- 1Eawag, Swiss Federal Institute of Aquatic Science and Technology, Department of Surface Waters, Research and Management, Kastanienbaum, Switzerland
- 2Institute of Biogeochemistry and Pollutant Dynamics, Swiss Federal Institute of Technology, Zurich, Switzerland
Eutrophication is expected to increase methane production in freshwater sediments worldwide over the coming decades. Methane-oxidizing bacteria (MOB) consume a significant fraction of this sedimentary methane, but the factors that control their distributions and activities are not understood. By combining genetic approaches (pmoA, 16S rRNA gene, metagenomics) with geochemical and sedimentological analyses, we investigate the role of trophic state, electron acceptors, oxygen (O2) and methane fluxes, and potential methylotrophic partner organisms in driving the distributions, abundances, and community compositions of MOB across five lakes in central Switzerland. Although methane fluxes were highest in the eutrophic lakes, methanotrophic abundances peaked in oxic and anoxic sediments of an oligotrophic lake. In all lakes, Type I gammaproteobacterial Methylococcaceae dominated oxic and suboxic bottom water and surface sediments, showing strong correlations with abundances of putatively methylotrophic Methylophilaceae, whereas Type II alphaproteobacterial Methylocystaceae increased in deeper, anoxic sediment layers. Methanotrophic bacteria belonging to the NC10 phylum were predominantly detected within denitrifying sediment of the oligotrophic lake, matching their presumed nitrite-dependent lifestyle. While dominant MOB taxa at the genus-level follow vertical distributions of different aerobic and anaerobic respiration reactions, trophic state at the time of sediment deposition was the best predictor of MOB community structure at the operational taxonomic unit (OTU) level. Elevated methane fluxes combined with low MOB abundances in surface sediments of eutrophic lakes, moreover, support the notion that in eutrophic lakes a major portion of sedimentary methane bypasses the biological methane filter and escapes to overlying water.
Introduction
Freshwater lakes account for 6–16% of natural emissions of the greenhouse gas methane (CH4) to the atmosphere (Bastviken et al., 2004). Most of this methane is biologically produced in lake sediments. A fraction of this biologically produced methane escapes into the water column by diffusion, ebullition, and advective processes, and is subsequently emitted to the atmosphere (Tranvik et al., 2009; Bastviken et al., 2011; Dean et al., 2018). Methane emissions from lakes to the atmosphere are predicted to increase due to global temperature increases over the coming decades (Dean et al., 2018; Guo et al., 2020; Zhu et al., 2020).
Besides warming, anthropogenic increases in nutrient inputs that enhance primary production and organic carbon loading (eutrophication), can increase methane emissions from lakes (Beaulieu et al., 2019). Hereby enhanced supplies of algal organic carbon stimulate organic matter (OM) mineralization rates and lead to higher respiration rates of the electron acceptors dioxygen (O2), nitrate (NO3−), sulfate (SO42−), and metal oxides (Fe(III), Mn(IV)) in lake water and surface sediment. The more rapid depletion of these electron acceptors in lake sediments, combined with the higher OM availability, promotes microbial methane production (methanogenesis) (Fiskal et al., 2019) and results in increased sedimentary methane release under eutrophic conditions (Beaulieu et al., 2019; Sanches et al., 2019).
Most microbially produced methane in lake sediments is microbially oxidized in surface sediments before it can escape to the overlying water. Methane oxidation removes from ∼50 to 99% of sedimentary methane, with removal efficiencies being highest in oligotrophic and lowest in eutrophic lakes (D’Ambrosio and Harrison, 2021). Previously, it was believed that lake sedimentary methanotrophy was performed exclusively by aerobic methane-oxidizing bacteria (MOB) within the Alpha- and Gammaproteobacteria (e.g., Auman et al., 2000; Frenzel et al., 1990; Pester et al., 2011; Reim et al., 2012; reviewed in; Knief, 2015). Since then it was shown that Bacteria of the genus Methylomirabilis (phylum: NC10) perform anaerobic methane oxidation coupled to nitrite (NO2−) reduction (denitrification; Ettwig et al., 2010; Deutzmann et al., 2014), while Archaea of the family Methanoperedenaceae (phylum: Euryarchaeota) couple the anaerobic oxidation of methane to the reduction of NO3−, Mn(IV) or Fe(III) (Ettwig et al., 2016; Leu et al., 2020).
Although historically considered to be obligately aerobic, MOB are widespread in O2-depleted sediments, and in some cases even reach their highest gene copy numbers in anoxic subsurface layers (Rahalkar et al., 2009; Pester et al., 2011; Tsutsumi et al., 2011; He et al., 2021; Lyautey et al., 2021). Recently, members of the gammaproteobacterial genera Methylobacter and Methylomonas were shown to oxidize methane syntrophically with denitrifying methylotrophic Betaproteobacteria (Methylophilaceae). Hereby the MOB partially oxidize methane to methanol, H2, or short-chain organic acids (e.g., formate, acetate), which the partner organisms subsequently oxidize to gain energy by denitrification (Beck et al., 2013; Kalyuzhnaya et al., 2013; Oshkin et al., 2014). In addition, several gammaproteobacterial genera (e.g., Methylomonas, Methylobacter, Methylomicrobium) have been shown to directly couple methane oxidation to denitrification under O2-limiting conditions (Kits et al., 2015b; Cao et al., 2019; van Grinsven et al., 2020a). Recently, stimulation of bacterial methane oxidation rates by iron (III), manganese (IV), and humic compounds has been shown (Oswald et al., 2016; He et al., 2021; van Grinsven et al., 2021) and that certain strains of Methylomonas (Gammaproteobacteria) and Methylosinus (Alphaproteobacteria) can use ferrihydrite minerals as electron acceptors to oxidize methane (Zheng et al., 2020).
The community composition of MOB in lake sediments has so far mainly been studied by microbial enrichment and isolation methods (e.g., Auman et al., 2000; He et al., 2012; Danilova et al., 2016), and by cultivation-independent methods involving fluorescence-in-situ-hybridization (FISH; Rahalkar et al., 2009), sequencing of 16S rRNA genes (Fiskal et al., 2021; Pierangeli et al., 2021), and sequencing of catabolic functional genes (for reviews see (McDonald et al., 2008; Knief, 2015). Hereby pmoA, a functional gene that encodes the catalytic subunit of particulate methane monooxygenase (pMMO), an enzyme which performs the initial conversion of methane to methanol, has been widely targeted. The reasons are that pmoA is present in almost all known MOB (exception: Beijerinckiaceae), pmoA-based phylogenies agree largely with 16S rRNA gene-based phylogenies, and pmoA is sufficiently conserved to be suitable for polymerase chain reaction (PCR)-based quantification and sequencing methods.
Even though these methods have greatly advanced knowledge on the distributions of MOB in lake sediments, major knowledge gaps remain. A crucial knowledge gap concerns how increases in sedimentary methane production due to eutrophication and in response to warming impact the activity and community structure of MOB. Moreover, while changes in MOB community structure across oxic-anoxic interfaces are well-studied, little is known about MOB distributions in relation to anaerobic electron acceptors in deeper layers, where MOB often remain abundant. Vertical distributions of these electron acceptors in sediments could shape niches of MOB in ways similar to stratified eutrophic lakes, in which vertical zonations of MOB along redox and methane gradients have been documented (Mayr et al., 2020).
Here we investigate the influence of eutrophication and electron acceptor gradients on the activity, abundance, and community structure of MOB by analyzing sediment cores from five lakes in central Switzerland. These lakes have well-documented trophic histories and today range from oligotrophic (Lake Lucerne) to mesotrophic (Lake Zurich) to eutrophic (Lake Zug, Lake Baldegg, Lake Greifen) (Fiskal et al., 2019). By analyzing sedimentary records that span the entire history of anthropogenic eutrophication starting in the late 19th and early 20th century, we examine if (past) changes in lake trophic state are reflected in MOB community structure at the genus- and operational taxonomic unit (OTU)-level based on pmoA sequences today. Based on pmoA copy numbers as a proxy for MOB abundance and diffusive fluxes of O2 and methane in surficial sediment, we investigate whether methane or O2 availability are key drivers of MOB community size. Using published vertical distributions of respiration reactions (Fiskal et al., 2019), we examine relationships between in situ distributions of respiration reactions and MOB abundances and community structure. Finally, we explore the influence of (past) trophic state and dominant respiration reactions on syntrophic partnerships of MOB and methylotrophic partner organisms based on correlations between MOB genera and OTUs from this study and published 16S rRNA gene sequences of methylotrophic bacteria from Han et al. (2020). Our results provide key insights into how eutrophication history and present-day electron acceptor distributions interact to control the community structure and activity of MOB in lake sediments.
Materials and Methods
Site Descriptions and Lake Trophic Histories
This study is part of the larger research effort “Lake Eutrophication Impacts on Carbon Accumulations in Sediments” (LEICAS), in which the long-term consequences of eutrophication on sediment biogeochemistry, microbiology, and ecosystem ecology are investigated. LEICAS was initiated in 2016, when Lake Lucerne, Lake Zurich, Lake Zug, Lake Baldegg, and Lake Greifen were each sampled at three stations that covered a water depth gradient from shallow sublittoral to profundal sediments. Biogeochemical, organic geochemical, and 16S rRNA gene-based microbiological data on all stations were published previously by Fiskal et al. (2019, 2021a) and Han et al. (2020). An experimental study to investigate impacts of lake “oligotrophication” on sedimentary processes has also been published (Fiskal et al. 2021b). Depth profiles of respiration zones are summarized in Table 1.

TABLE 1. Depth intervals (in cm) of respiration reactions (average ±standard deviation of three stations) across the five lakes (based on Figure 5 in Fiskal et al., 2019).
The five lakes all experience seasonal changes in primary production, thermal stratification, and sedimentation (Teranes et al., 1999; Bürgi, 2000; Naeher et al., 2013), but differ in land use and anthropogenic eutrophication histories (Fiskal et al., 2019). Despite minor increases in total P concentrations in the 1960s and 1970s, Lake Lucerne has always remained oligotrophic. Lake Zurich became eutrophic, as evidenced by the development of permanently hypoxic conditions in its deep basin, around 1890 (Naeher et al., 2013), but has been mesotrophic since ∼1980 due to improved wastewater management. The three eutrophic lakes became eutrophic at different times (Lake Baldegg: ∼1870; Lake Greifen: ∼1920; Lake Zug: ∼1930) and have remained eutrophic since. The high rates of primary production in Lake Greifen, Lake Baldegg, and Lake Zug are sustained by the retention and recycling of P that was introduced in the 20th century. Despite artificial water column mixing and aeration (Lake Baldegg: since 1982/83; Lake Greifen; since 2009), Lake Baldegg experiences strong decreases in O2 concentrations over the summer months, and Lake Greifen continues to develop seasonal hypoxia or anoxia below 10 m water depth from June-December (for details see Supplement of Fiskal et al., 2019, and references therein).
Sample Collection
In June and July 2016, three stations that differed in water depth were sampled in each lake (Lake Lucerne: 15, 24, 32 m; Lake Zurich: 25, 45, 137 m; Lake Zug: 25, 35, 50 m; Lake Baldegg: 21, 45, 66 m; Lake Greifen: 15, 24, 32 m) using 15-cm diameter gravity cores (UWITEC, AT). Bottom water temperatures at the time of sampling were 6–7°C at all stations except the shallow (9°C) and deep stations (5°C) in Lake Lucerne. All sites were bioturbated and contained benthic infaunal chironomids and/or oligochaetes (Fiskal et al., 2021a), except the deep, permanently hypoxic station in Lake Zurich. Sediment porewater for downstream measurements of dissolved electron acceptors (nitrate, sulfate, dissolved inorganic carbon) and respiration end products (Mn2+, Fe2+, ammonium) was sampled using rhizons (0.2 μm pore size, Rhizosphere) from a designated core with pre-drilled holes that were taped prior to coring. Solid-phase sediment samples for cell counts, DNA analyses, methane and total organic carbon (TOC) quantifications, and physical property determinations (porosity, density) were taken from an additional core using sterile cut-off syringes. Porewater cores were sampled in 1-cm depth intervals from 0–4 cm, 2-cm intervals from 4–20 cm, and 4-cm intervals from 36–40 cm. The same sampling resolution was applied during solid-phase sediment sampling, except that the top 0–2 cm were sampled at enhanced resolution (0.5 cm depth intervals). For further details on all sampling procedures, see Fiskal et al. (2019).
Additional cores were taken at each station for the measurement of O2 concentration profiles in surface sediments using Clark-type microsensors (Unisense A/S, DK), macrofaunal community analyses, and sedimentological analyses (including Cs-137 and unsupported Pb-210 for sediment dating; further details and data in Fiskal et al. (2019, 2021a)).
Geochemical Analyses
O2 and methane concentration profiles were published previously by Fiskal et al. (2019). Vertical concentration profiles of O2 were measured using Clark-type microsensors (Unisense A/S) while methane concentrations were measured by gas chromatography using a flame-ionization detector (for details see Fiskal et al. (2019). Vertical distributions of respiration reactions, based on porewater-dissolved concentrations of O2, nitrate, sulfate, Mn2+, Fe2+ and CH4, were also determined in Fiskal et al. (2019).
DNA Extraction
DNA was extracted from all solid-phase samples according to Lever et al. (2015). Sediments from Lake Zug, Lake Zurich and Lake Lucerne were extracted according to lysis protocol II. Due to high humic acid concentrations that interfered with post-extraction column-based DNA purification, sediments from Lake Greifen and Lake Baldegg were treated with an additional humic acid removal step prior to purification (lysis protocol III). For detailed protocols, see Han et al. (2020).
Quantification and Sequencing of pmoA Genes
pmoA was PCR-amplified using the general A189F - Mb661R pmoA primer combination (Holmes et al., 1995; Costello and Lidstrom, 1999) and the new NC10-pmoA239F–NC10-pmoA590R primer pair (Table 2A). The latter primer pair was specifically designed to amplify pmoA sequences of denitrifying methane-oxidizing Methylomirabilales, which are not amplified using the general primer pair but were detected in 16S rRNA gene libraries of the five lakes (Han et al., 2020). The general A189F - Mb661R combination was used both for quantitative PCR (qPCR), using complete pmoA gene sequences of Methylococcus capsulatus as qPCR standards, and for Illumina MiSeq-based community analyses.
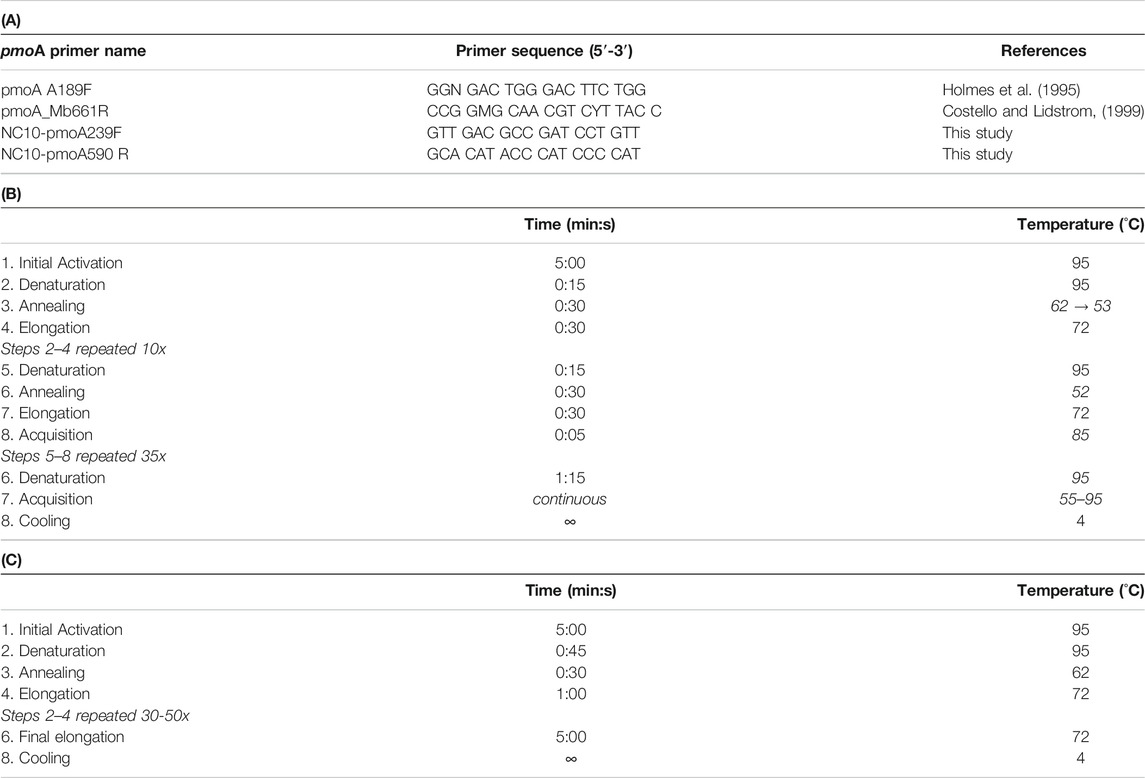
TABLE 2. (A) pmoA primers used in this study. (B) Protocol for qPCR amplification using general pmoA primer pair (A189F–Mb661R). During the initial 10 cycles a touch-down PCR was used, during which the annealing temperature decreased in 1°C intervals. The same protocol was used for the NC10-pmoA29F/NC10-pmoA590R primer combination except that the annealing temperature was maintained at 62°C. (C) PCR amplification protocols for sequencing. The same protocol was used for both primer combinations.
For each of the 15 stations, qPCR analyses and sequencing were performed on 7 to 10 samples (Supplementary Table S1). Key sample targets included bottom water, sediments above and right below the oxic-anoxic transition in the upper few centimeters, samples from anoxic layers with denitrification and sulfate reduction (typically to ≤10 cm), and samples from deeper layers where methanogenesis, iron (III) and manganese (IV) reduction were the only detectable respiration reactions (Table 1). qPCR was done with SYBR Green I Master Mix on a LightCycler 480 II (Roche Molecular Systems). Each 10-μL reaction mixture consisted of 5 µl Roche Light Cycler Master Mix containing SYBR™ Green, 0.5 µl of each 50 µM primer solution, 1 µl of 1 mg ml−1 bovine serum albumin, 2 µl of DNA extract, and 1 µl of molecular-grade water (for qPCR cycler protocol see Table 2B). The new NC10-pmoA239F–NC10-pmoA590R was only used for Illumina MiSeq-based community analyses but not quantification due to non-target PCR amplicons in several qPCR products.
pmoA sequencing libraries were produced according to the work flow of Han et al. (2020), which included an initial booster PCR to obtain similar amplicon concentrations across all samples, and was followed by tailed-primer PCRs (10 cycles) and index PCRs (8 cycles). For the 25-µl PCR reaction mixtures the hot start version of the TaKaRa Ex Taq DNA polymerase was used. Reaction mixtures consisted of 2.5 µl 10x Ex Taq Buffer, 2 µl dNTP solution (2.5 mM each), 0.75 µl of each primer mixture (10 µM concentrations), 2 µl of bovine serum albumin (1 mg ml−1), 0.125 µl of TaKaRa Ex Taq HS, 2 µl of DNA extract, and 14.9 µl of molecular-grade water (for PCR protocols see Table 2C). Paired-end sequencing (600 cycles) was done using a MiSeq Personal Sequencer (Illumina, San Diego, CA).
Amplicon Sequence Data Processing
All DNA sequence data were processed according to the pipeline outlined in Han et al. (2020). Briefly, raw reads were quality-checked by FastQC, followed by read end trimming by seqtk. Trimmed reads were then merged into amplicons by flash (max mismatches density, 0.15) and primer sites removed by usearch (in-silico PCR). Quality filtering was done by prinseq (GC range, 30–70; Min Q mean, 20). OTUs were then clustered according to 97% similarity thresholds (proxy for species-level) to a final number of 927 representative sequences using the USEARCH otutab (), which was also used to remove chimeric sequences. OTU sequences were then used for phylogenetic and bioinformatic analyses.
pmoA Sequences From Lake Metagenomes
To identify potential blind spots or phylogenetic biases of the pmoA primer combinations used, we performed complementary pmoA analyses on lake sedimentary metagenomes. Metagenomes of DNA extracts from five sediment depths of the deepest station in each lake were sequenced at the Joint Genome Institute (JGI; Berkeley, CA, United States; Supplementary Table S1). Paired sequence reads were generated on an Illumina NovaSeq S4 sequencer in 2 × 150 bp mode. Assembly and annotation were performed by the standard automated pipeline of the JGI (see Supplementary Material) and loaded into the Integrated Microbial Genomes and Metagenomes (IMG/M) platform (Chen et al., 2021) for exploration and systematic gene search. Potential pmoA genes from all 25 metagenomes were identified by the AMO Pfam motif (PF02461) detected in the encoded proteins by the IMG annotation pipeline (Chen et al., 2021) resulting in 105 genes in total. The sequences were downloaded and dereplicated (100% nucleotide sequence identity) with Vsearch (v.2.14.1; Rognes et al., 2016) using standard settings, resulting in 81 unique sequences. pmoA genes were distinguished from similar amoA (ammonia monooxygenase subunit A genes) based on their placement in phylogenetic trees (see below).
pmoA Phylogenetic Analyses
Taxonomic assignments were performed using a newly constructed, comprehensive and up-to-date pmoA sequence alignment database in ARB (Ludwig et al., 2004). This database included published pmoA amplicon and metagenome sequences and was initially based on an alignment produced on a subset of sequences using ClustalW in SeaView. This gene data base has since been expanded to include >5,000 pmoA sequences in addition to representatives of pmoA-like RA21 and pxmA (putative copper-containing monooxygenase) and amoA gene clusters. In the process of database expansion, the overall alignment has been updated and optimized. All amplicon sequences obtained with the general pmoA and NC10-specific primer pairs and metagenomic pmoA sequences were added and aligned to the curated pmoA database. De-novo phylogenetic trees, calculated using ARB Neighbour-Joining with Jukes-Cantor Correction (1,000 bootstraps), were then used to taxonomically classify all pmoA OTUs to the genus-level. Tree calculations were based on a ∼470-base pair region that corresponds to the amplified region of the A189F-Mb661R primer pair.
Sequence Annotations and Accession Numbers
All pmoA OTU taxonomic assignments are shown in Supplementary Material S1. Amplicon sequences are publicly accessible at the NCBI Sequence Read Archive (SRA) under Bioproject ID PRJNA785131. Samples used for metagenomes can be found under the ID Gs0142423 on JGI GOLD (https://gold.jgi.doe.gov/, Mukherjee et al., 2021). Metagenomic datasets are publicly available at the JGI genome portal (https://genome.jgi.doe.gov/portal/) under the proposal Id 504756 and at NCBI (PRJNA620348–PRJNA620356, PRJNA620360–PRJNA620364, PRJNA654670–PRJNA654680).
Data Visualizations and Statistical Analyses
Diversity and richness indices were calculated with the functions “diversity” and “specnumber” in the R-package vegan (v. 2.5–7 (Oksanen et al., 2020). Kruskal–Wallis tests, combined with Dunn’s posthoc tests with Bonferroni P correction, were performed to check for significant differences in methane concentrations, pmoA copy numbers, and pmoA/16S rRNA gene ratios in relation to trophic state using R stats version 4.0.3 and the R-package FSA (Ogle et al., 2021). Non-parametric Kruskal–Wallis tests were used instead of One-way Analyses of Variance (ANOVA) due to non-normal data distributions. To test for significant relationships between MOB community composition and sediment depth, used as a proxy for geochemical gradients, and trophic state at the time of sediment deposition, Permutational Multi-variate Analyses Of Variance (PERMANOVA) were performed on genus- and OTU-level community compositions. PERMANOVAs were performed in R with the adonis2 () function in vegan based on Bray-Curtis dissimilarities (9,999 permutations). An Analysis of Similarity (ANOSIM; Bray-Curtis, 9,999 permutations) was used to test for significant differences in MOB communities between individual trophic state categories (eutrophic, mesotrophic, oligotrophic) using the anosim () function in vegan. Non-linear Multi-Dimensional Scaling (NMDS) analyses based on Bray-Curtis dissimilarities (Beals, 1984) were performed using the ‘metaMDS’ function of vegan and used to examine community dissimilarities between samples. Similarity percentage (SIMPER) analyses with the ‘simper’ function (999 permutations) in vegan were used to identify genus-level taxa and OTUs that contribute significantly to community dissimilarities between sample categories (i.e., trophic state, respiration zone). To identify potential syntrophic interactions between methanotrophic and methylotrophic microorganisms, correlations between the abundances of dominant MOB taxa and OTUs and potentially methylotrophic bacteria were determined based on Spearman’s rank correlation coefficients. The latter were calculated according to Lloréns-Rico et al. (2021) with the correlation. R script (https://github.com/raeslab/benchmark_microbiome_transformations/tree/master/scripts/, accessed on 3 July 2021).
All plots were generated with ggplot2 R-package (https://ggplot2.tidyverse.org; Wickham (2016)), basic R plotting functions, LibreOffice Calc, and Microsoft Excel.
Diffusive Fluxes of Methane and O2
Diffusive porewater fluxes of methane and O2 across the sediment-water interface were calculated from vertical concentration profiles using a one-dimensional reaction-transport model (Müller et al., 2003; equation 5) applying published diffusion coefficients (DO2 (5°C): 1.25 × 10–5 cm2 s−1 (Müller et al., 2003); DCH4 (5°C): 9.5 × 10–6 cm2 s−1 (Lerman, 1979)). An empirical formation factor F of 1.2 (Maerki et al., 2004) was used to correct for porosity and tortuosity of the sediment.
Thermodynamic Calculations
Standard Gibbs energies of potential aerobic and denitrifying methanotrophic reactions, and widespread, potentially competing aerobic organotrophic and lithotrophic reactions, were calculated based on ΔGr° values published in Thauer et al. (1977). The range of in situ Gibbs energies (ΔGr’) of these reactions in lake sediments was calculated for a range of environmentally relevant educt and product concentrations according to the equation
where R is the universal gas constant (0.008314 kJ mol−1 K−1), T is standard temperature (298.15K), and Qr is the reaction quotient. For further details, see Table 5.
Results
Methane Concentration Profiles and Potential for Aerobic Methanotrophy
Porewater-dissolved methane concentrations increased with trophic state, with sediment depth, and in several cases with station water depth (Lake Zug, Lake Zurich, Lake Lucerne; Figure 1A). Eutrophic lakes (Lakes Greifen, Baldegg, and Zug) had higher methane concentrations than the oligotrophic Lake Lucerne (p < 0.001, Kruskal–Wallis test followed by a Dunn’s post hoc test). The highly eutrophic Lake Greifen and Lake Baldegg individually also had higher methane concentrations than Lake Lucerne (p < 0.05, same test).
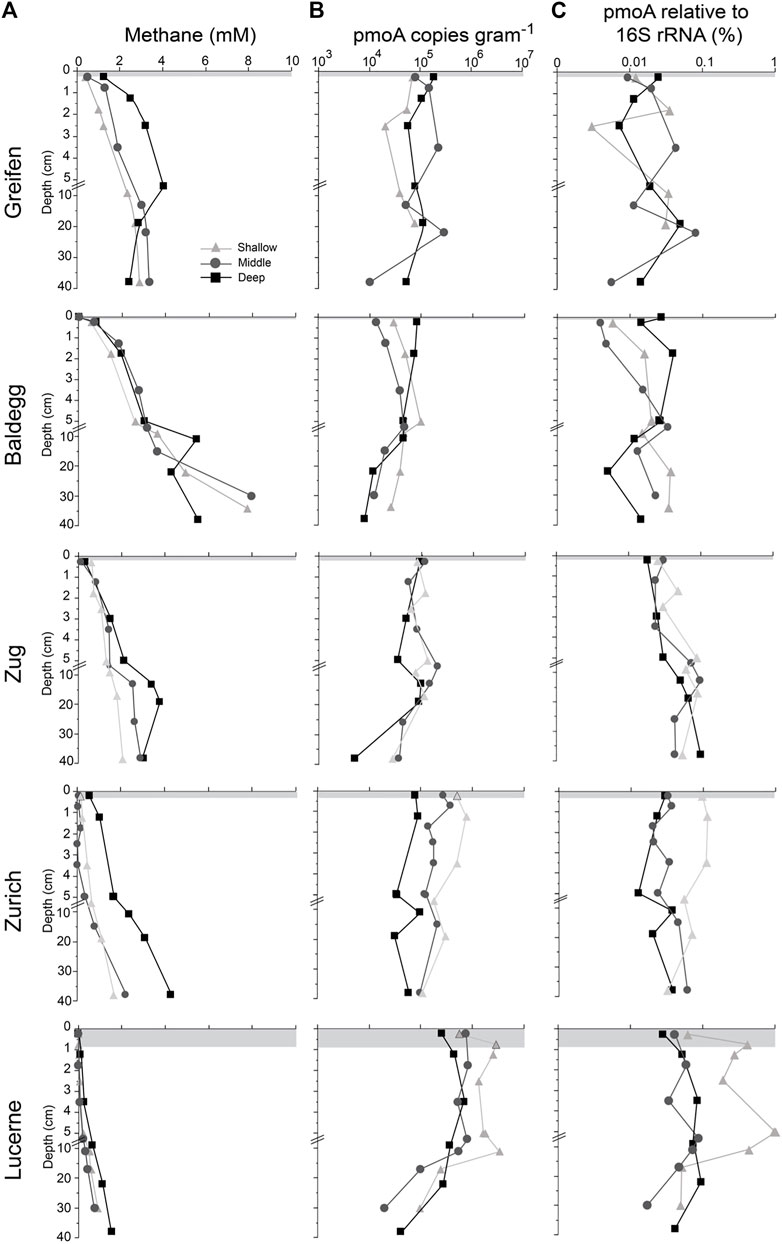
FIGURE 1. Methane, O2 and MOB dynamics in sediments of the three lakes. (A) methane concentrations profiles and depth of O2 penetration (gray area below sediment surface). (B) Absolute abundances of pmoA as determined by qPCR. (C) Relative contribution of MOB to the total microbial community, estimated from ratios of pmoA to total 16S rRNA gene copies. Note the log-scale in (B) and (C).
All stations in the eutrophic lakes and the hypoxic deep station in Lake Zurich displayed steep increases in methane concentrations from the sediment surface downward. This and the concave-down shapes of these concentration profiles indicate that net methane production sets in within the top 1 cm of sediment at these stations. These stations also had elevated methane concentrations all the way to the shallowest sampling interval (0–0.5 cm sediment depth). By contrast, methane concentrations at the middle and shallow stations in mesotrophic Lake Zurich and all stations in oligotrophic Lake Lucerne remained at lower levels (<0.5 mM) in the top cm. These stations only showed strong methane concentration increases, combined with downward concavity indicative of net methane production, below 2–4 cm.
Matching the methane concentration profiles, modeled methane fluxes increase with trophic state (Figure 2A; Table 3). The highest methane fluxes were detected at the deepest stations of all lakes except the year-round artificially aerated Lake Baldegg. By contrast dissolved O2 fluxes into sediments did not change clearly with trophic state (Figure 2B). O2 fluxes were consistently higher in eutrophic Lake Baldegg and Lake Zug compared to oligotrophic Lake Lucerne. The lowest O2 fluxes were measured at the seasonally and permanently hypoxic deep stations of Lake Greifen and Lake Zurich, respectively. Ratios of methane to O2 fluxes reflect the high methane fluxes and low O2 fluxes at these two deep hypoxic stations (Figure 2C), where six times more (Lake Greifen) or equal amounts of methane (Lake Zurich) diffuse to the sediment surface as can be consumed by aerobic methane oxidation under the standard 1:2 stoichiometry of methane to O2. This is not the case for the remaining 13 stations, where – assuming 1:2 stoichiometry – at most 6–30% of O2 consumption can be accounted for by aerobic methane oxidation.
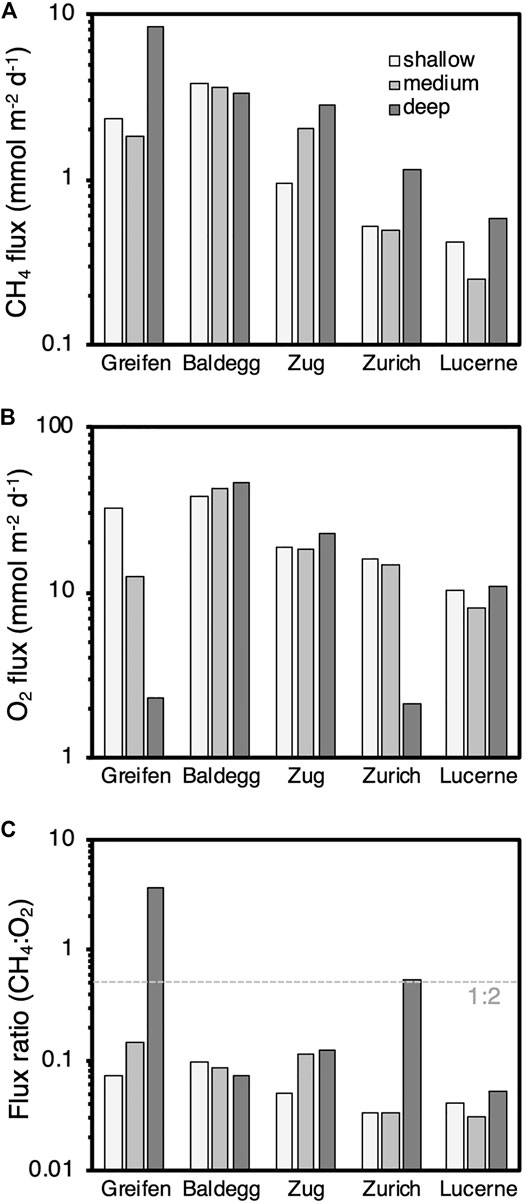
FIGURE 2. Modeled diffusive fluxes of (A) methane and (B) O2, and (C) diffusive flux ratios (CH4:O2) for all lake stations. The dashed line in (C) indicates a 1:2 flux ratio of CH4 to O2. Values below this value indicate a sufficiently high O2 flux for the complete oxidation of all upward diffusing CH4 to bicarbonate (HCO3−). Values above this dashed line (only deep hypoxic stations of Lake Greifen and Lake Zurich) indicate insufficient O2 influx to oxidize all CH4 to bicarbonate.
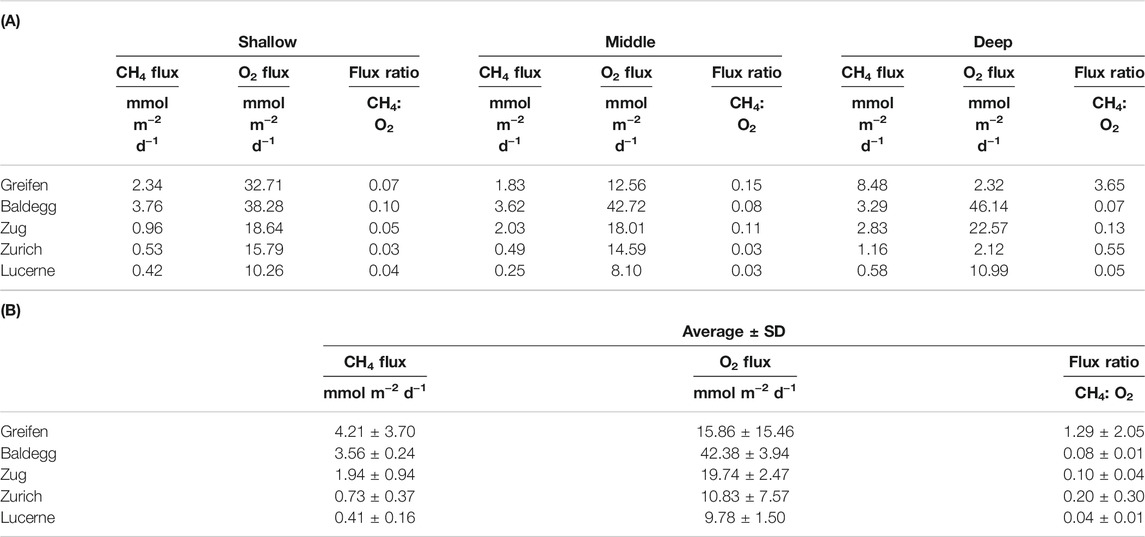
TABLE 3. (A) Modeled CH4 and O2 fluxes and flux ratios by lake and station. (B) Lake-specific average ±standard deviation (SD) of modeled CH4 and O2 fluxes and flux ratios shown in (A). At the standard stoichiometry for aerobic methane oxidation 2 O2 are reduced per CH4 oxidized. Thus, at a flux ratio of 0.03, approximately 6% of O2 that enters sediments is consumed by aerobic methane oxidation, assuming that all methane is oxidized in sediments via the standard stoichiometry of aerobic methane oxidation.
Trends in Absolute and Relative Abundances of MOB
Copy numbers of pmoA, which we used as a proxy for MOB abundance, generally ranged from 104 to 106 g−1 wet sediment and had their highest values in the top 10 cm (Figure 1B). Hereby, pmoA copy numbers varied between and within stations, but did not show consistent trends in relation to redox conditions or sediment depth. Consequently, pmoA copy numbers did not change significantly from oxic sediments to the shallowest samples below the depth of O2 depletion (p > 0.05, here and following paragraphs: Kruskal–Wallis with Dunn’s post hoc test). Furthermore, several sites had bimodal distributions, with surface and subsurface sedimentary pmoA copy number peaks, with the latter generally found in the zone of net methane production (methanogenesis zone).
Despite having the lowest methane fluxes (Figure 2; Table 3), Lake Lucerne had the highest pmoA copy numbers (Figure 1B, p < 0.001). These were up to 10-fold higher compared to the other lakes, both within and below the O2 penetration depth. By contrast, in Lake Baldegg, which had the highest methane fluxes (with Lake Greifen) and highest O2 fluxes (Table 3; Figure 2), and the shallowest O2 penetration (Table 1), pmoA copy numbers were the lowest of all lakes. These trends were statistically supported. The oligotrophic lake (Lake Lucerne) had significantly higher pmoA copy numbers than the combined eutrophic lakes (p < 0.001), but not Lake Zurich. Both Lake Zurich and Lake Lucerne also had higher pmoA copy numbers when individually compared to Lake Baldegg (p < 0.05 and p < 0.001, respectively).
The contribution of MOB to the total microbial community, expressed in % based on the ratio between pmoA and 16S rRNA gene copy numbers, ranged from ∼0.005 to 1% and was also highest in Lake Lucerne (Figure 1C). The highest values were reached at the shallow station in Lake Lucerne, despite the low methane concentrations (<2 mM; Figure 1A) and low methane fluxes (Table 3A) at this station. As for pmoA copies, Lake Lucerne had significantly higher contributions of MOB compared to the eutrophic lakes (p < 0.05), and when compared individually to Lake Baldegg (p < 0.01).
Phylogenetic Diversity of Methanotrophic Bacteria Based on pmoA
Phylogenetic analyses of pmoA sequences obtained by amplicon and metagenome sequencing revealed a phylogenetically diverse MOB assemblage (Figure 3). Groups detected include Type I and Type II MOB, Methylomirabilales, and less known pmoA-like gene clusters (RA21-like, pxma (putative copper-containing monooxygenase)).
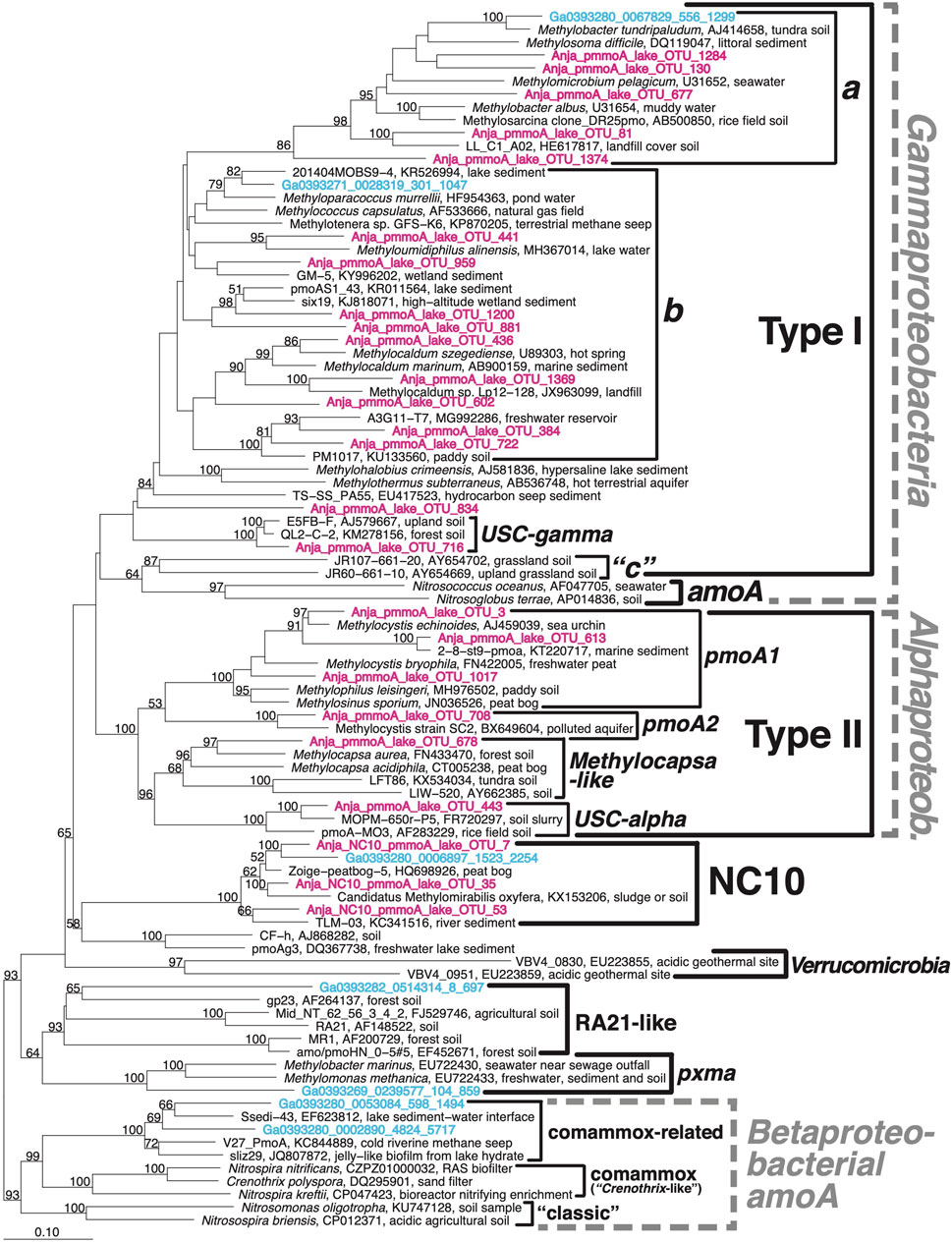
FIGURE 3. Neighbour-joining phylogenetic tree of pmoA amplicon sequences obtained using the general A1809/Mb66a and NC10-specific pmoA29F/590R primer combinations (pink) as well as metagenomic pmoA sequences (blue). The latter are only shown in cases where metagenomic analyses revealed the presence of pmoA (sub)clusters that were not detected by amplicon sequencing. Branching patterns with ≥50% bootstrap support (1,000 replicate calculations) are indicated at branch nodes.
Within the gammaproteobacterial Type I methanotrophs, diverse Type Ia and Type Ib OTUs dominated. All of these OTUs cluster more or less closely with Type Ia and Type Ib genera of the family Methylococcaceae, with the exception of several (represented by OTU_130) that cluster in the phylogenetic vicinity of Candidatus Crenothrix polyspora. Even though the latter is not phylogenetically separated from typical Type Ia Methylococcaceae pmoA (Figure 3) or 16S rRNA gene sequences (Supplementary Figure S3), it is often placed in its own family (Crenotricaceae) due to its distinct morphology. In addition to Type Ia and Type Ib pmoA sequences, Type I OTUs belonging to an unknown group (OTU_834) and the USC-gamma cluster were detected. Furthermore, one subcluster that was closely related to Methylobacter tundripaludicum was only detected by metagenome sequencing. Closest relatives of the detected Type I MOB OTUs were isolated or sequenced from diverse aquatic freshwater and marine water columns and sediments, as well as wetland and terrestrial soils.
Within the alphaproteobacterial Type II MOB, which had a lower OTU diversity than the gammaproteobacterial Type I MOB, pmoA sequences were dominated by the pmoA1 and pmoA2 clusters (collectively also known as Type IIa MOB). pmoA1 and pmoA2 encode different isoenzymes of pMMO and are found in the genera Methylocystis and Methylosinus, some members of which have both genes. In addition, we detected sequences that clustered with the genus Methylocapsa (also known as Type IIb cluster) and the less known USC-alpha cluster. Despite the known discrimination of the A1809/Mb66a primer pair against USC-alpha and Methylocapsa-like pmoA (Knief, 2015), metagenome sequencing did not yield any missing OTUs or phylogenetic clusters. The majority of Type II MOB sequences cluster with isolates and environmental sequences from terrestrial and wetland soils.
The new NC10-specific pmoA29F/590R primer combination detected sequences that cover the known phylogenetic diversity of NC10-pmoA. The fact that NC10 were also detected by metagenome sequencing, including one sequence with 100% sequence identity to Candidatus Methylomirabilis limnetica, indicates that this group occurred in significant numbers in certain samples (discussed more later). Detected NC10-pmoA sequences clustered with ones that were previously recovered from water-logged soils and aquatic sediments.
Two additional, pmoA-like clusters (RA21-like, pxma) were only detected by metagenome sequencing, matching past observations that the general A1809/Mb66a misses both of these divergent, functionally poorly understood pmoA clusters. The recovered sequences diverge strongly from previously published RA21-like or pxma metagenome sequences and are thus of unknown taxonomic origin.
Lake-specific Trends in pmoA OTU Richness, Diversity and Evenness
The methanotrophic community detected with the general A1809/Mb66a pmoA primer pair showed a similar OTU richness across the five lakes (Figure 4A; p > 0.05, Kruskal–Wallis test followed by a Dunn’s post hoc test). The diversity and evenness was, however, significantly lower in oligotrophic Lake Lucerne compared to each of the other lakes (Figures 4B,C; p < 0.001, same tests). This indicates that, despite harboring a comparable total number of OTUs, MOB communities in Lake Lucerne are dominated by few highly abundant OTUs. By contrast, the other four lakes shared a similar, higher evenness and diversity of OTUs. Richness and diversity comparisons were not performed on NC10, as only 19 OTUs belonging to this phylum were recovered, most of which were unique to sediments of Lake Lucerne.
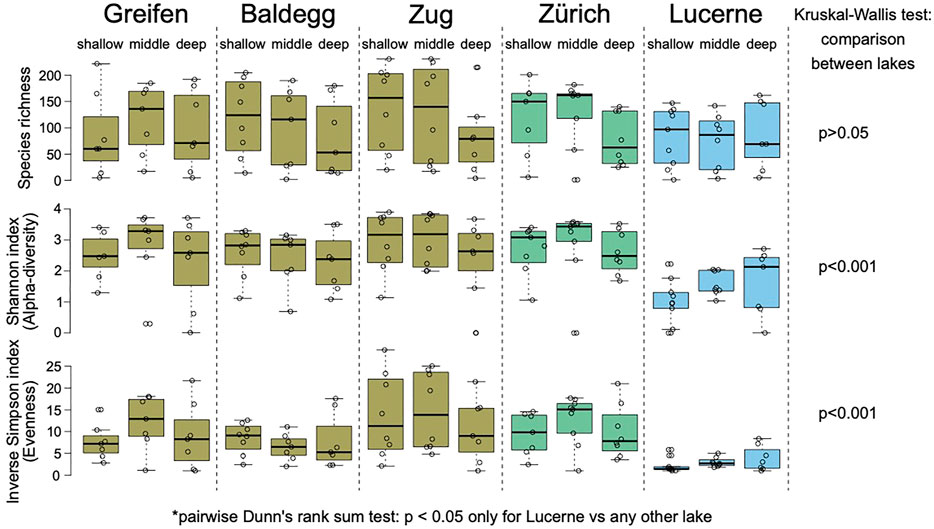
FIGURE 4. OTU Richness (total number of OTUs), Shannon Index (diversity), and Inverse Simpson Index (evenness) of pmoA sequences across the different lakes and sampling stations. The colors represent the current trophic states: eutrophic (brown), mesotrophic (green) and oligotrophic (blue). Far right: results of Kruskal–Wallis test with Dunn’s posthoc test showing that the Shannon and Inverse Simpson Indices differ significantly between Lake Lucerne and the other four lakes, but not between the other four lakes.
Depth Zonation and Trophic Preference of Methanotrophic Communities
pmoA taxonomic compositions based on the general A1809/Mb66a pmoA primer pair showed systematic trends in relation to sediment depth and to a lesser degree trophic state (Figure 5). Clear trends within lakes in relation to water depth or from oxic to anoxic sediment layers within the same sediment cores were absent.
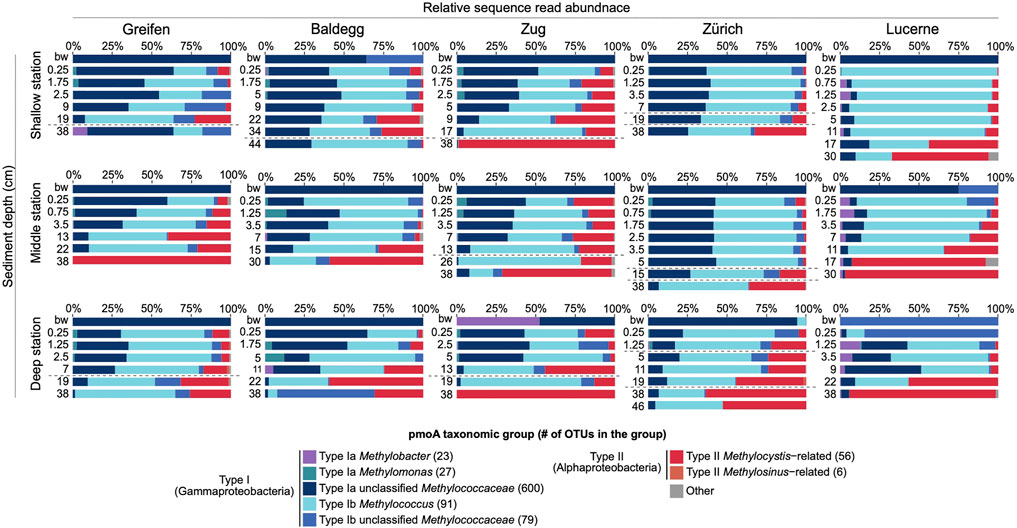
FIGURE 5. Barcharts showing depth distributions of dominant genus-level pmoA taxa/clades across lakes and stations (x-axis: percentage of total pmoA reads per sample; y-axis: sediment depth in cm; bw = bottom water). OTUs that could not be assigned to any known MOB genera were termed “Unclassified” (Type Ia/Type Ib Unclassified Methylococcaceae). Stations are ordered from shallow (top row) to deep (bottom row). Dashed horizontal lines indicate transitions from oligotrophic to eutrophic for Lake Greifen, Lake Baldegg, Lake Zug, and Lake Zurich, at stations where the deepest recovered samples were deposited when these lakes were still oligotrophic. A second dashed horizontal line in shallower layers of Lake Zurich indicates the transition from eutrophic to mesotrophic around 1980. Note: measurable O2 concentrations (>1–2 µM) were only present in the shallowest sediment sample from 0.25 cm, except at the shallow station in Lake Lucerne where O2 was measurable at 0.25 and 0.75 cm sediment depth.
Type I methanotrophic communities in all five lakes were generally dominated by three taxonomic groups, termed Type Ia Unclassified Methylococcaceae, Type Ib Methylococcus, and Type Ib Unclassified Methylococcaceae (Figure 5). Bottom water was dominated by Type Ia Unclassified Methylococcaceae (in most cases >95% of reads). Exceptions are the deep stations in Lake Zug and Lake Lucerne, which are dominated by Type Ia Methylobacter and Type Ib Unclassified Methylococcaceae pmoA sequences, respectively. Communities shift systematically from bottom water into sediments, where pmoA sequences that cluster with the genus Methylococcus (Type Ib), which were nearly absent from bottom water, emerge as a second dominant group.
Percentages of Type Ia Unclassified Methylococcaceae are on average higher in sediments of today’s eu- and mesotrophic lakes compared to oligotrophic Lake Lucerne. Percentages of this group also decrease gradually with sediment depth at most stations, although these decreases do not closely match depth distributions of O2 or anaerobic respiration reactions, or past shifts in trophic state. Similar depth- or trophic state-related changes in Type Ib Methylococcus-type pmoA contributions are less evident, though, notably, this cluster drops in relative abundances in deep sediment layers of oligotrophic Lake Lucerne and most deep oligotrophic layers of today’s eutrophic lakes. The third major Type I cluster (Type Ib Unclassified Methylococcaceae) fluctuates in percentages without clear trends in relation to O2 or respiration zone, sediment depth, past or present trophic state. Other Type I clusters that accounted for significant, but minor percentages of pmoA reads included Type Ia Methylomonas- and Methylobacter-like sequences, Type Ib Methylocaldum-like sequences, and Unclassified Type I-sequences (Figure 5). Read percentages of these groups also did not show clear trends in relation to O2 or respiration zone, sediment depth, past or present trophic state.
The general decreases in Type I pmoA sequence percentages with sediment depth coincided with strong increases in facultative Type II methanotrophs that mainly grouped with the Methylocystis-type pmoA1 cluster (Figure 5). This cluster was almost absent from bottom water but – independent of trophic state - became dominant below the depths of O2, nitrate, and sulfate depletion in iron-reducing and methanogenic sediment layers.
Comparative Analyses of MOB Based on 16S rRNA Gene Sequences
We checked whether sedimentary trends in pmoA sequences were reproducible in the complementary 16S rRNA gene data of Han et al. (2020), and how MOB that were missed by the general A1809/Mb66a pmoA primer pair compared in relative abundances (Supplementary Figure S1). These analyses confirm the shift in dominance from gammaproteobacterial to alphaproteobacterial MOB with increasing sediment depth (and age) observed in pmoA sequence compositions. Moreover, in sediments of Lake Lucerne, members of Candidatus Methylomirabilia (NC10) dominate shallow sediments along with Type Ia Methylococcaceae. Methylomirabilia furthermore account for up to ∼30% of methanotrophic 16S reads in deep sediment layers of the eutrophic lakes, and even dominate sediments below 5 cm depth in Lake Zurich. All Methylomirabilia-related 16S rRNA gene sequences could be assigned to the order Methylomirabilales. Most reads in Lake Lucerne belong to the genus Methylomirabilis, while most reads in the other lakes group with the uncharacterized Sh765B-TzT-35 cluster.
A striking difference to the pmoA analyses was the much lower percentage of Type Ib in the 16S rRNA gene data set. In addition, alphaproteobacterial Type II were dominated by 16S rRNA sequences that cluster with the mostly methylotrophic genus Methyloceanibacter (family: Methyloligellaceae), which only includes one confirmed methanotrophic member that, however, lacks pmoA (M.oceanibacter methanicus; Vekeman et al., 2016). By comparison, percentages of sequences related to facultatively methanotrophic Methylocystis are much lower, though these also increase with sediment depth. Lastly, 16S rRNA gene data indicate the presence of low read fractions (∼0.1%) of Type III methanotrophs (Methylacidiphilaceae; Verrucomicrobia) in surface layers of the middle stations in Lake Baldegg and Lake Lucerne.
Taxonomic and OTU-Level Environmental Clustering of pmoA Sequences
A Non-linear Multi-dimensional scaling (NMDS) ordination of samples based on major taxa recovered with the general A1809/Mb66a pmoA primer pair confirms the main inference from Figure 5 that MOB communities at the genus-level are more strongly structured in relation to sediment depth than trophic state (Figure 6A). This is confirmed based on the results of a PERMANOVA (Table 4). These show that while both sediment depth and trophic state have a significant impact on MOB community structure at the genus-level (both p < 0.001), depth explains a higher portion of the observed community variation (R2 = 0.22) than trophic state (R2 = 0.16). Complementary ANOSIM analyses, moreover, show that trophic state at the time of deposition only shows significant differences between samples that were deposited under oligotrophic vs. eutrophic conditions (R = 0.30, p < 0.001), but not between eutrophic and mesotrophic (R = 0.08, p > 0.05) or mesotrophic and oligotrophic conditions (R = -0.16, p > 0.05).
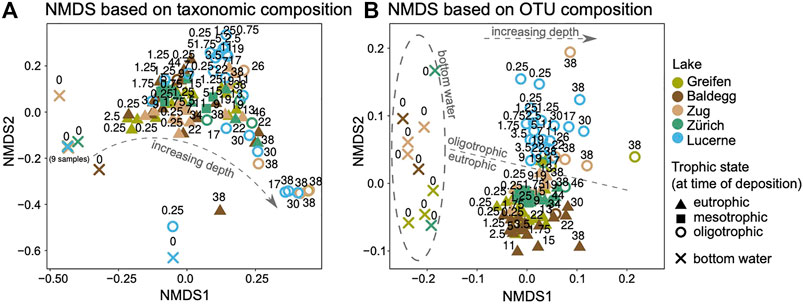
FIGURE 6. Non-linear multi-dimensional scaling (NMDS) ordination of sediment and water samples based on (A) taxonomic groups shown in Figure 5, and (B) OTUs (only general A1809/Mb66a pmoA primer pair). Sediment depths of key samples (in cm) are written into both panels. The low OTU diversity (19 OTUs in total, mainly Lake Lucerne) did not allow for an NMDS analysis of NC10 pmoA sequences.
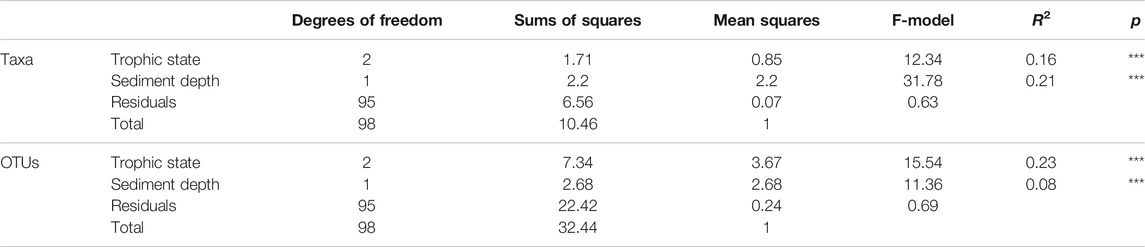
TABLE 4. Permutational Multi-variate Analysis of Variance (PERMANOVA) results for differences in community composition in relation to trophic state at time of deposition and sediment depth (as a proxy for vertical respiration zone gradients). Taxa-level tests were used as a proxy for MOB community trends at the genus-level, while OTU-level tests were used as a proxy for community trends at the species-level. [*** indicates p < 0.001.].
The pattern shifts when NMDS analyses are performed at the OTU-level (“species-level”; Figure 6B). Here trophic state is more strongly correlated with community structure than sediment depth, as indicated by the clear separation of oligotrophic Lake Lucerne from the other four lakes. By contrast, OTU compositions of sediment samples that were deposited under mesotrophic conditions in Lake Zurich are highly similar to samples deposited under eutrophic conditions in Lake Zurich or the other presently eutrophic lakes. Interestingly, OTU samples from the deep oligotrophic layers in Lake Zug and one deep oligotrophic layer in Lake Greifen cluster most closely with oligotrophic communities from Lake Lucerne (Figure 6B), suggesting that trophic state at the time of deposition is the key driver of MOB community structure at the OTU-level. This is confirmed based on a PERMANOVA test (Table 4), which shows that while both sediment depth and trophic state have a significant impact on MOB community structure at the OTU-level (both p < 0.001), trophic state explains a much higher portion of the observed community variation (R2 = 0.23) than sediment depth (R2 = 0.08).
Further OTU-level analyses, reveal that the three main pmoA clusters (Figure 5; Type Ia unclassified Methylococcaceae, Type Ib Methylococcus, Type II Methylocystis) in eu- and mesotrophic lakes are dominated by a larger number of OTUs than in Lake Lucerne (Supplementary Figure S2). Most of these dominant OTUs in the eu- and mesotrophic lakes are shared among these lakes. By contrast, as already indicated by Figure 4, Lake Lucerne is dominated by a smaller number of OTUs with very high relative abundances. These dominant OTUs in Lake Lucerne represent a subset of the OTUs that dominate sediments of the eu- and mesotrophic lakes. Additional similarity percentage (SIMPER) analyses reveal that some OTUs are highly abundant in oligotrophic samples of Lake Lucerne and deep oligotrophic layers of Lakes Greifen, Baldegg, and Zug, but nearly absent from sediment layers that were deposited under eutrophic conditions (e.g. Methylococcaceae OTU2, Methylocystis‐related OTU3; Supplementary Figure S2).
Potential Environmental and Biological Drivers of MOB Communities
Abundance analyses of pmoA copy numbers and pmoA/16S ratios in relation to respiration reactions and trophic state confirm both the absence of a clear pmoA copy number relationship with respiration zone as well as the clear relationship between pmoA copy number and trophic state (Figure 7A). On average, pmoA copy numbers increase by a factor of 8 and pmoA/16S ratios increase by a factor of 4 from sediment samples that were deposited under eutrophic conditions to ones that were deposited under oligotrophic conditions. Both increases are significant (p < 0.001, Kruskal–Wallis followed by Dunn’s post hoc test).
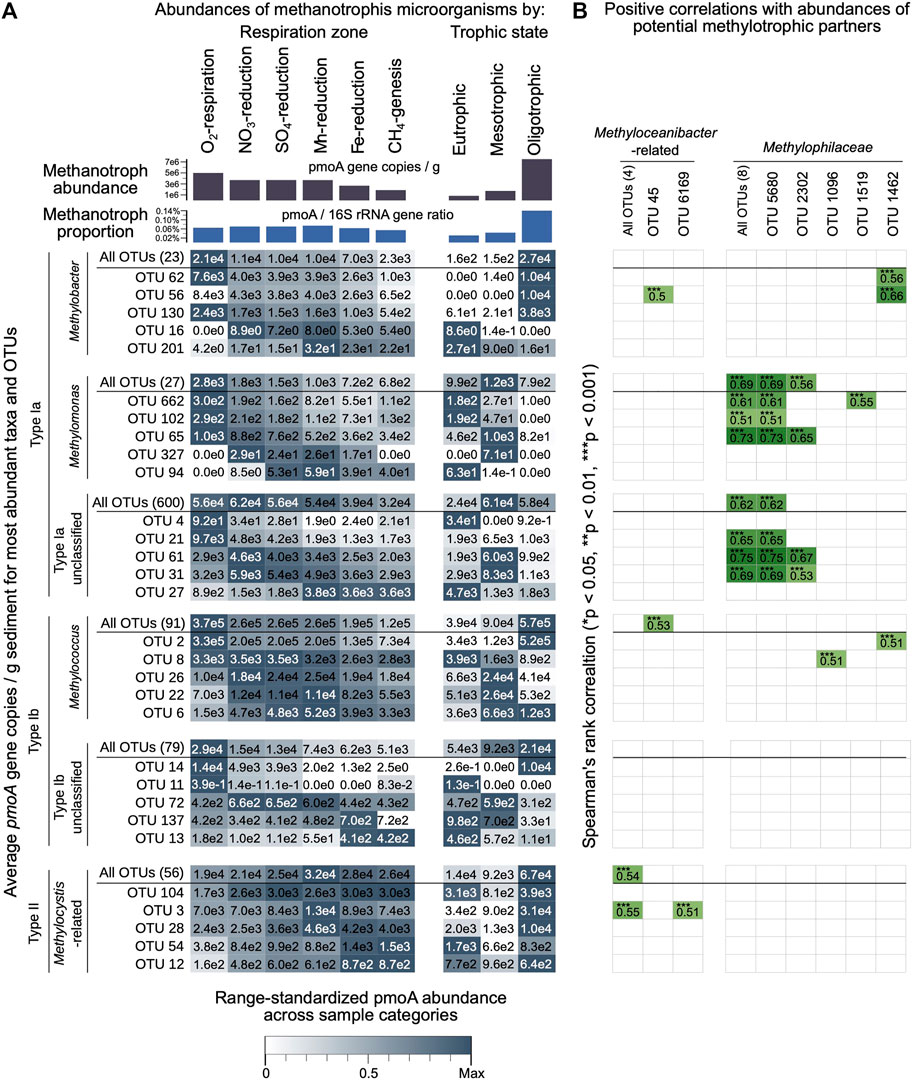
FIGURE 7. (A) Total pmoA copy numbers, relative abundances of pmoA relative to 16S rRNA gene copy numbers (bar charts at top), and calculated gene copy numbers of dominant pmoA taxa and their five most abundant OTUs across different respiration zones and in relation to lake trophic state at the time of sediment deposition (remaining graph). Total pmoA copy numbers and pmoA/16S rRNA gene percentages are averages of values shown in Figures 1B,C, respectively. Gene copy numbers of dominant pmoA taxa and OTUs were calculated by multiplying relative pmoA abundances by total pmoA copy numbers per gram of wet sediment. The heatmap color intensity reflects the standardized average pmoA copy numbers per taxon or OTU, with highest intensity representing the highest average copy number within each comparison (respiration zone, trophic state). (B) Spearman correlation matrix of calculated pmoA copy numbers per taxon or dominant OTU (calculated as in (A)) vs. calculated 16S rRNA gene copy numbers of dominant methylotrophic taxa (Methylophilaceae, Methyloceanibacter-related) and their dominant OTUs as determined by multiplying 16S rRNA gene sequence contributions by qPCR-based total 16S rRNA gene copy numbers (all 16S data from Han et al., 2020). Spearman’s rank correlation coefficients of ≥0.5 are shaded in green and listed in the graph. Asterisks indicate significance level (*p < 0.05; **p < 0.01; ***p < 0.001).
In addition, dominant pmoA taxonomic groups (Figure 5) and their most abundant OTUs show clear patterns in relation to vertical respiration zone and to a lesser degree trophic state (Figure 7A). The observed patterns in relation to respiration zone explain the observed significant correlations of pmoA community structure at the genus- and OTU-level with sediment depth, which per se is not a likely community driver (see previous section). The Type Ia MOB genera Methylobacter and Methylomonas and Type Ib genus Methylococcus, and dominant OTUs within all three genera, have their highest calculated average pmoA abundances in oxic sediments. By contrast, the Type Ia Unclassified Methylococcaceae and Type II Methylocystis, and several dominant OTUs within these groups, have peak pmoA abundances in denitrifying, sulfate- and manganese-reducing (Unclassified Type Ia) and in manganese- and iron-reducing and methanogenic layers (Methylocystis) (Figure 7A). With respect to abundances in relation to trophic state, we generally observe that Type Ia Methylobacter and Type II pmoA sequences, and their dominant OTUs, reach their peak abundances under oligotrophic conditions, whereas Type Ia Methylomonas and Unclassified Methylococcaceae, and their dominant OTUs, reach their highest abundances under meso- and eutrophic conditions. Highly similar patterns to those in Figure 7A were observed when calculated absolute abundances of dominant taxa and OTUs were substituted with relative abundances (Supplementary Figure S4).
Calculated pmoA abundances of major MOB taxa and their dominant OTUs correlate strongly with major methylotrophic taxa and their dominant OTUs (Figure 7B). Type Ia Unclassified Methylococcaceae, Type Ia Methylomonas, and Type Ib Methylococcus, as well as dominant OTUs within these three groups, show strong positive Spearman’s correlations (Rho>0.5, p < 0.001) with 16S rRNA gene-based abundances of Methylophilaceae and dominant OTUs of Methylophilaceae. In addition, matching their observed pmoA peak abundancess in deeper layers, pmoA abundances of Methylocystis and one of its dominant OTUs (OTU_3) are correlated with 16S gene-based abundances of Methyloceanibacter and one of its dominant OTUs (OTU_45). Nonetheless, pmoA OTUs with highest abundances in the oxic or denitrifying sediment layers (Type Ia and Type Ib MOB) were much more likely to correlate with methylotrophic OTUs than pmoA OTUs reaching peak abundances in deeper anoxic layers (Type II). Among pmoA OTUs with peak abundances in the oxic or denitrifying layer, 19 and 22%, respectively, showed a significant positive correlation (Rho>0.5, p < 0.05) with methylotrophic 16S OTUs. This proportion dropped to 10 and 9% for OTUs most abundant in the sulfate and manganese reduction zones, and was 0 and 2%, respectively, in the iron reduction and methanogenesis zones. Moreover, of the 64 Methylocystis OTUs, it was only the aforementioned OTU_3 (Figure 7B) that showed significant correlations with Methyloceanibacter.
We found no clear relationship between trophic state and the percentage of pmoA OTUs that correlate significantly with the percentage of methylotrophic 16S OTUs (Rho>0.5, p < 0.05). Based on calculated absolute abundances, 10 and 12% of pmoA OTUs with peak abundances in sediments deposited under eutrophic and oligotrophic conditions, respectively, were significantly correlated with methylotrophs.
Discussion
Methanotrophic bacteria play a key role in mediating methane emissions from lakes across the world (Bastviken et al., 2008; Thottathil et al., 2018). Although eutrophication enhances organic carbon inputs to lake sediments (Heathcote and Downing, 2012; Anderson et al., 2014) and increases lake sedimentary methane production and emissions (Beaulieu et al., 2019; Fiskal et al., 2019), its effects on the activity, abundance, and community structure of sedimentary MOB is not understood. By performing detailed quantitative and phylogenetic analyses on MOB communities in sediment cores from five lakes that span the past centuries, and by analyzing this community data in the context of trophic history, present-day respiration zone distributions, O2 and methane fluxes, we produce novel insights into the potential controls on MOB communities in lake sediments. We summarize major findings in the following paragraphs and discuss these in further detail in the subsequent thematically structured subsections.
Summary of Major Findings
Matching measured methane concentrations (Figure 1A) and modeled methane production profiles (Fiskal et al., 2019), we observe clear increases in surface sedimentary methane fluxes in eutrophic lakes (Figure 2A; Table 3). Yet, gene copy number-based estimates indicate that MOB are on average 8-fold more abundant and account for 4-fold higher fractions of the total microbial community in samples deposited under oligotrophic compared to eutrophic conditions (Figure 7). An insufficient supply of O2 can be ruled out as a first-order explanation as, except at the hypoxic deep stations in Lake Greifen and Lake Zurich, only a minor fraction (6–30%) of the diffusive flux of O2 can be accounted for by aerobic methane oxidation assuming the standard 2:1 stoichiometry of O2:CH4 (Figure 2C).
Matching previous studies on lake sediments (Rahalkar et al., 2009; Pester et al., 2011; Tsutsumi et al., 2011; He et al., 2021; Lyautey et al., 2021), pmoA copy numbers do not change significantly from oxic to anoxic sediments (Figure 1B). Based on calculated pmoA gene copy numbers of individual MOB genera, certain Type II MOB taxa (mainly Methylocystis) even reach peak abundances in deep methanogenic and metal reducing sediment (Figure 5; Supplementary Figure S1). The observation of high or even elevated pmoA copies below the oxic-anoxic transition is at odds with cultivation data, which indicate that the vast majority of alpha and gammaproteobacterial MOB, including all Methylocystaceae, are obligate aerobes (Bowman, 2014). This raises questions concerning whether these pmoA sequences belong to catabolically active, dormant, inactive, or even dead cells.
While Type II MOB dominate deeper layers, Type I MOB (Methylococcacea) are the major group in oxic and suboxic bottom water and surface sediments (Figure 6), matching other studies on freshwater surface sediments (e.g., Rahalkar et al., 2009; Lyautey et al., 2021). Hereby the (near) absence of Type Ib, but not Type Ia, MOB in overlying water suggests a habitat preference of Type Ib for sediments. Similar to previous studies (Yang et al., 2016; He et al., 2021; Lyautey et al., 2021), Methylococcus-type pmoA dominate Type Ib communities. By contrast, Methylobacter-type Type Ia sequences, which frequently dominate lake sediments elsewhere (Tsutsumi et al., 2011; Reim et al., 2012; Yang et al., 2016), are less abundant. Instead, the majority of Type Ia sequences fall outside of known genera (‘Type Ia Unclassified Methylococcaceae’). Some of these sequences cluster loosely with Candidatus Crenothrix polyspora (Figure 3), raising questions regarding their identity as filamentous Crenotrichaceae or unicellular (mostly coccoid) Methylococcaceae. Phylogenetic trees based on both pmoA and 16S rRNA gene sequences, in which Crenotrichaceae (Crenothrix polyspora) group with well-known Methylococcaceae isolates (Figure 3, Supplementary Figure S3) could not resolve this issue. Besides Type I and Type II MOB taxa, we detect NC10-type denitrifying methanotrophs (Methylomirabilota) based on 16S rRNA gene analyses (Supplementary Figure S1). Members of the type genus Methanomirabilis occur at similar relative abundances to Type I methanotrophs in denitrifying sediments of Lake Lucerne, whereas uncultivated groups of Methylomirabilota (mainly Sh765B−TzT−35) occur at significant abundances in deeper, nitrate-depleted, fully anoxic layers of most lakes.
While the drivers of (uncultivated) Methylomirabilota communities remain uncertain, Type I and Type II MOB taxa at the genus-level show vertical distributions that match distributions of major respiration reactions (Figure 7A and Supplementary Figure S4). This raises the possibility that distributions of MOB genera in lake sediments reflect genus-level differences in the ability to live without O2 and respire different anaerobic electron acceptors. Moreover, OTU-level analyses indicate lake trophic state as a major driver of MOB diversity and community structure at the species-level (Figure 4, 6B & 7A). Potential explanations are that differences in anabolic nutrient regimes or micro-scale habitat heterogeneity impact MOB communities at the species-level. By contrast, based on correlation analyses of MOB taxa and potential methylotrophic partner organisms (Results text), we find no evidence that co-dependencies of MOB on syntrophic partners vary with trophic state. Instead, correlations between MOB and methylotrophic bacterial OTUs follow clear taxonomic and environmental trends, being most frequently observed within Type I taxa that dominate oxic and suboxic surface sediments.
Explanations for the Higher MOB Gene Copy Numbers and Percentages Under Low CH4 fluxes
The order of magnitude higher pmoA copy numbers in oligotrophic Lake Lucerne compared to the eutrophic lakes (Figure 1B & 7A), show parallels with quantitative data on total microbial community size. Total 16S rRNA gene copy numbers were also highest in Lake Lucerne (Han et al., 2020) and were generally supported by microscopic cell counts which indicated higher microbial cell abundances in Lake Lucerne and Lake Zurich than in the three eutrophic lakes (Fiskal et al., 2019). These trends seem paradoxical given that the higher deposition and burial rates of organic carbon in the eutrophic lakes sustain higher total respiration and methanogenesis rates throughout cores from these lakes (Fiskal et al., 2019). A possible explanation is that microbial cell-specific energy requirements are higher in eutrophic lake sediment, e.g. due to enhanced physiological stress from elevated toxic contaminant loads. Yet, though more research is needed, previous studies suggest minor effects of anthropogenic toxin contamination on sediment microbial communities (Chen et al., 2014; Di Cesare et al., 2020). Notably also, the shallow and medium station in Lake Zurich are located in a Zinn (Sn)-polluted area (Lennartz, 2010), and yet had high cell counts and high qPCR values of pmoA and total 16S genes.
An alternative explanation is that – matching the higher microbial activity - sediments of eutrophic lakes have higher microbial growth rates, but that these coincide with higher rates of mortality, e.g. due to viral lysis or predation. Though we lack data on viral lysis rates, previous work has shown high abundances of oligochaetes (Tubificidae) to 20 cm sediment depth in the three eutrophic lakes and absence of these worms from all but the sediment surface in Lake Lucerne (Fiskal et al., 2021a). Gut microbiome analyses suggest protein-rich diets that are consistent with microbial grazing as a major food source of tubificids (Fiskal et al., 2021a; de Valk et al., 2017). While lake sedimentary invertebrates are known to stimulate growth of MOB in surface sediments (“microbial gardening”), leading to MOB becoming a major food source of these invertebrates in some lakes (Kiyashko et al., 2001; Deines et al., 2007; Jones and Grey, 2011), none of the stations in this study show evidence for MOB gardening (Fiskal et al., 2021a). We thus propose that non-selective grazing by tubificids causes higher mortality and ultimately results in lower abundances of microorganisms, including MOB, in sediments of the eutrophic lakes.
While non-selective grazing by oligochaetes explains lower absolute abundances of MOB, it does not explain the lower relative abundances (percentages) of MOB in the eutrophic lakes (Figure 1C, 7A, and Supplementary Figure S2). These lower percentages are particularly striking given the steep, overlapping O2 and CH4 concentration gradients in the top 0.5 cm of eutrophic lakes (Figure 1A), which imply high potential for aerobic methanotrophy. In the following paragraphs, we evaluate three potential hypotheses to explain the lower percentages of MOB in eutrophic lake sediments with high methane fluxes.
Direct observations on sediment cores from the eutrophic lakes revealed the presence of gas bubbles starting just below the 1 to 2 cm thick, flocculent surface layer. While gas bubbles also appeared in sediment of Lake Lucerne and the shallow and medium stations in Lake Zurich, these bubbles were restricted to deeper, more compacted sediment layers and less numerous in these locations. Based on these observations, it is possible that most methane in sediments of eutrophic lakes is present in gas bubbles rather than bioavailable dissolved form. These methane bubbles may bypass the “biological methane filter” in oxic surface sediments by rapid transit through the flocculent surface layer followed by ebullition into overlying water, explaining why methane oxidation only supports a small percentage of the microbial community in eutrophic lake sediments. The importance of ebullitive release of methane from lake sediments to overlying water and the atmosphere is well-known and especially high for shallow and/or eutrophic lakes (Thebrath et al., 1993; Schubert et al., 2012), though little is known about how ebullitive losses quantitatively compare to biological consumption by MOB in lake sediments.
Given the high sedimentation rates of labile microalgal biomass (Han et al., 2020) and shallow depletion of O2 and nitrate in the three eutrophic lakes (Table 1), competition for O2 or nitrate between MOB and other microorganisms could also explain the lower MOB percentages in eutrophic lakes. Thermodynamic calculations (Table 5) indicate that aerobic organotrophic microorganisms have substantially higher energy yields than aerobic or denitrifying MOB. Organotrophs could thus outcompete MOB for O2 and nitrate, and thereby suppress methane consumption rates and the efficiency of the biological methane filter in the eutrophic lakes. By comparison, sediments of Lake Lucerne have deeper O2 and nitrate penetration (Table 1) and receive less labile algal organic matter than the eutrophic lakes (Han et al., 2020). Here competition for O2 or nitrate could be less intense due to stronger electron donor limitation of organotrophs, and lead to MOB being major O2 consumers. In Lake Lucerne, MOB may even have energetic advantages over competing lithotrophic nitrifying bacteria (Table 5), which are major microbial community members in this lake (Han et al., 2020). Reduced competition for O2 and nitrate could also explain why MOB effectively deplete upward-diffusing methane in the surface centimeters of Lake Lucerne and the oxic shallow and medium stations of Lake Zurich.
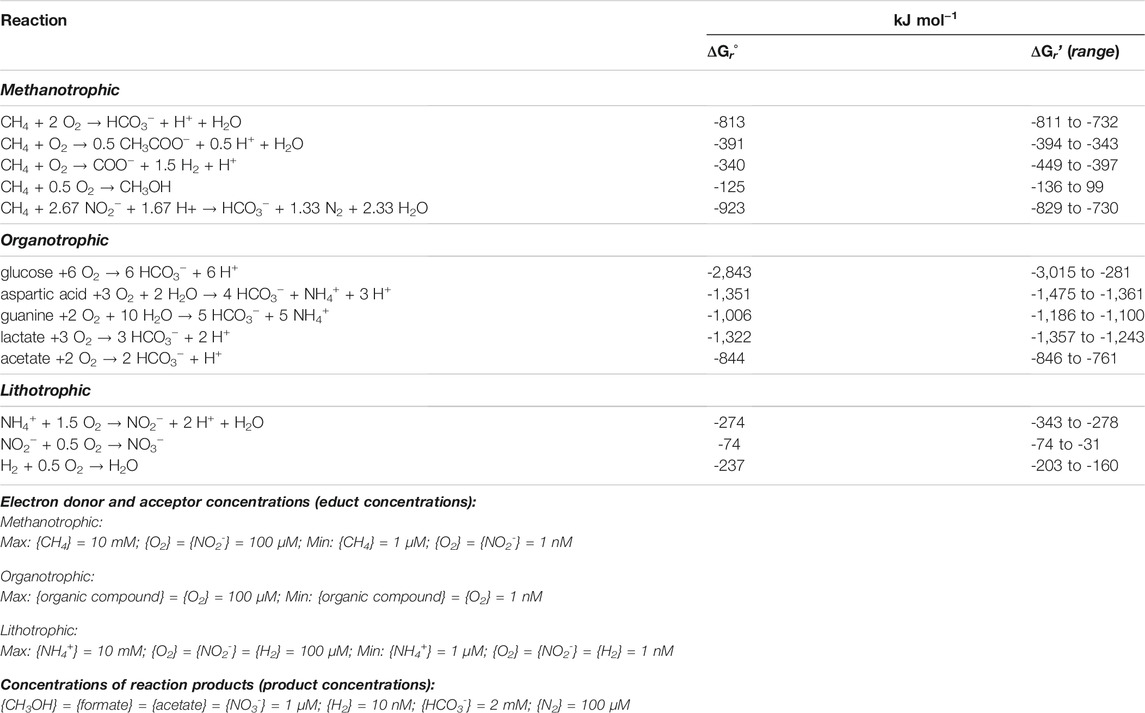
TABLE 5. Gibbs energies of potential aerobic and denitrifying methanotrophic reactions, organotrophic reactions, and lithotrophic reactions. We include Gibbs energies under standard conditions (ΔGr°), and for a range of environmentally relevant educt and product concentrations (ΔGr° (range), see footnote below table) assuming standard temperature, pressure, and pH (Note: the impact of in situ temperature, pressure, and pH on Gibbs energy yields is negligible within the range of calculated educt and product concentrations).
The third hypothesis concerns potential changes in dominant methanotrophic reactions in relation to trophic state. Besides performing the complete oxidation of methane to CO2, MOB are known to under certain conditions only partially oxidize methane to low molecular weight intermediates, such as methanol, H2, formate, or acetate, which are then utilized by denitrifying partner organisms (Kalyuzhnaya et al., 2013; Beck et al., 2013; Oshkin et al., 2014; Hernandez et al., 2015). The factors that determine whether MOB perform complete or partial oxidation are not understood. O2 concentrations may affect the MOB genera involved, but not necessarily the occurrence of syntrophic reactions (Beck et al., 2013; Hernandez et al., 2015; Oshkin et al., 2014). Compared to the complete oxidation of methane to CO2, the Gibbs energies of partial oxidation reactions are ∼6-fold (methanol) or 3- to 4-fold (formate, acetate) lower (Table 5). Higher contributions of energetically less favorable partial methane oxidation reactions in the eutrophic lakes could thus contribute to the lower MOB percentages in the latter. Yet, while we observe strong correlations between calculated pmoA copy numbers of certain Type I genus-level taxa and OTUs with calculated 16S gene copy numbers of Methylophilaceae (Figure 7B), the percentage of taxa or OTUs with such correlations does not change in relation to trophic state.
In conclusion, based on available data, enhanced oligochaete grazing is a plausible reason for the lower absolute abundances of MOB in eutrophic lakes, whereas the lower percentages of MOB in eutrophic lakes can be explained with enhanced methane ebullition and/or outcompetition of MOB for O2 by aerobic organotrophs. By contrast, based on our correlation analyses, enhanced energy-sharing with syntrophic partner organisms is less likely to drive the lower percentages of MOB in eutrophic lakes.
Role of Respiration Zone in Structuring Major MOB Taxa
Analyses of pmoA genus-level taxa distributions indicate strong overlaps in community structure between different trophic states, and thus argue against the existence of distinct oligotrophic or eutrophic MOB taxa (Figure 5 & 6A). Instead, the highly significant correlation of sediment depth with taxon-level MOB community structure, combined with the clear taxon-specific differences in distributions relative to vertical respiration zones, point to respiration zone as a key driver of MOB community composition at the genus-level (Figure 5, 6A, and 7A).
Interestingly, the Type Ia genera Methylobacter and Methylomonas and Type Ib genus Methylococcus, and dominant OTUs within these genera, had their highest calculated average pmoA abundances in oxic sediments (Figure 7), even though at least members of Methylobacter and Methylomonas have been linked to facultative anaerobic metabolism with or without partner organisms (Methylomonas: NO2−, NO3−, ferrihydrite (Kits et al., 2015b, 2015a; Zheng et al., 2020)); Methylobacter: NO3− (Smith et al., 2018; van Grinsven et al., 2020a)). Other MOB, including the Unclassified Type Ia Methylococcaceae have peak pmoA abundances in suboxic layers (Figure 7), suggesting that members of this paraphyletic group might couple methane oxidation to nitrate reduction. Syntrophic interactions between unclassified Type Ia Methylococcaceae and Methylomonas and methylotrophic Methylophilaceae with denitrifying potential were previously proposed (Beck et al., 2013; Cao et al., 2019; van Grinsven et al., 2020b) and would match found correlations between pmoA abundances of both groups and 16S rRNA gene-based abundances of Methylophilaceae (Figure 7B).
While the observed distributions of Type I taxa are generally consistent with previous studies, the observed trends in Type II taxa are more difficult to reconcile. Type II alphaproteobacterial Methylocystaceae sequences dominated deep anoxic sediments of all lakes (Figure 6). It remains unclear whether these deep Methylocystis-related pmoA sequences derive from metabolically active cells, resting (dormant) or inactive cells, or dead organisms. For this DNA to be from dead cells, DNA of Methylocystis would need to be more effectively preserved than DNA of Type I methanotrophs, e.g. via shielding by more degradation-resistant cell walls. Members of Methylocystis produce lipoidal cysts as resting stages, which are compositionally distinct from the carbohydrate-rich (“Azotobacter-type”) cell walls of cysts produced by most Methylococcaceae (Bowman 2014). These lipoidal cysts may be more degradation-resistant in lake sediments than Azotobacter-type cysts. Yet, enhanced stability of resting stages, which requires substantiation, does not explain why dominant Methylocystis-related OTUs reach their highest gene copy numbers in anoxic layers where respiration is dominated by metal reduction and methanogenesis (Figure 7A), or why previous studies have reported increases in relative abundances and active assimilation of methane-C by Type II methanotrophs in anoxic sediment (Yang et al., 2019; He et al., 2012). A potential explanation is that Type II Methylocystis-related MOB oxidize methane via strictly anaerobic associations with partner organisms. Yet, only one Methylocystis OTU (out of 64) showed a significant correlation with a methylotrophic bacterial OTU (genus Methyloceanibacteria; Figure 7B and related text). Thus, deep Type II MOB might depend less on syntrophic interactions than their Type I counterparts in shallower layers, or not at all. Future research will reveal whether members of Methylocystis are involved in methane oxidation, e.g. coupled to metal reduction, as was previously shown for members of Methylosinus, a sister genus of Methylocystis (Zheng et al., 2020), or even subside on substrates other than methane, such as acetate or ethanol as was previously shown for (however aerobic) members of this genus (Haque et al., 2020; Oshkin et al., 2020).
Lake Trophic State as a Driver of MOB Communities at the Species-Level
While pmoA-based community structure at the genus-level was similar across different trophic states, analyses at the OTU-level indicate trophic state as a major driver of MOB community structure at the species-level (Figure 6B). Sediments of the oligotrophic Lake Lucerne have a significantly lower OTU diversity and evenness than sediments of the meso- and eutrophic lakes (Figure 4) and are dominated by a small number of OTUs that are also present though not dominant in eu- and mesotrophic lakes (Supplementary Figure S2). Interestingly, as previously demonstrated for 16S rRNA gene-based analyses of bacterial communities (Han et al., 2020), the trophic state at the time of sediment deposition appears to better predict the present-day MOB community structure than the present-day trophic state of the lake in numerous cases. This is indicated by the fact that OTU community fingerprints of several deep samples from Lake Zug and Lake Greifen, that were deposited under oligotrophic conditions, cluster with samples from the always oligotrophic Lake Lucerne (Figure 6B). This legacy effect was most evident in a Type Ib Methylococcaceae-related (OTU_2) and a Type II Methylocystis-related OTU (OTU_3). These OTUs dominated sediment samples from Lake Lucerne and had high relative abundances in deep oligotrophic samples from Lake Zug and Lake Greifen, while being nearly absent from all other samples (Supplementary Figure S2).
Our results indicate that MOB community structure is controlled by different environmental variables on different phylogenetic levels. The strong community structuring of MOB genus-level taxa in relation to respiration zones could reflect differences in electron acceptor use or methylotrophic partners among these taxa. By contrast, the clear structuring of OTU-based communities relative to trophic state suggests that additional factors control MOB communities at the species or strain-level, such as differences in enzyme and transporter affinities in relation to nutrient regimes or competition with other microorganisms for O2. The low community alpha-diversity and evenness in oligotrophic Lake Lucerne, where organic matter is more degraded and contains lower fractions of algal organic carbon, could reflect lower geochemical niche diversity, reduced availability of biosynthetic nutrients and reduced competition for O2. In comparison, higher availability of nutrients needed for biomass production and higher diversity of microniches with distinct redox conditions could support higher numbers of co-existing OTUs and higher evenness in eutrophic lake sediments.
Conclusion
Although eutrophication is expected to increase lacustrine methane emissions worldwide, its effects on the methanotrophic and wider carbon-cycling microbial community are not well understood. Across five lakes in central Switzerland that range in present-day trophic state from highly eutrophic to oligotrophic, and differ significantly in trophic histories, we observe the highest pmoA copy numbers and contributions of MOB to total microbial communities under oligotrophic conditions. This observation is remarkable given the higher methane fluxes in the eutrophic lakes and considering that O2 fluxes into sediments vastly exceed the amount required for complete mineralization of methane at most stations. Potential explanations include that most methane produced in eutrophic sediments “bypasses” MOB communities by ebullition into overlying water and/or that MOB are outcompeted for O2 by organotrophic microorganisms with higher in situ energy yields in eutrophic lakes. In addition, microbial community size based on gene copy numbers may not represent a reliable proxy for MOB activity, suggesting that follow-up studies in which methanotrophic activity is measured are needed.
The consistent depth zonation of “aerobic” MOB in suboxic and anoxic sediments suggests that a much higher proportion of MOB taxa is capable of anaerobic metabolism than previously assumed. The observed OTU-level community structuring according to trophic state at the time of sediment deposition, moreover, indicates a legacy effect of eutrophication on methanotrophic communities. Correlation analyses of MOB and potential methylotrophic partner organisms suggest that syntrophic interactions between MOB and methylotrophs are most important in oxic and denitrifying sediment, and less important in deeper layers where O2 and nitrate are presumed to be absent. Future research will reveal whether Type II MOB that increase in deep, Fe-reducing and methanogenic layers engage in an unknown form of methanotrophy, and, if so, how methane oxidation is thermodynamically possible in the same sediment layers in which methane is also produced.
Data Availability Statement
The datasets presented in this study can be found in online repositories. The names of the repository/repositories and accession number(s) can be found in the article/Supplementary Material.
Author Contributions
ML designed the study. AM and XH performed the laboratory analyses. DM, SvG, ML, and CS analyzed the data. ML, SvG, and DM wrote the manuscript with input from all co-authors.
Funding
This study was funded by the Swiss National Science Foundation (project 205321_163371 “Role of bioturbation in controlling microbial community composition and biogeochemical cycles in marine and lacustrine sediments” awarded to ML).
Conflict of Interest
The authors declare that the research was conducted in the absence of any commercial or financial relationships that could be construed as a potential conflict of interest.
Publisher’s Note
All claims expressed in this article are solely those of the authors and do not necessarily represent those of their affiliated organizations, or those of the publisher, the editors and the reviewers. Any product that may be evaluated in this article, or claim that may be made by its manufacturer, is not guaranteed or endorsed by the publisher.
Acknowledgments
We thank Beat Müller (Eawag) for assistance with diffusive flux calculations, Nathalie Dubois, Alois Zwyssig, Alfred Lück, and Irene Brunner for sampling assistance (all Eawag), Annika Fiskal, Philip Eickenbusch, and Lorenzo Lagostina (all ETH Zurich) for technical support, and Silvia Kobel, Aria Minder, and Jean-Claude Walser from the Genetic Diversity Centre (GDC; ETH Zurich) for assistance with next-generation sequencing and bioinformatic analyses.
Supplementary Material
The Supplementary Material for this article can be found online at: https://www.frontiersin.org/articles/10.3389/fenvs.2022.857358/full#supplementary-material
References
Anderson, N. J., Bennion, H., and Lotter, A. F. (2014). Lake Eutrophication and its Implications for Organic Carbon Sequestration in Europe. Glob. Change Biol. 20, 2741–2751. doi:10.1111/gcb.12584
Auman, A. J., Stolyar, S., Costello, A. M., and Lidstrom, M. E. (2000). Molecular Characterization of Methanotrophic Isolates from Freshwater lake Sediment. Appl. Environ. Microbiol. 66, 5259–5266. doi:10.1128/aem.66.12.5259-5266.2000
Bastviken, D., Cole, J. J., Pace, M. L., and van de Bogert, M. C. (2008). Fates of Methane from Different lake Habitats: Connecting Whole-lake Budgets and CH4emissions. J. Geophys. Res. 113, a–n. doi:10.1029/2007JG000608
Bastviken, D., Cole, J., Pace, M., and Tranvik, L. (2004). Methane Emissions from Lakes: Dependence of lake Characteristics, Two Regional Assessments, and a Global Estimate. Glob. Biogeochem. Cycles 18, a–n. doi:10.1029/2004GB002238
Bastviken, D., Tranvik, L. J., Downing, J. A., Crill, P. M., and Enrich-Prast, A. (2011). Freshwater Methane Emissions Offset the Continental Carbon Sink. Science 331, 50. doi:10.1126/science.1196808
Beals, E. W. (1984). Bray-curtis Ordination: An Effective Strategy for Analysis of Multivariate Ecological Data. Adv. Ecol. Res. 14, 1–55. doi:10.1016/S0065-2504(08)60168-3
Beaulieu, J. J., DelSontro, T., and Downing, J. A. (2019). Eutrophication Will Increase Methane Emissions from Lakes and Impoundments during the 21st century. Nat. Commun. 10, 3–7. doi:10.1038/s41467-019-09100-5
Beck, D. A. C., Kalyuzhnaya, M. G., Malfatti, S., Tringe, S. G., Glavina del Rio, T., Ivanova, N., et al. (2013). A Metagenomic Insight into Freshwater Methane-Utilizing Communities and Evidence for Cooperation between theMethylococcaceaeand theMethylophilaceae. PeerJ 1, e23. doi:10.7717/peerj.23
Bowman, J. P. (2014). The Family Methylococcaceae. The Prokaryotes: Gammaproteobacteria 9783642389, 411–440. doi:10.1007/978-3-642-38922-1_237
Buergi, H. R., and Stadelmann, R. (2000). für, R. S.-I. V., and 2000, undefinedChange of phytoplankton diversity during long-term restoration of Lake Baldegg (Switzerland). SIL Proc. 1922-2010 27, 574–581. doi:10.1080/03680770.1998.11901300
Cao, Q., Liu, X., Ran, Y., Li, Z., and Li, D. (2019). Methane Oxidation Coupled to Denitrification under Microaerobic and Hypoxic Conditions in Leach Bed Bioreactors. Sci. Total Environ. 649, 1–11. doi:10.1016/j.scitotenv.2018.08.289
Chen, I.-M. A., Chu, K., Palaniappan, K., Ratner, A., Huang, J., Huntemann, M., et al. (2021). The IMG/M Data Management and Analysis System v.6.0: New Tools and Advanced Capabilities. Nucleic Acids Res. 49, D751–D763. doi:10.1093/NAR/GKAA939
Chen, J., He, F., Zhang, X., Sun, X., Zheng, J., and Zheng, J. (2014). Heavy Metal Pollution Decreases Microbial Abundance, Diversity and Activity within Particle-Size Fractions of a Paddy Soil. FEMS Microbiol. Ecol. 87, 164–181. doi:10.1111/1574-6941.12212
Costello, A. M., and Lidstrom, M. E. (1999). Molecular Characterization of Functional and Phylogenetic Genes from Natural Populations of Methanotrophs in Lake Sediments. Appl. Environ. Microbiol. 65, 5066–5074. doi:10.1128/aem.65.11.5066-5074.1999
D’Ambrosio, S., and Harrison, J. A. (2021). Methanogenesis Exceeds CH4 Consumption in Eutrophic lake Sediments. Limnol. Oceanogr. Lett. 6, 173–181. doi:10.1002/lol2.10192
Danilova, O. V., Suzina, N. E., Van De Kamp, J., Svenning, M. M., Bodrossy, L., and Dedysh, S. N. (2016). A New Cell Morphotype Among Methane Oxidizers: A Spiral-Shaped Obligately Microaerophilic Methanotroph from Northern Low-Oxygen Environments. ISME J. 10, 2734–2743. doi:10.1038/ismej.2016.48
Dean, J. F., Middelburg, J. J., Röckmann, T., Aerts, R., Blauw, L. G., Egger, M., et al. (2018). Methane Feedbacks to the Global Climate System in a Warmer World. Rev. Geophys. 56, 207–250. doi:10.1002/2017RG000559
Deines, P., Bodelier, P. L., and Eller, G. (2007). Methane-derived Carbon Flows through Methane-Oxidizing Bacteria to Higher Trophic Levels in Aquatic Systems. Environ. Microbiol. 9, 1126–1134. doi:10.1111/j.1462-2920.2006.01235.x
Deutzmann, J. S., Stief, P., Brandes, J., and Schink, B. (2014). Anaerobic Methane Oxidation Coupled to Denitrification Is the Dominant Methane Sink in a Deep lake. Proc. Natl. Acad. Sci. USA 111, 18273–18278. doi:10.1073/pnas.1411617111
de Valk, S., Khadem, A. F., Foreman, C. M., van Lier, J. B., and de Kreuk, M. K. (2017). Physical and Biochemical Changes in Sludge Upon Tubifex Tubifex Predation. Environ. Technol. 38, 1524–1538. doi:10.1080/09593330.2016.1236150
Di Cesare, A., Pjevac, P., Eckert, E., Curkov, N., Miko Šparica, M., Corno, G., et al. (2020). The Role of Metal Contamination in Shaping Microbial Communities in Heavily Polluted marine Sediments. Environ. Pollut. 265, 114823. doi:10.1016/j.envpol.2020.114823
Ettwig, K. F., Butler, M. K., Le Paslier, D., Pelletier, E., Mangenot, S., Kuypers, M. M. M., et al. (2010). Nitrite-driven Anaerobic Methane Oxidation by Oxygenic Bacteria. Nature 464, 543–548. doi:10.1038/nature08883
Ettwig, K. F., Zhu, B., Speth, D., Keltjens, J. T., Jetten, M. S. M., and Kartal, B. (2016). Archaea Catalyze Iron-dependent Anaerobic Oxidation of Methane. Proc. Natl. Acad. Sci. USA 113, 12792–12796. doi:10.1073/pnas.1609534113
Farhan Ul Haque, M., Xu, H.-J., Murrell, J. C., and Crombie, A. (2020). Facultative Methanotrophs - Diversity, Genetics, Molecular Ecology and Biotechnological Potential: a Mini-Review. Microbiol. (United Kingdom) 166, 894–908. doi:10.1099/mic.0.000977
Fiskal, A., Anthamatten, E., Deng, L., Han, X., Lagostina, L., Michel, A., et al. (2021a). Carbon Sources of Benthic Fauna in Temperate Lakes across Multiple Trophic States. Biogeosciences 18, 4369–4388. doi:10.5194/bg-18-4369-2021
Fiskal, A., Gaillard, A., Giroud, S., Malcic, D., Joshi, P., Sander, M., et al. (2021b). Effects of Macrofaunal Recolonization on Biogeochemical Processes and Microbiota a Mesocosm Study. Water 13, 1599. doi:10.3390/w13111599
Fiskal, A., Deng, L., Michel, A., Eickenbusch, P., Han, X., Lagostina, L., et al. (2019). Effects of Eutrophication on Sedimentary Organic Carbon Cycling in Five Temperate Lakes. Biogeosciences 16, 3725–3746. doi:10.5194/bg-16-3725-2019
Frenzel, P., Thebrath, B., and Conrad, R. (1990). Oxidation of Methane in the Oxic Surface Layer of a Deep lake Sediment (Lake Constance). FEMS Microbiol. Lett. 73, 149–158. doi:10.1016/0378-1097(90)90661-910.1111/j.1574-6968.1990.tb03935.x
Grinsven, S., Sinninghe Damsté, J. S., Engelmann, J. C., Harrison, J., Villanueva, L., and Villanueva, L. (2020a). Methane Oxidation in Anoxic lake Water Stimulated by Nitrate and Sulfate Addition. Environ. Microbiol. 22, 766–782. doi:10.1111/1462-2920.14886
Grinsven, S., Sinninghe Damsté, J. S., Polerecky, L., Villanueva, L., and Villanueva, L. (2020b). Nitrate Promotes the Transfer of Methane‐derived Carbon from the Methanotroph Methylobacter Sp. To the Methylotroph Methylotenera Sp. in Eutrophic lake Water. Limnol. Oceanogr. 66, 878–891. doi:10.1002/lno.11648
Guo, M., Zhuang, Q., Tan, Z., Shurpali, N., Juutinen, S., Kortelainen, P., et al. (2020). Rising Methane Emissions from Boreal Lakes Due to Increasing Ice-free Days. Environ. Res. Lett. 15, 064008. doi:10.1088/1748-9326/ab8254
Han, X., Schubert, C. J., Fiskal, A., Dubois, N., and Lever, M. A. (2020). Eutrophication as a Driver of Microbial Community Structure in lake Sediments. Environ. Microbiol. 22, 3446–3462. doi:10.1111/1462-2920.15115
He, R., Wooller, M. J., Pohlman, J. W., Quensen, J., Tiedje, J. M., and Leigh, M. B. (2012). Shifts in Identity and Activity of Methanotrophs in Arctic Lake Sediments in Response to Temperature Changes. Appl. Environ. Microbiol. 78, 4715–4723. doi:10.1128/AEM.00853-12
He, R., Wang, J., Pohlman, J. W., Jia, Z., Chu, Y.-X., Wooller, M. J., et al. (2021). Metabolic Flexibility of Aerobic Methanotrophs under Anoxic Conditions in Arctic lake Sediments. ISME J. 16, 78–90. doi:10.1038/s41396-021-01049-y
Heathcote, A. J., and Downing, J. A. (2012). Impacts of Eutrophication on Carbon Burial in Freshwater Lakes in an Intensively Agricultural Landscape. Ecosystems 15, 60–70. doi:10.1007/s10021-011-9488-9
Hernandez, M. E., Beck, D. A. C., Lidstrom, M. E., and Chistoserdova, L. (2015). Oyxgen Availability is a Major Factor in Determining the Composition of Microbial Communities Involved in Methane Oxidation. Peer J. 3, e801. doi:10.7717/peerj.801
Holmes, A. J., Costello, A., Lidstrom, M. E., and Murrell, J. C. (1995). Evidence that Participate Methane Monooxygenase and Ammonia Monooxygenase May Be Evolutionarily Related. FEMS Microbiol.Lett. 132, 203–208. doi:10.1111/j.1574-6968.1995.tb07834.x
Jones, R. I., and Grey, J. (2011). Biogenic Methane in Freshwater Food Webs. Freshw. Biol. 56, 213–229. doi:10.1111/j.1365-2427.2010.02494.x
Kalyuzhnaya, M. G., Yang, S., Rozova, O. N., Smalley, N. E., Clubb, J., Lamb, A., et al. (2013). Highly Efficient Methane Biocatalysis Revealed in a Methanotrophic Bacterium. Nat. Commun. 4, 1–7. doi:10.1038/ncomms3785
Kits, K. D., Campbell, D. J., Rosana, A. R., and Stein, L. Y. (2015a). Diverse Electron Sources Support Denitrification under Hypoxia in the Obligate Methanotroph Methylomicrobium Album Strain BG8. Front. Microbiol. 6, 1–11. doi:10.3389/fmicb.2015.01072
Kits, K. D., Klotz, M. G., and Stein, L. Y. (2015b). Methane Oxidation Coupled to Nitrate Reduction under Hypoxia by the GammaproteobacteriumMethylomonas Denitrificans, Sp. Nov. Type Strain FJG1. Environ. Microbiol. 17, 3219–3232. doi:10.1111/1462-2920.12772
Kiyashko, S., Narita, T., and Wada, E. (2001). Contribution of Methanotrophs to Freshwater Macroinvertebrates: Evidence from Stable Isotope Ratios. Aquat. Microb. Ecol. 24, 203–207. doi:10.3354/ame024203
Knief, C. (2015). Diversity and Habitat Preferences of Cultivated and Uncultivated Aerobic Methanotrophic Bacteria Evaluated Based on pmoA as Molecular Marker. Front. Microbiol. 6, 1346. doi:10.3389/fmicb.2015.01346
Lerman, A. (1979). Geochemical Processes: Water and Sediment Environments. New York, NY: John Wiley & Sons.
Leu, A. O., Cai, C., McIlroy, S. J., Southam, G., Orphan, V. J., Yuan, Z., et al. (2020). Anaerobic Methane Oxidation Coupled to Manganese Reduction by Members of the Methanoperedenaceae. ISME J. 14, 1030–1041. doi:10.1038/s41396-020-0590-x
Lever, M. A., Torti, A., Eickenbusch, P., Michaud, A. B., Săntl-Temkiv, T., Jørgensen, B. B., et al. (2015). A Modular Method for the Extraction of DNA and RNA, and the Separation of DNA Pools from Diverse Environmental Sample Types. Front. Microbiol. 6, 476. doi:10.3389/fmicb.2015.00476
Lloréns-Rico, V., Vieira-Silva, S., Gonçalves, P. J., Falony, G., and Raes, J. (2021). Benchmarking Microbiome Transformations Favors Experimental Quantitative Approaches to Address Compositionality and Sampling Depth Biases. Nat. Commun. 12. doi:10.1038/s41467-021-23821-6
Ludwig, W., Strunk, O., Westram, R., Richter, L., Meier, H., Yadhukumar, A., et al. (2004). ARB: A Software Environment for Sequence Data. Nucleic Acids Res. 32, 1363–1371. doi:10.1093/nar/gkh293
Lyautey, E., Billard, E., Tissot, N., Jacquet, S., and Domaizon, I. (2021). Seasonal Dynamics of Abundance, Structure, and Diversity of Methanogens and Methanotrophs in Lake Sediments. Microb. Ecol. 82, 559–571. doi:10.1007/s00248-021-01689-9
Maerki, M., Wehrli, B., Dinkel, C., and Müller, B. (2004). The Influence of Tortuosity on Molecular Diffusion in Freshwater Sediments of High Porosity 1 1Associate Editor: M. L. Machesky. Geochimica et Cosmochimica Acta 68, 1519–1528. doi:10.1016/j.gca.2003.09.019
Mayr, M. J., Zimmermann, M., Guggenheim, C., Brand, A., and Bürgmann, H. (2020). Niche Partitioning of Methane-Oxidizing Bacteria along the Oxygen-Methane Counter Gradient of Stratified Lakes. ISME J. 14, 274–287. doi:10.1038/s41396-019-0515-8
McDonald, I. R., Bodrossy, L., Chen, Y., and Murrell, J. C. (2008). Molecular Ecology Techniques for the Study of Aerobic Methanotrophs. Appl. Environ. Microbiol. 74, 1305–1315. doi:10.1128/AEM.02233-07
Mukherjee, S., Stamatis, D., Bertsch, J., Ovchinnikova, G., Sundaramurthi, J. C., Lee, J., et al. (2021). Genomes OnLine Database (GOLD) v.8: Overview and Updates. Nucleic Acids Res. 49, D723–D733. doi:10.1093/nar/gkaa983
Müller, B., Wang, Y., Dittrich, M., and Wehrli, B. (2003). Influence of Organic Carbon Decomposition on Calcite Dissolution in Surficial Sediments of a Freshwater lake. Water Res. 37, 4524–4532. doi:10.1016/S0043-1354(03)00381-6
Naeher, S., Gilli, A., North, R. P., Hamann, Y., and Schubert, C. J. (2013). Tracing Bottom Water Oxygenation with Sedimentary Mn/Fe Ratios in Lake Zurich, Switzerland. Chem. Geology. 352, 125–133. doi:10.1016/j.chemgeo.2013.06.006
Ogle, D., Doll, J., Wheeler, P., and Dinno, A. (2021). FSA: Fisheries Stock Analysis. R package version 0.9.1, Available at: https://github.com/droglenc/FSA.
Oksanen, J., Blanchet, F. G., Friendly, M., Kindt, R., Legendre, P., and McGlinn, D. (2020). Vegan: Community Ecology Package: R Package Version 2.5-7. Available at: https://scholar.google.com/scholar?hl=en&as_sdt=0%2C5&q=vegan%3A+Community+Ecology+Package.+R+++package+version+2.5-7&btnG= [Accessed January 11, 2022].
Oshkin, I. Y., Beck, D. A., Lamb, A. E., Tchesnokova, V., Benuska, G., McTaggart, T. L., et al. (2014). Methane-fed Microbial Microcosms Show Differential Community Dynamics and Pinpoint Taxa Involved in Communal Response. ISME J. 9, 1119–1129. doi:10.1038/ismej.2014.203
Oshkin, I. Y., Miroshnikov, K. K., Grouzdev, D. S., and Dedysh, S. N. (2020). Pan-genome-based Analysis as a Framework for Demarcating Two Closely Related Methanotroph Genera Methylocystis and Methylosinus. Microorganisms 8, 768. doi:10.3390/microorganisms8050768
Oswald, K., Milucka, J., Brand, A., Hach, P., Littmann, S., Wehrli, B., et al. (2016). Aerobic Gammaproteobacterial Methanotrophs Mitigate Methane Emissions from Oxic and Anoxic lake Waters. Limnol. Oceanogr. 61, S101–S118. doi:10.1002/lno.10312
Pester, M., Schleper, C., and Wagner, M. (2011). The Thaumarchaeota: an Emerging View of Their Phylogeny and Ecophysiology. Curr. Opin. Microbiol. 14, 300–306. doi:10.1016/j.mib.2011.04.007
Pierangeli, G. M. F., Domingues, M. R., Jesus, T. A. d., Coelho, W. S., Pompêo, M. L. M., Saia, F. T., et al. (2021). Higher Abundance of Sediment Methanogens and Methanotrophs Do Not Predict the Atmospheric Methane and Carbon Dioxide Flows in Eutrophic Tropical Freshwater Reservoirs. Front. Microbiol. 12, 1–15. doi:10.3389/fmicb.2021.647921
Rahalkar, M., Deutzmann, J., Schink, B., and Bussmann, I. (2009). Abundance and Activity of Methanotrophic Bacteria in Littoral and Profundal Sediments of lake Constance (Germany). Appl. Environ. Microbiol. 75, 119–126. doi:10.1128/AEM.01350-08
Reim, A., Lüke, C., Krause, S., Pratscher, J., and Frenzel, P. (2012). One Millimetre Makes the Difference: High-Resolution Analysis of Methane-Oxidizing Bacteria and Their Specific Activity at the Oxic-Anoxic Interface in a Flooded Paddy Soil. ISME J. 6, 2128–2139. doi:10.1038/ismej.2012.57
Rognes, T., Flouri, T., Nichols, B., Quince, C., and Mahé, F. (2016). VSEARCH: A Versatile Open Source Tool for Metagenomics. PeerJ 4, e2584. doi:10.7717/PEERJ.2584/FIG-7
Sanches, L. F., Guenet, B., Marinho, C. C., Barros, N., and de Assis Esteves, F. (2019). Global Regulation of Methane Emission from Natural Lakes. Sci. Rep. 9, 1–10. doi:10.1038/s41598-018-36519-5
Schubert, C. J., Diem, T., and Eugster, W. (2012). Methane Emissions from a Small Wind Shielded lake Determined by Eddy Covariance, Flux chambers, Anchored Funnels, and Boundary Model Calculations: A Comparison. Environ. Sci. Technol. 46, 4515–4522. doi:10.1021/es203465x
Smith, G. J., Angle, J. C., Solden, L. M., Borton, M. A., Morin, T. H., Daly, R. A., et al. (2018). Members of the Genus Methylobacter Are Inferred to Account for the Majority of Aerobic Methane Oxidation in Oxic Soils from a Freshwater Wetland. MBio 9, 1–17. doi:10.1128/mBio.00815-18
Teranes, J. L., McKenzie, J. A., Lotter, A. F., and Sturm, M. (1999). Stable Isotope Response to lake Eutrophication: Calibration of a High-Resolution Lacustrine Sequence from Baldeggersee, Switzerland. Limnol. Oceanogr. 44, 320–333. doi:10.4319/lo.1999.44.2.0320
Thauer, R. K., Jungermann, K., and Decker, K. (1977). Energy Conservation in Chemotrophic Anaerobic Bacteria. Bacteriol. Rev. 41, 100–180. doi:10.1128/br.41.1.100-180.1977
Thebrath, B., Rothfuss, F., Whiticar, M. J., and Conrad, R. (1993). Methane Production in Littoral Sediment of Lake Constance. FEMS Microbiol. Ecol. 102, 279–289. doi:10.1111/j.1574-6968.1993.tb05819.x
Thottathil, S. D., Reis, P. C. J., del Giorgio, P. A., and Prairie, Y. T. (2018). The Extent and Regulation of Summer Methane Oxidation in Northern Lakes. J. Geophys. Res. Biogeosci. 123, 3216–3230. doi:10.1029/2018JG004464
Tranvik, L. J., Downing, J. A., Cotner, J. B., Loiselle, S. A., Striegl, R. G., Ballatore, T. J., et al. (2009). Lakes and Reservoirs as Regulators of Carbon Cycling and Climate. Limnol. Oceanogr. 54, 2298–2314. doi:10.4319/lo.2009.54.6_part_2.2298
Tsutsumi, M., Kojima, H., and Fukui, M. (2012). Vertical Profiles of Abundance and Potential Activity of Methane-Oxidizing Bacteria in Sediment of Lake Biwa, Japan. Microb. Environ. 27, 67–71. doi:10.1264/jsme2.ME11285
van Grinsven, S., Oswald, K., Wehrli, B., Jegge, C., Zopfi, J., Lehmann, M. F., et al. (2021). Methane Oxidation in the Waters of a Humic-Rich Boreal lake Stimulated by Photosynthesis, Nitrite, Fe(III) and Humics. Biogeosciences 18, 3087–3101. doi:10.5194/bg-18-3087-2021
Vekeman, B., Kerckhof, F.-M., Cremers, G., de Vos, P., Vandamme, P., Boon, N., et al. (2016). New Methyloceanibacter Diversity from North Sea Sediments Includes Methanotroph Containing Solely the Soluble Methane Monooxygenase. Environ. Microbiol. 18, 4523–4536. doi:10.1111/1462-2920.13485
Yang, Y., Chen, J., Tong, T., Li, B., He, T., Liu, Y., et al. (2019). Eutrophication Influences Methanotrophic Activity, Abundance and Community Structure in Freshwater Lakes. Sci. Total Environ. 662, 863–872. doi:10.1016/j.scitotenv.2019.01.307
Yang, Y., Zhao, Q., Cui, Y., Wang, Y., Xie, S., and Liu, Y. (2016). Spatio-temporal Variation of Sediment Methanotrophic Microorganisms in a Large Eutrophic lake. Microb. Ecol. 71, 9–17. doi:10.1007/s00248-015-0667-7
Zheng, Y., Wang, H., Liu, Y., Zhu, B., Li, J., Yang, Y., et al. (2020). Methane-Dependent Mineral Reduction by Aerobic Methanotrophs under Hypoxia. Environ. Sci. Technol. Lett. 7, 606–612. doi:10.1021/acs.estlett.0c00436
Keywords: greenhouse gas emissions, MOB, methanotrophy, microbial community, eutrophication, oligotrophic, methane oxidation, pmoA
Citation: van Grinsven S, Meier DV, Michel A, Han X, Schubert CJ and Lever MA (2022) Redox Zone and Trophic State as Drivers of Methane-Oxidizing Bacterial Abundance and Community Structure in Lake Sediments. Front. Environ. Sci. 10:857358. doi: 10.3389/fenvs.2022.857358
Received: 18 January 2022; Accepted: 14 February 2022;
Published: 10 March 2022.
Edited by:
Yves T Prairie, Université du Québec à Montréal, CanadaReviewed by:
Nils Risgaard-Petersen, Aarhus University, DenmarkDirk de Beer, Max Planck Society, Germany
Copyright © 2022 van Grinsven, Meier, Michel, Han, Schubert and Lever. This is an open-access article distributed under the terms of the Creative Commons Attribution License (CC BY). The use, distribution or reproduction in other forums is permitted, provided the original author(s) and the copyright owner(s) are credited and that the original publication in this journal is cited, in accordance with accepted academic practice. No use, distribution or reproduction is permitted which does not comply with these terms.
*Correspondence: Sigrid van Grinsven, c2lncmlkLnZhbmdyaW5zdmVuQGVhd2FnLmNo; Mark A. Lever, bWFyay5sZXZlckB1c3lzLmV0aHouY2g=
†Present address: Xingguo Han, Federal Institute for Forest, Snow, and Landscape Research (WSL), Zürcherstrasse, Switzerland
‡These authors have contributed equally to this work and share first authorship