- 1ICAR- Indian Institute of Soil Science, Nabibagh, Bhopal, India
- 2Department of Soil Science and Agriculture Chemistry, College of Agriculture, RVSKVV, Gwalior, India
Crop residues as key organic carbon inputs have the potential for soil carbon sequestration. However, previous studies have shown an inconsistent effect of residue return on the direction and magnitude of soil nitrous oxide (N2O) emission. We used a laboratory-based soil incubation study to test the response of N2O emission to crop residue type, soil moisture, and how nutrient management modulates these responses. In this study, we incorporated crop residues with different qualities (wheat, rice, soybean, and maize) at two soil moisture contents {80% field capacity (FC) and 60% FC} and under seven nutrient levels: N0P0K0 (no nutrients), N0PK, N100PK, N150PK, N100PK + manure@ 5 Mg ha−1, N100PK + biochar@ 5 Mg ha−1, and N150PK + biochar@ 5 Mg ha−1. The results demonstrated significant (p < 0.01) differences in the magnitude of N2O emissions among treatments. However, only the interaction effect of residue × nutrient and nutrient × moisture was significant (p < 0.05). N100PK and N150PK at 80% FC mitigated N2O emission by approximately 20% in wheat residue-amended soil (cf. control soil without residue). In contrast, maize residue amendment (cf. control soil) increased N2O emission by 130% under N0P0K0 and 80% FC. Residue effects were negatively correlated with the C:N ratio, and a strong positive correlation (p < 0.01) was obtained between N2O emission and CO2 respiration, labile carbon, mineral N, and residue total nitrogen (TN). When no nutrients were added, N2O emission was higher in residue returned soil. However, cumulative fluxes of N2O decreased by 6–17% when maize and wheat residues (cf. control soil) were applied with nutrients. Negative fluxes of N2O indicating consumption were observed in every treatment after 57 days of incubation and were most pronounced in control soil without residue and nutrients. Decreasing the soil moisture from 80% FC to 60% FC, the N2O consumption rate increased by 6.6 times across residue types and nutrient management. The regression analysis and structural equation modeling (SEM) results showed that residue TN, soil CO2 emission, NO3-N, and labile SOC were the key predictor variables and could explain 82% variability in the soil N2O emission in the Vertisols of Central India. The results suggested that nutrient addition (NPK) could alter the magnitude and direction of soil N2O flux by residue type and soil moisture by influencing the underlying soil microbial processes of the C and N cycle in the Vertisol of subtropical India.
Introduction
Sustainable mitigation strategies to reduce soil nitrous oxide emission are vital for cleaner and carbon-neutral agricultural production. It is also because nitrous oxide is an important greenhouse gas (GHG), and the agricultural soils contribute approximately 78% of total anthropogenic N2O emissions, with a global warming potential of 265 times greater than CO2 (Mutegi et al., 2010; Sangeeta Lenka et al., 2017). Moreover, N2O is the main source of stratospheric nitric oxide, which damages the ozone layer (Lenka et al., 2020a). Increasing crop production to meet the growing food demand of the rising population could generate crop residues as high as 4 billion metric tonnes annually globally (Chen et al., 2021). Crop residue incorporation in the soil is a sustainable and eco-friendly residue recycling and reuse mechanism in agriculture. It improves plant growth by enhancing soil carbon sequestration and nutrient cycling by driving soil processes (Wei et al., 2015; Lenka et al., 2020b; Jin et al., 2020; Wu et al., 2020). In addition, its beneficial effect is well proven and documented for soil and water conservation (Kaleeem Abbasi et al., 2015; Nath et al., 2021). However, incorporating crop residues in the soil could be a potential source of agricultural N2O emissions during decomposition and mineralization. The N2O emissions from crop residue return could offset the beneficial effects of crop residue recycling in agriculture (Wang and Luo, 2018; Hu et al., 2019). As the emphasis on the surface retention or incorporation of crop residue is growing with the increase in area under conservation agriculture globally, it is essential to devise strategies for reducing GHG N2O emissions from crop residue return.
The crucial factors governing N2O emission from residue could be 1) residue quality, 2) soil properties, 3) soil environment (temperature and moisture), and 4) exogenous nutrient input (inorganic/organic). Previous studies have reported inconsistent effects of crop residue return on N2O emission, i.e., either positive (Velthof et al., 2002; Wang et al., 2011; Venterea et al., 2012; Chen et al., 2013; Gao et al., 2016; Wang and Luo, 2018) or negative and no effect (Jianwen et al., 2004; Mutegi et al., 2010; Chen et al., 2013; Hu et al., 2013, 2019; Charles et al., 2017). The crop C:N ratio, cellulose, lignin, total carbon, and nitrogen concentration are essential biochemical compositions governing the residue mineralization and the associated N2O emission. In their meta-analysis, Shan and Yan (2013) showed that the N2O emission from soil was negatively correlated with the C:N ratio of residue added, indicating that crop residue with a wider C:N ratio is more effective in reducing N2O emissions. Apart from the crop C:N ratio, other quality parameters regulating N2O emissions are content of total nitrogen, lignin, cellulose, polyphenols, lignin/N ratio, and soluble C (Muhammad et al., 2011; Chen et al., 2013; Kaleeem Abbasi et al., 2015; Hu et al., 2019). The high C: N ratio of the crop input compared with soil microbial biomass destabilizes the soil and microbial nutrient stoichiometry that alters the SOC and residue C mineralization and the resultant N2O emission (Velthof et al., 2002; Toma and Hatano, 2007; Muhammad et al., 2011; Chen et al., 2013). The variable effect of crop residue C may arise because residue C regulates the same enzyme differently being dependent on concentration, or the same residue C may affect different reductases of the N cycle differently (Giles et al., 2012).
The shifts in nutrient stoichiometry after residue addition are critical to soil N mineralization and immobilization and the associated N2O emissions (Giles et al., 2012; Thomson et al., 2012; Wu et al., 2020). Evidence suggests that nutrient inputs drive N mineralization from crop residue and the subsequent N losses (N2O, NO3 leaching, and volatilization) or use (crop uptake) from the soil system (Rahn et al., 2003; Toma and Hatano, 2007; Muhammad et al., 2011; Li et al., 2013; Xu et al., 2021). Exogenous nutrient input with crop residues influences the magnitude of N2O fluxes by alleviating nutrient limitation to soil microbes (Finn et al., 2016; Fang et al., 2018a; Soong et al., 2018). The input of crop residue with only inorganic nutrients or integrated use of nutrients was reported to either increase (Baggs et al., 2000; Toma and Hatano, 2007; Datta et al., 2019; Lenka et al., 2019; Fang et al., 2020), decrease, or no effect (Al-Kaisi and Yin, 2005; Toma and Hatano, 2007; Fang et al., 2018b; Datta et al., 2019; Hu et al., 2019) on SOC mineralization. Mechanisms attributed to the variable effect of crop residue addition with exogenous nutrient on the magnitude and direction of SOC mineralization is that 1) crop residue inputs affect the magnitude of native SOC mineralization called priming effect compared with no residue input (Zimmerman et al., 2011; Fang et al., 2018a; Lenka et al., 2019); 2) N addition with crop residue increases microbial carbon use efficiency of residue carbon (Zang et al., 2016; Mehnaz et al., 2019; Fang et al., 2020); 3) reduced mineral nitrogen changes the soil microbial community structure that mineralizes the native SOC more to meet their requirement of growing energy and nutrition (Zang et al., 2016; Li et al., 2017; Zhang et al., 2018); 4) suppresses the SOC mineralization through reduced extracellular enzyme activity. Therefore, underlying mechanisms driving N2O emission from crop residue return are complex and remain uncertain that demands further investigation (Hu et al., 2019).
In addition to the chemical composition of crop residue and nutrient input, environmental factors such as soil moisture regulates the oxygen diffusion rates in soil, a determinant for nitrification and denitrification (Wang et al., 2011; Venterea et al., 2012; Chen et al., 2013). Generally, denitrification is the primary source of N2O production in soils under anaerobic conditions accounting for 1–100% of the total N2O production. However, nitrification dominated the N2O production under aerobic conditions (Giles et al., 2012; Hu et al., 2019; Wang et al., 2021). Soil moisture is the key factor governing soil’s aerobic/anaerobic status through the water-filled pore space. Under the aerobic conditions, return of crop residues could further modify the microbial oxygen demand due to enhanced microbial activity in response to high residue C, which is a key factor driving soil nitrification and denitrification for N2O emission (Baggs et al., 2000; Toma and Hatano, 2007; Hu et al., 2019). Ciarlo et al. (2007) found that the N2O fluxes were greater at 80% than 60% water-filled porosity space (WFPS) when NO3− concentration was nonlimiting. Therefore, assessing the magnitude of soil N2O emission in response to different crop residue inputs and nutrient management under specified soil moisture is essential for identifying management practices vital to mitigate GHG emissions and global changes.
The individual effect of crop residue, nutrient management, and soil moisture on N2O emissions was well documented by previous studies (Feng et al., 2003; Toma and Hatano 2007; Mutegi et al., 2010; Ponce-Mendoza et al., 2010; Chen et al., 2013; Shan and Yan 2013; Gao et al., 2016; Lan et al., 2017; Kumar et al., 2018; Takakai et al., 2018; Tao et al., 2018; Hongjin Zhang et al., 2020; Liyanage et al., 2020; Wu et al., 2020; Yujin Zhang et al., 2020; Li et al., 2021). However, the interactive effect of crop residues, nutrients, and soil moisture driving the magnitude and direction of soil N2O emission and underlying mechanisms is lacking. Therefore, the objectives of the present investigation were to 1) quantify the interactive effect of crop residues, nutrients, and soil moisture on nitrogen mineralization and related soil properties, 2) assess the effect of crop residue return on N2O emission from Vertisols under different nutrient management and soil moisture, and 3) quantify the relative importance of soil and residue properties in predicting soil N2O emission.
Materials and Methods
Study Site
We used a laboratory-based soil incubation approach to test whether crop residue addition in the soil can affect nitrous oxide emissions at different moisture contents and how these responses are modulated by nutrient management. In this study, we incorporated crop residues (wheat, rice, soybean, and maize) with two contrasting soil moisture contents (see the following) at seven nutrient levels (see the following). The surface soil (0–15 cm) used in this study was collected from a long-term experimental field under conservation tillage and nutrient management at ICAR-Indian Institute of Soil Science, Bhopal, India. The experimental site is located at 23°15′N latitude and 77°25′E longitude, at 427 m above the mean sea level and is characterized by a humid subtropical climate with mild, dry winters and hot summers followed by a humid monsoon season. For other experimental details and initial soil properties of the site, a reference is made to Singh et al. (2020, 2014). The on-site soil is deep Vertisols (Isohyperthermic Typic Haplustert) with clay texture (52% clay), bulk density of 1.34 Mg/m3 at 0.27 g/g soil water content, and total soil organic carbon content of 0.99% (0–15 cm soil depth). The soil is neutral to alkaline in reaction (pH-7.85) with an electrical conductivity of 0.3 ds/m and Ca2+ as the dominant exchangeable cation in the Ap horizon.
Incubation Experimental Detail
The surface soil (0–15 cm) was collected after the wheat harvest in 2020 from a 12-year-old conservation tillage experiment in a soybean–wheat cropping system under reduced tillage with 30% residue return plus the recommended dose of nutrients. For soybean, the recommended dose was 30:60:30 and for wheat, it was 100:60:30 kg N–P2O5–K2O per ha. All visible roots and crop residue materials were removed from the collected soil, and the samples were passed through a 2-mm sieve to remove larger residue fragments and particles. Soils were stored at 4°C until further analysis. The crop residues of rice, maize, soybean, and wheat used in this study were air-dried, and the samples of crop residues were milled and sieved to 2 mm. The subsamples of the residues were dried at 65°C for 48 h for gravimetric water content assessment and ground and sieved to <2 mm for chemical analysis. The concentration of C and N in the initial bulk soil, the residues, manure, and biochar was determined using an elemental analyzer (NC analyzer, Thermo Fisher, Flash 2000 model). The lignin and cellulose contents were determined by the acid detergent fiber method using the 1-mm-sized materials (Rowland and Roberts, 1994). The moisture content of the sieved material was determined by oven-drying at 105°C overnight. Selected properties of residues, sugarcane bagasse biochar, farmyard manure, and soil used in this study are listed in Table 1. For further details of biochar, reference is made to Raul et al. (2020).
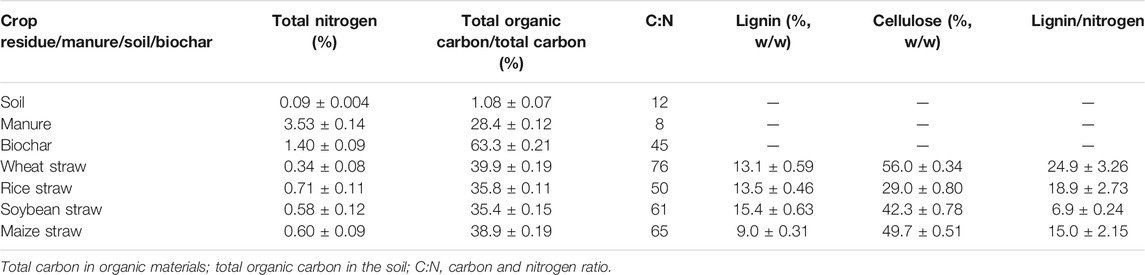
TABLE 1. Selected chemical properties of the residues, biochar, manure, and soil used in this study.
The soil was preincubated for 10 days at room temperature and 70% of the two moisture levels to minimize the possible disturbance effect during soil sample preparation. After preincubation, the crop residues (<2 mm) were thoroughly mixed with soil (<2 mm) and homogenized before incubation. In brief, the treatments consisted of 1) 20 g soils (dry weight basis) added with wheat, maize, soybean, or rice straw residues at the rate of 2.23 mg g−1 soil, corresponding to 5 Mg ha−1 residue incorporation and 2) 20 g soil (dry weight basis) without crop residue (control). The treatments were taken in 460-ml glass jars. The control soil and residue-incorporated soil were given seven nutrient addition treatments: N0P0K0 (no nutrient), N0PK, N100PK, N150PK, N100PK + manure at 5 Mg ha−1, N100PK + biochar@ 5 Mg ha−1, and N150PK + biochar@ 5 Mg ha−1. The N100 and N150 denote the rates of 100 and 150 kg N ha−1. Phosphorus (P) at 22 kg/ha and potassium (K) at 21 kg/ha were added to each nutrient treatment except N0P0K0 to study the effect of increasing N levels. The P and K additions were made to maintain an ideal N:P:K ratio of 4:2:1 corresponding to 100 kg N/ha. The N addition was made through AR-grade ammonium nitrate, whereas P and K additions were made through potassium dihydrogen phosphate. All the nutrients were applied through distilled water, and then the incubation moisture level was adjusted to 80% field capacity (FC) and 60% FC with distilled water. The field capacity of the soil was determined at matric potentials of −33 kPa using sieved (<2 mm) soil samples in pressure plate extractors (Soil Moisture Equipment Corp., Santa Barbara, CA, United States). A blank glass jar without soil or residue was included to account for the atmospheric CO2 and N2O concentration present in the headspace of the incubation jars and for calculating the amount of evolved gases from the soil or soil plus residue treatments. All treatments and blank were replicated six times and incubated under two different moisture contents: 80% FC and 60% FC at 30°C. The incubation temperature of 30°C was based on long-term annual average temperature of the study region. Soil moisture was adjusted to 80% FC and 60% FC at the start of the incubation and maintained periodically throughout the experiment by weighing the jars and adding water to replace water lost to evaporation during each gas sampling time.
Greenhouse Gas Sampling and Measurements
Headspace gases were sampled at regular intervals on fixed days (0, 1, 4, 10, 17, 26, 33, 40, 47, 57, 67, 77 and 87 days of incubation). The gas samples were drawn from the incubation jars using a syringe and immediately transferred to an evacuated glass vial (Lenka et al., 2019). After all the jars were sampled, jars were opened for 30 min to refresh headspace oxygen and CO2 to ambient concentration and then hermetically resealed with the aluminum caps. The concentration of total headspace CO2 and N2O was measured via gas chromatography (Agilent Technologies model 7890A). The unequal interval was designed to capture the asymptotic decrease commonly observed in incubation experiments. The N2O/CO2 flux rate was calculated as the change in headspace N2O/CO2 concentration (μg N or mg C) per kg soil (dry wt. equivalent) per unit incubation time (day). The ideal gas law was used to convert flux values from a volumetric basis to a mass basis. Cumulative CO2 and N2O emissions were calculated by adding the fluxes from each measurement time. Three replicates were destructively harvested at 57 days and stored at −20°C for analysis of soil mineral N, enzymes, and labile SOC. The three remaining replicates were monitored for GHG fluxes until they were destructively harvested at 87 days. Apparent residue C mineralization was calculated as the change in CO2 emission between residue-amended and control soil samples at the respective nutrient level.
Post-Incubation Soil Analysis
After 57 and 87 days of incubation, soil samples were analyzed for soil mineral N (NO3, NO2, and NH4), soil dehydrogenase activity (DHA), and labile SOC following standard analytical procedures. A part of the moist composite soil samples was used for the determination of gravimetric moisture content by the oven-dry method, and the rest of the samples were extracted immediately with 2 M KCl (1:10 soil:extractant ratio). The extracts were analyzed for NH4-N, NO2-N, and NO3-N using the indophenol blue method (Kempers, 1974) and copperized cadmium reduction method (Jackson et al., 1975). In brief, soil nitrite (NO2-N) was determined by diazotizing with sulfanilamide and coupling with N-(1-naphthyl)-ethylene diamine dihydrochloride to form a pink-colored azo dye that is measured colorimetrically (Jackson et al., 1975).
Dehydrogenase activity was estimated by monitoring the rate of production of triphenyl formazon (TPF) from triphenyl tetrazolium chloride used as an electron acceptor. The method of Klein et al. (1971) was followed for the assay of dehydrogenase activity as outlined in the following. In a 15-ml screw-capped tube, one gram of air-dried soil was placed. To this, 0.2 ml of 3% triphenyl tetrazolium chloride and 0.5 ml of 1% glucose were added, and the tubes were incubated at 28°C for 24 h. After incubation, 10 ml of methanol was added and shaken for precisely 1 min. It was allowed to stand in the dark for 6 h. The color intensity developed was measured at 485 nm (blue filter). From the standard curve, drawn in the range of 0.004–0.4 mg TPF per 10 ml of methanol, the TPF produced in the samples was computed. Dehydrogenase activity was expressed as TPF formed per gram soil for 24 h on an oven-dry weight basis.
Labile soil organic carbon was computed in the soil samples after an incubation period of 57 and 87 days by the potassium permanganate oxidation (0.33 M KMnO4) method as described by Blair et al. (1995) and Islam et al (2003). In brief, 5 g of the soil sample was weighed in a centrifuge tube, and then 20 ml of 0.02 M KMnO4 was added. After shaking it for 2 min on a horizontal shaker (120 rpm), it was centrifuged at 4000–5000 rpm to clear the supernatant. Then, 1 ml of the clear supernatant solution was taken in a 25-ml volumetric flask, and by taking 200 µl in microplate, absorbance was read at 550 nm on a microplate reader.
Statistical Analysis
All data were tested for normality and homogeneity of variance. Log-transformation was applied if the transformation improved the normality and variance substantially. The data were statistically analyzed using SPSS software (version 21.0, SPSS Inc., Chicago, IL, United States); the significance level was set at p = 0.05. As appropriate, the data were analyzed using a general linear model univariate and repeated measures ANOVA. Tukey’s HSD multiple comparisons were used to compare the main factors’ means and obtain the homogenous subsets. We applied Fisher’s protected least significant difference (LSD) for the multiple mean comparisons between treatments when the interaction effect was significant. The Pearson correlation (two-tailed significance) and stepwise multiple regression analysis were applied to detect the predictors of soil N2O emissions. Structural equation modeling (SEM) was performed to determine how crop residue and soil properties estimate soil N2O emissions using AMOS 26.0 (Amos Development Corporation, Meadville, PA, United States). The Chi-square (χ2) statistic of the absolute model fit and various descriptive model fit indices were used to validate the proposed model. The model was considered to have a good fit when χ2 < 5.0, p > 0.05, RMSEA< 0.08, AGFI≥ 0.90, indicating that the proposed model produces a population covariance matrix consistent with the sample covariance matrix (Chen et al., 2021).
Results
Soil Nitrogen Mineralization
The soil NO3-N decreased significantly (p < 0.05) in all residue-amended soil (cf. control soil) across nutrient management and soil moisture, demonstrating immobilization by these materials (Figure 1 and Supplementary Tables S1–S4) at 57 and 87 days of incubation, except soybean residue-amended soil at 87 days. The analysis of variance (ANOVA) for mineral N (NO3-N, NO2-N, and NH4-N) to interpret the main and interactive effects of residue type, soil moisture, nutrient, and repeated measures ANOVA for the two time periods are presented in Tables 2, 3.The content of soil NO3-N ranked wheat < soybean < rice < maize < control soil at 57 days and wheat < rice < maize < control soil < soybean at 87 days of incubation (Figure 1). Nitrogen application with inorganic (P and K) and organic (manure and biochar) nutrients narrowed down the difference between the control (without residue) and residue-amended soil and increased the NO3-N. Across nutrient management, the NO3-N concentration ranged from 35.9 to 108.0 mg kg−1 soil (control soil), 21.0–68.8 mg kg−1 soil (wheat), 21.5–95.5 mg kg−1 soil (rice), 42.6–133.1 mg kg−1 soil (soybean), and 30.0–115.3 mg kg−1 soil (maize). Overall, in the nutrient management treatments, amendment with wheat and rice residues resulted in N immobilization, but soybean and maize residue addition produced net N mineralization. Over the incubation period, the mean cumulative soil NH4-N and NO3-N concentrations increased the response to nitrogen application. In general, biochar application at 5 Mg ha−1 with addition of N (N100PK and N150PK) enhanced the NH4-N concentration as compared to control and residue-amended soil samples.
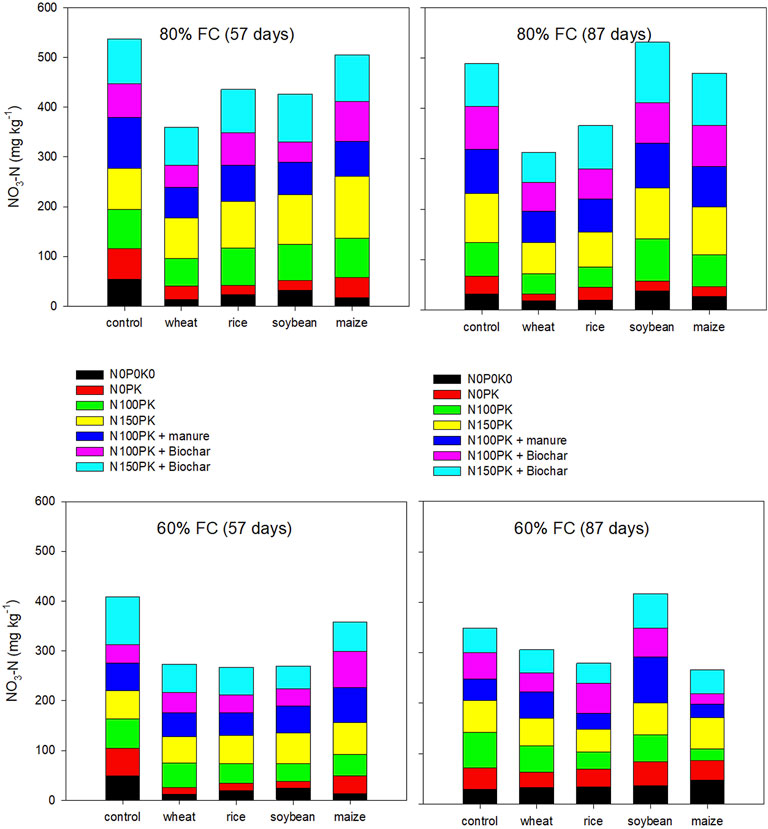
FIGURE 1. Soil NO3-N (mg kg−1 soil) as influenced by residues and nutrients at 57 and 87 days of incubation under 80 and 60% FC soil moisture. Details of statistical analysis are provided in Tables 2, 3.
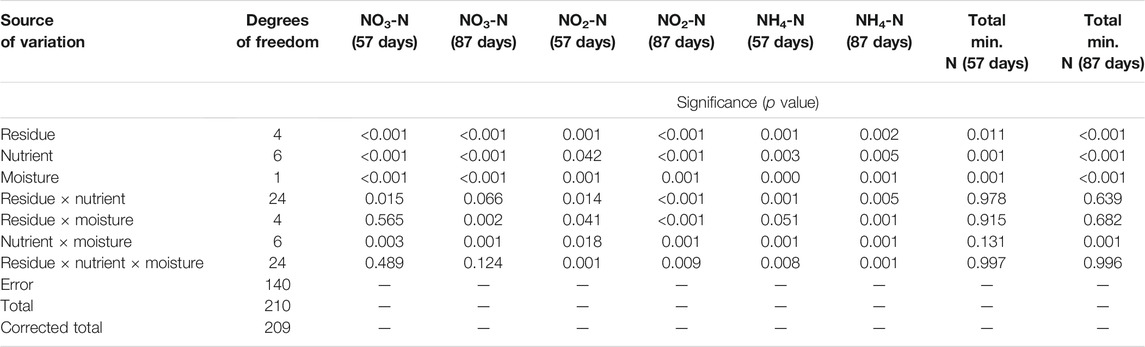
TABLE 2. ANOVA for nitrate N, Nitrite N, ammonical N, and total mineral N at 57 and 87 days of incubation.
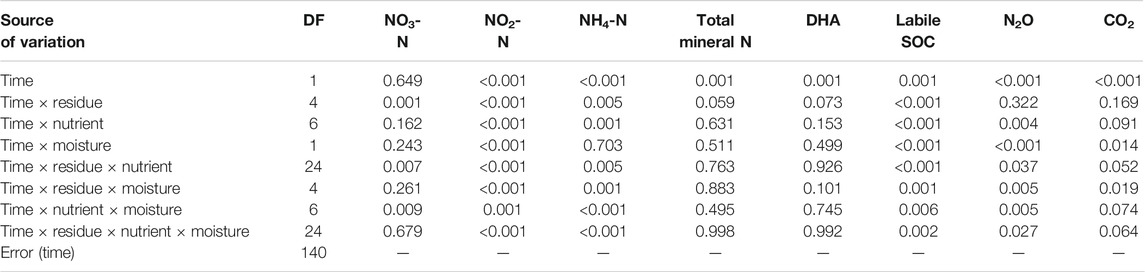
TABLE 3. Results of p value based on repeated measures ANOVA for nitrate N, Nitrite N, ammonical N, total mineral N, dehydrogenase activity (DHA), and labile SOC, CO2, and N2O emission during the incubation period.
During the 87 days of incubation, the total mineral N (NH4 + NO3 + NO2) in the residue + nutrient treatments decreased by 10–27% (wheat), 14–20% (rice), 3–14% (soybean), and 6–17% (maize) as compared to control soil + nutrient treatment, at 80% FC. Similarly, at 60% FC, residue + nutrient treatments decreased the total mineral N compared with control soil + nutrient treatments. In contrast, soybean and maize addition produced net N mineralization in N100PK + manure, N100PK + biochar, and N150PK + biochar treatments, at 80% FC and only soybean at 60% FC moisture content. Overall, soil mineral N and total and individual pools (NH4 NO3, and NO2) decreased significantly (p < 0.01) by reducing the soil moisture from 80 to 60% FC in all nutrient management treatments except a few due to significant nutrient × moisture interaction (p < 0.01).
Nitrous Oxide (N2O) Fluxes
Overall, the N2O fluxes were significantly affected by nutrient management, residue type, soil moisture, and the interaction of residue × nutrient and nutrient × moisture (Table 4). The N2O fluxes over time were initially high, reached their maximum on fourth day (80% FC) and first day (60% FC) of incubation, then decreased exponentially, and was near zero or negative after the 57th day of incubation across nutrient management (Supplementary Figures S1, S2). Therefore, cumulative N2O flux after 87 days of incubation was less than that at 57 days. The percent change in cumulative N2O fluxes at 87 days of incubation over 57 days reflects that the N2O consumption was significantly (p < 0.001) influenced by the interactive effect of residues, nutrients, and moisture (Table 5). Integrated use of residue and nutrients reduced the consumption of N2O across soil moisture. Therefore, control soil without residue had significantly higher N2O consumption than soil amended with crop residue. On decreasing the soil moisture from 80% FC to 60% FC, the N2O consumption rate increased by 6.6 times across residue types and nutrient management. Across nutrient management and soil moisture, the N2O consumption followed the order control soil>maize≈wheat>rice>soybean.
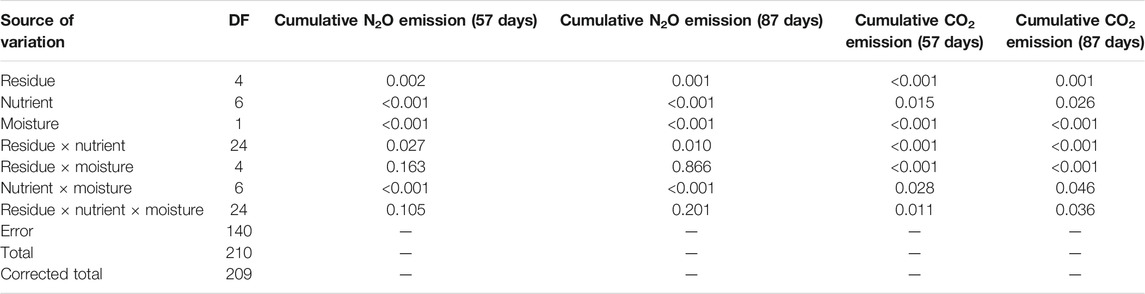
TABLE 4. ANOVA for cumulative N2O emission and soil CO2 respiration after 57 and 87 days of incubation.
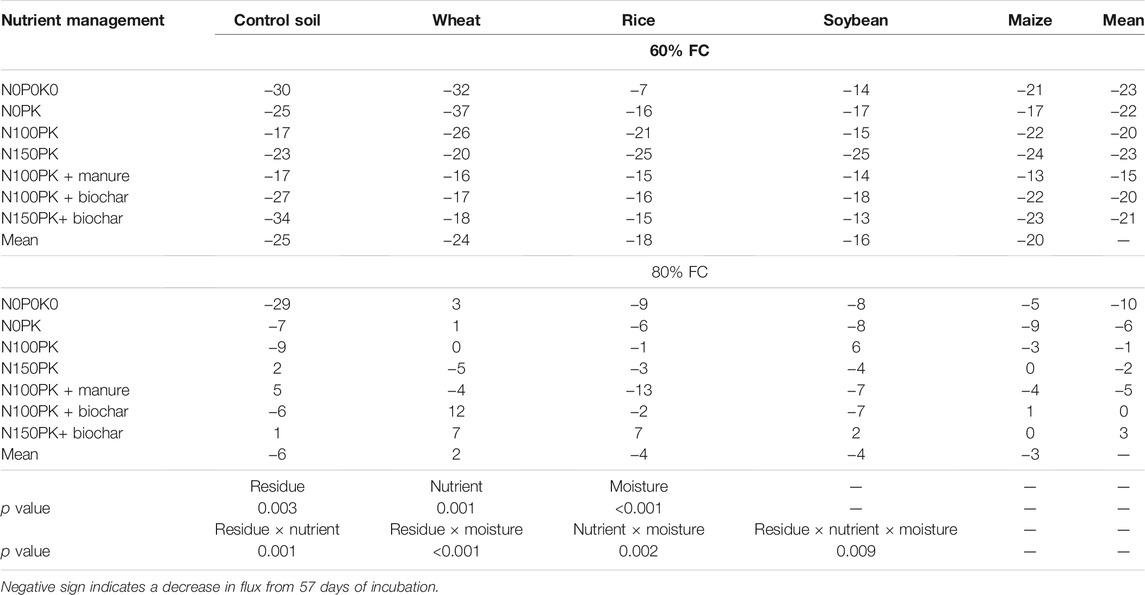
TABLE 5. Percent change in cumulative N2O fluxes at 87 days of incubation over 57 days as influenced by residues and nutrients.
Our results indicated significant interaction of residue × nutrient and nutrient × moisture on net nitrous oxide emission magnitude after 87 days of the incubation period. The simple effects of nutrients were significant for control soil without residue and in soybean and wheat residue-amended treatments. Residue types significantly influenced N2O emissions in N0P0K0, N0PK, and N150PK + biochar. Different nutrient management significantly (p < 0.001) influenced cumulative N2O fluxes at 80% FC soil moisture for residue types, but 60% FC had no effect. For control soil without residue, N100PK + manure and N150PK led to higher N2O emission than N0P0K0 averaged across soil moisture, but there were no differences between nutrient treatments when treated with maize and rice residues. Nutrient addition significantly (p < 0.0001) increased the cumulative N2O emissions in control and residue-amended soils except for maize residue after 87 days of incubation in both soil moisture contents. For example, in control soil without residue, nutrient addition increased the mean N2O emission by 5% (N0PK), 42% (N100PK), 44% (N150PK), 45% (N100PK + manure), 12% (N100PK + biochar), and 21% (N150PK + biochar) compared with no nutrient (N0P0K0) across soil moisture. The results showed the effect of biochar in reducing N2O emission from the nitrogen fertilizer (N100PK and N150PK) was regulated by residue type and residue return. Integrated use of inorganic nutrients with biochar has decreased the N2O emission compared with inorganic fertilizer in soil without residue and maize and rice residue-amended soils (Figure 1). Regardless of soil moisture, integrated use of maize residue and nutrients reduced N2O emission by 31% (N0PK), 19% (N100PK), 20% (N150PK), 19% (N100PK + manure), 29% (N100PK + biochar), and 22% (N150PK + biochar) compared with no nutrient (N0P0K0). Without nutrients, cumulative N2O emission from the residue-amended soil (N0P0K0) was significantly greater than that from the control soil without residue (Figures 2, 3). Without nutrients, in residue-amended and unamended soil, the cumulative N2O emissions during 87 days of incubation followed the order maize>rice≈soybean>wheat>control soil. However, in the plus nutrient treatments in which all the three nutrients were given (N100PK, N150PK, and N100PK + manure), residue addition decreased the soil N2O emission compared with control without residue at 80% FC soil moisture (Figure 3). Unlike 80% FC, the residue addition with nutrients at 60% FC had no consistent effect (increased or decreased) on the emission of N2O. At 87 days of incubation at 80% FC, FC soil moisture had significantly higher (1.53 times) N2O emission than 60% FC.
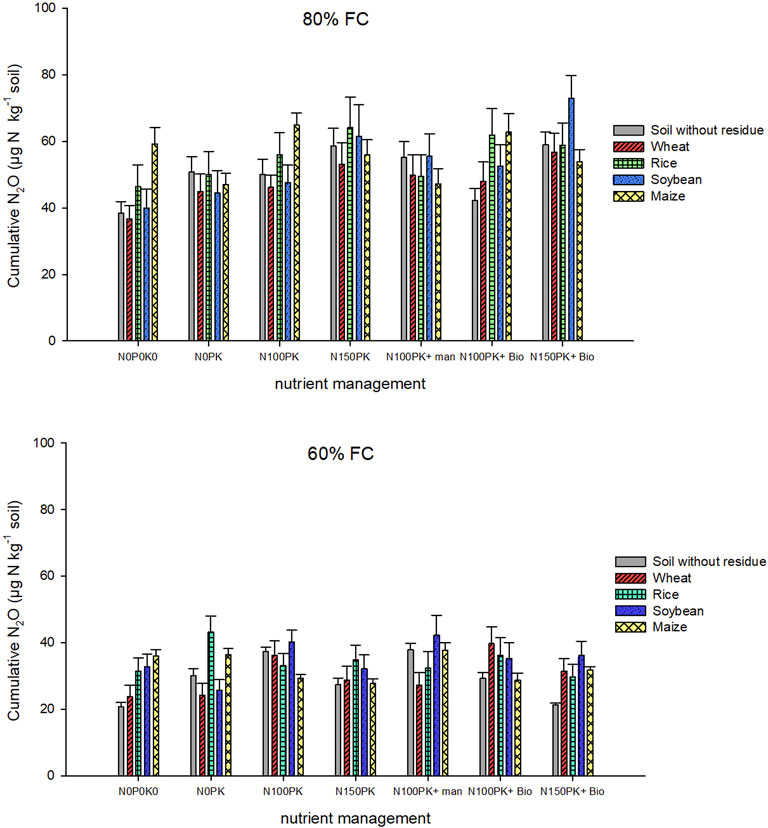
FIGURE 2. Cumulative soil nitrous oxide flux (µg-N kg−1 soil) as influenced by residue types, nutrients, and soil moisture after 87 days of incubation. Details of cumulative N2O flux statistics are provided in Tables 3, 4.
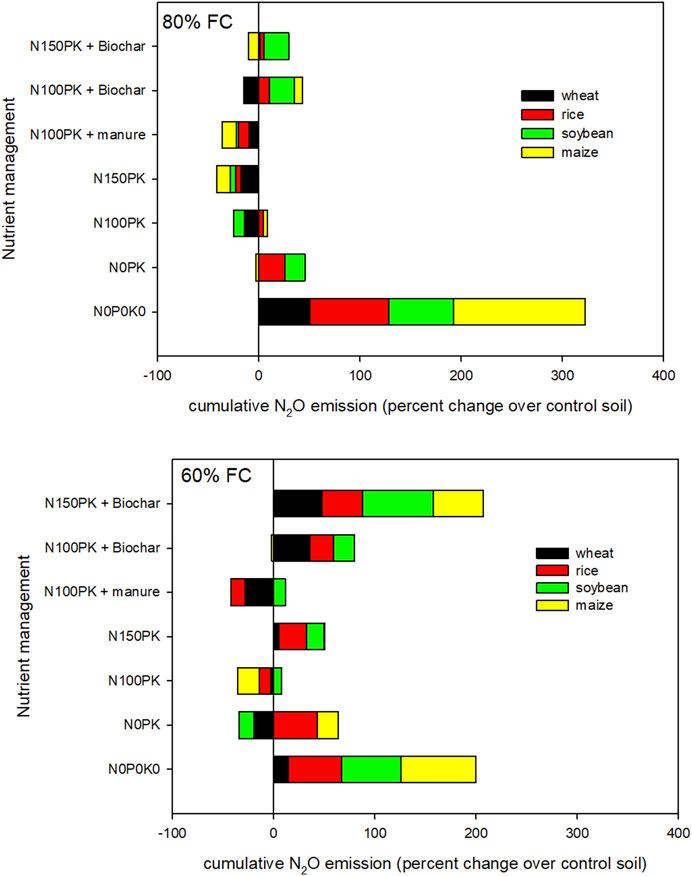
FIGURE 3. Comparative change in cumulative soil nitrous oxide flux (µg-N kg−1 soil) in residue-returned soil over residue-control soil under different residue types, nutrients, and soil moisture levels after 87 days of incubation.
Soil CO2 Respiration
CO2 flux measurement was used to measure the effect of residue on soil CO2 respiration. The interactive effects of time, residue, moisture, and nutrient management significantly influenced the soil CO2 respiration (Tables 3, 4). The soil CO2 respiration rates reached their maximum on the first and fourth day of incubation at 60% FC and 80% FC, respectively, and then decreased gradually (Supplementary Figures S3, S4). The respiration rate was highly variable among residues in the first several days of incubation. Across residue types and nutrient management, the CO2 emission decreased by 62% as the incubation moisture content decreased from 80% FC to 60% FC (Figure 4). Residue addition triggered the mineralization of SOC to CO2, which was significantly greater at higher (80% FC) than lower moisture content (60% FC). Across nutrient and soil moisture, CO2 release was higher by 2.3 (wheat), 2.0 (rice), 1.9 (soybean), and 2.4 (maize) times in residue-returned soils compared with control soil. The order of CO2 release was control < soybean ≈ rice < maize ≈ wheat. There was no significant difference in CO2 release observed between N0P0K0 and nutrient plus treatment except for significantly (p < 0.01) lower values of cumulative CO2 release in N100PK and N150PK + biochar. Across nutrient management, at 80% FC, the cumulative soil respiration (mg CO2-C Kg−1 soil) ranged from 53 to 117 (control soil), 174–258 (wheat residue-amended), 115–201 (rice residue-amended), 128–202 (soybean residue-amended), and 150–221 (maize residue-amended). In maize residue-returned soil under the two soil moisture contents, the cumulative CO2 release decreased with increasing N levels from N0 to N150. For example, at 80% FC soil moisture, the cumulative CO2 release decreased by 19% (N0PK), 28% (N100PK), 25% (N150PK), 34% (N100PK + manure), 3% (N100PK + biochar), and 37% (N150PK + biochar) compared with N0P0K0 (no nutrients) in maize residue-returned treatment.
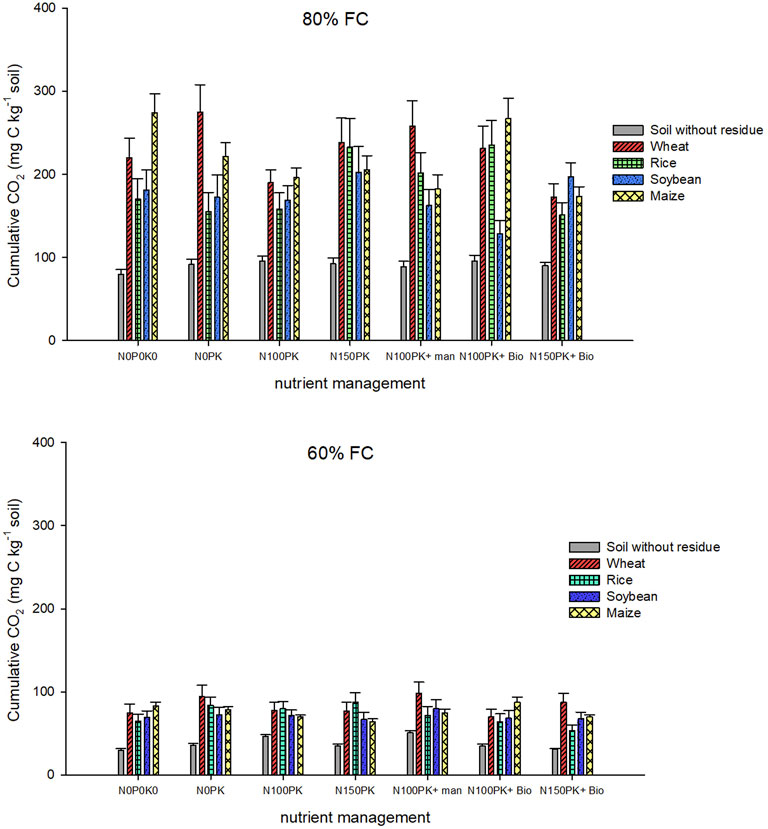
FIGURE 4. Cumulative soil CO2 respiration (mg-C kg−1 soil) as influenced by residue types, nutrients, and soil moisture after 87 days of incubation. Details of statistics of cumulative CO2-C mineralization are provided in Table 4.
Soil Enzyme Activity
The viable microbial activity is determined by the dehydrogenase enzyme (DHA) (Balota et al., 2004). Hence, it can be used as an indicator of soil microbial activity. The DHA was significantly influenced by the main effects of residue types, moisture, temperature, and time (Tables 3, 6). The average DHA ranged between 15.0 and 74.8 µg TPF g−1 soil at 24 h−1 (80% FC) and 14.6–47.9 µg TPF g−1 soil at 24 h−1 (60% FC) and decreased by 23% with a reduction of moisture content from 80% FC to 60% FC (Supplementary Figure S5). Averaged across nutrient treatments, addition of residue significantly (p < 0.001) increased the DHA by 96 and 26% at 80% FC and 60% FC, respectively, as compared with control soil after 87 days of incubation. However, there were no significant differences in DHA between the different types of residues. The DHA significantly increased with nutrient inputs in both residue and moisture levels. Averaged across residue and moisiture levels, the DHA was higher by 21% (N0PK), 50% (N100PK), 75% (N150PK), 57% (N100PK + manure), 38% (N100PK + biochar), and 57% (N150PK + biochar) than that of no nutrient (N0P0K0) treatment.
Labile Soil Organic Carbon Fraction of Soil
Soil organic C oxidized by neutral KMnO4 was used to quantify the labile carbon fraction of soil. Labile SOC was significantly higher at 87 than 57 days of incubation, supported by the significant interactive effect of time × residue × nutrient × moisture (p = 0.002) (Table 3). However, at 57 and 87 days of incubation, only moisture had a significant effect (p < 0.001) on labile SOC (Table 6). Without nutrients (N0P0K0), residue application decreased the labile SOC compared with control soil, at 60% FC (Figure 5). However, integrated use of residue and nutrients relatively increased the labile SOC compared with control soil though not significant. Averaged across residue and nutrient treatments at 87 days of incubation, the labile SOC was higher by 10% due to nutrient addition than that of no nutrient treatment at 60% FC. In contrast, at 80% FC, wheat residue treatment increased the labile SOC compared with control soil, while rice, soybean, and maize residue decreased the labile fraction across nutrient management. At the end of the incubation period (87 days) and averaged across all treatments, labile SOC was lower by 13% at 60% FC than 80% FC. In the control soil without residue, the biochar plus nutrients (N100PK and N150PK) treatments decreased labile SOC compared to other nutrient management treatments without biochar. However, in soil with residue, biochar plus nutrients (N100PK and N150PK) increased the labile SOC by 14% compared with control soil at 60% FC moisture only. In contrast, the effect of biochar was negative or had no impact on labile SOC at the higher moisture content (80% FC) in all residue treatments. Over 87 days, the average labile SOC fraction ranged from 583 to 659 mg kg−1 across treatments at 80% FC and 470–580 mg kg−1 soil at 60% FC.
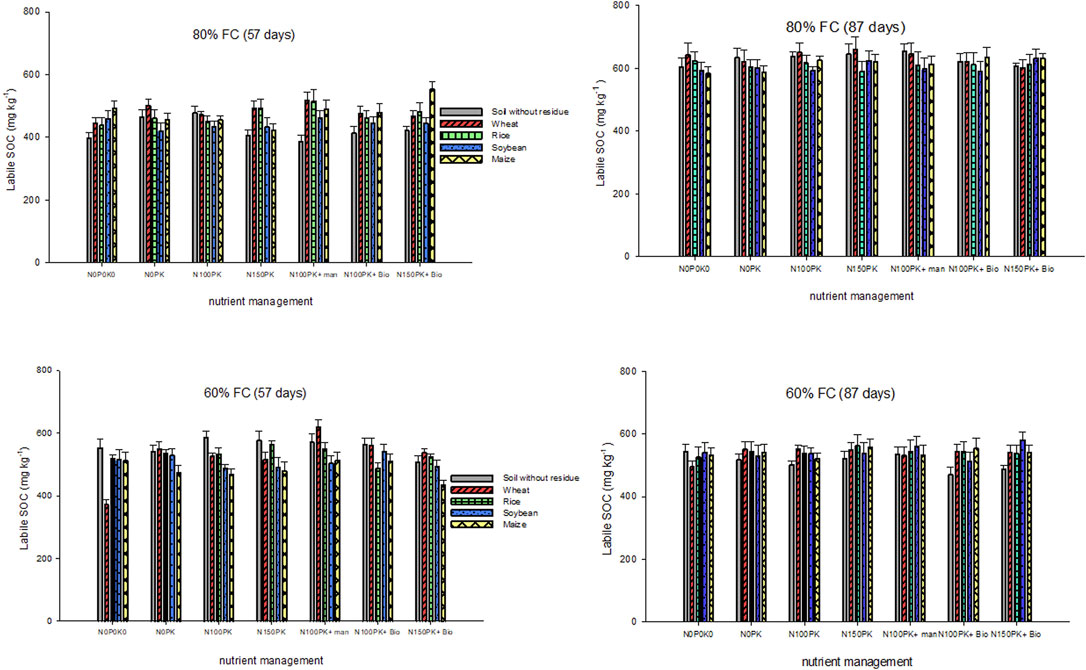
FIGURE 5. Labile soil organic carbon (mg kg−1) as influenced by residue types, nutrients, and soil moisture after 57 and 87 days of incubation. Details of labile soil organic carbon statistics are provided in Tables 3, 6.
Relationship Between Soil N2O Emission and Related Soil and Crop Properties
The cumulative N2O emission was significantly (p < 0.01) and positively correlated with soil as well as crop residue characteristics. Among the soil properties, significant (p < 0.01) and positive correlations were observed with NO3-N, NO2-N, NH4-N, DHA, labile SOC, soil CO2 emission, and soil moisture content. Among the crop residue characteristics, residue total nitrogen (TN) was positively correlated and C:N ratio of crop residue was negatively correlated with the N2O flux (Table 7). The quantification of the individual effects of the selected soil and crop residue characteristics on N2O emissions was carried out by stepwise multiple regression analysis. Eq. 1 was used to describe N2O emission as a function of studied soil and crop properties. The constant and each coefficient of variables, the R2 (0.82) and adjusted R2 (0.81), were significant at p < 0.0001 in Eq. 1.
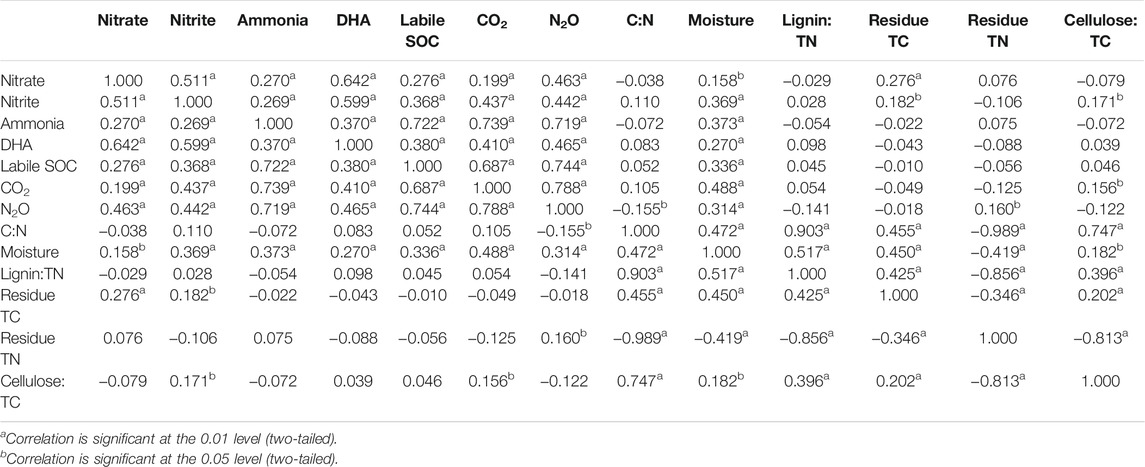
TABLE 7. Pearson’s correlation coefficient between soil N2O emission and related soil and crop properties.
The regression analysis and structural equation modeling (SEM) results showed that out of all the variables, only residue TN, soil CO2 emission, NO3-N, and labile SOC were the highly influencing factors and could explain 82% variability in the soil N2O emission in the Vertisols (overall model R2 = 0.82; p < 0.001 Figure 6 and Eq. 1).
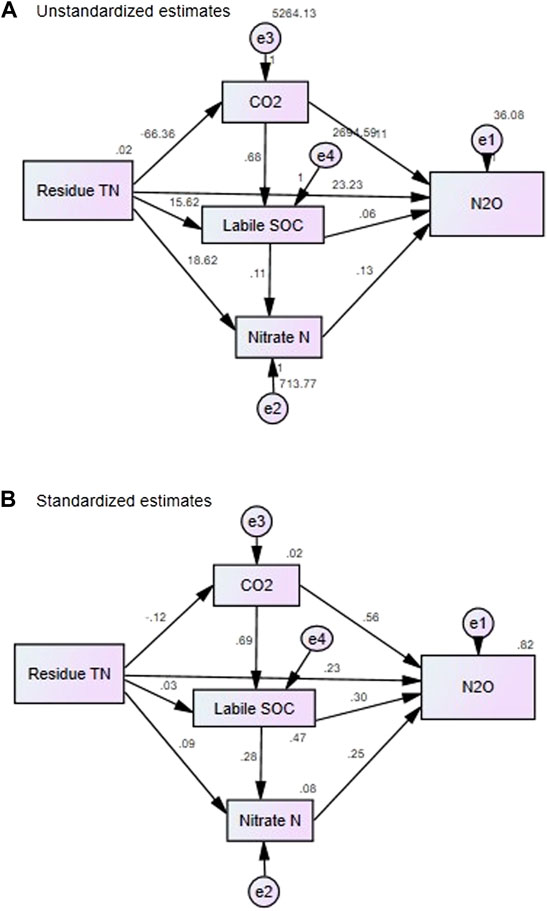
FIGURE 6. Structural equation model (SEM) of soil N2O emission based on residue and soil properties. The numbers next to lines represent (A) unstandardized path coefficients and (B) standardized path coefficients. R2 values above each rectangular box in (B) represent the proportion of the variance explaining endogenous variables. The standardized regression weights represent the amount of change in the dependent variable that is attributable to a single standard deviation unit’s worth of change in the predictor variable. The unstandardized path coefficients (A) represent the amount of change in the dependent variable given a single raw score unit change in the predictor variable. Goodness-of-fit statistics are shown underneath the modeling frame.
Discussion
Soil Nitrogen Mineralization
Returning crop residues and other organic inputs to the soil effectively sustain the soil mineral N (NH4, NO3, and NO2) and crop productivity. The concentration of mineral N is dependent on the microbial transformation of organic inputs such as decomposition and mineralization. The responses of microbial transformations to crop residue inputs are regulated by soil moisture, temperature, and the quality of crop residue (Raiesi, 2006; Muhammad et al., 2011; Lehtinen et al., 2014; Datta et al., 2019). In this experiment, the extent of N mineralization and immobilization was significantly affected by soil moisture and residue biochemical quality, as the investigation was conducted under same temperature (30 ± 1°C). The NO3-N pool decreased significantly (p < 0.05) in all residue-amended soils (cf. control soil), probably due to immobilization of available N by microbes (Kaleeem Abbasi et al., 2015; Datta et al., 2019) because of higher C:N ratio of residues (Table 1). The N immobilization in wheat, rice, and maize residue-amended soil samples was substantially greater than that observed in soybean-amended soils because former residues had a comparatively higher C:N ratio. This study demonstrated that the mineral N input (N100PK, N150PK, and integrated use of nutrients) could meet the assimilation needs of the microbes and decrease the immobilization of mineral N, as evident from higher soil NO3-N in these treatments. Organic materials with a C:N ratio less than 30 are expected to result in net N mineralization, while those with C:N ratios higher than 30 cause immobilization (Muhammad et al., 2011). Because all the crop residues added in the current experiment had C:N ratio >30:1, their addition might have resulted in net N immobilization. Furthermore, crop residue additions could have induced soil and microbial nutrient stoichiometry changes (C:N; N:P, and C:P) needed for SOC and residue C mineralization (Shen et al., 2021). Nutrient inputs might have improved the availability of nutrients to the microbial community, thereby changing the microbial nutrient stoichiometry and microbial community as the residues are decomposed (Kirkby et al., 2013, 2016; Fang et al., 2020; Shen et al., 2021). In crop residue-amended treatments, microbial N limitation was primarily affected by the supply–demand relationships between microorganisms and resources. Alternatively, integrated inorganic nutrients (N100PK) with manure characterized with a lower C:N ratio of 8:1, and crop residue return could increase the mineral N content indicating net N mineralization (Baggs et al., 2000).
However, the addition of biochar (C:N:: 45:1) with N100PK and N150PK had a mixed effect (positive and negative) on soil NO3 concentration, probably, because the soil NO3 concentration is dependent on three predominant microbial processes, viz., nitrification, denitrification, and dissimilatory nitrate reduction (Baggs et al., 2000; Toma and Hatano, 2007; Wang and Luo, 2018). Oladele et al. (2019), in a field study, reported that the NH4-N and NO3-N content increased with increasing doses of rice husk biochar and N fertilizer at the 0–10 cm soil layer, and the concentration decreased at the 10–20 cm soil layer under rainfed rice crop. The inconsistent effect of biochar on soil mineral N could probably be attributed to variance in soil moisture, residue quality, and N rates (Kanthle et al., 2016; Oladele et al., 2019; Liao et al., 2020). Soybean and maize residues maintained a significantly higher NO3-N across all nutrient management under 80% FC except only soybean residue at 60% FC probably because of high lignin and less cellulose content compared to other crop residues (Raiesi, 2006; Chen et al., 2014; Liang et al., 2017; Hu et al., 2019; Jin et al., 2020). This study revealed a negative relationship (not significant) between C:N of crop residue and NO3-N and NH4-N (Table 7), and similar responses of net N mineralization (immobilization) to C:N of crop residues were observed in previous studies (Baggs et al., 2000; Toma and Hatano, 2007; Wang et al., 2011; Lehtinen et al., 2014; Wang and Luo, 2018).
Nitrous Oxide (N2O) Fluxes
Our study showed that nutrient management and soil moisture content could change the magnitude and direction of N2O fluxes in residue-returned soils. The cumulative N2O fluxes were comparable between 80% FC and 60% FC soil moisture, despite the two levels of soil moisture contents responding differently to the treatments (Figures 2, 3). At 80% FC, the N2O emissions increased with increasing levels of N input (N0PK, N100PK, and N150PK) in control and residue-amended soils (except maize). In contrast, at 60% FC, increasing N levels had no consistent effects on cumulative N2O fluxes in control and residue-amended soil. It is generally recognized that increasing N levels can exacerbate N2O fluxes by favoring microbial growth, oxygen consumption, and denitrification through elevated labile SOC and mineral N concentrations (Ullah et al., 2016; Fang et al., 2020; Yujin Zhang et al., 2020). However, reducing the soil moisture from 80 to 60% FC decreased the soil microbial activity, as evident from significantly lower CO2 release (Figure 4), limiting denitrification and the associated N2O release (Dick et al., 2008). Previous studies have reported that denitrification is the more predominant process in N2O emission than nitrification (Klemedtsson et al., 1988; Ciarlo et al., 2007; Troy and Tang, 2011). A nonlinear relationship between increasing N levels and N2O production is consistent with previous works, which reported that inhibition of N2O reductase activity occurs at very high N concentrations because of the competitive effect of NO3-N and N2O as electron acceptors during denitrification (García-Marco et al., 2014). Increased fluxes of nitrous oxide, as well as increased CO2 emission, mineral N, labile SOC, and soil enzyme activity, were found in response to treatments with higher nutrients, moisture levels, and residue return (Letey et al., 1981; Klemedtsson et al., 1988; Ciarlo et al., 2007; Mutegi et al., 2010; Chen et al., 2019; Hongjin Zhang et al., 2020; Wu et al., 2020; Yujin Zhang et al., 2020). The correlation analysis in the study also found a significant positive relation between N2O emission and all the studied soil properties and residue total nitrogen and a significantly negative correlation with the C:N ratio of crop residue. Further integrated use of nutrients (N100PK + manure, N100PK + biochar, and N150PK + biochar) in control and residue-amended soil considerably reduced N2O emission from fertilizer N at 80% FC than 60% FC which is consistent with previous studies (Hu et al., 2019; Wu et al., 2020). That indicates biochar/manure with nutrients (N100PK or N150PK) might have influenced the N cycling enzymes, mineral N, and substrate availability and might have stimulated the reduction of nitrous oxide to dinitrogen (N2) (Troy and Tang, 2011; Sangeeta Lenka et al., 2017). Integrated use of nutrients (N100PK + manure) relatively increased the soil enzyme activity in all residue-amended soils compared with control soil. Similar positive effects of NPK addition with crop residue and manure on soil DHA are reported by previous researchers (Liang et al., 2012; Šimon and Czakó, 2014; Narendra K. Lenka et al., 2017). The application of biochar with NPK and crop residue has increased the dehydrogenase activity by 70 and 40% under 80 and 60% FC, respectively, compared with control, which could be attributed to enhanced soil CO2 respiration from biochar addition. This could be attributed to the induction of metabolically available labile-C compounds associated with the biochar that alters the CO2 fluxes and SOC mineralization by targeting the recalcitrant C of soil (Anderson et al., 2011).
An interesting result was obtained for maize residue, which had a high C:N ratio (65:1) in reducing N2O emission from fertilizer N by 1–17% in all nutrient management treatments at 60% FC soil moisture compared with 80% FC. This was supported by the significantly highest N2O consumption by maize residue (Table 5), in which NO3-N availability was reduced because of immobilization (Figure 1). Furthermore, control soil (without residue) and residue amended (wheat and rice) with the N fertilizer (N100PK and N150PK) has significantly reduced the emission of N2O from fertilizer N at 60% FC relative to 80% FC supported by relatively lower cumulative CO2 emissions and NO3-N from these treatments. In their review, Hu et al. (2019) have shown significantly lower N2O fluxes observed at the N application rate of 100–150 kg ha−1 regarding residue returning. Compared with control soil, the cumulative N2O emission after 57 days of incubation was considerably higher in residue with inorganic nutrients (N100PK and N150PK) than integrated use of nutrients (N100PK + manure, N100PK + biochar, and N150PK + biochar). Among the different crop residue-amended soil treatments, the daily and cumulative N2O emission was the lowest in wheat and maize residue-amended soils and highest in soybean and rice residue-amended soils after 57 days of incubation. The average N2O emission from wheat residue-amended soil was even lower than that from control soil. Our results are in agreement with previous studies reporting comparatively large N2O emissions after application of readily degradable crop residues with a low C:N ratio and application of manure (Toma and Hatano, 2007; Muhammad et al., 2011; Lehtinen et al., 2014; Wang and Luo, 2018). This is probably a reflection of the rapid stimulation of microbial mineralization (Lenka et al., 2019), the possible creation of anaerobic microsites resulting from microbial respiration (Muhammad et al., 2011; Shan and Yan, 2013; Lehtinen et al., 2014; Wu et al., 2020), and the increased C supply and substrate for nitrification and denitrification (Khalil et al., 2005; Lehtinen et al., 2014). Furthermore, previous studies also reported that the addition of high C:N ratio residues leads to high rates of microbial N immobilization in soil, limiting N for nitrifiers and denitrifiers (Muhammad et al., 2011; Thomson et al., 2012). Our study could further identify the key factors from the regression analysis and structural equation modeling (SEM) results, which showed that residue TN, soil CO2 emission, NO3-N, and labile SOC were the highly influencing factors and could explain 82% variability in the soil N2O emission in the Vertisols (overall model R2 = 0.82; p < 0.001 Figure 6 and Eq. 1).
The negative fluxes of N2O observed after 57 days of incubation indicate N2O consumption in all treatments. The N2O consumption observed in the study could be attributed to less substrate availability for the denitrifiers, and in such conditions, N2O serves as electron acceptors for heterotrophic denitrifiers (Cavigelli and Robertson, 2001; Chapuis-lardy et al., 2007; García-Marco et al., 2014). The significantly higher N2O consumption of control soil without residue and nutrients at 60% FC moisture than that of other treatments supports the assertion that N2O consumption could be increased at low mineral levels and soil moisture and without residue return (Chapuis-lardy et al., 2007; Pauleta et al., 2013; García-Marco et al., 2014; Yoon et al., 2019). This study further reveals that applying crop residues with integrated organic and inorganic nutrients (N100PK + manure, N100PK + biochar, and N150PK + biochar) reduced the N2O consumption (Table 6). The influence of nutrient management and crop residue return was evident at the drier soil moisture content (60% FC) than 80% FC. This result strongly indicates the effect of low mineral N, labile SOC, and enzyme activity in regulating N2O consumption because of drier soil moisture at 60% FC. Although there was no significant difference between residue types on N2O consumption, however, the cereal residues with a high C:N ratio (maize> wheat> rice) relatively had higher N2O consumption than soybean, a legume residue. Furthermore, previous studies also reported that the addition of high C:N ratio residues leads to high rates of microbial N immobilization in soil, and N2O emissions have been reported to be lowered (Muhammad et al., 2011; Thomson et al., 2012). Our results highlight the need to examine residue type, nutrient management, and soil moisture in Vertisols which are essential drivers of the GHG N2O emission and global C and N cycle.
Conclusion
Our study gives new insights into the sustainable management of different crop residues, nutrients, and soil moisture for reducing soil N2O emission by studying the underlying mechanisms of soil microbial processes (soil respiration and extracellular enzyme activity), soil properties (mineral N and labile SOC), and residue quality (TN, C:N, lignin:TN, and cellulose:TC). The study showed that soybean residue return with nutrient addition increased the N2O emission at each soil moisture level. In contrast, N2O consumption was higher in maize and wheat residue-amended soils. The best treatment for mitigation of N2O emission was N100PK and N150PK at 80% field capacity in wheat residue-amended soil, under which approximately 20% lower net emission was observed (cf. control soil without residue). In terms of the absolute value, N2O consumption was significantly higher at 60% FC than 80% FC, and the reverse was true for N2O emission. Our results demonstrated that nutrient addition (P and K) is essential to reduce the emissions of N2O from fertilizer N application in residue-amended soils. Residue addition under N0P0K0 and N0PK treatments increased the N2O emissions compared with control soil under both moisture content levels. The comparative reduction in the residue-returned soil as compared to residue-control soil was higher at 80% FC and in the nutrient addition treatments over the corresponding treatments at 60% FC. The regression analysis and structural equation modeling (SEM) results showed that residue TN, soil CO2 emission, NO3-N, and labile SOC were the highly influencing factors and could explain 82% variability in the soil N2O emission in the Vertisols of Central India. The results from incubation studies might not exactly match the results from field studies because of field variability, but they provide strong indication about the likely impact of the input variables. Thus, the underlying mechanisms regulating N2O emission under different levels of nutrient, soil moisture, and crop residue addition needs further investigation and validation in field experiments. Moreover, the resulting information can be integrated into a mechanistic model for predicting the magnitude of N2O emission and soil N storage through integrated residue and nutrient management practices in the selected agroecosystem.
Data Availability Statement
The original contributions presented in the study are included in the article/Supplementary Material, further inquiries can be directed to the corresponding authors.
Author Contributions
SL: conceptualization, data curation, formal analysis, funding acquisition, investigation, methodology, project administration, resources, software, supervision, visualization, writing–original draft, and writing–review and editing. RC: data curation, formal analysis, investigation, methodology, software, supervision, visualization, and writing–original draft. NL: formal analysis, funding acquisition, investigation, methodology, project administration, resources, software, supervision, validation, visualization, and writing–review and editing. JS: investigation, methodology, project administration, resources, supervision, validation, visualization, and writing–review and editing. DA: data curation, formal analysis, methodology, software, supervision, validation, visualization, and writing–original draft. AP: conceptualization, project administration, resources, supervision, validation, visualization, and writing–review and editing. VG: formal analysis, investigation, methodology, software, supervision, and validation. DS: formal analysis, investigation, methodology, software, and validation.
Funding
The senior author acknowledges the research grant provided by the National Agricultural Science Fund of the Indian Council of Agricultural Research, New Delhi (Grant No. NASF/CA-7019/2018-19), and Science and Engineering Research Board, POWER Fellowship (Grant No. SPF/2020/000022).
Conflict of Interest
The authors declare that the research was conducted in the absence of any commercial or financial relationships that could be construed as a potential conflict of interest.
Publisher’s Note
All claims expressed in this article are solely those of the authors and do not necessarily represent those of their affiliated organizations, or those of the publisher, the editors, and the reviewers. Any product that may be evaluated in this article, or claim that may be made by its manufacturer, is not guaranteed or endorsed by the publisher.
Acknowledgments
We also thank reviewers and the editor for their valuable and constructive comments.
Supplementary Material
The Supplementary Material for this article can be found online at: https://www.frontiersin.org/articles/10.3389/fenvs.2022.857233/full#supplementary-material
References
Al-Kaisi, M. M., and Yin, X. (2005). Tillage and Crop Residue Effects on Soil Carbon and Carbon Dioxide Emission in Corn-Soybean Rotations. J. Environ. Qual. 34, 437–445. doi:10.2134/jeq2005.0437
Anderson, C. R., Condron, L. M., Clough, T. J., Fiers, M., Stewart, A., Hill, R. A., et al. (2011). Biochar Induced Soil Microbial Community Change: Implications for Biogeochemical Cycling of Carbon, Nitrogen and Phosphorus. Pedobiologia 54, 309–320. doi:10.1016/j.pedobi.2011.07.005
Baggs, E. M., Rees, R. M., Smith, K. A., and Vinten, A. J. A. (2000). Nitrous Oxide Emissions from Soils after Incorporating Crop Residues. Soil Use Manag. 16, 82–87. doi:10.1002/9781118676332.ch8
Balota, E. L., Kanashiro, M., Colozzi, A., Andrade, D. S., and Dick, R. P. (2004). Soil Enzyme Activities Under Long-term Tillage and Crop Rotation Systems in Subtropical Agro-eco Systems. Braz. J. Microbiol. 35, 300–306.
Blair, G., Lefroy, R., and Lisle, L. (1995). Soil Carbon Fractions Based on Their Degree of Oxidation, and the Development of a Carbon Management index for Agricultural Systems. Aust. J. Agric. Res. 46, 1459–1466. doi:10.1071/AR9951459
Cavigelli, M. A., and Robertson, G. P. (2001). Role of Denitrifier Diversity in Rates of Nitrous Oxide Consumption in a Terrestrial Ecosystem. Soil Biol. Biochem. 33, 297–310. doi:10.1016/S0038-0717(00)00141-3
Chapuis-lardy, L., Wrage, N., Metay, A., Chotte, J.-L., and Bernoux, M. (2007). Soils, a Sink for N2O? A Review. Glob. Change Biol. 13, 1–17. doi:10.1111/j.1365-2486.2006.01280.x
Charles, A., Rochette, P., Whalen, J. K., Angers, D. A., Chantigny, M. H., and Bertrand, N. (2017). Global Nitrous Oxide Emission Factors from Agricultural Soils after Addition of Organic Amendments: A Meta-Analysis. Agric. Ecosyst. Environ. 236, 88–98. doi:10.1016/j.agee.2016.11.021
Chen, H., Li, X., Hu, F., and Shi, W. (2013). Soil Nitrous Oxide Emissions Following Crop Residue Addition: A Meta-Analysis. Glob. Change Biol. 19, 2956–2964. doi:10.1111/gcb.12274
Chen, X., Wang, X., Liebman, M., Cavigelli, M., and Wander, M. (2014). Influence of Residue and Nitrogen Fertilizer Additions on Carbon Mineralization in Soils with Different Texture and Cropping Histories. PLoS One 9, e103720. doi:10.1371/journal.pone.0103720
Chen, Y., Wang, Q., Zhao, S., Yang, W., Wang, H., and Jia, W. (2019). Removal of Nutrients and Emission of Nitrous Oxide during Simultaneous Nitrification, Denitrification and Phosphorus Removal Process with Metal Ions Addition. Int. Biodeterior. Biodegr. 142, 143–150. doi:10.1016/j.ibiod.2019.05.016
Chen, Z., Tu, X., Meng, H., Chen, C., Chen, Y., Elrys, A. S., et al. (2021). Microbial Process-Oriented Understanding of Stimulation of Soil N2O Emission Following the Input of Organic Materials. Environ. Pollut. 284, 117176. doi:10.1016/j.envpol.2021.117176
Ciarlo, E., Conti, M., Bartoloni, N., and Rubio, G. (2007). The Effect of Moisture on Nitrous Oxide Emissions from Soil and the N2O/(N2O+N2) Ratio under Laboratory Conditions. Biol. Fertil. Soils 43, 675–681. doi:10.1007/s00374-006-0147-9
Datta, A., Jat, H. S., Yadav, A. K., Choudhary, M., Sharma, P. C., Rai, M., et al. (2019). Carbon Mineralization in Soil as Influenced by Crop Residue Type and Placement in an Alfisols of Northwest India. Carbon Manage. 10, 37–50. doi:10.1080/17583004.2018.1544830
Dick, J., Kaya, B., Soutoura, M., Skiba, U., Smith, R., Niang, A., et al. (2008). The Contribution of Agricultural Practices to Nitrous Oxide Emissions in Semi-arid Mali. Soil Use Manag. 24, 292–301. doi:10.1111/j.1475-2743.2008.00163.x
Fang, Y., Nazaries, L., Singh, B. K., and Singh, B. P. (2018a). Microbial Mechanisms of Carbon Priming Effects Revealed during the Interaction of Crop Residue and Nutrient Inputs in Contrasting Soils. Glob. Change Biol. 24, 2775–2790. doi:10.1111/gcb.14154
Fang, Y., Singh, B. P., Collins, D., Li, B., Zhu, J., and Tavakkoli, E. (2018b). Nutrient Supply Enhanced Wheat Residue-Carbon Mineralization, Microbial Growth, and Microbial Carbon-Use Efficiency when Residues Were Supplied at High Rate in Contrasting Soils. Soil Biol. Biochem. 126, 168–178. doi:10.1016/j.soilbio.2018.09.003
Fang, Y., Singh, B. P., Collins, D., Armstrong, R., Van Zwieten, L., and Tavakkoli, E. (2020). Nutrient Stoichiometry and Labile Carbon Content of Organic Amendments Control Microbial Biomass and Carbon-Use Efficiency in a Poorly Structured Sodic-Subsoil. Biol. Fertil. Soils 56, 219–233. doi:10.1007/s00374-019-01413-3
Feng, K., Yan, F., Hütsch, B. W., and Schubert, S. (2003). Nitrous Oxide Emission as Affected by Liming an Acidic mineral Soil Used for Arable Agriculture. Nutrient Cycl. Agroecosyst. 67, 283–292. doi:10.1023/B:FRES.0000003664.51048.0e
Finn, D., Page, K., Catton, K., Kienzle, M., Robertson, F., Armstrong, R., et al. (2016). Ecological Stoichiometry Controls the Transformation and Retention of Plant-Derived Organic Matter to Humus in Response to Nitrogen Fertilisation. Soil Biol. Biochem. 99, 117–127. doi:10.1016/j.soilbio.2016.05.006
Gao, J., Xie, Y., Jin, H., Liu, Y., Bai, X., Ma, D., et al. (2016). Nitrous Oxide Emission and Denitrifier Abundance in Two Agricultural Soils Amended with Crop Residues and Urea in the North China Plain. PLoS One 11, e0154773–15. doi:10.1371/journal.pone.0154773
García-Marco, S., Ravella, S. R., Chadwick, D., Vallejo, A., Gregory, A. S., and Cárdenas, L. M. (2014). Ranking Factors Affecting Emissions of GHG from Incubated Agricultural Soils. Eur. J. Soil Sci. 65, 573–583. doi:10.1111/ejss.12143
Giles, M., Morley, N., Baggs, E. M., and Daniell, T. J. (2012). Soil Nitrate Reducing Processes - Drivers, Mechanisms for Spatial Variation, and Significance for Nitrous Oxide Production. Front. Microbio. 3, 1–16. doi:10.3389/fmicb.2012.00407
Zhang, H., Yao, X., Zeng, W., Fang, Y., and Wang, W. (2020). Depth Dependence of Temperature Sensitivity of Soil Carbon Dioxide, Nitrous Oxide and Methane Emissions. Soil Biol. Biochem. 149, 107956. doi:10.1016/j.soilbio.2020.107956
Hu, Z., Cui, H., Chen, S., Shen, S., Li, H., Yang, Y., et al. (2013). Soil Respiration and N2O Flux Response to UV-B Radiation and Straw Incorporation in a Soybean-winter Wheat Rotation System. Water Air Soil Pollut. 224, 1394. doi:10.1007/s11270-012-1394-z
Hu, N., Chen, Q., and Zhu, L. (2019). The Responses of Soil N2O Emissions to Residue Returning Systems: A Meta-Analysis. Sustainability 11, 748. doi:10.3390/su11030748
Islam, K. R., Stine, M. A., Gruver, J. B., Samson-Liebig, S. E., and Weil, R. R. (2003). Estimating Active Carbon for Soil Quality Assessment: A Simplified Method for Laboratory and Field Use. Am. J. Altern. Agric. 18, 3–17. doi:10.1079/AJAA2003003
Jackson, R. C., Weber, G., and Morris, H. P. (1975). IMP Dehydrogenase, an Enzyme Linked with Proliferation and Malignancy. Nature 256, 331–333. doi:10.1038/256331a0
Jianwen, Z., Yao, H., Lianggang, Z., Xunhua, Z., and Yuesi, W. (2004). Carbon Dioxide, Methane, and Nitrous Oxide Emissions from a rice-wheat Rotation as Affected by Crop Residue Incorporation and Temperature. Adv. Atmos. Sci. 21, 691–698. doi:10.1007/bf02916366
Jin, Z., Shah, T., Zhang, L., Liu, H., Peng, S., and Nie, L. (2020). Effect of Straw Returning on Soil Organic Carbon in rice-wheat Rotation System: A Review. Food Energy Secur. 9, 1–13. doi:10.1002/fes3.200
Kaleeem Abbasi, M., Mahmood Tahir, M., Sabir, N., and Khurshid, M. (2015). Impact of the Addition of Different Plant Residues on Nitrogen Mineralization-Immobilization Turnover and Carbon Content of a Soil Incubated under Laboratory Conditions. Solid Earth 6, 197–205. doi:10.5194/se-6-197-2015
Kanthle, A. K., Lenka, N. K., Lenka, S., and Tedia, K. (2016). Biochar Impact on Nitrate Leaching as Influenced by Native Soil Organic Carbon in an Inceptisol of central India. Soil Tillage Res. 157, 65–72. doi:10.1016/j.still.2015.11.009
Kempers, A. J. (1974). Determination of Sub-microquantities of Ammonium and Nitrates in Soils with Phenol, Sodiumnitroprusside and Hypochlorite. Geoderma 12, 201–206. doi:10.1016/0016-7061(74)90068-8
Khalil, M. I., Hossain, M. B., and Schmidhalter, U. (2005). Carbon and Nitrogen Mineralization in Different upland Soils of the Subtropics Treated with Organic Materials. Soil Biol. Biochem. 37, 1507–1518. doi:10.1016/j.soilbio.2005.01.014
Kirkby, C. A., Richardson, A. E., Wade, L. J., Batten, G. D., Blanchard, C., and Kirkegaard, J. A. (2013). Carbon-nutrient Stoichiometry to Increase Soil Carbon Sequestration. Soil Biol. Biochem. 60, 77–86. doi:10.1016/j.soilbio.2013.01.011
Kirkby, C. A., Richardson, A. E., Wade, L. J., Conyers, M., and Kirkegaard, J. A. (2016). Inorganic Nutrients Increase Humification Efficiency and C-Sequestration in an Annually Cropped Soil. PLoS One 11, e0153698–17. doi:10.1371/journal.pone.0153698
Klein, D. A., Loh, T. C., and Goulding, R. L. (1971). A Rapid Procedure to Evaluate the Dehydrogenase Activity of Soils Low in Organic Matter. Soil Biol. Biochem. 3, 385–387. doi:10.1016/0038-0717(71)90049-6
Klemedtsson, L., Svensson, B. H., and Rosswall, T. (1988). Relationships between Soil Moisture Content and Nitrous Oxide Production during Nitrification and Denitrification. Biol. Fert Soils 6, 106–111. doi:10.1007/BF00257658
Kumar, M., Kundu, D. K., Ghorai, A. K., Mitra, S., and Singh, S. R. (2018). Carbon and Nitrogen Mineralization Kinetics as Influenced by Diversified Cropping Systems and Residue Incorporation in Inceptisols of Eastern Indo-Gangetic Plain. Soil Tillage Res. 178, 108–117. doi:10.1016/j.still.2017.12.025
Lan, Z. M., Chen, C. R., Rashti, M. R., Yang, H., and Zhang, D. K. (2017). Stoichiometric Ratio of Dissolved Organic Carbon to Nitrate Regulates Nitrous Oxide Emission from the Biochar-Amended Soils. Sci. Total Environ. 576, 559–571. doi:10.1016/j.scitotenv.2016.10.119
Lehtinen, T., Schlatter, N., Baumgarten, A., Bechini, L., Krüger, J., Grignani, C., et al. (2014). Effect of Crop Residue Incorporation on Soil Organic Carbon and Greenhouse Gas Emissions in European Agricultural Soils. Soil Use Manage 30, 524–538. doi:10.1111/sum.12151
Lenka, S., Trivedi, P., Singh, B., Singh, B. P., Pendall, E., Bass, A., et al. (2019). Effect of Crop Residue Addition on Soil Organic Carbon Priming as Influenced by Temperature and Soil Properties. Geoderma 347, 70–79. doi:10.1016/j.geoderma.2019.03.039
Lenka, S., Lenka, N. K., and Pathak, H. (2020a). “Reducing Emission of Greenhouse Gases from Fertilizer Use in India,” in Soil and Fertilizers. Editor R. Lal (Taylor & Francis, CRC Press.), 357. doi:10.1201/9780429471049-7
Lenka, S., Malviya, S. K., Malviya, S. K., Lenka, N. K., Sahoo, S., Bhattacharjya, S., et al. (2020b). Manure Addition Influences the Effect of Tillage on Soil Aggregation and Aggregate Associated Carbon in a Vertisol of Central India. J. Environ. Biol. 41, 1585–1593. doi:10.22438/jeb/41/6/SI-221
Letey, J., Valoras, N., Focht, D. D., and Ryden, J. C. (1981). Nitrous Oxide Production and Reduction during Denitrification as Affected by Redox Potential. Soil Sci. Soc. Am. J. 45, 727–730. doi:10.2136/sssaj1981.03615995004500040010x
Li, L.-J., Han, X.-Z., You, M.-Y., Yuan, Y.-R., Ding, X.-L., and Qiao, Y.-F. (2013). Carbon and Nitrogen Mineralization Patterns of Two Contrasting Crop Residues in a Mollisol: Effects of Residue Type and Placement in Soils. Eur. J. Soil Biol. 54, 1–6. doi:10.1016/j.ejsobi.2012.11.002
Li, X. G., Jia, B., Lv, J., Ma, Q., Kuzyakov, Y., and Li, F.-m. (2017). Nitrogen Fertilization Decreases the Decomposition of Soil Organic Matter and Plant Residues in Planted Soils. Soil Biol. Biochem. 112, 47–55. doi:10.1016/j.soilbio.2017.04.018
Li, Z., Reichel, R., and Brüggemann, N. (2021). Effect of C:N:P Stoichiometry on Soil Nitrous Oxide Emission and Nitrogen Retention. J. Plant Nutr. Soil Sci. 184, 520–529. doi:10.1002/jpln.202000416
Liang, Q., Chen, H., Gong, Y., Fan, M., Yang, H., Lal, R., et al. (2012). Effects of 15 Years of Manure and Inorganic Fertilizers on Soil Organic Carbon Fractions in a Wheat-maize System in the North China Plain. Nutr. Cycl Agroecosyst. 92, 21–33. doi:10.1007/s10705-011-9469-6
Liang, X., Yuan, J., Yang, E., and Meng, J. (2017). Responses of Soil Organic Carbon Decomposition and Microbial Community to the Addition of Plant Residues with Different C:N Ratio. Eur. J. Soil Biol. 82, 50–55. doi:10.1016/j.ejsobi.2017.08.005
Liao, J., Liu, X., Hu, A., Song, H., Chen, X., and Zhang, Z. (2020). Effects of Biochar-Based Controlled Release Nitrogen Fertilizer on Nitrogen-Use Efficiency of Oilseed Rape (Brassica Napus L.). Sci. Rep. 10, 1–14. doi:10.1038/s41598-020-67528-y
Liyanage, A., Grace, P. R., Scheer, C., de Rosa, D., Ranwala, S., and Rowlings, D. W. (2020). Carbon Limits Non-linear Response of Nitrous Oxide (N2O) to Increasing N Inputs in a Highly-Weathered Tropical Soil in Sri Lanka. Agric. Ecosyst. Environ. 292, 106808. doi:10.1016/j.agee.2019.106808
Mehnaz, K. R., Corneo, P. E., Keitel, C., and Dijkstra, F. A. (2019). Carbon and Phosphorus Addition Effects on Microbial Carbon Use Efficiency, Soil Organic Matter Priming, Gross Nitrogen Mineralization and Nitrous Oxide Emission from Soil. Soil Biol. Biochem. 134, 175–186. doi:10.1016/j.soilbio.2019.04.003
Muhammad, W., Vaughan, S. M., Dalal, R. C., and Menzies, N. W. (2011). Crop Residues and Fertilizer Nitrogen Influence Residue Decomposition and Nitrous Oxide Emission from a Vertisol. Biol. Fertil. Soils 47, 15–23. doi:10.1007/s00374-010-0497-1
Mutegi, J. K., Munkholm, L. J., Petersen, B. M., Hansen, E. M., and Petersen, S. O. (2010). Nitrous Oxide Emissions and Controls as Influenced by Tillage and Crop Residue Management Strategy. Soil Biol. Biochem. 42, 1701–1711. doi:10.1016/j.soilbio.2010.06.004
Lenka, N. K., Jaiswal, S. P., Thakur, J. K., Lenka, S., Mandal, A., Dwivedi, A. K., et al. (2017). Soil Degradation Effect on Soil Productivity, Carbon Pools and Soil Enzyme Activity. Curr. Sci. 112, 2434. doi:10.18520/cs/v112/i12/2434-2439
Nath, C. P., Kumar, N., Das, K., Hazra, K. K., Praharaj, C. S., and Singh, N. P. (2021). Impact of Variable Tillage Based Residue Management and Legume Based Cropping for Seven Years on Enzymes Activity, Soil Quality index and Crop Productivity in rice Ecology. Environ. Sustai. Indic. 10, 100107. doi:10.1016/j.indic.2021.100107
Oladele, S. O., Adeyemo, A. J., and Awodun, M. A. (2019). Influence of rice Husk Biochar and Inorganic Fertilizer on Soil Nutrients Availability and Rain-Fed rice Yield in Two Contrasting Soils. Geoderma 336, 1–11. doi:10.1016/j.geoderma.2018.08.025
Pauleta, S. R., Dell’Acqua, S., and Moura, I. (2013). Nitrous Oxide Reductase. Coord. Chem. Rev. 257, 332–349. doi:10.1016/j.ccr.2012.05.026
Ponce-Mendoza, A., Ceballos-Ramírez, J. M., Gutierrez-Micelli, F., and Dendooven, L. (2010). Emission of Nitrous Oxide and Carbon Dioxide from Semi-arid Tropical Soils in Chiapas México. Rev. Bras. Ciênc. Solo. 34, 1617–1628. doi:10.1590/s0100-06832010000500015
Rahn, C. R., Bending, G. D., Turner, M. K., and Lillywhite, R. D. (2003). Management of N Mineralization from Crop Residues of High N Content Using Amendment Materials of Varying Quality. Soil Use Manage. 19, 193–200. doi:10.1111/j.1475-2743.2003.tb00304.x
Raiesi, F. (2006). Carbon and N Mineralization as Affected by Soil Cultivation and Crop Residue in a Calcareous Wetland Ecosystem in Central Iran. Agric. Ecosyst. Environ. 112, 13–20. doi:10.1016/j.agee.2005.07.002
Raul, C., Bharti, V. S., Dar Jaffer, Y., Lenka, S., and Krishna, G. (2020). Sugarcane Bagasse Biochar: Suitable Amendment for Inland Aquaculture Soils. Aquac. Res. 52, 643–654. doi:10.1111/are.14922
Rowland, A. P., and Roberts, J. D. (1994). Lignin and Cellulose Fractionation in Decomposition Studies Using Acid‐detergent Fibre Methods. Commun. Soil Sci. Plant Anal. 25, 269–277. doi:10.1080/00103629409369035
Lenka, S., Lenka, N. K., Singh, A. B., Singh, B., and Raghuwanshi, J. (2017). Global Warming Potential and Greenhouse Gas Emission under Different Soil Nutrient Management Practices in Soybean-Wheat System of central India. Environ. Sci. Pollut. Res. 24, 4603–4612. doi:10.1007/s11356-016-8189-5
Shan, J., and Yan, X. (2013). Effects of Crop Residue Returning on Nitrous Oxide Emissions in Agricultural Soils. Atmos. Environ. 71, 170–175. doi:10.1016/j.atmosenv.2013.02.009
Shen, H., Zhang, Q., Zhu, S., Duan, P., Zhang, X., Wu, Z., et al. (2021). Organic Substitutions Aggravated Microbial Nitrogen Limitation and Decreased Nitrogen-Cycling Gene Abundances in a Three-Year Greenhouse Vegetable Field. J. Environ. Manage. 288, 112379. doi:10.1016/j.jenvman.2021.112379
Šimon, T., and Czakó, A. (2014). Influence of Long-Term Application of Organic and Inorganic Fertilizers on Soil Properties. Plant Soil Environ. 60, 314–319. doi:10.17221/264/2014-pse
Singh, R. C., Lenka, S., and Singh, C. D. (2014). Conservation Tillage and Manure Effect on Soil Aggregation, Yield and Energy Requirement for Wheat (Triticum aestivum) in Vertisols. Indian J. Agric. Sci. 84, 267–271.
Singh, D., Lenka, S., Lenka, N. K., Trivedi, S. K., Bhattacharjya, S., Sahoo, S., et al. (2020). Effect of Reversal of Conservation Tillage on Soil Nutrient Availability and Crop Nutrient Uptake in Soybean in the Vertisols of Central India. Sustainability 12, 6608. doi:10.3390/su12166608
Soong, J. L., Marañon-Jimenez, S., Cotrufo, M. F., Boeckx, P., Bodé, S., Guenet, B., et al. (2018). Soil Microbial CNP and Respiration Responses to Organic Matter and Nutrient Additions: Evidence from a Tropical Soil Incubation. Soil Biol. Biochem. 122, 141–149. doi:10.1016/j.soilbio.2018.04.011
Takakai, F., Hirano, S., Harakawa, Y., Hatakeyama, K., Yasuda, K., Sato, T., et al. (2018). Fate of Fertilizer-Derived N Applied to Enhance rice Straw Decomposition in a Paddy Field during the Fallow Season under Cool Temperature Conditions. Agriculture 8, 50. doi:10.3390/agriculture8040050
Tao, R., Wakelin, S. A., Liang, Y., Hu, B., and Chu, G. (2018). Nitrous Oxide Emission and Denitrifier Communities in Drip-Irrigated Calcareous Soil as Affected by Chemical and Organic Fertilizers. Sci. Total Environ. 612, 739–749. doi:10.1016/j.scitotenv.2017.08.258
Thomson, A. J., Giannopoulos, G., Pretty, J., Baggs, E. M., and Richardson, D. J. (2012). Biological Sources and Sinks of Nitrous Oxide and Strategies to Mitigate Emissions. Phil. Trans. R. Soc. B 367, 1157–1168. doi:10.1098/rstb.2011.0415
Toma, Y., and Hatano, R. (2007). Effect of Crop Residue C:N Ratio on N2O Emissions from Gray Lowland Soil in Mikasa, Hokkaido, Japan. Soil Sci. Plant Nutr. 53, 198–205. doi:10.1111/j.1747-0765.2007.00125.x
Troy, C., and Tang, J. (2011). Effects of Temperature and Moisture Stress on Nitrous Oxide Production in Agricultural Soil, 1–21. Available at: https://www.mbl.edu/ses/files/2014/09/Troy-Cynthia.pdf (Accessed 12 August 2021).
Ullah, B., Shaaban, M., Hugui, R.-g., Zhao, J.-s., and Lin, S. (2016). Assessing Soil Nitrous Oxide Emission as Affected by Phosphorus and Nitrogen Addition under Two Moisture Levels. J. Integr. Agric. 15, 2865–2872. doi:10.1016/S2095-3119(16)61353-9
Velthof, G. L., Kuikman, P. J., and Oenema, O. (2002). Nitrous Oxide Emission from Soils Amended with Crop Residues. Nutr. Cycl. Agroecosyst. 62, 249–261. doi:10.1023/A:1021259107244
Venterea, R. T., Halvorson, A. D., Kitchen, N., Liebig, M. A., Cavigelli, M. A., Grosso, S. J. D., et al. (2012). Challenges and Opportunities for Mitigating Nitrous Oxide Emissions from Fertilized Cropping Systems. Front. Ecol. Environ. 10, 562–570. doi:10.1890/120062
Wang, X.-g., and Luo, Y. (2018). Crop Residue Incorporation and Nitrogen Fertilizer Effects on Greenhouse Gas Emissions from a Subtropical rice System in Southwest China. J. Mt. Sci. 15, 1972–1986. doi:10.1007/s11629-017-4810-4
Wang, J. Y., Jia, J. X., Xiong, Z. Q., Khalil, M. A. K., and Xing, G. X. (2011). Water Regime-Nitrogen Fertilizer-Straw Incorporation Interaction: Field Study on Nitrous Oxide Emissions from a rice Agroecosystem in Nanjing, China. Agric. Ecosyst. Environ. 141, 437–446. doi:10.1016/j.agee.2011.04.009
Wang, C., Amon, B., Schulz, K., and Mehdi, B. (2021). Factors that Influence Nitrous Oxide Emissions from Agricultural Soils as Well as Their Representation in Simulation Models: A Review. Agronomy 11, 770. doi:10.3390/agronomy11040770
Wei, T., Zhang, P., Wang, K., Ding, R., Yang, B., Nie, J., et al. (2015). Effects of Wheat Straw Incorporation on the Availability of Soil Nutrients and Enzyme Activities in Semiarid Areas. PLoS One 10, e0120994–15. doi:10.1371/journal.pone.0120994
Wu, L., Hu, R., Tang, S., Shaaban, M., Zhang, W., Shen, H., et al. (2020). Nitrous Oxide Emissions in Response to Straw Incorporation Is Regulated by Historical Fertilization. Environ. Pollut. 266, 115292. doi:10.1016/j.envpol.2020.115292
Xu, H., Cai, A., Wu, D., Liang, G., Xiao, J., Xu, M., et al. (2021). Effects of Biochar Application on Crop Productivity, Soil Carbon Sequestration, and Global Warming Potential Controlled by Biochar C:N Ratio and Soil pH: A Global Meta-Analysis. Soil Tillage Res. 213, 105125. doi:10.1016/j.still.2021.105125
Yoon, S., Song, B., Phillips, R. L., Chang, J., and Song, M. J. (2019). Ecological and Physiological Implications of Nitrogen Oxide Reduction Pathways on Greenhouse Gas Emissions in Agroecosystems. FEMS Microbiol. Ecol. 95, 1–15. doi:10.1093/femsec/fiz066
Zhang, Y., Ma, M., Fang, H., Qin, D., Cheng, S., and Yuan, W. (2020). Impacts of Nitrogen Addition on Nitrous Oxide Emission: Comparison of Five Nitrous Oxide Modules or Algorithms. Ecol. Model. 421, 108963. doi:10.1016/j.ecolmodel.2020.108963
Zang, H., Wang, J., and Kuzyakov, Y. (2016). N Fertilization Decreases Soil Organic Matter Decomposition in the Rhizosphere. Appl. Soil Ecol. 108, 47–53. doi:10.1016/j.apsoil.2016.07.021
Zhang, T. a., Chen, H. Y. H., and Ruan, H. (2018). Global Negative Effects of Nitrogen Deposition on Soil Microbes. ISME J. 12, 1817–1825. doi:10.1038/s41396-018-0096-y
Keywords: greenhouse gas, residue quality, C:N ratio, climate change, soil respiration, CO2 flux, global warming potential
Citation: Lenka S, Choudhary R, Lenka NK, Saha JK, Amat D, Patra AK, Gami V and Singh D (2022) Nutrient Management Drives the Direction and Magnitude of Nitrous Oxide Flux in Crop Residue-Returned Soil Under Different Soil Moisture. Front. Environ. Sci. 10:857233. doi: 10.3389/fenvs.2022.857233
Received: 18 January 2022; Accepted: 28 February 2022;
Published: 22 March 2022.
Edited by:
Lourdes Morillas, University of Lisbon, PortugalReviewed by:
Curtis Dell, United States Department of Agriculture, United StatesSegun Oladele, Adekunle Ajasin University, Nigeria
Copyright © 2022 Lenka, Choudhary, Lenka, Saha, Amat, Patra, Gami and Singh. This is an open-access article distributed under the terms of the Creative Commons Attribution License (CC BY). The use, distribution or reproduction in other forums is permitted, provided the original author(s) and the copyright owner(s) are credited and that the original publication in this journal is cited, in accordance with accepted academic practice. No use, distribution or reproduction is permitted which does not comply with these terms.
*Correspondence: Sangeeta Lenka, c2FuZ2VldGFfMkByZWRpZmZtYWlsLmNvbQ==; Narendra Kumar Lenka, bmtsZW5rYUByZWRpZmZtYWlsLmNvbQ==