- 1Natural Resources Management and Environmental Sciences, College of Agriculture, Food and Environmental Sciences, California Polytechnic State University, San Luis Obispo, CA, United States
Agricultural plastics support crop production and quality by reducing weeds, improving irrigation efficiency, and regulating soil conditions, but can also become a soil pollutant. While microplastic effects on soil function are increasingly well-understood, the impacts of agricultural macroplastic (>5 mm) contamination on soils are poorly documented. Prolonged exposure to plastic macrofragments may alter microbial decomposer community structure and function, since plastic can directly affect edaphic factors while leaching novel compounds. To better characterize how plastic contamination influences the soil habitat, we sampled three farms characterized by agricultural plastic pollution in Monterey County, CA, United States. Using a randomized block design, we collected surface soil samples from the fields (“bulk PC soil”) to compare with soil directly in contact with the remaining polyethylene (PE) mulch and polyvinyl chloride (PVC) dripline fragments (“plastic-associated soil”). Soil directly associated with plastic fragments was hypothesized to have reduced microbial biomass and decomposer activities relative to the bulk soil, due to a greater likelihood of toxicity. In contrast to our expectations, we found that both PE and PVC macrofragments support a distinct microbial habitat that hosts a larger, more efficient microbial biomass with greater labile nutrient pools than the surrounding bulk soil. Because of the scope of macroplastic pollution likely occurring in agricultural soils, our findings suggest that this novel plastisphere habitat may significantly alter ecological functions critical to agricultural soils over time by encouraging microbial colonization within plastic debris.
Introduction
The quantity of plastic released into terrestrial systems is 4–23 times greater than that released into freshwater systems, yet the implications of plastic pollution have not yet been fully studied nor quantified (Horton et al., 2017). The use of agricultural plastics has grown rapidly and now covers millions of acres of farmland globally. Single-use plastic has become an essential tool for weed management, air and soil temperature and moisture modulation in specialty crop fields, allowing for the efficient, cost-effective production of specialty crops (Steinmetz et al., 2016; Jiang et al., 2017; Sanchez-Hernandez, 2019; Zhang et al., 2020). Polyethylene (PE) plastic is used in greenhouses, walk-in tunnels, irrigation tape, and in the field as plastic mulch. Polyvinyl chloride (PVC) irrigation dripline is a rigid, non-flexible piping also commonly used in agricultural fields (Yan et al., 2020). While plastics benefit agricultural productivity, they can remain in the soil environment far beyond their original intended and useful lifespan, with poorly quantified impacts on soil functioning.
Plastic removal from fields is labor-intensive and disposal is costly due to the adhesion of soil particles to the films. As a result, agricultural plastic are rarely completely removed from a field and decompose extremely slowly (Selke et al., 2015), leaving plastic residues that remain in soil for decades to centuries and leach, thus also polluting water systems (Jambeck et al., 2015; Brodhagen et al., 2017). The soil remaining associated with these plastic fragments are therefore expected to be strongly influenced by its proximity to the plastic. For instance, PE and PVC become brittle and fragment following light exposure, leading to the release of micro and nanofragments which may affect soil physical and chemical properties. A further risk of PVC material is the release of phthalate esters (i.e., “phthalates”), plasticizer additives that are largely used in the production of PVC (Pivnenko et al., 2016). Phthalates are hydrophobic and have a high lipid affinity, so tend to accumulate on the surface of microbes and soil organic matter rather than being leached out of the system (Wang et al., 2016; Lü et al., 2018; Scalenghe, 2018).
Plastic fragments can remain in the soil environment for decades to centuries, and can accumulate into plants as well as leach into connected watersheds long after their use has ceased (Birch et al., 2020; Li et al., 2020). Plastic mulch residue accumulation in agricultural soils can have multiple negative impacts on plant growth (e.g., reduced crop yield, plant height and root mass) and soil properties (e.g., lower water infiltration rate, organic matter content, and plant-available phosphorus), which threatens long-term food security if these responses are widespread (Zhang et al., 2020). When plastic remains in the soil, it can impact soil nutrient availability and negatively affect microbial communities due to the accumulation of plastic-derived particles and leachates(Fei et al., 2020; Helmberger et al., 2020) High PVC contamination can decrease the soil nitrate (NO3−) (Yan et al., 2020). Additionally, plastic particles can alter soil carbon (C) cycling as C-rich, inert plastics accumulate, widening the soil C:nitrogen (N) ratio and increasing microbial nutrient immobilization (De Souza MacHado et al., 2018; Rillig et al., 2019; Qi et al., 2020). While these recent studies provide evidence of the physical scale and potential biological effects of agriculturally derived microplastics on soil biological properties, identifying the impacts of macroplastic fragments themselves on the soil environment remains poorly documented.
To better characterize the implications of PE and PVC contamination on agricultural soils, we determined the impacts agricultural plastic contamination has on soil biological and physical properties. We sampled fields within three farms in Monterey County, CA—a region recognized for its significant use of agricultural plastics (Hurley, 2008)—that were identified as having substantial agricultural plastic pollution. PE mulch and PVC dripline were left the farms’ fields and marked quantities of these macroplastic fragments remain visible on the soil surface despite previous efforts to remediate the sites by hand collection of plastic. We haphazardly collected soil samples from the field (i.e., “bulk soil”) to compare with soil which had come directly in contact with the remaining surface mulch and dripline (i.e., “plastic-influenced soil”) using a randomized block design.
If plastic incorporation alters edaphic properties while leaching novel compounds, this likely alter both local abiotic conditions and microbial decomposer community structure and function. Thus, macro-plastic fragment additions may create novel habitats in the soil environment. We evaluated a suite of abiotic and biotic characteristics to assess the influence of plastic contamination on agricultural soils. We hypothesized that the soil directly associated with plastic fragments would have reduced microbial biomass and decomposer activities relative to the bulk soil, due to a greater likelihood of toxicity and altered microhabitat, particularly in the PVC-associated soil.
Methods
Site Description
To characterize the consequences of plasticulture on agricultural soil systems, we periodically surveyed three farms (“Site 1—3”) on the California Central Coast in Monterey County, CA (36.910233o N, -121.756897o W) from January-July of 2021. These sites are within a region characterized by significant agricultural plastic use (Brodhagen et al., 2017), with an annual temperature between 8–20°C and mean annual precipitation of 596.9 mm, which falls predominantly during the winter wet season (National Weather Service). These sample sites were selected to reflect sites that have extensive plastic pollution (dripline, mulch, and miscellaneous pieces of litter) despite having been actively remediated by hand-removal of visible plastic.
Site 1 is situated on a 195-acre ranch in Monterey County. It is an active farm which rotates celery, fava beans, romaine, squash, pumpkins, peppers, tomatoes, brussels sprouts, and flowers; in 2016 agricultural plastic was left in a field following flooding. The field was under active cultivation at the time of sampling. Soils are classified as a Santa Ynez fine sandy loam soil with 15–30 percent slopes. It is a moderately well drained alluvial soil derived from igneous and sedimentary rock. Sites 2 and 3 are comprised of standing plant biomass juxtaposed with macroplastic fragments. Site 2 is a fallowed strawberry field (∼50 acres) within a 107-acre parcel set on an Arnold loamy sand with 9–20 percent slopes. It is a somewhat excessively drained soil from residuum weathered from sandstone. Site 2 was a strawberry farm for four decades, but since 2016 has been managed the Elkhorn Slough Foundation. Site 3 is a fallowed field (13 acres) that was contaminated with agricultural plastics in 2018 and 2019. Site 3 is classified as an Arnold loamy sand with 15–50 percent slopes. It is a somewhat excessively drained soil from residuum weathered from sandstone. Each site was sampled on two occasions—once to estimate plastic mass using a transect sampling design and again using a randomized block design to assess the impact of plastic-association on soil properties.
Experimental Design
Surface Soil Contamination Survey
In February, March, and September 2021, surface macroplastic fragment contamination was assessed at Sites 1, 2, and 3, respectively, using a transect method where we sampled along the longest length of each field. A 30.5 m long transect was placed alongside the Site 1 plastic-contaminated field, a 55 m long transect was placed alongside the Site 2 plastic-contaminated field, and a 59 m long transect was run along the edge of the Site 3 plastic-contaminated field (transect survey length was determined by field length). A perpendicular transect (30.5 m) was run every 7 m along the length of each field and a 1 m2 quadrat was centered every 3 m along the perpendicular transect (i.e., 10 m2 quadrats/perpendicular transect). All visible surface plastic was collected from within each quadrat and separated in the lab by plastic type (Figure 1). We scaled the average amount of macroplastic fragments found per m2 to a ha (Piehl et al., 2018). The number of plastic fragments was recorded at the site level, while the mass was recorded at the quadrat level.
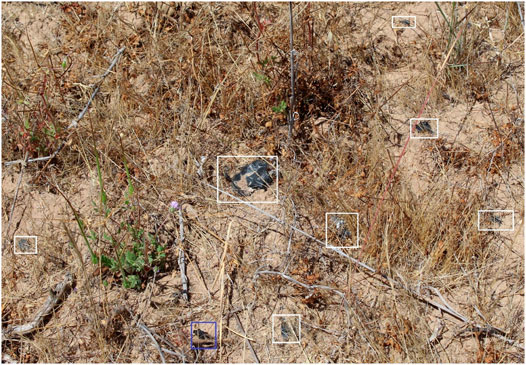
FIGURE 1. Macrofragments of plastic within a sample block at Site 3. White boxes highlight PE mulch fragments and the blue box indicates a PVC tube fragment.
Assessing Plastic Influence on Soil Properties
We used a block design to determine the potential impacts plastic contamination has on a suite of biotic and abiotic edaphic properties. In June and July 2021, a block design of eight haphazardly placed blocks separated by at least 3 m (10 × 30 m at Site 1 and 10 × 20 m at Sites 2 and 3) was established in each site and all visible PVC dripline and PE mulch were separately collected from the quadrat area, as well as eight bulk surface soil samples that were not in direct contact with visible plastic fragments (top 5 cm, homogenizing 3 sub-replicates per quadrat). Samples were returned to the lab at Cal Poly the same day as collection and stored in the fridge overnight until processing the following day. Bulk soils were sieved to remove stones and plant debris. Plastic-associated soil was separated from the macroplastic fragments by hand using spoons which were sterilized with 70% ethanol between samples. Dripline-associated, plastic mulch-associated, and bulk soils were pooled by block to represent a field replicate (N = 8 replicates/field, with 3 fields total sampled).
Soil Biotic Conditions
Microbial Decomposer Biomass
Substrate induced respiration (SIR) was used to estimate active soil microbial biomass (Anderson and Domsch, 1978; Kaiser et al., 1992). SIR is a method of estimating active microbial biomass by providing a labile carbon (C) source of autolyzed yeast to drive a maximum potential respiration rate. Ten mL of 12 g/L yeast extract (Difco) was added to 6 g of fresh soil (or 5 ml yeast extract to 3 g fresh soil when soil was limiting) in a half-pint size mason jar fitted with a gas tight septum (blue butyl rubber septum, Bellco Glass). Each jar shook at 240 rpm for fifteen minutes prior to the first measurement. A bench top infrared gas analyzer (IRGA, LI-COR 850) was used to measure CO2 (
Soil Respiration
To measure soil respiration, three grams of field-moist soil were added to a half-pint size mason jar fitted with a gas tight septum (blue butyl rubber septum, Bellco Glass) in duplicate (for bulk soil only). Respiration (CO2 production) was measured three times over a 24-h period (roughly 8 h apart) using a LiCOR 850 gas analyzer. The soils were kept at room temperature throughout the incubation period (∼23°C). Respiration rate is calculated as the slope of CO2 (
Decomposer Community-Level Physiological Profiling
BIOLOG EcoPlates™ were used to assess how plastic incorporation into agricultural soil impacts microbial community substrate use potential as an index of community-level physiological profile (Stefanowicz, 2006). Fresh soil samples were diluted with phosphate-buffered saline (PBS) to a concentration of 10–3 (g soil/ml PBS) using a serial dilution technique, with each dilution vortexed for 30 s, 100 µL of the 10–3 soil extract dilution was pipetted into each well of the 96 well EcoPlate in triplicate (3 analytical replicates). A negative control (no soil) PBS plate was included to monitor any background growth. Change in absorption over time (every 24 h for up to 6 days, until maximum growth rate was achieved) was measured at 590 nm (absorbance peak of tetrazolium) to evaluate color development plus turbidity and 750 nm to measure turbidity of dilutions is due to clay and humic particles in soil colloidal suspension (Sofo and Ricciuti, 2019) on a Tecan Infinite M Nano Plus plate reader. Substrate preference was assessed by grouping the 31 carbon substrates represented on the Ecoplates by: amine, amino acid, carbohydrate, carboxylic acid, phenolic compounds, and polymers (Sala et al., 2010). We calculated the Bray-Curtis dissimilarity index for bulk PC soil, dripline, and surface mulch associated soil (for all substrates and substrate separated by class when growth was detectable), which was used to calculate the Shannon diversity and richness of substrate use for those substrates on which significant growth was observed. To measure the microbial activity, the average well color development (AWCD) was calculated by determining the mean well absorbance for each sample at each timepoint and fitting a curve.
Soil Abiotic Conditions
Total Inorganic Nitrogen
Extractable inorganic N was assessed by adding 10 ml of 0.5 M K2SO4 to 2 g of fresh soil and shaking the slurry for 1 hour at 250 rpm. The soils settled for 5 min after shaking and were gravity filtered through a Whatman 1 filter paper. Extractant was analyzed using colorimetric microplate assays on a Tecan Infinite M Nano Plus platereader. Ammonium (NH4+) was determined using a modified Berlethot assay (Rhine et al., 1998) and nitrate (NO3−) was assessed using a modified Griess assay (Doane and Horwáth, 2003). NO3− and NH4+ were summed to calculate the total inorganic nitrogen (TIN) content in the soil.
Permanganate Oxidizable Carbon
To determine whether conventional plastic incorporation into the soil affects the readily oxidizable C fraction, permanganate-oxidizable C (POXC) analyses were conducted on air dried soil using a 96 well plate reading absorbance at 550 nm (Tecan Infinite M Nano Plus platereader) (Islam et al., 2003; Culman et al., 2012).
Carbon to Nitrogen Ratio
Soils were first air dried then 2 mm sieved to remove any remaining plant matter. The soils were then pulverized using a mortar and pestle. Soil total % C and % N were determined by weighing ∼1 g of dried soil into crucibles and analyzed using an elemental analyzer (Elementar VarioMax).
Gravimetric Water Content (θg)
Gravimetric water content was measured by weighing ∼10 g of fresh soil into pre-weighed oven tins. Samples were placed in the oven for 48 h at 105°C until the samples came to a stable oven dry weight.
Statistical Analysis
All data analysis was completed in R version 4.0.0 and RStudio version 1.4.1717 (R Core Team, 2021) using lme4 (Bates et al., 2015). For all response variables, plastic association treated as a fixed factor and block nested within site was treated as a random factor. Post-hoc tests to evaluate pairwise plastic association status differences were completed using the emmeans package with Tukey HSD (Lenth et al., 2021). Response variables were log-transformed when necessary to improve normality and for soil C:N following (Isles, 2020). Differences in C substrate use preference, richness, AWCD, and Shannon diversity among the different plastic associations was tested with an ANOVA; the adonis function in Vegan was used to test whether plastic association affected Bray-Curtis dissimilarity (Oksanen et al., 2020). After calculating the mean well absorbance, a curve was fit to determine the AWCD using the growthcurver package (Sprouffske and Wagner, 2016). All plots were made using the ggplot2 function in R (Wickham, 2016). Significant effects were reported for α ≤ 0.05 (overall effect), with pairwise significant differences (Tukey HSD) reported at α ≤ 0.05.
Results
Soil Macroplastic Fragment Contamination Level
Extensive macroplastic contamination was found at all sample sites (Table 1). We found 443 total macroplastic fragments (351 PE fragments and 92 PVC fragments over 66 m2 sampled) at Site 1, reflecting 2.03 ± 0.59 g/m2 total macroplastic fragments (0.47 ± 0.07 g/m2 PE and 1.56 ± 0.56 g/m2 PVC). At Site 2 we identified 77 total macroplastic fragments (60 PE fragments and 17 PVC fragments over 110 m2 sampled), representing 0.34 ± 0.13 g/m2 total macroplastic fragments (0.08 ± 0.04 g/m2 PE and 0.26 ± 0.12 g/m2 PVC). At Site 3, we identified 1,015 total macroplastic fragments (971 PE fragments and 44 PVC fragments over 110 m2 sampled), representing 1.46 ± 0.4 g/m2 total macroplastic fragments (0.6 ± 0.05 g/m2 PE and 0.85 ± 0.4 g/m2 PVC).

TABLE 1. A summary of the extent of macroplastic pollution observed at sample sites 1 – 3. Polyethlyene (PE) and polyvinylchoride (PVC) macrofragments were collected separately. Other non-agriculturally related plastic debris (e.g., miscellaneous litter) was not included in the survey.
Soil Biotic Conditions
Decomposer Biomass, Respiration, and Substrate Preference
Microbial biomass derived from soil associated with dripline and surface mulch had higher biomass relative to the bulk soil (F(2,67) = 19.57, p < 0.0001). However, basal soil respiration rates were similar in bulk and plastic-associated soils (p = 0.13). Specific respiration rate (qCO2) was reduced in both the surface mulch and PVC-associated soil (but did not differ from each other) [F(2,65) = 16.5, p < 0.0001] (Figure 2). Plastic association had no detectable effect on microbial C substrate preference as assessed by community-level physiological profiling; Shannon diversity, average well color development, relative growth on different substrate classes, and overall substrate use profiles were similar between plastic-associated and bulk soil (p > 0.1 in all cases, Supplementary Figures S1A–D).
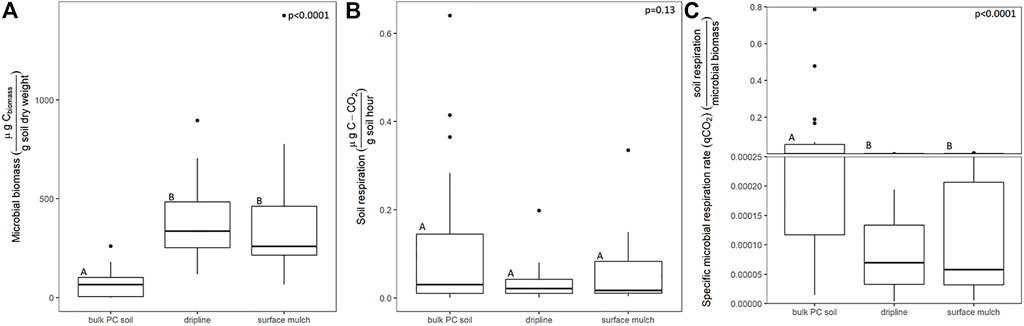
FIGURE 2. The effect of plastic-association (PE mulch and PVC fragments relative to bulk surface soil not directly in contact with plastic macrofragments “bulk pc soil”) on microbial biomass (µg CO2/g C soil/hr) (A), soil respiration (µg CO2/g C soil/hr) (B), and specific microbial respiration (qCO2) (C). The letters above each boxplot indicate the Tukey pairwise connecting letters among treatments following a mixed model, with block nested within site (8 blocks per site, 3 sites) treated as a random variable and plastic association treated as a fixed variable (n = 24). The lower and upper hinges of the boxplot correspond to the first and third quartiles and the median is represented by the line within the box, the whiskers extend no further than 1.5 Xs the interquartile range. Outlying points beyond this range are plotted individually.
Soil Abiotic Conditions
Extractable, Labile, and Total Soil C and N Pools
Plastic-association did not significantly affect total inorganic N (F(2,46) = 1.46, p = 0.24), but differently affected NO3− and NH4+. While NO3− contents did not differ among plastic associations (F(2,46) = 1.45, p = 0.24), NH4+ was significantly higher in both the surface mulch (by 80%) and dripline-associated soils (by 98%) (F(2,46) = 15.45, p < 0.0001) than the bulk soil (Figure 3). Plastic-associated soils had significantly higher POXC than the bulk soil but did not significantly differ from each other (F(2,67) = 7.87, p = 0.0009, Figure 4). Surface mulch-associated POXC values were 25% higher than the bulk soil and dripline-associated POXC values were 37% higher. Like POXC, soil % C was ∼16% greater than bulk soil (F(2,46) = 16.03, p < 0.0001) and soil % N was ∼18% greater than bulk soil (F(2,46 = 17.0, p < 0.0001) in plastic-associated samples, but the plastic-associated soils did not differ from each other (Figure 5). Plastic-association did not influence the soil C:N (F(2,67) = 1.78, p = 0.18).
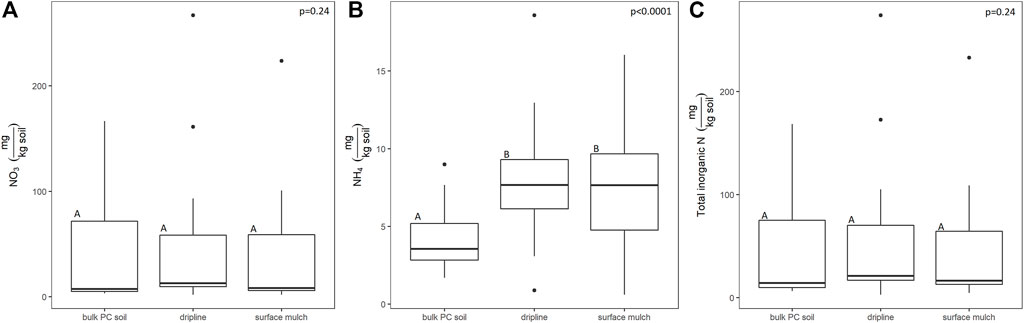
FIGURE 3. The influence of macroplastic association (PE mulch and PVC fragments relative to bulk surface soil not directly in contact with plastic macrofragments “bulk pc soil”) on NO3 (A), NH4 (B), and total inorganic N contents (C) (mg/kg soil). The letters above each boxplot indicate the Tukey pairwise connecting letters among treatments following a mixed model, with block nested within site (8 blocks per site, 3 sites) treated as a random variable and plastic association treated as a fixed variable (n = 24). The lower and upper hinges of the boxplot correspond to the first and third quartiles and the median is represented by the line within the box, the whiskers extend no further than 1.5 Xs the interquartile range. Outlying points beyond this range are plotted individually.
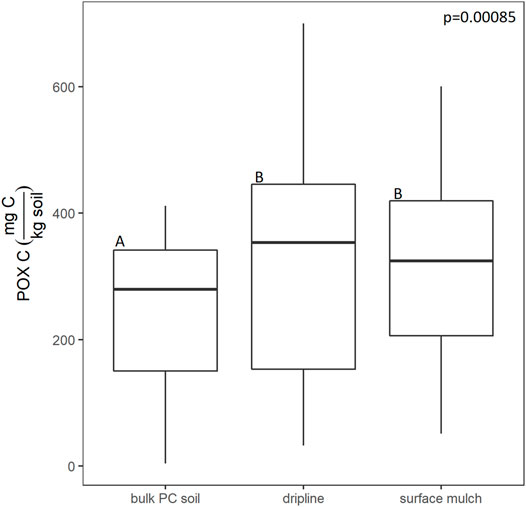
FIGURE 4. The effect of plastic-association (PE mulch and PVC fragments relative to bulk surface soil not directly in contact with plastic macrofragments “bulk pc soil”) on POXC content (mg C/kg soil). The letters above each boxplot indicate the Tukey pairwise connecting letters among treatments following a mixed model, with block nested within site (8 blocks per site, 3 sites) treated as a random variable and plastic association treated as a fixed variable (n = 24). The lower and upper hinges of the boxplot correspond to the first and third quartiles and the median is represented by the line within the box, the whiskers extend no further than 1.5 Xs the interquartile range. Outlying points beyond this range are plotted individually.
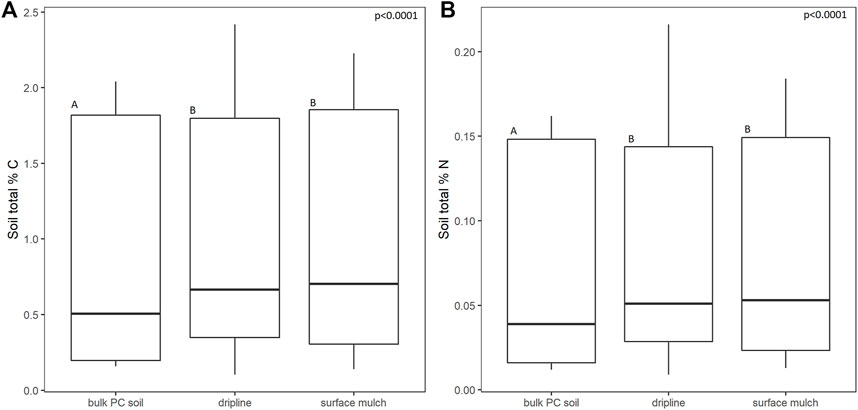
FIGURE 5. The effect of plastic-association (PE mulch and PVC fragments relative to bulk surface soil not directly in contact with plastic macrofragments “bulk pc soil”) on soil total % C (A) and % N (B) content. The letters above each boxplot indicate the Tukey pairwise connecting letters among treatments following a mixed model, with block nested within site (8 blocks per site, 3 sites) treated as a random variable and plastic association treated as a fixed variable (n = 24). The lower and upper hinges of the boxplot correspond to the first and third quartiles and the median is represented by the line within the box, the whiskers extend no further than 1.5 Xs the interquartile range. Outlying points beyond this range are plotted individually.
Discussion
While a valuable agricultural technology, the rise of plasticulture is of growing concern from an environmental and human health perspective, with significant cost burdens to both farmers and society at large (Brodhagen et al., 2017; Piehl et al., 2018; Fakour et al., 2021). Attempts have been made to quantify plastic concentrations within soils, yet the ecological implications of this growing pollution burden remain largely unknown (Huerta Lwanga et al., 2016; Piehl et al., 2018; Fakour et al., 2021). Macroplastic fragments are likely widespread within agricultural soils due to the extent of both plasticulture and plastic that is embedded into other agricultural products (Piehl et al., 2018; Weithmann et al., 2018; Huang et al., 2020). Mexican home gardens were found to host 74,000 ± 65,000 plastic ha−1 (Huerta Lwanga et al., 2017) and PE film macrofragments covered ∼10% of the horticultural soil surface sampled in Argentina (Ramos et al., 2015), while a European field not known to be exposed to agricultural plastics contained approximately 206 macroplastic fragments ha−1 (Piehl et al., 2018).. These studies highlight the potential for extensive plastic contamination in regions with intensive agricultural land use as well as smallholder agricultural production.
We found 5,454–88,272 PE and 1,545–13,939 PVC macrofragments ha−1 which appear to support a distinct microbial environment relative to the bulk soil. Notably, in a field reported to be free of known plastic-contamination at Site 3, we found 1,254 total macroplastic fragments over 110 m2 sampled (1,211 PE fragments (110,090 fragments ha−1) and 43 PVC fragments (3,909 fragments ha−1), suggesting that even adequately managed fields can be macroplastic accumulators. Given the potential scale of macroplastics in agricultural soils, it is critical to characterize both the extent and implications of this novel pollution burden in agricultural systems. Our work suggests that macroplastic fragments integrated into agricultural soils support a unique microhabitat that is distinct from the surrounding bulk soil environment in both nutrient availability and microbial activity.
Microplastics derived from mulching film provide novel microhabitats that support microbial communities which are distinct from the bulk soil (Zhang et al., 2019); this pattern appears to be comparable for soil macroplastic fragments. We found that both PE- and PVC-derived plastics can support biotic and abiotic conditions that are distinct from the neighboring bulk soil, but notably, that they are not distinct from each other despite significant differences in plastic polymer composition (Ru et al., 2020) and evidence that PVC (but not PE) microplastics inhibits both nitrification and denitrification in sediment (Seeley et al., 2020). Soil NH4+, labile C (POXC), microbial biomass, soil % C and % N were all greater in the surface macroplastic fragments than the proximal bulk soil, suggesting these novel materials both support microbial hotspots—or a small soil volume characterized by significantly greater biological activity than the average soil conditions (Kuzyakov and Blagodatskaya, 2015).
Notably, microbial respiration did not parallel the higher microbial biomass and nutrient pools observed in macroplastic fragment-associated soils. Dripline and surface mulch associated soil microbial communities had a lower specific respiration rate, suggesting a larger, but higher efficiency microbial community is supported in the plastic-associated microhabitat (Badia and Alcañiz, 1993). Greater metabolic efficiency of the plastic-associated soil microbial community may reflect changes in microbial community composition and/or reduced physiological stress (Wardle and Ghani, 1995) within the “plastisphere” environment. Our results are consistent with reduced nutrient limitation stress as a driver of reduced mass-specific respiration in the plastisphere, where labile C and ammonium availability were elevated.
On the other hand, our results do not support changes in microbial communities being associated with changes in mass-specific respiration and the abiotic soil environment. This may be because although plastic exposure can influence the composition and behavior of microbial communities, the response is variable across plastic types (Seeley et al., 2020). Microplastic fragments artificially added to agricultural soils have been observed to enrich bacterial taxa capable of plastic polymer biodegradation by selectively accumulating these organisms within the pits and flakes on their surfaces (Fei et al., 2020). Macroplastic fragments in the same agricultural soils were enriched in Bacteroidetes [whose members can both degrade crude oil and other organic polymers and have extensive potential for surface adhesion (Viñas et al., 2005; Bauer et al., 2006)] and Saccharibacteria [which are a sink for organic C in hydrocarbon-enriched environments (Figueroa-Gonzalez et al., 2020)]. However, macroplastic-associated soil was depleted in a range of phyla, including Acidobacteria, Actinobacteria, Chloroflexi, Gemmatimonadetes, Proteobacteria and Tectomicrobia (Fei et al., 2020). Acidobacteria, Actinobacteria, and Proteobacteria are important for the sustained productivity of agricultural soils and these phyla are considered indicative of soil health (Hartmann and Widmer, 2006; Wolińska et al., 2017).
Although we were unable to directly sequence microbial communities from the plastic-associated and bulk soils, our findings support the notion that like microplastic fragments, macroplastics support a distinct habitat for the soil microbiome. Intriguingly, the community-level physiological profiling (Ecoplate) patterns suggests that a more efficient microbial community, coupled to greater microbially available soil C and N levels directly associated with macroplastic fragments may not substantially alter substrate preference, despite potentially shifting microbial community structure (Fei et al., 2020). Similarly, although PE and PVC microplastics differently affect sediment soil N cycling dynamics (Seeley et al., 2020) and are associated with distinct leachates(Pivnenko et al., 2016), we found a comparable influence of macroplastic fragment association on increasing NH4+ (but not NO3−) availability. This further suggests that physical habitat changes created by the macroplastic fragments drive microbial hotspot characteristics, whether or not there were functional shifts in microbial community structure.
Both PVC and PE macroplastic fragments in our focal agricultural fields altered soil microbial and physical properties relative to the neighboring bulk soil. Given the increasing evidence of microplastic pollution impacts on soil systems (De Souza MacHado et al., 2018; Qi et al., 2018; Corradini et al., 2019; Rillig et al., 2019; Liang et al., 2021), whether the novel conditions created by macroplastics embedded in soils is associated with a higher concentration of microplastics, and how macroplastic impacts on soils relate to those of microplastics remains a critical unknown. Carbon-poor soils are more susceptible to disruption of microbial enzyme activities and water-stable aggregate formation by microplastic fibers than soils with greater organic matter content (Liang et al., 2021). If the macroplastic PE and PVC associated soil supports higher microplastic contaminants than the surround bulk soil, this highly spatially heterogenous pollution burden may create a novel stressor for the immediate microbial community. Our study provides strong evidence for the formation of a novel habitat on the surface of macroplastic fragments in relatively C-poor agricultural soils which supports a larger, more efficient microbial biomass with greater labile nutrient pools than the surrounding bulk soil. Thus, the addition of macroplastic fragments to soil, regardless of specific polymer composition, physical structure, and degradability, may have comparable and sustained effects on a suite of critical soil biological and abiotic characteristics with implications for sustained agroecological function.
Data Availability Statement
The original contributions presented in the study are included in the article/Supplementary Material, further inquiries can be directed to the corresponding author.
Author Contributions
SS, OM, and GP designed the research. OM, PM, and SS collected field samples. OM, PM, and GP completed laboratory analyses. OM, SS, and GP conducted statistical analyses. OM and SS wrote the manuscript, all authors contributed to editing.
Funding
This research was supported by a California State University Agricultural Research Institute grant (20-01-103) to SS.
Conflict of Interest
The authors declare that the research was conducted in the absence of any commercial or financial relationships that could be construed as a potential conflict of interest.
Publisher’s Note
All claims expressed in this article are solely those of the authors and do not necessarily represent those of their affiliated organizations, or those of the publisher, the editors and the reviewers. Any product that may be evaluated in this article, or claim that may be made by its manufacturer, is not guaranteed or endorsed by the publisher.
Acknowledgments
The authors wish to thank the sample farms for allowing us to study their lands, and Craig Stubler, Apollonia Arellano, and Sophie Aubry for their help with laboratory analyses.
Supplementary Material
The Supplementary Material for this article can be found online at: https://www.frontiersin.org/articles/10.3389/fenvs.2022.838455/full#supplementary-material
References
Anderson, J. P. E., and Domsch, K. H. (1978). A Physiological Method for the Quantitative Measurement of Microbial Biomass in Soils. Soil Biol. Biochem. 10, 215–221. doi:10.1016/0038-0717(78)90099-8
Badia, D., and Alcañiz, J. M. (1993). Basal and Specific Microbial Respiration in Semiarid Agricultural Soils: Organic Amendment and Irrigation Management Effects. Geomicrobiology J. 11, 261–274. doi:10.1080/01490459309377956
Bates, D., Mächler, M., Bolker, B., and Walker, S. (2015). Fitting Linear Mixed-Effects Models Usinglme4. J. Stat. Soft. 67, 1–48. doi:10.18637/jss.v067.i01
Bauer, M., Kube, M., Teeling, H., Richter, M., Lombardot, T., Allers, E., et al. (2006). Whole Genome Analysis of the marine Bacteroidetes 'Gramella Forsetii' Reveals Adaptations to Degradation of Polymeric Organic Matter. Environ. Microbiol. 8, 2201–2213. doi:10.1111/j.1462-2920.2006.01152.x
Birch, Q. T., Potter, P. M., Pinto, P. X., Dionysiou, D. D., and Al-Abed, S. R. (2020). Sources, Transport, Measurement and Impact of Nano and Microplastics in Urban Watersheds. Rev. Environ. Sci. Biotechnol. 19, 275–336. doi:10.1007/s11157-020-09529-x
Brodhagen, M., Goldberger, J. R., Hayes, D. G., Inglis, D. A., Marsh, T. L., and Miles, C. (2017). Policy Considerations for Limiting Unintended Residual Plastic in Agricultural Soils. Environ. Sci. Pol. 69, 81–84. doi:10.1016/j.envsci.2016.12.014
Corradini, F., Meza, P., Eguiluz, R., Casado, F., Huerta-Lwanga, E., and Geissen, V. (2019). Evidence of Microplastic Accumulation in Agricultural Soils from Sewage Sludge Disposal. Sci. Total Environ. 671, 411–420. doi:10.1016/j.scitotenv.2019.03.368
Culman, S. W., Snapp, S. S., Freeman, M. A., Schipanski, M. E., Beniston, J., Lal, R., et al. (2012). Permanganate Oxidizable Carbon Reflects a Processed Soil Fraction that Is Sensitive to Management. Soil Sci. Soc. America J. 76, 494–504. doi:10.2136/SSSAJ2011.0286
De Souza MacHado, A. A., Lau, C. W., Till, J., Kloas, W., Lehmann, A., Becker, R., et al. (2018). Impacts of Microplastics on the Soil Biophysical Environment. Environ. Sci. Technol. 52, 9656–9665. doi:10.1021/acs.est.8b02212
Doane, T. A., and Horwáth, W. R. (2003). Spectrophotometric Determination of Nitrate with a Single Reagent. Anal. Lett. 36, 2713–2722. doi:10.1081/AL-120024647
Fakour, H., Lo, S.-L., Yoashi, N. T., Massao, A. M., Lema, N. N., Mkhontfo, F. B., et al. (2021). Quantification and Analysis of Microplastics in farmland Soils: Characterization, Sources, and Pathways. Agriculture 11, 330. doi:10.3390/agriculture11040330
Fei, Y., Huang, S., Zhang, H., Tong, Y., Wen, D., Xia, X., et al. (2020). Response of Soil Enzyme Activities and Bacterial Communities to the Accumulation of Microplastics in an Acid Cropped Soil. Sci. Total Environ. 707, 135634. doi:10.1016/j.scitotenv.2019.135634
Figueroa-Gonzalez, P. A., Bornemann, T. L. V., Adam, P. S., Plewka, J., Révész, F., von Hagen, C. A., et al. (2020). Saccharibacteria as Organic Carbon Sinks in Hydrocarbon-Fueled Communities. Front. Microbiol. 11, 3343. doi:10.3389/FMICB.2020.587782
Hartmann, M., and Widmer, F. (2006). Community Structure Analyses Are More Sensitive to Differences in Soil Bacterial Communities Than Anonymous Diversity Indices. Appl. Environ. Microbiol. 72, 7804–7812. doi:10.1128/AEM.01464-06
Helmberger, M. S., Tiemann, L. K., and Grieshop, M. J. (2020). Towards an Ecology of Soil Microplastics. Funct. Ecol. 34, 550–560. doi:10.1111/1365-2435.13495
Horton, A. A., Walton, A., Spurgeon, D. J., Lahive, E., and Svendsen, C. (2017). Microplastics in Freshwater and Terrestrial Environments: Evaluating the Current Understanding to Identify the Knowledge Gaps and Future Research Priorities. Sci. Total Environ. 586, 127–141. doi:10.1016/j.scitotenv.2017.01.190
Huang, Y., Liu, Q., Jia, W., Yan, C., and Wang, J. (2020). Agricultural Plastic Mulching as a Source of Microplastics in the Terrestrial Environment. Environ. Pollut. 260, 114096. doi:10.1016/j.envpol.2020.114096
Huerta Lwanga, E., Gertsen, H., Gooren, H., Peters, P., Salánki, T., Van Der Ploeg, M., et al. (2016). Microplastics in the Terrestrial Ecosystem: Implications for Lumbricus Terrestris (Oligochaeta, Lumbricidae). Environ. Sci. Technol. 50, 2685–2691. doi:10.1021/acs.est.5b05478
Huerta Lwanga, E., Mendoza Vega, J., Ku Quej, V., Chi, J. d. l. A., Sanchez del Cid, L., Chi, C., et al. (2017). Field Evidence for Transfer of Plastic Debris along a Terrestrial Food Chain. Sci. Rep. 7, 1–7. doi:10.1038/s41598-017-14588-2
Hurley, S. (2008). Postconsumer Agricultural Plastic Report. Sacramento, CA: California Integrated Waste Management Board.
Islam, K. R., Stine, M. A., Gruver, J. B., Samson-Liebig, S. E., and Weil, R. R. (2003). Estimating Active Carbon for Soil Quality Assessment: A Simplified Method for Laboratory and Field Use. Am. J. Altern. Agric. 18, 3–17. doi:10.1079/AJAA2003003
Isles, P. D. F. (2020). The Misuse of Ratios in Ecological Stoichiometry. Ecology 101, e03153. doi:10.1002/ECY.3153
Jambeck, J. R., Geyer, R., Wilcox, C., Siegler, T. R., Perryman, M., Andrady, A., et al. (2015). Plastic Waste Inputs from Land into the Ocean. Science 347, 768–771. doi:10.1126/science.1260352
Jiang, X. J., Liu, W., Wang, E., Zhou, T., and Xin, P. (2017). Residual Plastic Mulch Fragments Effects on Soil Physical Properties and Water Flow Behavior in the Minqin Oasis, Northwestern China. Soil Tillage Res. 166, 100–107. doi:10.1016/j.still.2016.10.011
Kaiser, E. A., Mueller, T., Joergensen, R. G., Insam, H., and Heinemeyer, O. (1992). Evaluation of Methods to Estimate the Soil Microbial Biomass and the Relationship with Soil Texture and Organic Matter. Soil Biol. Biochem. 24, 675–683. doi:10.1016/0038-0717(92)90046-Z
Kuzyakov, Y., and Blagodatskaya, E. (2015). Microbial Hotspots and Hot Moments in Soil: Concept & Review. Soil Biol. Biochem. 83, 184–199. doi:10.1016/J.SOILBIO.2015.01.025
Lenth, R. V., Buerkner, P., Herve, M., Love, J., Riebl, H., and Singmann, H. (2021). Emmeans: Estimated Marginal Means, Aka Least-Squares Means.
Li, L., Luo, Y., Li, R., Zhou, Q., Peijnenburg, W. J. G. M., Yin, N., et al. (2020). Effective Uptake of Submicrometre Plastics by Crop Plants via a Crack-Entry Mode. Nat. Sustain. 3, 929–937. doi:10.1038/s41893-020-0567-9
Liang, Y., Lehmann, A., Yang, G., Leifheit, E. F., and Rillig, M. C. (2021). Effects of Microplastic Fibers on Soil Aggregation and Enzyme Activities Are Organic Matter Dependent. Front. Environ. Sci. 9, 1–11. doi:10.3389/fenvs.2021.650155
Lü, H., Mo, C.-H., Zhao, H.-M., Xiang, L., Katsoyiannis, A., Li, Y.-W., et al. (2018). Soil Contamination and Sources of Phthalates and its Health Risk in China: A Review. Environ. Res. 164, 417–429. doi:10.1016/j.envres.2018.03.013
Oksanen, J., Blanchet, F. G., Friendly, M., Kindt, R., Legendre, P., Mcglinn, D., et al. (2020). Vegan: Community Ecology Package, R Package Version 2, 3–1.
Piehl, S., Leibner, A., Löder, M. G. J., Dris, R., Bogner, C., and Laforsch, C. (2018). Identification and Quantification of Macro- and Microplastics on an Agricultural farmland. Sci. Rep. 8, 17950. doi:10.1038/s41598-018-36172-y
Pivnenko, K., Eriksen, M. K., Martín-Fernández, J. A., Eriksson, E., and Astrup, T. F. (2016). Recycling of Plastic Waste: Presence of Phthalates in Plastics from Households and Industry. Waste Manage. 54, 44–52. doi:10.1016/j.wasman.2016.05.014
Qi, Y., Beriot, N., Gort, G., Huerta Lwanga, E., Gooren, H., Yang, X., et al. (2020). Impact of Plastic Mulch Film Debris on Soil Physicochemical and Hydrological Properties. Environ. Pollut. 266, 115097. doi:10.1016/j.envpol.2020.115097
Qi, Y., Yang, X., Pelaez, A. M., Huerta Lwanga, E., Beriot, N., Gertsen, H., et al. (2018). Macro- and Micro- Plastics in Soil-Plant System: Effects of Plastic Mulch Film Residues on Wheat (Triticum aestivum) Growth. Sci. Total Environ. 645, 1048–1056. doi:10.1016/j.scitotenv.2018.07.229
R Core Team (2021). R: The R Project for Statistical Computing. Available at: https://intro2r.com/citing-r.html.
Ramos, L., Berenstein, G., Hughes, E. A., Zalts, A., and Montserrat, J. M. (2015). Polyethylene Film Incorporation into the Horticultural Soil of Small Periurban Production Units in Argentina. Sci. Total Environ. 523, 74–81. doi:10.1016/j.scitotenv.2015.03.142
Rhine, E. D., Mulvaney, R. L., Pratt, E. J., and Sims, G. K. (1998). Improving the Berthelot Reaction for Determining Ammonium in Soil Extracts and Water. Soil Sci. Soc. Am. J. 62, 473. doi:10.2136/sssaj1998.03615995006200020026x
Rillig, M. C., Lehmann, A., Souza Machado, A. A., and Yang, G. (2019). Microplastic Effects on Plants. New Phytol. 223, 1066–1070. doi:10.1111/NPH.15794
Ru, J., Huo, Y., and Yang, Y. (2020). Microbial Degradation and Valorization of Plastic Wastes. Front. Microbiol. 11, 442. doi:10.3389/fmicb.2020.00442
Sala, M. M., Arrieta, J. M., Boras, J. A., Duarte, C. M., and Vaqué, D. (2010). The Impact of Ice Melting on Bacterioplankton in the Arctic Ocean. Polar Biol. 33, 1683–1694. doi:10.1007/s00300-010-0808-x
Sanchez-Hernandez, J. C. (2019). Bioremediation of Agricultural Soils. Boca Raton, FL: CRC Press, Taylor & Francis Group. doi:10.1201/9781315205137
Scalenghe, R. (2018). Resource or Waste? A Perspective of Plastics Degradation in Soil with a Focus on End-Of-Life Options. Heliyon 4, e00941. doi:10.1016/j.heliyon.2018.e00941
Seeley, M. E., Song, B., Passie, R., and Hale, R. C. (2020). Microplastics Affect Sedimentary Microbial Communities and Nitrogen Cycling. Nat. Commun. 11, 1–10. doi:10.1038/s41467-020-16235-3
Selke, S., Auras, R., Nguyen, T. A., Castro Aguirre, E., Cheruvathur, R., and Liu, Y. (2015). Evaluation of Biodegradation-Promoting Additives for Plastics. Environ. Sci. Technol. 49, 3769–3777. doi:10.1021/es504258u
Sofo, A., and Ricciuti, P. (2019). A Standardized Method for Estimating the Functional Diversity of Soil Bacterial Community by Biolog EcoPlatesTM Assay-The Case Study of a Sustainable Olive Orchard. Appl. Sci. 9, 4035–4039. doi:10.3390/app9194035
Sprouffske, K., and Wagner, A. (2016). Growthcurver: an R Package for Obtaining Interpretable Metrics from Microbial Growth Curves. BMC Bioinformatics 17, 1. doi:10.1186/S12859-016-1016-7
Stefanowicz, A. (2006). The Biolog Plates Technique as a Tool in Ecological Studies of Microbial Communities. Polish J. Environ. Stud. 15 (5), 669–676.
Steinmetz, Z., Wollmann, C., Schaefer, M., Buchmann, C., David, J., Tröger, J., et al. (2016). Plastic Mulching in Agriculture. Trading Short-Term Agronomic Benefits for Long-Term Soil Degradation? Sci. Total Environ. 550, 690–705. doi:10.1016/j.scitotenv.2016.01.153
Viñas, M., Sabaté, J., Guasp, C., Lalucat, J., and Solanas, A. M. (2005). Culture-dependent and -independent Approaches Establish the Complexity of a PAH-Degrading Microbial Consortium. Can. J. Microbiol. 51, 897–909. doi:10.1139/w05-090
Wang, J., Lv, S., Zhang, M., Chen, G., Zhu, T., Zhang, S., et al. (2016). Effects of Plastic Film Residues on Occurrence of Phthalates and Microbial Activity in Soils. Chemosphere 151, 171–177. doi:10.1016/j.chemosphere.2016.02.076
Wardle, D. A., and Ghani, A. (1995). A Critique of the Microbial Metabolic Quotient (qCO2) as a Bioindicator of Disturbance and Ecosystem Development. Soil Biol. Biochem. 27, 1601–1610. doi:10.1016/0038-0717(95)00093-T
Weithmann, N., Möller, J. N., Löder, M. G. J., Piehl, S., Laforsch, C., and Freitag, R. (2018). Organic Fertilizer as a Vehicle for the Entry of Microplastic into the Environment. Sci. Adv. 4, eaap8060. doi:10.1126/sciadv.aap8060
Wolińska, A., Kuźniar, A., Zielenkiewicz, U., Izak, D., Szafranek-Nakonieczna, A., Banach, A., et al. (2017). Bacteroidetes as a Sensitive Biological Indicator of Agricultural Soil Usage Revealed by a Culture-independent Approach. Appl. Soil Ecol. 119, 128–137. doi:10.1016/J.APSOIL.2017.06.009
Yan, Y., Chen, Z., Zhu, F., Zhu, C., Wang, C., and Gu, C. (2020). Effect of Polyvinyl Chloride Microplastics on Bacterial Community and Nutrient Status in Two Agricultural Soils. Bull. Environ. Contam. Toxicol. 107, 602–609. doi:10.1007/S00128-020-02900-2
Zhang, D., Ng, E. L., Hu, W., Wang, H., Galaviz, P., Yang, H., et al. (2020). Plastic Pollution in Croplands Threatens Long‐term Food Security. Glob. Change Biol. 26, 3356–3367. doi:10.1111/gcb.15043
Keywords: soil plastic pollution, plastisphere, macroplastic, soil hotspot, agricultural plastic
Citation: McKay O, Pold G, Martin P and Sistla S (2022) Macroplastic Fragment Contamination of Agricultural Soils Supports a Distinct Microbial Hotspot. Front. Environ. Sci. 10:838455. doi: 10.3389/fenvs.2022.838455
Received: 17 December 2021; Accepted: 20 January 2022;
Published: 15 February 2022.
Edited by:
John Scott, University of Illinois, United StatesReviewed by:
Jie Wang, China Agricultural University, ChinaAgnieszka Klimkowicz-Pawlas, Institute of Soil Science and Plant Cultivation, Poland
Copyright © 2022 McKay, Pold, Martin and Sistla. This is an open-access article distributed under the terms of the Creative Commons Attribution License (CC BY). The use, distribution or reproduction in other forums is permitted, provided the original author(s) and the copyright owner(s) are credited and that the original publication in this journal is cited, in accordance with accepted academic practice. No use, distribution or reproduction is permitted which does not comply with these terms.
*Correspondence: Seeta Sistla, c3Npc3RsYUBjYWxwb2x5LmVkdQ==
†Present Address: Grace Pold, Department of Forest Mycology and Plant Pathology, Swedish Agricultural University, Uppsala, Sweden