- 1Department of Civil, Construction and Environmental Engineering, Iowa State University, Ames, IA, United States
- 2Environmental Sciences Interdepartmental Graduate Program, Iowa State University, Ames, IA, United States
- 3Department of Biology, Washington University, Saint Louis, MO, United States
- 4Microbiology Interdepartmental Graduate Program, Iowa State University, Ames, IA, United States
Pathogen resistance against common disinfectants in drinking water treatment could have serious public health implications, particularly in potable water reuse. Frequent disinfection in potable water reuse has a potential to facilitate resistance development. This study investigated resistance development in Escherichia coli against repeated monochloramine and ferrate disinfection. E. coli cultures repeatedly treated with monochloramine developed resistance after 12 + treatment rounds, whereas repeated ferrate disinfection did not lead to resistance development. Monochloramine induced cells into the viable but nonculturable (VBNC) state in initial disinfection rounds; however, repeated monochloramine treatment caused increases in culturability, which corresponded to decreases in the fraction of VBNC cells post-disinfection. The cumulative number of disinfection episodes (∼12 times) rather than treatment frequency (e.g., every 48, 96, or 144 h) played a critical role in resistance development against monochloramine. In addition to preventing resistance, ferrate effectively inactivated (>3-log10) the evolved monochloramine-stressed cultures, suggesting that the evolutionary adaptations against monochloramine were ineffective against ferrate. The lack of resistance against ferrate renders it a promising disinfection agent that deserves further assessment. This work’s findings demonstrate that repeated disinfection coupled with the type of applied disinfectant can influence bacterial resistance development. Therefore, current and future water disinfection schemes, especially within potable water reuse, need regular monitoring to evaluate the resistance profile of pathogenic bacteria.
Introduction
Advances in the drinking water treatment industry significantly reduced mortality and illnesses caused by waterborne pathogens. Specifically, disinfection has led to enhanced pathogen removal, thereby limiting the spread of waterborne diseases. However, drinking water-associated outbreaks continue to reoccur in the United States (Gerberding et al., 2008; Benedict et al., 2017). Most recent surveillance data of waterborne illnesses published by the Centers for Disease Control and Prevention (CDC) reported 66 outbreaks associated with drinking water during 2019–2020, which accounted for at least 337 illness cases (CDC, 2020). According to CDC, 45 and 30% of the outbreaks and illnesses were triggered by exposures to Legionella and Norovirus infections, respectively (CDC, 2020).
The growing global water shortages further exacerbate challenges related to drinking water treatment and disinfection. For example, although the reuse of treated wastewater effluent could offer an alternative and sustainable potable water source, it is challenged by the public’s perception of potential health risks (Pecson et al., 2017; Soller et al., 2018; Kantor et al., 2019). The application of advanced water treatment (AWT), consisting of a multi-barrier treatment system, helps minimize potential health risks and improves public acceptance (USEPA, 2018). However, despite AWT, evidence of microbial growth in water distribution systems or reservoirs has been found (Park and Hu, 2010; Kantor et al., 2019). Reduction in microbial susceptibility to disinfection could have drastic implications for direct and indirect potable water reuse (i.e., DPR and IPR). As a result, an improved understanding of factors that impact microbial susceptibility to disinfectants is critical for predicting the effectiveness of disinfection, especially for potable water reuse. Various source water physical, chemical and microbiological characteristics, such as temperature, pH, organic content or microbial load, are known to interfere with disinfection effectiveness (Winward et al., 2008; Amiri et al., 2010). However, it is unknown if conditions relevant to potable water reuse, e.g., frequent disinfection, could facilitate bacterial resistance against disinfectants. A key question is whether surviving fractions of bacterial populations with altered physiology following disinfection go on to regrow, and after frequent disinfection, ultimately, develop resistance to disinfection. Hence, there is a need to determine the effect of frequent disinfection on bacterial resistance development for improved public health assessments of potable water reuse (USEPA, 2018).
While a significant body of literature has investigated the development of bacterial resistance to antibiotics, the influence of frequent disinfection on resistance development in bacteria is not well understood (van den Bergh et al., 2016; Levin-Reisman et al., 2017). Various defense responses are triggered against chemicals in bacterial cells such as cell damage repair, neutralization of toxic constituents, restoration of cellular homeostasis, or target inactivity through reduced cell growth and metabolism (Dukan et al., 1996; Carmel-Harel and Storz, 2000; Wang et al., 2009; Zhao et al., 2016; Ayrapetyan et al., 2018; Zhang et al., 2018). Collectively, defense mechanisms alter bacterial physiological responses at the phenotypic and, often, the genetic level, which decreases the susceptibility of subsequent generations to chemical exposure. However, reports of mechanisms contributing to bacterial resistance/tolerance against disinfectants in the literature are scarce (Farkas-Himsley, 1964; Haas and Morrison, 1981; Leyval et al., 1984; Hoff and Akin, 1986; Inatsu et al., 2010; Gundlach and Winter 2014). Additionally, the majority of studies have focused on resistance development against chlorine-based disinfectants due to their widespread use, though the studies show contradictory results. Farkas-Himsley (1964) found deliberate exposure of Escherichia coli to high chlorine concentrations in buffered solutions resulted in chlorine-resistant strains. Gundlach and Winter (2014) noted an incremental increase in resistance to hypochlorous acid (3.5–9.2 mM) in E. coli following multiple exposure cycles to the disinfectant in rich growth media. The buildup of resistance was found to be driven by the constitutive expression of OxyR-regulated genes, which enhanced the cells’ oxidative stress response (Gundlach and Winter 2014). Meanwhile, other studies have shown that high resistance to hypochlorous acid (3–7 mM) in E. coli occurred as a result of decreased cyclic adenosine monophosphate (cAMP) levels and cAMP receptor protein (CRP) and, in turn, the accumulation of cellular general stress response regulator (RpoS) (Barth et al., 2009).
In contrast, Haas and Morrison (1981) showed that repeated chlorination of E. coli did not yield highly tolerant strains. However, in this study bacterial sensitivity may have been altered by the protocol’s intermediate sub-culturing step in rich growth media between each chlorine exposure (Haas and Morrison, 1981). Similarly, Inatsu et al. (2010) showed no change in resistance against sodium hypochlorite among E. coli cultures repeatedly treated with the disinfectant. Fewer studies investigated resistance development in response to other disinfectants. A study using fast-acting hydroxyl radicals as the active disinfectant showed no resistance development in E. coli following 40 exposure cycles in phosphate-buffered saline (Ikai et al., 2013). As such, differences in disinfectants’ action and cultivation protocols relevant to source water chemistry could influence mechanisms that lead to bacterial resistance/tolerance development.
In this work, the development of resistance in the model bacterium, E. coli, due to frequent (every 48 h) exposure to disinfection was investigated. E. coli is used as an indicator for fecal pollution for water quality monitoring, and was, thus, selected for this study. E. coli is used as an indicator for fecal pollution for water quality monitoring, and was, thus, selected for this study. To examine the effect of chemical-based differences between disinfectants on resistance development, two different chemical disinfectants, monochloramine and ferrate, were used. Bacterial physiological state and treatment conditions relevant to drinking water treatment and potable water reuse were also applied by cultivating E. coli populations to late-stationary phase in a nutrient-poor growth media, i.e., lake water media.
Materials and Methods
E. coli Cultivation
Lake water media was sampled from the north wetland inlet of Ada Hayden Lake in Ames, IA (29 May 2018) and used for bacterial cultivation to simulate microbial growth conditions common in source surface waters for drinking water treatment. Characteristics of the sampled and amended lake water for bacterial cultivation have been described previously (Daer et al., 2021). E. coli K-12 cultures were cultivated based on previous work (Daer et al., 2021). Briefly, E. coli K-12 cultures were cultivated for 24 h in modified Lysogeny broth (LB) media (tryptone 10 g/L, yeast extract 5 g/L, sodium chloride 5 g/L) at room temperature (23 ± 1.0 °C) under continuous shaking at 160 rpm. Cell pellets were harvested by centrifugation (14,000×g, 1 min), washed twice with sterile 10 mM sodium phosphate buffer (pH 7.0), and resuspended in amended lake water media. Cultures were grown for 24 h (160 rpm, 23 ± 1.0°C) to adapt to new growth conditions and were subsequently transferred to fresh amended lake water and regrown for 48 h to reach late stationary phase. This original population of E. coli is referred to as the ancestral culture since they represented the first generation of cells before they undergo multiple rounds of growth and disinfection.
Experimental Evolution and Disinfection
Ancestral cultures at late stationary phase were treated with either monochloramine, ferrate, or water to generate parallel monochloramine-stressed (EMS), ferrate-stressed (EFS), and control (Econtrol) cultures, respectively (Figure 1). Every 48 h of growth, the cultures were treated for 60 min and subsequently transferred to fresh amended lake water media to start a new growth round. As described in previous work (Daer et al., 2021), 2.5 mg/L as Cl2 (35 µM) of sodium hypochlorite (8.25% w/v, commercial bleach) or 22.3 mg/L as Fe (400 µM) of potassium ferrate (97% purity, Element 26, League City, TX) were used to disinfect cultures for 60 min at each round. The water matrix composition pre-disinfection had a Cl2:NH3-N molar ratio below 1.0, which contributed to the generation of monochloramine due to hypochlorite addition. Econtrol cultures were treated with an equivalent volume of sterile nanopure water for 60 min. Disinfection was quenched by transferring 2% (v/v) of cultures to fresh media of amended lake water. After 24 rounds of growth and disinfection, stressed cultures were regrown for an additional 5 rounds (48 h interval) without exposure to disinfectants. Changes in bacterial inactivation were monitored at select rounds. All experiments were conducted with at least three biological replicates.
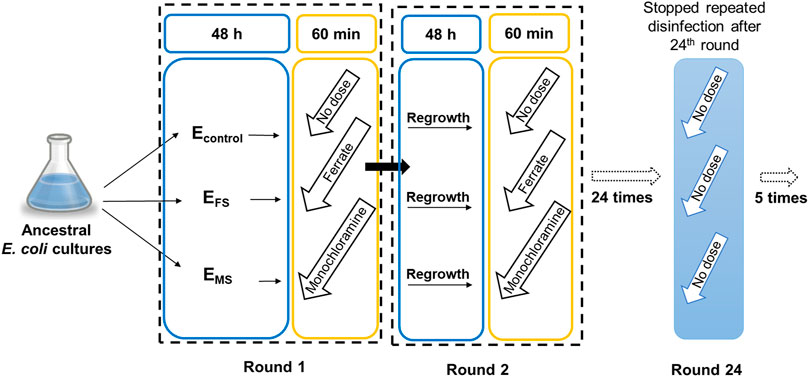
FIGURE 1. Schematic of the experimental evolution experiment carried out to determine resistance development against disinfection. Experimental evolution of EMS, EFS, and Econtrol under repeated monochloramine, ferrate and water treatment, respectively, every 48 h of bacterial growth.
Determination of E. coli Inactivation and Cellular Viability Post-disinfection
Culturable cell concentrations of EMS and EFS cultures were measured before and after disinfection to determine cellular inactivation. Econtrol cultures were divided into three separate subcultures treated with either water, ferrate, or monochloramine (Figure 1). Econtrol subcultures treated with water were transferred to the next round following 60 min, whereas the cellular inactivation of Econtrol subcultures treated with ferrate and monochloramine was determined for comparison. Culturable cell concentrations were measured using heterotrophic plate counting following standard microbiological procedures (Reasoner, 2002). Cell inactivation was calculated based on the decrease in culturable cell concentration within 60 min of disinfection:
where N0 and N represent culturable cell concentrations (CFU/ml) present initially and following disinfection, respectively.
Cellular viability based on intact membranes was assessed using LIVE/DEAD Baclight Bacterial Viability Kit (Molecular Probes, Eugene, OR). Briefly, EMS, EFS, and Econrol were treated with monochloramine, ferrate, or water for 60 min, respectively. All cultures were subsequently pelleted, re-concentrated by 5 times in 0.9% (w/v) sodium chloride solution (pH 7.0 ± 0.1), and treated with LIVE/DEAD™ BacLight bacterial viability kit as per manufacturer’s instructions (L13152, Invitrogen, Carlsbad, CA). The percentage of live cells was determined for stressed and control cultures by microscopically analyzing at least 500 cells using a Zeiss Axio Imager fluorescence microscope (Zeiss, Oberkochen, Germany). All experiments were conducted in biological triplicates.
Whole Genome Sequencing of Ancestral and Evolved Cultures
Genomic DNA of ancestral (5 isolates) as well as EMS (5 isolates), EFS (3 isolates) and Econtrol (3 isolates) cultures at the 32nd growth round was obtained and sequenced. Extraction of DNA was performed using GeneJET FFPE DNA Purification Kit as per manufacturer’s instructions (ThermoFisher Scientific, Waltham MA). Sequencing was performed on Illumina HiSeq 3,000 using single-end layout mode and with a read length of 150 bases. The quality of the reads was inspected using FASTQC (v. 0.11.7) program (Simon, 2018) Demultiplexed files were mapped against the indexed reference genome of E. coli strain 1,223 (Genbank ID CP023383.1) using BWA (v 0.7.16) (Li and Durbin, 2010). Single nucleotide polymorphism (SNP) calling was performed using FreeBayes (v 1.0.2–38) and visualized using TASSEL (v 5.0) (Bradbury et al., 2007). Sequencing data has been made publicly available (Daer and Ikuma, 2021).
Effect of cAMP Levels on E. coli Culturability Following Monochloramine Disinfection
EMS and Econtrol cultures were treated with 1 mM of adenosine 3′, 5′-cyclic monophosphoric acid (cAMP) (Fisher Scientific, Waltham, MA) prior to disinfection to test whether changes in log inactivation were linked to culturability instead of cell viability. The preparation and dosage of cAMP were adapted from previous work by (Nosho et al., 2018). The pH of each culture was adjusted using 300 mM phosphate buffer (pH 8.0) to account for the increased acidity due to cAMP addition. Cultures with and without cAMP were subsequently treated with 2.5 mg/L as Cl2 (35 µM) of monochloramine. Culturable cell concentrations were determined through heterotrophic plate counting following standard microbiological procedures (Reasoner, 2002). All experiments were conducted in biological triplicates.
Effect of Disinfection Frequency on Resistance Development
The time interval between each disinfection round was extended from 48 h to 96 and 144 h to examine the effect of monochloramine disinfection frequency on resistance development. The extension in the time interval to 96 and 144 h was selected because it was double and triple the 48 h growth interval, respectively. Cultures treated with monochloramine every 96 and 144 h are referred to as EMS-96 and EMS-144, respectively. Parallel control cultures (Econtrol-96 and Econtrol-144) were also grown and divided into two separate subcultures at specific disinfection rounds to receive either water or monochloramine. Within each round, cultures were transferred every 48 h to maintain a comparable water chemistry and physiological state (late stationary phase) as the 48 h cultures. At least 15 rounds of repeated disinfection were performed. All experiments were conducted in biological triplicates.
Statistical Analysis
Unless otherwise specified, all mean and standard errors were derived based on 3 biological replicates per treatment. Statistical significance was determined using two-tailed unpaired Student’s t-tests in Excel. Unless otherwise specified, the confidence interval in all statistical tests was set at 95% with results being considered significantly different if the measured p-value was less than 0.05.
Results
Effect of Repeated Disinfection on E. coli Inactivation and Resistance
E. coli were repeatedly disinfected with monochloramine (2.5 mg/L as Cl2) or ferrate (22.3 mg/L as Fe) to examine changes in bacterial tolerance against both disinfectants. Inactivation of EMS cultures significantly decreased from 4.0 ± 0.3-log10 to 2.0 ± 0.2-log10 within 12 monochloramine disinfection rounds (p-value = 0.01) (Figure 2A). In contrast, there was no significant change in the inactivation of EFS cultures relative to ancestral (p-value = 0.3) and Econtrol (p-value = 0.5) cultures after 21 rounds of ferrate disinfection (Figure 2B). These results indicate that repeated disinfection yielded bacterial populations that became less susceptible to monochloramine but not to ferrate.
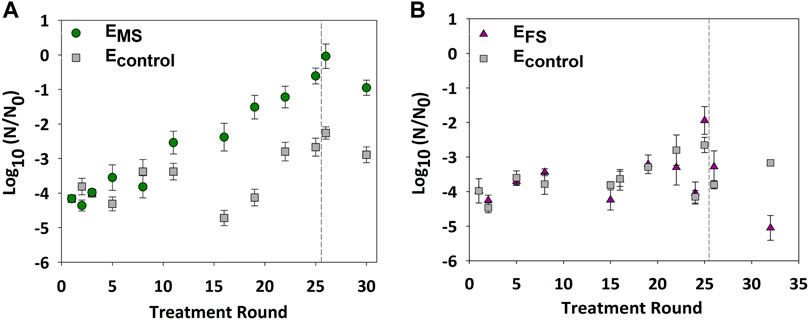
FIGURE 2. E. coli log10 inactivation after multiple rounds of growth and disinfection with either 2.5 mg/L as Cl2 of monochloramine or 11.2 mg/L as Fe of ferrate. (A) Bacterial inactivation of EMS due to repeated monochloramine disinfection. (B) Bacterial inactivation of EFS due to repeated ferrate disinfection. Econtrol cultures were not repeatedly treated; separate aliquots of the Econtrol were obtained at select rounds and treated for comparison. Rounds in which stressed cultures were regrown without exposure to disinfection are presented after the dashed line. Each data point represents an average of at least triplicate samples. Error bars represent one standard error of at least triplicate samples. Initial cell concentration (N0) ∼ 108 CFU/ml; pH = 8.4; room temperature.
A heritable and non-reversible increase in monochloramine tolerance could point to resistance development in the repeatedly treated cultures. Therefore, EMS cultures were regrown for an additional five rounds without exposure to monochloramine to examine if cultures maintained the high monochloramine tolerance in the absence of repetitive disinfection. Cell inactivation of EMS cultures in round 30 remained significantly lower than inactivation observed in ancestral (p-value = 0.00003) and Econtrol (p-value = 0.0003) cultures by at least 1-log10 (Figure 2A). The genomes of ancestral and the evolved EMS (round 32) and Econtrol strains (round 32) were sequenced and compared against the E. coli 1,223 reference genome to examine for changes in genomic mutations due to repeated disinfection. No differences between the evolved, ancestral, and the reference genome were found (data available via public repository; Daer and Ikuma, 2021).
Effect of Repeated Disinfection on Cellular Viability of E. coli
As the loss of culturability does not necessarily indicate cell death, LIVE/DEAD staining was used as a culture-independent method based on membrane intactness to assess cell viability at select disinfection rounds. A significantly higher percentage of EMS cells (60–80%) were viable (i.e., intact) compared to EFS cells (<20%) (p-value = 0.0002) post-disinfection throughout the repeated disinfection cycles (Figure 3), pointing to the formation of VBNC cells in response to monochloramine disinfection. The viability of EMS and EFS cultures did not significantly change in response to repeated monochloramine and ferrate disinfection, respectively.
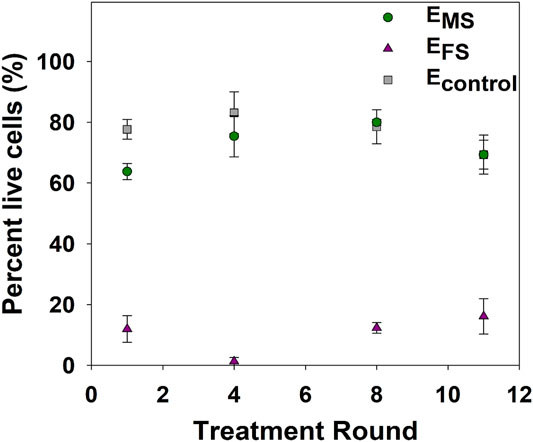
FIGURE 3. Percentage of viable cells after 60 min of treatment with 11.2 mg/L as Fe of ferrate (EFS) or 2.5 mg/L as Cl2 of monochloramine (EMS), or water (Econtrol). Each data point represents an average of at least triplicate samples. Error bars represent one standard error of at least triplicate samples.
Influence of cAMP Concentration on VBNC Induction in Evolved Cultures
The effect of exogenous cAMP addition on the culturability of evolved EMS and Econtrol cultures after 60 min of monochloramine treatment was investigated. Culturable cell concentration in EMS cultures pretreated with cAMP was lower by 1.8 ± 0.4-log10 compared to EMS cultures without cAMP pretreatment (p-value = 0.0006, Figure 4). The percentage of viable cells in EMS with and without cAMP addition was not significantly different (p-value > 0.05, Supplementary Figure S2), suggesting that the decrease in culturable cell concentrations in response to cAMP addition was not caused by losses in cell viability. Interestingly, cAMP addition did not affect culturable cell concentrations of Econtrol cultures following monochloramine treatment (Figure 4). This observation suggests that cAMP addition to controls did not appear to cause additional cells to lose culturability and become VBNC. In addition, there was no statistical difference between culturable cell concentrations in control cultures treated with or without cAMP and EMS cultures treated with cAMP (Figure 4). Therefore, the significant increase in cell culturability caused by repeated monochloramine disinfection could be reversed back to baseline levels in the presence of higher cAMP concentrations.
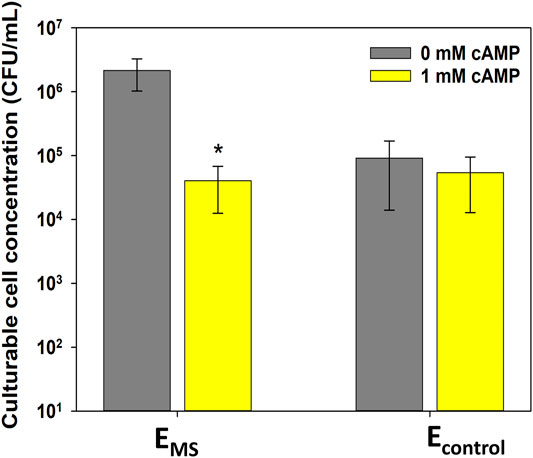
FIGURE 4. Effect of cAMP addition on culturable cell concentration of Econtrol and EMS cultures after 60 min of monochloramine treatment at the 16th disinfection round. Error bars represent one standard deviation of at least triplicate samples. Asterisks (*) indicate statistical significance (p-value < 0.05) compared to 0 mM cAMP addition.
Effect of Monochloramine Disinfection Frequency on Resistance Development
To examine if a decrease in disinfection frequency could hinder resistance development, we incrementally extended the time interval between each monochloramine disinfection round from 48 to 96 and 144 h, thus generating EMS-96 and EMS-144 cultures, respectively. Inactivation of EMS-96 cultures significantly decreased by 2-log10 compared to ancestral (p-value = 4 × 10–5) and Econtrol (p-value = 0.001) after 12 rounds of disinfection (Figure 5A). A significant drop in inactivation of EMS-144 was also observed following 12 monochloramine disinfection rounds compared to ancestral (p-value = 3 × 10–6) and Econtrol (p-value = 7 × 10–8) (Figure 5B).
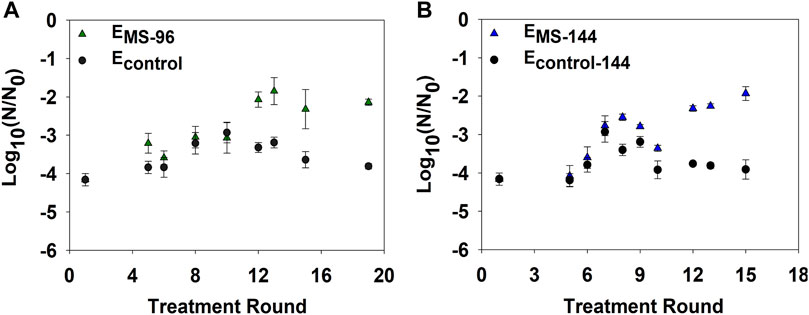
FIGURE 5. E. coli log10 inactivation after multiple rounds of growth and disinfection with 2.5 mg/L as Cl2 of monochloramine. (A) Bacterial inactivation of EMS-96 due to repeated monochloramine disinfection every 96 h. (B) Bacterial inactivation EMS-144 due to repeated monochloramine disinfection every 144 h. Econtrol-96 and Econtrol-144 were not repeatedly treated; subcultures of each were obtained and treated at select treatment rounds for comparison. Error bars represent one standard error of at least triplicate samples. Initial cell concentration (N0) ∼108 CFU/ml; pH = 8.4; room temperature.
Susceptibility of Stressed E. coli Cultures to Cross Disinfection
EMS and EFS cultures were cross-disinfected with ferrate and monochloramine, respectively, to determine if evolutionary adaptations triggered by frequent disinfection could protect cells against exposure to the other disinfectant. The susceptibility of EMS cultures to ferrate was higher by 2.96 ± 0.40-log10 compared to monochloramine (p-value = 0.0009) (Figure 6). In fact, EMS cultures were found to be more susceptible to ferrate compared to control and EFS cells (p-value < 0.05). In contrast, EFS cultures were inactivated to similar extents by ferrate and monochloramine (p-value > 0.05) (Figure 6).
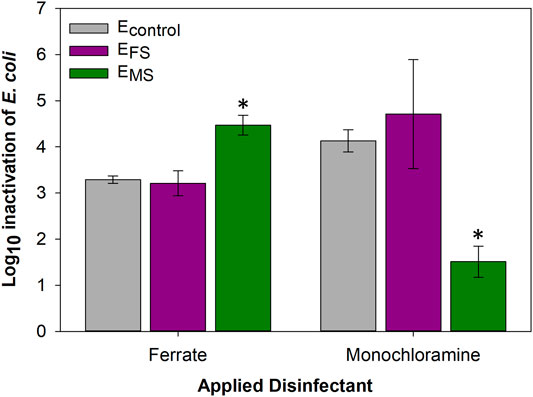
FIGURE 6. E. coli log10 inactivation of controls and evolved (EFS and EMS) cultures cross-disinfected with ferrate or monochloramine at the 18th disinfection round. Single asterisks (*) represent statistical significance (p-value < 0.05) compared to Econtrol cultures treated with the respective disinfectant. Error bars represent one standard deviation of at least triplicate samples.
Discussion
In this work, repeated growth and disinfection of E. coli cultures enhanced bacterial tolerance against monochloramine (Figure 2A), but not ferrate (Figure 2B). Results also showed that removing repeated exposure to monochloramine after 25 disinfection rounds did not restore the EMS tolerance against monochloramine to levels observed in ancestral cultures (Figure 2A). Since monochloramine tolerance did not reverse back to ancestral susceptibility levels, repeated monochloramine disinfection likely drove resistance in E. coli cultures against monochloramine. These results are in line with earlier studies that reported chlorine resistance in E. coli following deliberate and repeated exposure to the disinfectant (Farkas-Himsley, 1964; Leyval et al., 1984; Gundlach and Winter 2014). In previous studies, E. coli cultures that were either isolated from source water routinely treated with chlorine (Farkas-Himsley, 1964) or repeatedly exposed to free chlorine (Leyval et al., 1984; Gundlach and Winter 2014) tolerated increasingly higher chlorine dosages. The underlying mechanisms contributing to resistance against chlorine or oxidative stress-inducing conditions remain not well understood (Du et al., 2015; da Cruz Nizer et al., 2020; Wang et al., 2020). Adaptive laboratory evolution of E. coli in the presence of hypochlorite (Gundlach and Winter 2014) and hydrogen peroxide (Anand et al., 2020) showed that cells acquired resistance due to evolutionary processes that favored the constitutive expression of OxyR-regulated genes. In these studies, the evolutionary adaptations were attributed to either genomic mutations (Anand et al., 2020) or epigenetic changes (Gundlach and Winter 2014). To examine if the evolved resistance against monochloramine was due to genomic mutations in this study, we sequenced the genomes of the ancestral and the evolved EMS (round 32) and Econtrol strains (round 32) and compared them against the E. coli 1,223 reference genome. The lack of notable mutations in EMS cultures suggests that the evolutionary adaptions could be due to epigenetic changes or the constitutive expression of stress-related responses.
Mechanisms that trigger tolerance or resistance, or lack thereof, against disinfectants remain poorly understood, although a handful of studies have suggested the potential involvement of oxidative stress responses (Dukan and Touati, 1996; Berry et al., 2010; Gundlach and Winter 2014). As highlighted previously (Gundlach and Winter 2014), showed that E. coli populations resisted frequent and incremental increases in hypochlorite dosage due to elevated OxyR-dependent oxidative stress responses. On the other hand, Ikai et al. (2013) showed that frequent disinfection using fast-acting oxidants involving hydroxyl radicals did not alter E. coli susceptibility following 40 exposure cycles. It was hypothesized that the fast-acting hydroxyl radical indiscriminately targeted cellular structures and metabolic pathways, preventing the evolution of resistance (Ikai et al., 2013). Similarly, ferrate exhibits a faster mode of disinfection compared to monochloramine (Ramseier et al., 2011; Daer et al., 2021). Therefore, the fast action of ferrate and ferrate-derived reactive oxygen species may have hindered adaptive processes against the disinfectant, leading to the lack of resistance development against ferrate. Additionally, we have previously shown that E. coli cultures treated with ferrate resulted in a different gene expression profile than cultures treated with monochloramine, suggesting that the disinfectants trigger different cellular responses (Daer et al., 2021).
An analysis of cellular viability post-disinfection showed that ferrate resulted in a significant loss in viability compared to monochloramine (Figure 3). In line with previous studies (Ramseier et al., 2011; Linklater, 2017; Daer et al., 2021), ferrate consistently inactivated EFS cultures in each disinfection round by disrupting the cell envelope, as evidenced by the significantly higher percentage of damaged cells after each disinfection episode (Figure 3). On the other hand, the observed differences between LIVE/DEAD results and culturable cell concentrations (Figure 2A and Figure 3) of EMS cultures indicate that monochloramine induced a fraction of E. coli into the viable but nonculturable (VBNC) state in the initial disinfection rounds. The VBNC fraction of EMS cultures likely decreased after 12 + disinfection rounds with more cells retaining culturability as evidenced by the increase in culturable cell concentrations (Figure 2A). As such, the disinfectants’ mode of action and adaptive responses against disinfection may have contributed to the observed differences in the inactivation trend over the course of repeated disinfection rounds.
VBNC induction in response to UV and chlorine-based disinfectants has been observed (Ben Said et al., 2010; Lin et al., 2017; Chen et al., 2018; Zhang et al., 2018). Although VBNC cells are viable and intact, they exhibit limited metabolism compared to culturable counterparts (Zhao et al., 2016; Lin et al., 2017; Ye et al., 2020). By reducing essential metabolic processes and becoming dormant, VBNC cells can withstand various stressors, including oxidative stress (Chen et al., 2018; Liao et al., 2019), prolonged starvation (Liu et al., 2009), and extreme environments (Boaretti et al., 2003), and may resuscitate if favorable growth conditions are restored. Similarly, VBNC induction may be a survival strategy adopted by a fraction of the E. coli population in response to monochloramine disinfection. As opposed to ferrate, cells’ ability to withstand monochloramine while retaining viability may have driven resistance development against repeated monochloramine disinfection.
Following 12 + disinfection rounds, the observed increase in cell culturability within the population post-disinfection was not likely caused by VBNC cells resuscitating and becoming culturable within the 60 min disinfection timeframe. Bacterial resuscitation from VBNC states has been shown to be slow and dependent on inducers’ presence in the resuscitation media (Liu et al., 2009). The change in culturable cell concentrations over the exposure time was examined to confirm the absence of cell resuscitation. As shown in Supplementary Figure S1, the loss of culturable cell concentrations over the disinfection exposure time (60 min) was gradual and significantly smaller in EMS cultures compared to ancestral and Econtrol. These observations indicate that the percentage of the E. coli cells likely to enter the VBNC state decreased following multiple rounds of monochloramine disinfection. VBNC-induced state in E. coli by monochloramine may be a regulated process which is also influenced through repeated exposure to the disinfectant. Figure 7 schematically illustrates the potential shift in the fraction of E. coli population that enter VBNC due to multiple episodes of monochloramine disinfection, leading to the observed resistance pattern against monochloramine.
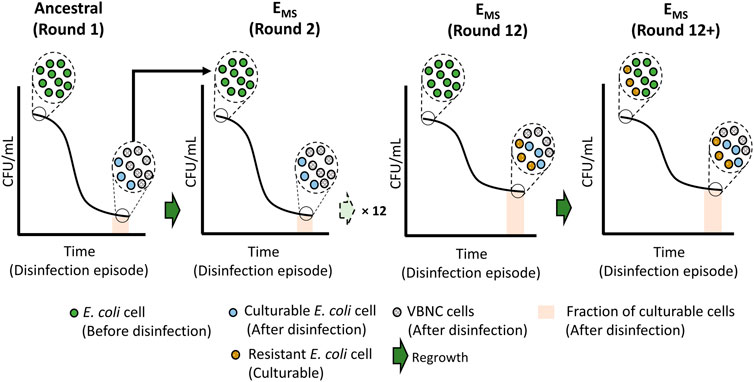
FIGURE 7. Schematic demonstrating the potential emergence of resistant cells and the shift in the fraction of VBNC and culturable cells post-disinfection in response to multiple rounds of monochloramine disinfection.
An understanding of the molecular pathways that regulate entry into the VBNC state remains elusive, although critical to better understand this study’s findings (Boaretti et al., 2003; Li et al., 2014; Pinto et al., 2015; Dong et al., 2020). Recent literature suggests that interrelated mechanisms involving the major stress regulator (RpoS), guanosine pentaphosphate [(p) ppGpp], LysR-type transcriptional factor (OxyR), cAMP, and cAMP-receptor protein (CRP) contribute to the formation of VBNC cells (Boaretti et al., 2003). Boaretti et al. (2003) showed that E. coli strains with increased (p) ppGpp production did not readily lose culturability and enter VBNC state following prolonged starvation. Nosho et al. (2018) reported that starvation caused wild-type cells to lose culturability (i.e., enter a VBNC state) but not mutant E. coli strains that lacked cAMP or CRP production. Furthermore, E. coli strains with decreased cAMP levels and cAMP-CRP activity conferred high resistance to hypochlorous acid due to the accumulation of cellular RpoS (Barth et al., 2009). Hence, cAMP and the cAMP-CRP regulatory pathway could play a role in VBNC induction and resistance to monochloramine. We hypothesized that a drop in cellular cAMP concentrations in EMS cultures may be the underlying cause for the lower occurrence of the cAMP-CRP-induced VBNC state and, in turn, the simultaneous increase in bacterial culturability. In other words, EMS cultures may have adapted to repeated monochloramine disinfection by regulating cAMP levels and minimizing the likelihood of the monochloramine-induced VBNC state. Although transcriptomic analysis was beyond the scope of this study, it is the next step for identifying the regulatory pathways and genes involved in the drop of cAMP levels due to frequent monochloramine treatment.
Given the hypothesized relationship between cellular cAMP concentration, entry into the VBNC state, and monochloramine resistance, we examined the effect of cAMP levels on culturability of evolved E. coli cultures. As results show, evolved EMS cultures that were pretreated with cAMP were significantly less culturable following monochloramine treatment compared to EMS cultures that were not pretreated with cAMP (Figure 4). In addition, presence of exogenous cAMP during disinfection changed the culturability of EMS but not Econtrol, indicating that repeated monochloramine disinfection triggered changes in the cAMP-CRP system in resistant cells, possibly through decreased intracellular cAMP concentrations.
Intracellular cAMP levels are dynamically regulated through multiple, complex mechanisms that involve the sugar phosphotransferase system (PTS), cellular ATP levels, efflux proteins, adenyl cyclases, and phosphodiesterases (Green et al., 2014). Higher cAMP levels have been linked to nutrient and environmental stress in bacteria (Shimizu, 2013). In fact, results from our previous study indicate that a one-time monochloramine dose (i.e., disinfection round 1) induces the expression of PTS-related genes and downregulates genes encoding cAMP-relevant efflux proteins such as tolC in late stationary phase E. coli cells (Daer et al., 2021), both of which could lead to increased intracellular cAMP levels (Postma et al., 1993; Green et al., 2014) in the ancestral and Econtrol cultures receiving only single disinfectant doses without repetition. Though more work is needed to determine the mechanisms, our observations suggest that repeated monochloramine disinfection triggered evolutionary processes that led to lower intracellular cAMP concentrations in resistant EMS cells.
Another key question is whether a lower disinfection frequency could prevent the emergence of bacterial resistance against monochloramine. Recurring stressors can facilitate resistance development in bacteria by increasing the frequency of stress-induced mutagenesis, epigenetic changes, or selection of favorable adaptations (Gundlach and Winter 2014; LaCroix et al., 2015; Lukačišinová et al., 2017; Anand et al., 2020). However, stress-induced adaptations confer a fitness cost that discourages resistance evolution under favorable growth conditions in which growth stressors are absent or not frequent (Björkholm et al., 2001; Day, 2016; van den Bergh et al., 2016). For example, bacterial populations exposed to a disinfectant every 100 generations may trigger resistance, whereas exposure every 1,000 generations may not be frequent enough to drive the mutagenesis or selection of adaptive traits. In a potable water reuse context, time between disinfection events (i.e., disinfection frequency) could be controlled by the size of the environmental buffer (for IPR) or the length of retention times within pipes and treatment trains (for DPR). However, our results indicate that monochloramine resistance occurred despite the reduction in disinfection frequency (Figure 5A). As mentioned above, frequent monochloramine disinfection (every 48 h) drove monochloramine resistance by increasing the fraction of cells that retained culturability post-disinfection through mechanisms involving cAMP (Figure 4). Similarly, culturable cell concentrations in EMS-96 and EMS-144 cultures pretreated with cAMP were lower by 1.5 ± 0.5-log10 and 0.5 ± 0.4-log10 compared to EMS cultures without cAMP pre-treatment, respectively (Supplementary Figure S3, S4). As such, the relative increase in culturable cell concentrations upon monochloramine exposure in evolved populations of E. coli appears to be regulated by cAMP-dependent pathways even when the intervals between disinfection episodes were varied. These observations further suggest that despite infrequent disinfection, similar physiological responses evolved within the bacterial populations, leading to resistance against repeated monochloramine disinfection.
The recurrence of other growth-related stressors during each growth round, i.e., late stationary phase, may explain monochloramine resistance development despite infrequent disinfection. During late stationary phase, bacterial cultures grow in harsher conditions due to nutrient deprivation, resource competition, and other growth-related stressors. For example, Lisle et al. (1998) observed that phenotypic tolerance against chlorine increased in E. coli cultures that were carbon-starved. Du et al. (2015) found that starved E. coli cultures grown solely in phosphate-buffered saline solutions resisted monochloramine treatment by triggering proteomic adaptations against starvation and oxidative stress. In a previous study, we noted the elevated expression levels of general stress responses pre-disinfection due to late-stationary phase growth stressors, which could influence bacterial stress responses following monochloramine treatment (Daer et al., 2021). Frequent regrowth into late stationary phase and recurrence of stationary phase stress factors may have imposed an additional selective pressure for monochloramine resistance. Finished water quality in water reuse is oligotrophic due to the low concentration of nutrients and organic matter post-treatment, pointing to the likelihood that bacterial cells present in the water would have late stationary phase-like physiologies. Therefore, growth-related stressors in potable water reuse may further exacerbate monochloramine resistance.
Lastly, EMS and EFS cultures were cross-disinfected with ferrate and monochloramine, respectively, to determine if evolutionary adaptations triggered by frequent disinfection could protect cells against exposure to the opposite disinfectant. Cross-disinfection results showed that EMS cultures were significantly more susceptible to ferrate compared to monochloramine (Figure 6), suggesting that the cellular adaptations triggered by the repeated monochloramine treatments did not enhance tolerance against ferrate. Ferrate can react more strongly with cell membranes compared to chlorine-based disinfectants and has been shown to exhibit faster disinfection kinetics (Daer et al., 2021). Ferrate also possesses a higher oxidation potential (E0 = 2.2V, acidic media (Wood, 1958)) than monochloramine (E0 = 1.5V, pH 0 (Rajasekharan et al., 2007)), which could result in different oxidative reactions in bacterial cells. Similarly, previous studies have shown that a greater percentage of E. coli cells lost cell integrity and, in turn, viability due to ferrate exposure compared to hypochlorite (Linklater, 2017). Ramseier et al. (2011) noted that the damage to the membranes of drinking water bacterial cells was two orders of magnitude higher due to ferrate (∼9 × 10–3 L/mg×min) compared to monochloramine (∼4 × 10–5 L/mg×min) treatment. Furthermore, bacterial mixtures from the chlorine-resistant genera, such as Mycobacterium and Bacillus, were inactivated by more than 99.8% using ferrate, despite their durable cell wall structure (Gombos et al., 2012). Therefore, we speculate that ferrate and its intermediates indiscriminately and rapidly (within minutes) oxidize cellular components, thereby causing irreversible cellular damage and interfering with the evolution of adaptations against disinfection. As such, intracellular adaptations against repeated monochloramine treatment did not protect adapted cells against ferrate-driven oxidation of the membrane and its constituents.
Environmental Implications
As water utilities move towards shorter urban water cycles, microbial resistance and decreased susceptibility to conventional disinfectants pose new challenges to disinfection. This study explored the potential development of bacterial resistance in response to repeated disinfection that may occur in potable water reuse scenarios. Our observations suggest there is a potential risk in microbial resistance developing against repeated disinfection that is mediated by changes in the culturability of bacterial cells. At the molecular level, epigenetic changes or the constitutive expression of stress-related responses in E. coli may have triggered the enhanced bacterial response against frequent monochloramine disinfection. However, the lack of resistance observed under repeated ferrate disinfection also suggests that using strong oxidants with high reactivity for primary disinfection may be advantageous for pathogen eradication (resistant or otherwise) compared to slower-acting chlorine-based disinfection. Since the development of disinfection resistance is highly undesirable, AWT trains used in potable water reuse will need to implement appropriate disinfectants and measures that minimize the formation of resistant organisms post-disinfection. Disinfectants that remove pathogens while preventing pathogen resistance are crucial in potable water reuse systems. Based on this study, the reactivity of a disinfectant may be an important criterion to consider for the selection of effective and alternative primary disinfectants in potable water reuse, even if slower-acting chlorine-based compounds are necessary as residual disinfectants. Furthermore, this work showed that bacterial physiological responses, such as entry into the VBNC state, could facilitate the long-term emergence of bacterial resistance in response to repeated exposure to monochloramine. Future work is needed to unravel the molecular pathways that govern and regulate those physiological responses among various emerging pathogens of concern. Overall, the findings of this study are important for re-evaluating and re-designing current and future water disinfection schemes to protect public health even as climate and water use patterns continue to evolve and change.
Data Availability Statement
The dataset accompanying this study has been made publicly available on Figshare. Data can be accessed using the following link: https://doi.org/10.6084/m9.figshare.14785845.v1.
Author Contributions
SD conducted the experiments, analyzed the data, and wrote the manuscript. ER and JR conducted the experiments. KI designed the experiments, modified the manuscript and provided feedback. All authors reviewed the manuscript.
Funding
Authors acknowledge the partial support by the Department of Interior, Bureau of Reclamation (R17AC00133) for this research. The funding agency played no other role in this research or reporting.
Conflict of Interest
The authors declare that the research was conducted in the absence of any commercial or financial relationships that could be construed as a potential conflict of interest.
Publisher’s Note
All claims expressed in this article are solely those of the authors and do not necessarily represent those of their affiliated organizations, or those of the publisher, the editors, and the reviewers. Any product that may be evaluated in this article, or claim that may be made by its manufacturer, is not guaranteed or endorsed by the publisher.
Acknowledgments
The authors acknowledge and thank Element 26 Technology (400 Hobbs Road, Suite 107, League City, TX 77546; c291bmRhci5yYW1jaGFuZHJhbkBnbWFpbC5jb20=; anRzdHJlaGxAZ21haWwuY29t) for supplying the potassium ferrate product used in this study.
Supplementary Material
The Supplementary Material for this article can be found online at: https://www.frontiersin.org/articles/10.3389/fenvs.2022.855224/full#supplementary-material
References
Amiri, F., Mesquita, M. M. F., and Andrews, S. A. (2010). Disinfection Effectiveness of Organic Chloramines, Investigating the Effect of pH. Water Res. 44, 845–853. doi:10.1016/j.watres.2009.09.004
Anand, A., Chen, K., Catoiu, E., Sastry, A. V., Olson, C. A., Sandberg, T. E., et al. (2020). OxyR Is a Convergent Target for Mutations Acquired during Adaptation to Oxidative Stress-Prone Metabolic States. Mol. Biol. Evol. 37, 660–667. doi:10.1093/molbev/msz251
Ayrapetyan, M., Williams, T., and Oliver, J. D. (2018). Relationship between the Viable but Nonculturable State and Antibiotic Persister Cells. J. Bacteriol. 200 (20), e00249–18. doi:10.1128/jb.00249-18
Barth, E., Gora, K. V., Gebendorfer, K. M., Settele, F., Jakob, U., and Winter, J. (2009). Interplay of Cellular cAMP Levels, σ S Activity and Oxidative Stress Resistance in Escherichia coli. Microbiology 155, 1680–1689. doi:10.1099/mic.0.026021-0
Ben Said, M., Masahiro, O., and Hassen, A. (2010). Detection of Viable but Non Cultivable Escherichia coli after UV Irradiation Using a Lytic Qβ Phage. Ann. Microbiol. 60, 121–127. doi:10.1007/s13213-010-0017-4
Benedict, K. M., Reses, H., Vigar, M., Roth, D. M., Roberts, V. A., Mattioli, M., et al. (2017). Surveillance for Waterborne Disease Outbreaks Associated with Drinking Water - United States, 2013-2014. MMWR Morb Mortal Wkly Rep. 66, 1216–1221. doi:10.15585/mmwr.mm6644a3External
Berry, D., Holder, D., Xi, C., and Raskin, L. (2010). Comparative Transcriptomics of the Response of Escherichia coli to the Disinfectant Monochloramine and to Growth Conditions Inducing Monochloramine Resistance. Water Res. 44, 4924–4931. doi:10.1016/j.watres.2010.07.026
Björkholm, B., Sjölund, M., Falk, P. G., Berg, O. G., Engstrand, L., and Andersson, D. I. (2001). Mutation Frequency and Biological Cost of Antibiotic Resistance in Helicobacter pylori. Proc. Natl. Acad. Sci. 98, 14607–14612. doi:10.1073/pnas.241517298
Boaretti, M., del Mar Lleo, M., Bonato, B., Signoretto, C., and Canepari, P. (2003). Involvement of rpoS in the Survival of Escherichia coli in the Viable but Non-Culturable State. Environ. Microbiol. 5, 986–996. doi:10.1046/j.1462-2920.2003.00497.x
Bradbury, P. J., Zhang, Z., Kroon, D. E., Casstevens, T. M., Ramdoss, Y., and Buckler, E. S. (2007). TASSEL: Software for Association Mapping of Complex Traits in Diverse Samples. Bioinformatics 23, 2633–2635. doi:10.1093/bioinformatics/btm308
Carmel-Harel, O., and Storz, G. (2000). Roles of the Glutathione- and Thioredoxin-Dependent Reduction Systems in the Escherichia Coli and Saccharomyces Cerevisiae Responses to Oxidative Stress. Annu. Rev. Microbiol. 54, 439–461. doi:10.1146/annurev.micro.54.1.439
CDC (2020). National Outbreak Reporting System. Available at: https://www.cdc.gov/nors/data/dashboard/index.html (Accessed February 14, 2022).
Chen, S., Li, X., Wang, Y., Zeng, J., Ye, C., Li, X., et al. (2018). Induction of Escherichia coli into a VBNC State through Chlorination/Chloramination and Differences in Characteristics of the Bacterium between States. Water Res. 142, 279–288. doi:10.1016/j.watres.2018.05.055
da Cruz Nizer, W. S., Inkovskiy, V., and Overhage, J. (2020). Surviving Reactive Chlorine Stress: Responses of Gram-Negative Bacteria to Hypochlorous Acid. Microorganisms 8, 1220. doi:10.3390/microorganisms8081220
Daer, S., Goodwill, J. E., and Ikuma, K. (2021). Effect of Ferrate and Monochloramine Disinfection on the Physiological and Transcriptomic Response of Escherichia coli at Late Stationary Phase. Water Res. 189, 116580. doi:10.1016/j.watres.2020.116580
Daer, S., and Ikuma, K. (2021). Whole Genome Sequencing and Identification of Mutations in E. coli Cultures after Repeated Disinfection with Monochloramine. https://doi.org/10.6084/m9.figshare.14785845.v1. (Accessed February 23, 2022).
Day, T. (2016). Interpreting Phenotypic Antibiotic Tolerance and Persister Cells as Evolution via Epigenetic Inheritance. Mol. Ecol. 25, 1869–1882. doi:10.1111/mec.13603
Dong, K., Pan, H., Yang, D., Rao, L., Zhao, L., Wang, Y., et al. (2020). Induction, Detection, Formation, and Resuscitation of Viable but Non‐Culturable State Microorganisms. Compr. Rev. Food Sci. Food Saf. 19, 149–183. doi:10.1111/1541-4337.12513
Du, Z., Nandakumar, R., Nickerson, K. W., and Li, X. (2015). Proteomic Adaptations to Starvation Prepare Escherichia coli for Disinfection Tolerance. Water Res. 69, 110–119. doi:10.1016/j.watres.2014.11.016
Dukan, S., Dadon, S., Smulski, D. R., Belkin, S., Dukan, S. A. M., Dadon, S., et al. (1996). Hypochlorous Acid Activates the Heat Shock and soxRS Systems of Escherichia coli. Appl. Environ. Microbiol. 62, 4003–4008. doi:10.1128/aem.62.11.4003-4008.1996
Dukan, S., and Touati, D. (1996). Hypochlorous Acid Stress in Escherichia coli: Resistance, DNA Damage, and Comparison with Hydrogen Peroxide Stress. J. Bacteriol. 178, 6145–6150. doi:10.1128/jb.178.21.6145-6150.1996
Farkas-Himsley, H. (1964). Killing of Chlorine-Resistant Bacteria by Chlorine-Bromine Solutions. Appl. Microbiol. 12, 1–6. Available at: http://aem.asm.org/cgi/content/abstract/12/1/1. doi:10.1128/am.12.1.1-6.1964
Gerberding, J., Popovic, T., Stephans, J., Solomon, S., Bernhardt, J., and Daniel, K. (2008). Surveillance for Waterborne Disease and Outbreaks Associated with Recreational Water Use and Other Aquatic Facility-Associated Health Events — and Outbreaks Associated with Drinking Water and Water Not Intended for Drinking —United States, 2005-2006. CDC- Morbidity Mortality Weekly Rep. 57, 2–67.
Gombos, E., Felföldi, T., Barkács, K., Vértes, C., Vajna, B., and Záray, G. (2012). Ferrate Treatment for Inactivation of Bacterial Community in Municipal Secondary Effluent. Bioresour. Technol. 107, 116–121. doi:10.1016/j.biortech.2011.12.053
Green, J., Stapleton, M. R., Smith, L. J., Artymiuk, P. J., Kahramanoglou, C., Hunt, D. M., et al. (2014). Cyclic-AMP and Bacterial Cyclic-AMP Receptor Proteins Revisited: Adaptation for Different Ecological Niches. Curr. Opin. Microbiol. 18, 1–7. doi:10.1016/j.mib.2014.01.003
Gundlach, J., and Winter, J. (2014). Evolution of Escherichia coli for Maximum HOCl Resistance through Constitutive Expression of the OxyR Regulon. Microbiology (Reading, England) 160, 1690–1704. doi:10.1099/mic.0.074815-0
Haas, C. N., and Morrison, E. C. (1981). Repeated Exposure of Escherichia coli to Free Chlorine: Production of Strains Possessing Altered Sensitivity. Water Air Soil Pollut. 16, 233–242. doi:10.1007/bf01046857
Hoff, J. C., and Akin, E. W. (1986). Microbial Resistance to Disinfectants: Mechanisms and Significance. Environ. Health Perspect. 69, 7–13. doi:10.1289/ehp.8669710.2307/3430366
Ikai, H., Odashima, Y., Kanno, T., Nakamura, K., Shirato, M., Sasaki, K., et al. (2013). In Vitro evaluation of the Risk of Inducing Bacterial Resistance to Disinfection Treatment with Photolysis of Hydrogen Peroxide. PLoS ONE 8, e81316. doi:10.1371/journal.pone.0081316
Inatsu, Y., Bari, M. L., Kitagawa, T., Kawasaki, S., Juneja, V. K., and Kawamoto, S. (2010). The Effect of Repeated Sodium Hypochlorite Exposure on Chlorine Resistance Development in Escherichia coli O157:H7. Food Sci. Technol. Res. 16, 607–612. doi:10.3136/fstr.16.607
Kantor, R. S., Miller, S. E., and Nelson, K. L. (2019). The Water Microbiome through a Pilot Scale Advanced Treatment Facility for Direct Potable Reuse. Front. Microbiol. 10, 1–15. doi:10.3389/fmicb.2019.00993
LaCroix, R. A., Sandberg, T. E., O'Brien, E. J., Utrilla, J., Ebrahim, A., Guzman, G. I., et al. (2015). Use of Adaptive Laboratory Evolution to Discover Key Mutations Enabling Rapid Growth of Escherichia coli K-12 MG1655 on Glucose Minimal Medium. Appl. Environ. Microbiol. 81, 17–30. doi:10.1128/AEM.02246-14
Levin-Reisman, I., Ronin, I., Gefen, O., Braniss, I., Shoresh, N., and Balaban, N. Q. (2017). Antibiotic Tolerance Facilitates the Evolution of Resistance. Science 355, 826–830. doi:10.1126/science.aaj2191
Leyval, C., Arz, C., Block, J. C., and Rizet, M. (1984). Escherichia coli Resistance to Chlorine after Successive Chlorinations. Environ. Technol. Lett. 5, 359–364. doi:10.1080/09593338409384286
Li, H., and Durbin, R. (2010). Fast and Accurate Long-Read Alignment with Burrows-Wheeler Transform. Bioinformatics 26, 589–595. doi:10.1093/bioinformatics/btp698
Li, L., Mendis, N., Trigui, H., Oliver, J. D., and Faucher, S. P. (2014). The Importance of the Viable but Non-Culturable State in Human Bacterial Pathogens. Front. Microbiol. 5, 258. doi:10.3389/fmicb.2014.00258
Liao, H., Zhong, X., Xu, L., Ma, Q., Wang, Y., Cai, Y., et al. (2019). Quorum-Sensing Systems Trigger Catalase Expression to Reverse the oxyR Deletion-Mediated VBNC State in Salmonella Typhimurium. Res. Microbiol. 170, 65–73. doi:10.1016/j.resmic.2018.10.004
Lin, H., Ye, C., Chen, S., Zhang, S., and Yu, X. (2017). Viable but Non-Culturable E. coli Induced by Low Level Chlorination Have Higher Persistence to Antibiotics Than Their Culturable Counterparts. Environ. Pollut. 230, 242–249. doi:10.1016/j.envpol.2017.06.047
Linklater, N. (2017). Individual and Combined Use of Ultraviolet (UV) Light with Hydrogen Peroxide, Peracetic Acid, and Ferrate (VI) for Wastewater Disinfection. Doctoral dissertation. Ottawa, (ON): Carlton University.
Lisle, J. T., Broadaway, S. C., Prescott, A. M., Pyle, B. H., Fricker, C., and Mcfeters, G. A. (1998). Effects of Starvation on Physiological Activity and Chlorine Disinfection Resistance in Escherichia coli O157:H7. Appl. Environ. Microbiol. 64, 4658–4662. doi:10.1128/aem.64.12.4658-4662.1998
Liu, Y., Wang, C., Tyrrell, G., Hrudey, S. E., and Li, X.-F. (2009). Induction of Escherichia coli O157:H7 into the Viable but Non-culturable State by Chloraminated Water and River Water, and Subsequent Resuscitation. Environ. Microbiol. Rep. 1, 155–161. doi:10.1111/j.1758-2229.2009.00024.x
Lukačišinová, M., Novak, S., and Paixão, T. (2017). Stress-induced Mutagenesis: Stress Diversity Facilitates the Persistence of Mutator Genes. Plos Comput. Biol. 13, e1005609. doi:10.1371/journal.pcbi.1005609
Nosho, K., Fukushima, H., Asai, T., Nishio, M., Takamaru, R., Kobayashi-Kirschvink, K. J., et al. (2018). cAMP-CRP Acts as a Key Regulator for the Viable but Non-Culturable State in Escherichia coli. Escherichia Coli. Microbiol. (United Kingdom) 164, 410–419. doi:10.1099/mic.0.000618
Park, S.-k., and Hu, J. Y. (2010). Assessment of the Extent of Bacterial Growth in Reverse Osmosis System for Improving Drinking Water Quality. J. Environ. Sci. Health A 45, 968–977. doi:10.1080/10934521003772386
Pecson, B. M., Triolo, S. C., Olivieri, S., Chen, E. C., Pisarenko, A. N., Yang, C.-C., et al. (2017). Reliability of Pathogen Control in Direct Potable Reuse: Performance Evaluation and QMRA of a Full-Scale 1 MGD Advanced Treatment Train. Water Res. 122, 258–268. doi:10.1016/j.watres.2017.06.014
Pinto, D., Santos, M. A., and Chambel, L. (2015). Thirty Years of Viable but Nonculturable State Research: Unsolved Molecular Mechanisms. Crit. Rev. Microbiol. 41, 61–76. doi:10.3109/1040841X.2013.794127
Postma, P. W., Lengeler, J. W., and Jacobson, G. R. (1993). Phosphoenolpyruvate: Carbohydrate Phosphotransferase Systems of Bacteria. Microbiol. Rev. 57, 543–594. doi:10.1128/mr.57.3.543-594.1993
Rajasekharan, V. V., Clark, B. N., Boonsalee, S., and Switzer, J. A. (2007). Electrochemistry of Free Chlorine and Monochloramine and its Relevance to the Presence of Pb in Drinking Water. Environ. Sci. Technol. 41, 4252–4257. doi:10.1021/es062922t
Ramseier, M. K., von Gunten, U., Freihofer, P., and Hammes, F. (2011). Kinetics of Membrane Damage to High (HNA) and Low (LNA) Nucleic Acid Bacterial Clusters in Drinking Water by Ozone, Chlorine, Chlorine Dioxide, Monochloramine, Ferrate(VI), and Permanganate. Water Res. 45, 1490–1500. doi:10.1016/j.watres.2010.11.016
Reasoner, D. (2002). Heterotrophic Plate Count Bacteria in Potable Water: Monitoring Methods and Application. Washington: U.S Environmental Protection Agency. EPA/600/D-89/072 (NTIS PB89223697).
Shimizu, K. (2013). Regulation Systems of Bacteria Such as Escherichia coli in Response to Nutrient Limitation and Environmental Stresses. Metabolites 4, 1–35. doi:10.3390/metabo4010001
Simon, A. (2018). FastQC: A Quality Control Tool for High Throughput Sequence Data. Available at: http://www.bioinformatics.babraham.ac.uk/projects/fastqc/(Accessed June 6, 2018).
Soller, J. A., Eftim, S. E., and Nappier, S. P. (2018). Direct Potable Reuse Microbial Risk Assessment Methodology: Sensitivity Analysis and Application to State Log Credit Allocations. Water Res. 128, 286–292. doi:10.1016/j.watres.2017.10.034
USEPA (2018). 2017 Potable Reuse Compendium. Available at: https://www.epa.gov/sites/production/files/2018-01/documents/potablereusecompendium_3.pdf (Accessed February 14, 2018).
van den Bergh, B., Michiels, J. E., Wenseleers, T., Windels, E. M., Boer, P. V., Kestemont, D., et al. (2016). Frequency of Antibiotic Application Drives Rapid Evolutionary Adaptation of Escherichia coli Persistence. Nat. Microbiol. 1, 1–7. doi:10.1038/nmicrobiol.2016.20
Wang, S., Deng, K., Zaremba, S., Deng, X., Lin, C., Wang, Q., et al. (2009). Transcriptomic Response of Escherichia coli O157:H7 to Oxidative Stress. Appl. Environ. Microbiol. 75, 6110–6123. doi:10.1128/AEM.00914-09
Wang, Z., Fang, Y., Zhi, S., Simpson, D. J., Gill, A., Mcmullen, L. M., et al. (2020). The Locus of Heat Resistance Confers Resistance to Chlorine and Other Oxidizing Chemicals in Escherichia coli. Appl. Environ. Microbiol. 86, 1–16. doi:10.1128/AEM.02123-19
Winward, G. P., Avery, L. M., Stephenson, T., and Jefferson, B. (2008). Chlorine Disinfection of Grey Water for Reuse: Effect of Organics and Particles. Water Res. 42, 483–491. doi:10.1016/j.watres.2007.07.042
Wood, R. H. (1958). The Heat, Free Energy and Entropy of the Ferrate(VI) Ion. J. Am. Chem. Soc. 80, 2038–2041. doi:10.1021/ja01542a002
Ye, C., Lin, H., Zhang, M., Chen, S., and Yu, X. (2020). Characterization and Potential Mechanisms of Highly Antibiotic Tolerant VBNC Escherichia coli Induced by Low Level Chlorination. Sci. Rep. 10, 1–11. doi:10.1038/s41598-020-58106-3
Zhang, S., Guo, L., Yang, K., Zhang, Y., Ye, C., Chen, S., et al. (2018). Induction of Escherichia coli into a VBNC State by Continuous-Flow UVC and Subsequent Changes in Metabolic Activity at the Single-Cell Level. Front. Microbiol. 9, 1–11. doi:10.3389/fmicb.2018.02243
Keywords: monochloramine, ferrate (VI), VBNC (viable but nonculturable), cAMP, culturability, water reuse
Citation: Daer S, Rehmann E, Rehmann J and Ikuma K (2022) Development of Resistance in Escherichia coli Against Repeated Water Disinfection. Front. Environ. Sci. 10:855224. doi: 10.3389/fenvs.2022.855224
Received: 14 January 2022; Accepted: 16 February 2022;
Published: 14 March 2022.
Edited by:
Susana Segura Munoz, University of São Paulo, BrazilReviewed by:
Guilherme Zagui, University of São Paulo, BrazilAsmaa Mohammed, Beni-Suef University, Egypt
Rosa Ramos, Paraguayan Teacher Medicine University, Paraguay
Copyright © 2022 Daer, Rehmann, Rehmann and Ikuma. This is an open-access article distributed under the terms of the Creative Commons Attribution License (CC BY). The use, distribution or reproduction in other forums is permitted, provided the original author(s) and the copyright owner(s) are credited and that the original publication in this journal is cited, in accordance with accepted academic practice. No use, distribution or reproduction is permitted which does not comply with these terms.
*Correspondence: Kaoru Ikuma, a2lrdW1hQGlhc3RhdGUuZWR1