., et al. (2021). Fluorinated Polymers in a Low Carbon, Circular and Toxic-free Economy Technical Report. ETC/WMGE 2021/9. Boeretang, Belgium: European Environment Agency.
Wallace, K. B., Kissling, G. E., Melnick, R. L., and Blystone, C. R. (2013). Structure-activity Relationships for Perfluoroalkane-Induced In Vitro Interference with Rat Liver Mitochondrial Respiration. Toxicol. Lett. 222, 257–264. doi:10.1016/j.toxlet.2013.07.025
Wallace, S., Lambrakos, S., Shabaev, A., and Massa, L. (2021). Calculated IR Absorption Spectra for Perfluoroalkyl and Polyfluoroalkyl (PFAS) Molecules. Struct. Chem. 32, 899–907. doi:10.1007/s11224-021-01738-6
Wang, Y., Darling, S. B., and Chen, J. (2021). Selectivity of Per- and Polyfluoroalkyl Substance Sensors and Sorbents in Water. ACS Appl. Mat. Interfaces 13 (51), 60789–60814. doi:10.1021/acsami.1c16517
Wanninayake, D. M. (2021). Comparison of Currently Available PFAS Remediation Technologies in Water: A Review. J. Environ. Manag. 283 (10), 111977. doi:10.1016/j.jenvman.2021.111977
Wei, H., Hossein Abtahi, S. M., and Vikesland, P. J. (2015). Plasmonic Colorimetric and SERS Sensors for Environmental Analysis. Environ. Sci. Nano 2, 120–135. doi:10.1039/C4EN00211C
Winchell, L. J., Wells, M. J. M., Ross, J. J., Fonoll, X., Norton, J. W., Kuplicki, S., et al. (2022). Per- and Polyfluoroalkyl Substances Presence, Pathways, and Cycling through Drinking Water and Wastewater Treatment. J. Environ. Eng. 148, 03121003. doi:10.1061/(ASCE)EE.1943-7870.0001943
Winchell, L. J., Wells, M. J. M., Ross, J. J., Fonoll, X., Norton, J. W., Kuplicki, S., et al. (2021). Analyses of Per- and Polyfluoroalkyl Substances (PFAS) through the Urban Water Cycle: Toward Achieving an Integrated Analytical Workflow across Aqueous, Solid, and Gaseous Matrices in Water and Wastewater Treatment. Sci. Total Environ. 774, 145257. doi:10.1016/j.scitotenv.2021.145257
Wongniramaikul, W., Limsakul, W., and Choodum, A. (2018). A Biodegradable Colorimetric Film for Rapid Low-Cost Field Determination of Formaldehyde Contamination by Digital Image Colorimetry. Food Chem. 249, 154–161. doi:10.1016/j.foodchem.2018.01.021
Wu, D., Li, X., Tang, Y., Lu, P., Chen, W., Xu, X., et al. (2017). Mechanism Insight of PFOA Degradation by ZnO Assisted-Photocatalytic Ozonation: Efficiency and Intermediates. Chemosphere 180, 247–252. doi:10.1016/j.chemosphere.2017.03.127
Wu, D., Li, X., Zhang, J., Chen, W., Lu, P., Tang, Y., et al. (2018). Efficient PFOA Degradation by Persulfate-Assisted Photocatalytic Ozonation. Sep. Purif. Technol. 207 (June), 255–261. doi:10.1016/j.seppur.2018.06.059
Wu, S., Guo, D., Xu, X., Pan, J., and Niu, X. (2020). Colorimetric Quantification and Discrimination of Phenolic Pollutants Based on Peroxidase-like Fe3O4 Nanoparticles. Sensors Actuators B Chem. 303, 127225. doi:10.1016/j.snb.2019.127225
Xiao, X., Ulrich, B. A., Chen, B., and Higgins, C. P. (2017). Sorption of Poly- and Perfluoroalkyl Substances (PFASs) Relevant to Aqueous Film-Forming Foam (AFFF)-Impacted Groundwater by Biochars and Activated Carbon. Environ. Sci. Technol. 51, 6342–6351. doi:10.1021/ACS.EST.7B00970/SUPPL_FILE/ES7B00970_SI_001.PDF
Xu, B., Ahmed, M. B., Zhou, J. L., Altaee, A., Wu, M., and Xu, G. (2017). Photocatalytic Removal of Perfluoroalkyl Substances from Water and Wastewater: Mechanism, Kinetics and Controlling Factors. Chemosphere 189, 717–729. doi:10.1016/j.chemosphere.2017.09.110
Xu, J.-L., Thomas, K. V., Luo, Z., and Gowen, A. A. (2019). FTIR and Raman Imaging for Microplastics Analysis: State of the Art, Challenges and Prospects. TrAC Trends Anal. Chem. 119, 115629. doi:10.1016/j.trac.2019.115629
Yang Js, J.-S., Lai, W. W.-P., Panchangam, S. C., and Lin, A. Y.-C. (2020). Photoelectrochemical Degradation of Perfluorooctanoic Acid (PFOA) with GOP25/FTO Anodes: Intermediates and Reaction Pathways. J. Hazard. Mater. 391 (2), 122247. doi:10.1016/j.jhazmat.2020.122247
Yang L, L., He, L., Xue, J., Ma, Y., Xie, Z., Wu, L., et al. (2020). Persulfate-based Degradation of Perfluorooctanoic Acid (PFOA) and Perfluorooctane Sulfonate (PFOS) in Aqueous Solution: Review on Influences, Mechanisms and Prospective. J. Hazard. Mater. 393 (2), 122405. doi:10.1016/j.jhazmat.2020.122405
Zhang, T., Zhao, H., Lei, A., and Quan, X. (2014). Electrochemical Biosensor for Detection of Perfluorooctane Sulfonate Based on Inhibition Biocatalysis of Enzymatic Fuel Cell. Electrochemistry 82, 94–99. doi:10.5796/electrochemistry.82.94
Zhang, W., Tooker, N. B., and Mueller, A. V. (2020). Enabling Wastewater Treatment Process Automation: Leveraging Innovations in Real-Time Sensing, Data Analysis, and Online Controls. Environ. Sci. Water Res. Technol. 6, 2973–2992. doi:10.1039/D0EW00394H
Zhang, Z., Sarkar, D., Biswas, J. K., and Datta, R. (2022). Biodegradation of Per- and Polyfluoroalkyl Substances (PFAS): A Review. Bioresour. Technol. 344, 126223. doi:10.1016/j.biortech.2021.126223
Zhao, B., Li, X., Yang, L., Wang, F., Li, J., Xia, W., et al. (2015). ß-Ga2O3Nanorod Synthesis with a One-step Microwave Irradiation Hydrothermal Method and its Efficient Photocatalytic Degradation for Perfluorooctanoic Acid. Photochem. Photobiol. 91 (1), 42–47. doi:10.1111/php.12383
Zheng, Z., Yu, H., Geng, W.-C., Hu, X.-Y., Wang, Y.-Y., Li, Z., et al. (2019). Guanidinocalix[5]arene for Sensitive Fluorescence Detection and Magnetic Removal of Perfluorinated Pollutants. Nat. Commun. 10, 5762. doi:10.1038/s41467-019-13775-1
Zhu, Y., Xu, T., Zhao, D., Li, F., Liu, W., Wang, B., et al. (2021). Adsorption and Solid-phase Photocatalytic Degradation of Perfluorooctane Sulfonate in Water Using Gallium-Doped Carbon-Modified Titanate Nanotubes. Chem. Eng. J. 421 (P1), 129676. doi:10.1016/j.cej.2021.129676
Keywords: PFAS, detection, biosensors, tertiary treatment, WWTP
Citation: Araújo RG, Rodríguez-Hernandéz JA, González-González RB, Macias-Garbett R, Martínez-Ruiz M, Reyes-Pardo H, Hernández Martínez SA, Parra-Arroyo L, Melchor-Martínez EM, Sosa-Hernández JE, Coronado-Apodaca KG, Varjani S, Barceló D, Iqbal HMN and Parra-Saldívar R (2022) Detection and Tertiary Treatment Technologies of Poly-and Perfluoroalkyl Substances in Wastewater Treatment Plants. Front. Environ. Sci. 10:864894. doi: 10.3389/fenvs.2022.864894
Received: 29 January 2022; Accepted: 13 April 2022;
Published: 29 April 2022.
Edited by:
Adalgisa Sinicropi, University of Siena, ItalyReviewed by:
Antonietta Siciliano, University of Naples Federico II, ItalyCopyright © 2022 Araújo, Rodríguez-Hernandéz, González-González, Macias-Garbett, Martínez-Ruiz, Reyes-Pardo, Hernández Martínez, Parra-Arroyo, Melchor-Martínez, Sosa-Hernández, Coronado-Apodaca, Varjani, Barceló, Iqbal and Parra-Saldívar. This is an open-access article distributed under the terms of the Creative Commons Attribution License (CC BY). The use, distribution or reproduction in other forums is permitted, provided the original author(s) and the copyright owner(s) are credited and that the original publication in this journal is cited, in accordance with accepted academic practice. No use, distribution or reproduction is permitted which does not comply with these terms.
*Correspondence: Hafiz M. N. Iqbal, aGFmaXouaXFiYWxAdGVjLm14; Roberto Parra-Saldívar, ci5wYXJyYUB0ZWMubXg=
Disclaimer: All claims expressed in this article are solely those of the authors and do not necessarily represent those of their affiliated organizations, or those of the publisher, the editors and the reviewers. Any product that may be evaluated in this article or claim that may be made by its manufacturer is not guaranteed or endorsed by the publisher.
Research integrity at Frontiers
Learn more about the work of our research integrity team to safeguard the quality of each article we publish.