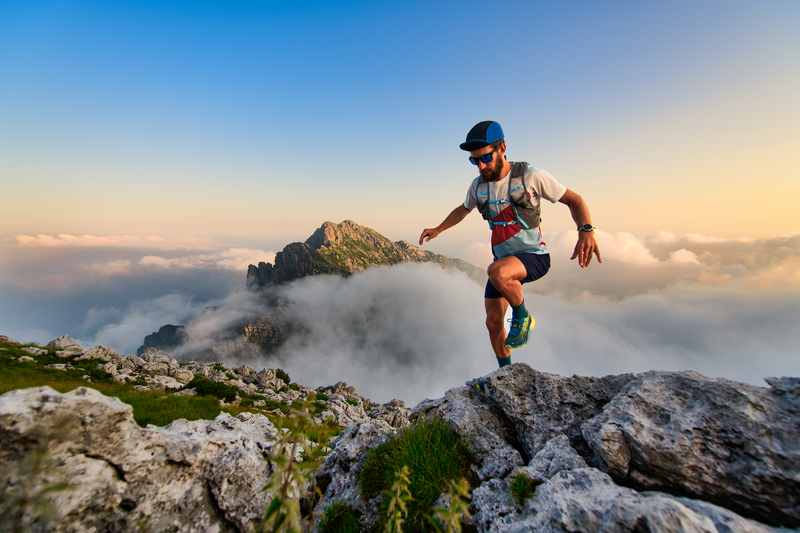
95% of researchers rate our articles as excellent or good
Learn more about the work of our research integrity team to safeguard the quality of each article we publish.
Find out more
ORIGINAL RESEARCH article
Front. Environ. Sci. , 25 May 2022
Sec. Biogeochemical Dynamics
Volume 10 - 2022 | https://doi.org/10.3389/fenvs.2022.829900
The release of potentially harmful elements (PHEs) into the environment in mineralised and mining areas has been associated with a variety of health-related disorders, especially non-communicable diseases such as cancer, heart and kidney failure and mental and cardiovascular disorders. The present study sought to evaluate the application of geochemical indices in assessing the degree of contamination at two sites, Kadoma and Hurungwe, both within the Sanyati Catchment, an important mining and agricultural hub in Zimbabwe. This evaluation was conducted by determining the concentration levels of 16 PHEs in 58 top-soil and stream sediment sample locations. The samples were collected during the period 2015–2017 and analysed for total PHE content using Inductively Coupled Plasma Mass Spectrometry (ICP-MS). To assess the degree of contamination of the soils and stream sediments, contamination indices were computed and the potential ecological risk to the area was evaluated. A correlation analysis revealed PHE associations as strongly influenced by lithology and Au mineralisation in the Kadoma setting. Assessment of multi-element contamination using the pollution load index revealed significant contamination in 52% of the soil sample sites and 38% of the sediment sample sites in Kadoma. The results indicate that As, Cr, Mo, and Sb are the main contaminant PHEs in the Kadoma site. Potential ecological risk ranged from moderate to very high at 71% of soil sample locations and 53% of sediment locations and the key contributors were Sb, As, and Hg. At the Hurungwe site, Cr and Mo were found to be key contaminants with a low potential ecological risk for all samples. This study demonstrates the successful application of geochemical indices in evaluating the degree of single and multi-element contamination as the first step toward a human health risk assessment in mining environments. It is expected that these results would assist municipal authorities in their effort to formulate credible mitigative measures to protect the health of nearby residents and surrounding ecosystems and make an informed decision regarding land use planning and post-mining rehabilitation of contaminated land at mining centres.
Geochemical mapping is useful in identifying regions of unusually high concentrations of chemical elements on or below the Earth’s surface and is applied in both mineral exploration and environmental studies. Anomalous levels of an element can signify a potential mineral deposit or the impact of anthropogenic contamination (Salomão et al., 2021). Some of these chemical elements can be found at potentially toxic levels, raising concerns for human health. The potentially harmful elements (PHEs) are derived from rocks, soils, natural waters and the atmosphere; these are environmental media, where their content varies depending on natural biogeochemical processes and anthropogenic perturbations in which they are involved throughout their environmental cycling. Soils, especially those found at or near the metalliferous mines and metal smelters, are often found to be highly contaminated with PHEs such as As, Cd, Cr, Cu, Pb, Ni, and Zn (Boularbah et al., 2006; Qu et al., 2012; Weissmannová et al., 2019). Over the years surface soils and stream sediments become contaminated through the effect of improper disposal of mining and other industrial wastes. The net result of such large-scale pollution is a heavy toll on human health as PHEs are transferrable through various pathways into the human food chain (Davies, 2013; Finkelman et al., 2018). It is thus becoming increasingly necessary for studies to be conducted to evaluate the level of pollution by PHEs from both geogenic and anthropogenic sources and to quantify the risk they pose to human health.
The mining areas in Zimbabwe are no exception to the accumulation of PHEs. A study on the challenges posed by the widely distributed mine tailings within the Zimbabwe mining landscape revealed that Sb, Cu, Co, Zn, Ni, As, and Pb may be high polluters in most areas in the vicinity of these mine wastes (Meck, 2013). Other studies in Zimbabwe have charted the dispersion of PHEs due to mining and reiterated the negative impacts this has on the environment (Ashton et al., 2001; Lupankwa et al., 2004; Tunhuma et al., 2004; Ravengai et al., 2005). However, there is a paucity of studies in Zimbabwe that have used geochemical indices or conducted evaluations of the potential ecological risk posed by anomalous concentrations of PHEs in soils or stream sediments. Examples of studies that have investigated human health impacts associated with geogenic or mining-related PHEs in Zimbabwe include those on the impact on local communities of anomalous levels of fluoride in drinking water leading to dental fluorosis (Mamuse and Watkins, 2016); the health impacts of the continued use of mercury (Hg) in gold (Au) processing by artisanal miners in Zimbabwe (Steckling et al., 2014; Becker et al., 2020; Bose-O’Reilly et al., 2020; Mambrey et al., 2020) and investigation of the impacts of As on human health at Cam and Motor mine (Mossop, 1989). Other studies conducted focused on the health risk of PHEs concerning human exposure through dietary intake (e.g., fish, animal products, crops or vegetables) (Muchuweti et al., 2006; Nharingo et al., 2015; Kanda et al., 2020). However, none of these studies have included an evaluation of geochemical signatures of the study areas nor the individual or combined potential ecological risk posed by the PHEs in the soils and stream sediments. The link between geology, soil and biota (humans included) is an important one as rocks and minerals sourced from the Earth’s crust are the basic “building blocks” of all life forms providing the major, minor and trace elements that are needed for health in humans and animals (Dissanayake, 2005; Finkelman and Centeno, 2020). PHE contaminants can affect human health through a variety of ways such as primary consumption (e.g., drinking water), or elemental biomagnification through the food chain, or through direct skin contact, inhalation (of dust and aerosols), or intended (geophagy), or unintended (ingestion of dirt or soil by children). Despite the challenges associated with identifying the aetiology, there are diseases and conditions such as tooth decay, dental and skeletal fluorosis, goitre and other iodine deficiency disorders, keratosis, Keshan disease, asbestosis, pneumoconiosis, silicosis, cardio-vascular diseases, renal failure, mesothelioma and a variety of other cancers that have been linked to either high levels of potentially toxic elements or non-optimal levels of certain essential elements and minerals that are found naturally occurring in the geological environment (Centeno et al., 2016; Finkelman et al., 2018). The current study aims to provide a model on how to fill this void in evaluating gold mining environments, which Zimbabwe is well endowed with and whose exploration and mining have been the country’s key economic drivers for centuries.
Geochemical indices are ratios of elements in an environment of concern and background reference concentrations, and have been used to evaluate soil physical and chemical development over geologic time (Mikkonen et al., 2016; Heidari et al., 2022). In environmental studies, the most commonly used soil contamination assessment methods can be assigned to two groups, namely quantitative and qualitative (Wu et al., 2014). The quantitative methods involve the evaluation of each PHE concentration in the site under investigation against a reference value. This reference value is also known as a geochemical background, baseline, or “normal” value and has been determined through investigations on the composition of the Earth’s crust (e.g., Turekian and Wedepohl, 1961; Taylor and McLennan, 1985; Rudnick and Gao, 2003) or by using various analytical and or statistical techniques at regional and national scales (Ander et al., 2013; Zhao et al., 2013; Hao et al., 2014; Kicińska and Turek, 2017). Examples of quantitative methods include geo-accumulation index (Igeo) and contamination factor (CF) which are singles indices. Complex indices can also be derived from them to assess the overall degree of contamination of the soil at a site. The quantitative contamination indices allow the comparative evaluation of contamination within study sites and against studies conducted by other researchers. However, due to the variations in the reference levels used, to determine credible thresholds for the different contamination categories it is often prudent to use the indices in combination to get a balanced interpretation of the extent and degree of contamination (Wu et al., 2014; Niu et al., 2021). Other methods involve multivariate analyses that employ various assumptions on the data distribution (normal or non-normal) of the variables under study and these include amongst others principal component analysis (PCA), correlation analysis and cluster analysis (Wu et al., 2014). Though these methods have been referred to as qualitative methods Wu et al. (2014) due to the generation of descriptive groupings, they still depend on quantitative data and are often used to investigate the correlations among contaminants and contaminant sources (Weissmannová et al., 2019; Rinklebe et al., 2019; Niu et al., 2021).
The analysis of PHE concentrations in soils can be used in generating an ecological risk index which is a quantitative measure of the ecological risk of a contaminant in a given system, thus, it constitutes a useful tool in pollution control (Hakanson, 1980; US EPA, 2002; Kumar et al., 2020). The behaviour of PHEs that enter the body (whether toxic or beneficial) is determined by several geochemical and biochemical factors engendering their (PHEs) transfer right from the source (e.g., soil particle size distribution, pH and organic matter content) via various migration pathways (mobility characteristics) into the body (e.g., chemical form and bioavailability), where they (PHEs) are involved in various metabolic processes (Antoniadis et al., 2019; Rinklebe et al., 2019). Tracking the fate of PHEs from their release, movement through the environment and their uptake and measurement as well as monitoring their levels in plants and other organisms is a mammoth task. Soil contamination indices have therefore been used successfully in evaluating the degree of contamination of a site, forming the basis for human health risk assessments associated with soil exposure (Liu et al., 2017; Proshad et al., 2018; Weissmannová et al., 2019; Rinklebe et al., 2019; Yu et al., 2021).
The main aim of this study was to evaluate the usefulness of geochemical indices in assessing the extent of single and multi-element contamination by PHEs at mining centres in the Sanyati Catchment and to ascertain if the overall degree of contamination by computing a Pollution Load Index (PLI). Through determination of an ecological risk index the study site was also assessed for PHEs that pose a threat to environmental and public health and correlation analysis used to identify potential PHE sources.
The Sanyati Catchment is one of the seven river catchments in Zimbabwe covering an area of approximately 7,300 km2 and administratively straddles three provinces, Mashonaland West, Midlands and Mashonaland East. The catchment is host to some of the largest, richest and most productive gold mines hosted in the oldest historic Archaean greenstone belts, i.e., key gold mining zones of the country (Ashton et al., 2001; Ravengai et al., 2005). The term “greenstone belt” as used here describes deformed and metamorphosed exceptionally thick volcano-sedimentary successions, generally enveloped by granitic and gneissic rocks, and confined to the Archaean, which ranges in age from the Eoarchaean (>3,600 Ma) to the Neoarchaean (ca. 2,500 Ma) (Anhaeusser, 2014) (Figure 1). Greenstone belts are known to be extremely varied in their lithological composition, including a diverse range of volcanic, plutonic and sedimentary units that are structurally complex having undergone multiple phases of deformation, metamorphism and metasomatic alteration as well as successive intrusions (Anhaeusser, 2014). These ultra-mafic to mafic and volcanic lithological units are associated with varied mineralisation that includes the occurrences of Cr, Ni/Cu, chrysotile asbestos, Au/Ag, ores of Fe, magnesite, talc and barite, Cu-Zn (+/− Cu, Sb, Hg, Ag, As, W), as well as lesser amounts of pyrite—pyrrhotite and corundum. The large Cam and Motor mine in Kadoma was historically both a Au and Sb mine and other Sb deposits are found within the Kadoma area. The sedimentary units also found here, host Fe ore, barite, Au, and limestone while the granites are associated with pegmatites and may host Sn, corundum, emeralds, beryl, mica, bismuth, Mo and Ta-Nb (Anhaeusser, 2014).
FIGURE 1. Overview of the geology of the HUR and KAD areas showing details of the varying lithology for the sites.
The catchment comprises 10 sub-catchments and is classified as semi-arid receiving approximately 635 mm of rainfall per annum over a wet summer season that runs from November to April and is characterized by annual mean maximum and minimum temperatures of approximate 28–33°C and 19–9°C in November and June respectively (Davis et al., 2014; Mashizha et al., 2017; Chanza and Gundu-Jakarasi, 2020). The key economic activities within the catchment include mining, mineral processing and agriculture. The Major rivers that drain into Sanyati River are Munyati, Muzvezve, Sebakwe, Kwekwe and Mupfure. The major towns within this catchment are Kwekwe, Kadoma, Chegutu, Gokwe, Chivhu and Mvuma. The two study sites selected for sampling are within two districts of Hurungwe and Kadoma (Figure 2).
All the mining activities at the Kadoma site (KAD) are situated within commercial and resettlement agricultural areas which have a tradition of being the leading producers of cotton and significant quantities of maize (Shoko and Veiga, 2004). Thriving small and medium scale horticultural activities are also hosted within these mining areas making use of the highly fertile soils and floodplains. The second site examined in this study is in the Hurungwe (HUR) District whose geological location is within the Proterozoic Magondi Belt and lies southeast of the town of Karoi. This site spans an area of approximately 24 km by 18 km, is characterised by granitic-gneiss lithology (Figure 1), and has been minimally impacted by large-scale mining and related activities since it is virtually bereft of economically viable sulphide gold ore deposits as found in KAD. The granites of HUR have been identified as hosts of pegmatites and much like those of greenstone belts and as such some beryl deposits have been identified in HUR. The closest gold and base metal mines at the HUR site are located beyond a 40 km radius from the study site making it a suitable control for this study concerning the absence of ore rock mining-related activities. There is however informal alluvial gold mining in HUR, with key informal gold panning sites along the Sanyati River as it passes through the town of Magunje (Manjengwa et al., 2012).
The evaluation of the degree of soil contamination by the PHEs (As, Ba, Be, Cd, Co, Cr, Cu, Fe, Hg, Mn, Mo, Ni, Pb, Sb, V, and Zn) was done by: 1) systematically collecting soil and sediment samples and analysing their PHE content, 2) calculating soil contamination (geochemical) indices, 3) spatially assessing the pollution load index 4) computing an ecological risk index of the study area and 5) evaluating the possible sources of the PHEs through a correlation analysis.
Stream catchments were selected at each of the sites, HUR and KAD using geological and topographic maps in QGIS to ensure representation of the diversity of lithological units of the sites. Actual sample locations were confirmed using a handheld Garmin e-Trex 10 GPS. Sample sites are displayed in Figure 2. Soil samples were taken using a hand auger (2.5 cm in diameter), each composite (approximately 1 kg) per sample location comprised of only the upper soil horizons (A and B; from a depth of 0 to approximately 25 cm) representing the root zone, and therefore the uptake zone for nutrients and PHEs by plants. Material from the organic-rich layers, where present, was excluded. Stream sediment samples were collected from areas of active sedimentation along the river channel at a distance from the edge to minimise contamination and bulked to one composite sample (approximately 1 kg). The soil and stream sediment samples were disaggregated and wet sieved (2 mm) at the Geochemical Laboratory of the Geology Department at the University of Zimbabwe. The soil samples were then air-dried and sent to the Performance Laboratories at Ruwa, Zimbabwe, where they were pulverised into a fine powder. The samples were then packed into small 10–15 g packets before sending for analysis. Sampling campaigns were conducted during the period November 2015 to March 2017 ensuring repeat samples were collected at each site and gathering a total of 174 soil and stream sediment samples from 58 locations in KAD and HUR. Multiple sampling campaigns were considered a necessity for monitoring the spatial and temporal (seasonal) variation of the pollution plumes, as such knowledge would aid in the formulation of mitigative measures.
Further processing and analyses of samples took place at the Bureau Veritas Minerals (BVM) Laboratories in Canada. The prepared samples were digested with a modified Aqua Regia solution of equal parts concentrated hydrochloric acid (HCl), nitric acid (HNO3) and deionized water (DI H2O) for 1 hour on a heating block or hot water bath. Each sample was made up to volume with dilute HCl and analysed for 53 elements by the ICP-MS method for soils and stream sediments. The detection limits for the elements considered in this paper were: 1% for Fe; 0.01% for Al; 1 ppm for Mn; 2 ppm for V; 0.5 ppm for Ba and Cr; 0.1 ppm for As, Be, Co, Ni, and Zn; 0.02 ppm for Sb; 0.01 ppm for Cd, Cu, Mo, Pb; and 5 ppb for Hg. For quality control purposes and provision of an effective framework for interpreting the ensuing geochemical datasets, duplicate samples were randomly inserted in the laboratory and blind duplicate samples were also included with each batch sent. Method blanks and certified reference materials, STD DS10 and STD OREAS45EA (for soils and stream sediments), were determined intermittently during the analyses of the samples to monitor background or reagent contamination and interferences. The relative standard deviation (RSD) for a combination of lab duplicates and blind duplicates in the soil and sediment samples varied from 0.3% to 10% for all PHEs analysed with Cd recording a RSD of 20%–23%. The standard errors for the measurements in the soil samples were in the range of 0.01–5.
Data cleaning was conducted in Microsoft Excel and analysis was performed in R (RStudio Team, 2021). Initial data cleaning involved handling data falling below the limit of detection (LOD) or left-censored data (See, e.g., Canales et al., 2018). Left-censored values were replaced using the simple method of substituting with LOD/2, a well-established method in geochemical studies (See: de Caritat and Mann, 2018). Standard statistical procedures followed in this study involved the calculation of several descriptive statistical parameters, arithmetic mean, standard deviation (SD), minimum (Min), median (Med) and maximum (Max), construction of graphs (box plots, scatter plots and quantile-quantile (Q-Q) plots. The data for this study were evaluated for normality and were found not to follow normal distribution owing to a large number of outliers. As the outliers were deemed important to this study they were not removed from the data for the bulk of the analysis and instead robust non-parametric statistical techniques were utilised (Schober and Schwarte, 2018; Statistics Solutions, 2019; Sorensen et al., 2021). To assess the significance of the differences in PHE levels in the two geological terranes, the results also were subjected to the Mann-Whitney-Wilcoxon Test. The degree of association between PHE levels within each site was evaluated with the Spearman’s rank correlation test.
Evaluation of the contamination of the soils and stream sediments was performed using a select set of geochemical indices, namely: Igeo, CF and the Tomlinson Pollution Load Index (PLI) (Tomlinson et al., 1980; Mortazavi et al., 2017; Tchounwou et al., 2019; Niu et al., 2021). In this study upper continental crust values as determined by Rudnick and Gao (2003) were used as the geochemical background values and in mg/kg these are: As: 4.80; Ba: 628, Be: 2.1; Cd: 0.09, Co: 17.3, Cr: 92, Cu: 28, Fe: 50,400, Hg: 0.05, Mn: 1,000, Mo: 1.1, Ni: 47, Pb: 17, Sb: 0.4, V: 97, and Zn: 67. For comparison, the results of the low-density regional geochemical survey as determined by Zhao et al. (2013) were also used in the computation of CF as background reference values for Zimbabwe. These in mg/kg are As: 4.22; Ba: 533, Be: 0.99; Cd: 0.07, Co: 10.48, Cr: 529, Cu: 16.82, Fe: 27,500, Hg: 0.02, Mn: 392.7, Mo: 0.41, Ni: 41.52, Pb: 17.94, Sb: 0.52, V: 62.1, and Zn: 26.1.
The Igeo was proposed by Müller (1969) to determine metal contamination in sediments by comparing current concentrations with pre-industrial levels and has since been used in several environmental pollution studies (Zhang et al., 2013; Meng et al., 2020; Niu et al., 2021). In the absence of pre-industrial values for the current study, continental crust values were utilized in Igeo calculation (Rahman et al., 2012). The Igeo was calculated as in Eq. 1 for which Müller (1969) distinguished seven classes of Igeo (0–6), as provided in Table 1.
TABLE 1. Classification of contamination levels based on calculated geochemical indices [Adapted from Barbieri (2016), Wu et al. (2014)].
The CF (Eq. 2) represents element input from anthropogenic sources. It relates the contamination of elements in the soil and sediment with geochemical background values (Balkhair, 2016; Kicińska and Turek, 2017; Rinklebe et al., 2019; Kumar et al., 2020).
For the Eqs 1, 2 Cn is the concentration of an element in the sample (n); Bn is the concentration of the same element in the background and a correction factor of 1.5 accounts for the variability of the background value in calculating Igeo (Kicińska and Turek, 2017).
The Tomlinson Pollution Load Index (PLI) is one overall contamination index of a sample and has been used often to assess the quality of soil in terms of contamination (Kicińska and Turek, 2017; Proshad et al., 2018; Rinklebe et al., 2019). The PLI is the nth root (depending on the number of potential contaminants taken into consideration) of the product of CF (calculated using Eq. 4). If PLI > 1, samples are considered significantly contaminated (Rinklebe et al., 2019).
Where CFs,1 × CFs,2, CFs,n are the CF of the elements 1, 2 ....n (Rinklebe et al., 2019). A PLI value equal to zero indicates non-polluted; a value of 1 indicates the presence of only a baseline level of pollutants and values above 1 indicate progressive deterioration due to trace element contamination (Rashed, 2010 as cited in Proshad et al., 2018). In this study, PLI was used in generating an interpolated map in QGIS to allow spatial interpretation of the impacts of the multi-element contamination.
The contamination indices computed were used to evaluate the degree of contamination in the study sites and to compare the present study results with those of other national and international investigations. For ease of comparison of the level of enrichment or degree of contamination computed through each index, the values were categorized into five classes (Table 1) as proposed in Wu et al. (2014) with some adaptations using other classifications (Barbieri, 2016). Classification thresholds indicated on the boxplots are the upper boundaries of the “Uncontaminated”, “Considerably contaminated” and “Extremely contaminated” classes.
The quantitative expression of the potential ecological risk posed by an individual element in a sample, defined by Hakanson (1980) as an ecological risk factor (ER also expressed in the equation as
In these Eq. 4 and Eq. 5,
TABLE 2. Terminology for the interpretation of Ecological risk factor
Statistical summaries and concentration levels of the PHEs considered in this study and of concern regarding human health are presented in Figure 3. The median values for total element concentrations were higher for all PHEs assessed on both KAD stream sediment and soil samples than in HUR except for Cr and Mo. Total element concentration was highest for As in KAD soils –reaching a maximum of 2,000.9 mg/kg, (Table 3). In the KAD sediments As reached a maximum of 178.4 mg/kg. In the KAD sediments, the highest total concentration was obtained for Mn at 3,645 mg/kg. The Mann-Whitney-Wilcoxon test showed that there is no significant difference between the two sites of KAD and HUR for the elements Ba, Be, Cd, Cr, Fe, Mn, Ni, Pb, and Zn in stream sediments (p > 0.05), while for the soils it was only Ba and Pb levels that were not significantly different (Table 4). The local geochemical signatures which characterise the KAD soils were markedly influenced by the diverse lithology and mineralisation of the greenstone belt described earlier and therefore could be considered as deriving from distinctly different terranes when compared to the granitic-gneiss lithology of HUR. Contaminant transport through river channels, erosion and dispersion or translocation of surface material through the river networks were evidenced by the greater similarities of PHE content resulting in 56% of the sediment samples in KAD and HUR not showing significant differences in their PHE levels, compared to 12.5% of soil samples.
FIGURE 3. Log transformed concentrations of potentially toxic elements in surface soil and stream sediment samples of the KAD and HUR domains.
TABLE 3. Descriptive statistics for PHE concentrations (mg/kg) in stream sediments and soil samples from the two domains of Hurungwe (HUR) and Kadoma (KAD) compared against Zimbabwe Levels (Zhao et al., 2013) Screening Levels for Residential Soil (SL) and Freshwater sediments Toxicity Reference Values (TRV) (US EPA, 1999).
TABLE 4. Summary of the p-values obtained from Mann-Whitney-Wilcoxon (MWW) Test used to verify the similarities in the distribution of the PHEs in HUR and KAD.
The average PHE concentrations in soils and sediments were evaluated against results from an earlier national geochemical study (Zhao et al., 2013). The current results show a similar pattern to those of the national geochemical mapping of Zimbabwe where high average concentration values in KAD are observed for those PHEs that are ore-forming elements, i.e., Cr, Ni, and Cu as well as those having potential for ore-formation, such as Pb, Zn, Hg, Sb, and Mo. The sediment samples gave values for the elements Mo, As, Sb, Hg, Cu, Co, Ni, and Mn that were above the national geochemical averages. In the HUR soil and sediment samples, only Mo exceeded the national average levels (by at least 20 times), while all other PHEs fell below the means.
The PHE levels in soil samples were also evaluated against the USEPA Regional Screening Levels (SL) using the Target Hazard Quotient (THQ) of 0.1 (US EPA, 2021) provided in Table 3. Results show that average As, Ni, Co, Mn, and V exceeded the screening levels (SLs) by 13,300%, 874%, 265%, 8,500%, and 183%, respectively for the KAD soil samples. An inspection of the maximum concentrations for these PHEs indicates a much higher exceedance of the SL in some localities. In HUR no soil samples exceeded the SL. For the sediment samples, a comparison was made with the Freshwater Sediment Toxicity Reference Values (TRV) developed as a guideline for assessing sediment contamination (US EPA, 1999). The following elements exceeded the TRV in KAD: Cr, As, Ni, and Cu by 1,038%, 494%, 306%, and 136%. In HUR Ba, Cr, and Ni levels exceeded the TRV by 535%, 1,300%, and 156%. Concentrations above SLs and TRV are an indication of levels that warrant further site-specific investigations for human health risks (US EPA, 2021).
The results of the average and maximum Igeo computations presented in Table 5 show distinct variations in the accumulation of PHEs in the two study sites. The Igeo values for the KAD soil and sediment samples reveal contamination levels of Igeo > 0 for a bulk of the samples for As, Sb, Cr, and Mo, as well as a varying proportion of samples (the upper 25%–50%) for Cu and Hg. The high anomalous Igeo values for As and Sb in soils and sediments at certain locations were classified as highly to extremely contaminated. The PHEs Ba, Be, Cd Co, Fe, Mn, Ni, Pb, V, and Zn were classified as uncontaminated (negative Igeo) in the bulk of the KAD sediment and soil samples.
Average Igeo values at HUR revealed Mo and Cr as contaminants in the soil and sediment samples at moderately to considerably contaminated levels ranging from 0.57 to 3.16. The Igeo for the HUR sediments, however, show outlier values of Sb, As, and Ni at 0.76, 0.28, and 0.08 respectively, falling in the moderately contaminated group. The occurrence of outlier sediment samples with Igeo > 0 values of Sb, As, and Ni that are not reflected in the soil samples suggests PHE translocation through the river system from upper portions of the HUR catchment. The translocation of PHEs from source has been reported in other studies to be in the order of hundreds of kilometres in rivers with high discharge flow rates, with changes in water chemistry aiding dissolution and precipitation and flooding events determining their eventual distribution in floodplains (Du Laing, 2009 as cited in Rinklebe et al., 2019).
The KAD results (Figure 4) show CF values for As and Sb that range from considerably to extremely contaminated in both the soil and sediment samples when evaluated against the upper continental crust reference values (Rudnick and Gao, 2003). Moderate to considerable contamination of a large proportion of samples is also observed for Cr, Cu, Hg, Mo, Ni, and V. A small proportion (about 25%) of the samples also show moderate contamination of Cd, Fe, Mn, Pb, and Zn. The samples were classified as uncontaminated for the rest of the PHEs. A comparison was made of the CF values obtained using the geochemical background values as determined for Zimbabwe (Zhao et al., 2013). These local background values were deemed more representative of the local geochemical background levels. The CF values for the KAD samples for As were similar to those computed earlier with the Rudnick and Gao (2003) references, while Sb classification fell slightly below the “moderate to considerable contamination” group and extreme contamination was observed for Mo in both soils and sediments. The CF value for Cr in all samples was in the uncontaminated class. This impact on CF values can be attributed to Zimbabwe’s vast mineralised regions particularly the auriferous geochemical domains and the Great Dyke with huge deposits of Cr and platinum group metals influencing the geochemical background values.
FIGURE 4. Box and whisker plots illustrating the single pollution indices Igeo (A), and CF of PHE in soils (B) and stream sediments (C). Outliers were not plotted to aid visualisation.
For the HUR area, only 2 PHEs Mo and Cr showed CF values above 1 with the upper continental crust value and the rest did not indicate any contamination in this site. Further inspection of the data on CF reveals that at certain sample locations in the sediment outlier values of As, Cu, Ni, Sb, and V also had CF > 1 values while in soils, Ni was the only other PHE showing the same.
The categories of the degree of contamination that emerged through the computation of CF were mostly comparable with those of the Igeo revealing similar categories for the contamination levels of As, Cr, Mo, and Sb. The difference, however, was that of Cr and Mo classification when CF was computed from the national geochemical background values of Zhao et al. (2013).
Results of PLI at several sample locations in KAD were >1 (See Figure 5) indicating significant contamination in 52% (11 out of 21) of the soil sample sites and 38% of the sediment samples sites (n = 13). The heatmap for the soil PLI values shows that the effect of multi-element contamination was highest at the location K4 (2.24) followed by K5 (1.64), both these locations being within 500 m of active gold mining operations. Other locations with PLI > 1 that show clear associations with active gold mining, mine waste dumps and milling operations, and potential point pollution sources, were: K1, K2, K3, K6, K10, K13, K17, and K20. An inspection of the CF values for each contributing PHE shows Cr and Mo to be consistently large contributors to all PLI values in both study sites. In the KAD sediments As and Sb were the next largest contributors of high PLI values for the soil samples of which they contributed approximately 50%. Location K18 falls within a residential area without a mining operation in its vicinity, though it exhibited a PLI > 1. A more equitable contribution of other PHEs was also observed (especially Cr > Se > Mo > Ni > Cu > Co > Cd > Zn) with As and Sb now only contributing 32% towards the PLI. The PLI value at sample location K20 was also not dominated by As and Sb for which their CF contributed only 15% to the total. An inspection of the CF values for each contributing PHE shows As and Sb as consistent and the largest contributors of high PLI values for the soil samples in which they contributed approximately 50%.
FIGURE 5. Heat map of the PLI values for the total PHEs in soil (above) and sediment (bottom) samples at the KAD site. The red colour ramp was used to indicate the intensity of the PLI and the PLI levels at each location are highlighted in yellow. K* represents the sample location ID.
The heatmap for the sediments (Figure 5) revealed a different pattern with fewer locations having PLI > 1. The highest values of PLI are at KI (1.87), K3 (1.86), and K5 (1.24) all of which are located downstream of the key mining and mineral processing operations in the area known as Eiffel Flats in which one of the country’s largest historically mined gold deposit is hosted (Cam and Motor Mine). At the locations in the southern part of the KAD area, K13 and K14 PLI > 1 are also attained. However, at these locations, As and Sb did not dominate in their contributions to PLI, only contributing 17% and 31% combined, while other elements particularly Hg show more prominence contributing 33% (K13) and 31% (K14), respectively. Spatial analysis of the satellite image indicates that locations K13 and 14 are downstream of gold mines where unrecovered slimes dams can still be seen, and are also popular informal small scale mining areas where the use of Hg in extracting gold is very common. The PLI values in the HUR site did not show any results above unity for any locations.
The maximum PLI value reached in the KAD site was 2.24 in soil samples, a relatively high value which is evidence of significant multi-element contamination, a phenomenon commonly associated with historically heavily industrialised sites (Rinklebe et al., 2019). This PLI value was compared to those obtained in other studies. In a study conducted on agricultural floodplain soils across the Central Elbe River in Germany (amongst the most highly PHE-polluted rivers in Europe due to a history of intensive industrial activity), the median topsoil PLI was 1.73 and a maximum of 3.20 (Rinklebe et al., 2019). The max KAD PLI value fell between this range signifying the comparatively high degree of contamination. An investigation of the degree of contamination of sediments in a stretch of the Subarnarekha River, India, passing through an important industrial hub, Jamshedpur city, found PLI values ranging from 0.436 to 6.92 in winter and summer respectively (Banerjee and Kumar, 2016). Previous studies of Subarnarekha River confirmed contamination and bioaccumulation problems in phytoplankton, molluscs and fish associated with this level of contamination (Banerjee et al., 2015).
The ER values generated in the assessment of the sediment and soil samples for the 13 PHEs for which toxic risk factors were available, show that for both sediments and soil samples Sb, As, and Hg, in the KAD sediment and soil samples surpass low-risk levels, Cd levels in both KAD and HUR closely approach the upper boundary of the low potential ecological risk category, while the rest of the elements studied fall below this level (Figure 6). Potential ecological risk for Sb at some locations, such as K3 and K15 in sediments, were between considerable to high risk, while in sample K1 a value in the very high-risk category was reached (See Supplementary Tables S1, S2 for the potential ecological risk factor and risk index at each sample location). Results of the potential ecological RI in both soils and sediments in KAD indicate considerable risk and those of HUR showed low risk. The results indicate that 71% of the KAD sediment and 53% of the soil sample locations show between low and considerable risk. Sediment samples from locations K1 and K3, both located along tributaries within 5 km downstream of the large Cam and Motor Mine and the Empress Nickel refinery, indicated very high RI values made up from mostly As and Sb contributions. Locations K15, K14, K13, K5, are at considerable risk, where Hg is the largest or significant contributor to risk. K12 shows moderate risk while the rest of the KAD sediments show low risk. In the KAD soils, RI at locations K4 and K5, indicates very high-risk levels, both influenced by anomalously high ER values for As. All the sediment and soil locations in HUR are in the low RI category.
FIGURE 6. Potential ecological risk factors for PHEs analysed in KAD and HUR sediments and soils. Intercepts are placed at 60, 80, 160, and 320 upper boundaries for low, moderate, considerable and high risk. Y-axis has been modified to max 500 so higher outliers in soil are omitted.
A Spearman Rank correlation analysis was applied to reveal the strength of associations of PHEs in the soils and stream sediments of the study sites and to evaluate the possible similarity of origins. The correlation coefficient (r) can be divided into four correlation classes as r ≤ 0.1 low, r in the range of 0.1–0.3 medium, r in the range of 0.3–0.5 high and r ≥ 0.5 very strong interrelationships among PHEs (Weissmannová et al., 2019). In interpreting the correlation observed strong positive correlation among the PHEs were attributed to similar types of sources for their origin and negative correlations to related geochemical behaviours (Kumar et al., 2020). The results of the correlation analyses for the study sites with statistical significance at p < 0.05 are presented as correlation plots (Figure 7).
FIGURE 7. Spearman Rank’s correlation analysis plots for PHEs in soils (A and C) and stream sediments (B and D) where blue represents positive correlation, red negative and a blank space denotes no existing correlation. p < 0.05.
Strong positive correlations are associated with similarity in the source of the PHE as well the method by which they reach or remain in the soil or sediments (Radomirović et al., 2020). In the KAD soils, many strong positive correlations between the PHEs were noted with coefficient values ranging from r = 0.55 to 0.94 (Figure 7A). The very strong associations among Al, V, Cu, Fe, Co, Mn, (r 0.84–0.94) as well as with As, Ni, Ba, and Be also showing high correlations coefficients >0.6–0.83 indicating a common source. These PHEs associated with the lithological units of the mineralised KAD area with ores of Au/Ag, Ni-Cu-Zn, Fe, barite and beryl (Anhaeusser, 2014). The positive associations in the soils observed for Cd, Zn, Ni, and Pb with Hg positively correlated with Cd, Zn, and Pb in that association, are common anthropogenic contaminants associated with industrial activities, sewage and automobile as well car exhausts (Lee et al., 2021). Hg in particular is commonly used in the KAD area by informal small-scale miners as part of the Au extraction technique. A combination of mining, urbanization and industrialisation are possible sources of the anthropogenic contaminants within the KAD setting.
The KAD sediments (Figure 7B) showed a strong positive correlation between Hg, Cd, Ni, Mn, Zn, Co, Fe, and Cu with all interrelationships with r values ranging from 0.75 to 0.97. Arsenic was also positively correlated with the PHEs in this group except for Hg. Sb showed a strong positive correlation with only As and no correlation with any of the other PHEs. Pb in sediments showed positive correlations with Be, Ba, Mn, Zn, Co, Fe, and Cu, this may result from the occurrence of galena (PbS), a lead sulphide ore within the greenstone belts together with other sulphide minerals (Ravengai et al., 2005). Cr in sediment had a strong positive correlation with Mo (0.81) and a strong negative correlation with Al (−0.67). Mo in sediments was strongly negatively correlated with Be (−0.82) Al (−0.8) and Ba (−0.7) and had no other correlations with the other PHEs. The KAD soils and sediments showed similar signatures due to the similarities of the bulk of PHE concentrations influenced by the natural background variation.
The HUR soils showed very few significant positive correlations (Figure 7C) mostly related to the signature imparted by the granitic-gneiss lithology of the area. The correlations in the HUR sediments (Figure 7D) are distinctly different from the soils at the site yet show similarities with those of the KAD sediments corroborating the suggestion of the significance of PHE dispersion within catchments and through the river system. In this assessment, Cr was only positively correlated with Mo suggesting similarity of source and showed negative correlations with Al, Fe, Co, and Hg.
The KAD site shows significant and very similar contamination in both soils and sediments. The large contribution of As and Sb to the overall contamination in the KAD area can be attributed to their close geochemical relationship. Arsenic and Sb have been described in other studies as metalloids that show similar chemical behaviour and lithogenic occurrence (Antoniadis et al., 2019). In Zimbabwe’s highly mineralised greenstone belt areas, Au is generally found bound in sulphides such as arsenopyrite (FeAsS), pyrite (FeS2), galena (PbS) and stibnite (Sb2S3) and therefore the mining and processing of its ore are linked to the release of As, Sb, Pb, Zn, and Fe (Ravengai et al., 2005). The CF values for Hg showed that the element contributes substantially to the overall PLI in the sample points associated with areas of increased informal mining activities, confirming the environmental impact of Hg use in the extraction of gold in the KAD area that has been reported by previous studies (Shoko and Veiga, 2004; Steckling et al., 2014).
The ecological risk to the freshwater ecosystem in the KAD area appears to be most affected by the processes associated with gold mining activities as evidenced by the key contributions of As, Sb, and Hg to the RI and other PHEs not showing much prominence. That said As and Hg are PHEs that have also been identified as agricultural (pesticides and fertilisers) and other non-mining (urbanization) industry contaminants Sharma et al. (2007), Wei and Yang (2010) and therefore the contribution of such activities cannot be ruled out in this setting. The influence of the natural geochemical background and mining operations in the KAD setting however outweigh the agricultural impact as observed in the land use pattern observable in satellite imagery. The contribution of Cd to the RI, though not major, may also indicate the contribution of other anthropogenic activities other than mining. Though the Empress Nickel processing plant is present in the KAD sub-catchment the results do not show Ni as a key contributor to the ecological risk as it is not a contaminant in the wastes of the roasting plant. Ore of Ni are usually low-grade high sulphide ores and the typical contaminants associated with extraction in the Ni roasting plants are gaseous emissions of sulphur dioxide that can lead to sulphuric acid production (Ekosse, 2008).
This study revealed much higher results of potential ecological risk indices for the KAD area when compared to similar studies in Zimbabwe’s gold mining areas. In the town of Shamva, which is located in similar greenstone belt settings, the PHEs: As, Cr, Cu, Fe, and Pb were all reported to be posing a low potential ecological risk with a low RI for the study site (Kanda et al., 2017). The exclusion of the Sb and other key PHEs that have been associated with contamination in mineralised and gold mining areas (Ravengai et al., 2005; Meck, 2013; Meck et al., 2020) may have had an impact on lowering the result of the ecological risk assessment in that study. When compared to other international studies, such as the review by Kumar et al. (2020) on the contamination of sediments in major Indian rivers, a far higher average RI is observed with Cd as a major contaminant attributed to industrialization, mining and urbanization. The current study however indicated a higher ER for As, Hg, and Sb which are also key contributors to contamination in the KAD area, though not assessed in the Indian study. The Sb mining activities in Hunan province in China show Sb, together with the associated Cd and Hg as the key contaminants contributing to the very high RI (Zhou et al., 2020) much higher than in KAD. The high contribution of Sb to the enhanced RI in KAD is not surprising, given the extent of pas Au and Sb mining activities in KAD.
The key PHE assemblages identified in the KAD area by the positive PHE correlations can be mainly attributed to the predominant lithological diversity and mineralisation of the site. The geochemical provinces of Au, Ni, Co, Cr, Pt, Pd, As, and Sb characterise the greenstone belt and the Great Dyke in the central Zimbabwe craton (Zhao et al. 2013) as well the multiple mineral assemblages of the greenstone belt lithologies, as described earlier, can be identified as the influence for the geochemical signatures and therefore correlations identified in both the KAD soil and sediment samples. The geochemically similar behaviour of PHEs such as that of As-Sb in the KAD setting does not follow for all elements as some will behave differently during secondary dispersion processes in the surficial media. This often results in only a partial agreement in their association once dispersed as compared to that determined from ore rock samples, making source identification a challenging process (Wang and Zuo, 2020).
Low or negative correlation of PHEs in soils can also refer to natural background variation or sources relating to natural processes Weissmannová et al. (2019), further it can also be attributed to several sources or a zone of high enrichment such as an ore deposit. This explanation can be used for the lack of positive associations or observed for Cr, Mo, and Sb. Cr is already known to occur in a major deposit, the Great Dyke that cuts across part of the Sanyati Catchment. Although economic deposits of Mo have not been discovered, anomalously high concentrations of this element were also identified through the National Geochemical Survey by Zhao et al. (2013) as worthy of further investigation and are known to be associated with granitic rocks. Antimony was once mined in KAD at the large Cam and Motor Mine. Both Cr and Mo are also common elements in agricultural fertilisers as well as key inputs in metal alloys and their correlation in the sediments only and not soils may point towards an anthropogenic influence associated with agricultural and industrial activities. As a nexus of mining, industrialization, farming and urbanization, source identification of the typical PHE associated with these anthropogenic activities, i.e., As, Cd, Cu, Zn, Ni, and Pb is compounded by the fact that these are also common ore forming elements in the KAD setting.
Arsenic and its compounds are known human carcinogens and several studies have indicated an association between As exposure and increased mortality due to cancer (Tchounwou et al., 2012; Smith et al., 2018). Other studies have also linked As consumption in drinking water with two non-carcinogenic health conditions of concern, i.e., hypertension and diabetes (WHO, 2018) and cardiovascular disease (Phung et al., 2017). Sb is an element of environmental concern due to its genotoxicity (Mao et al., 2019) and in epidemiological studies, exposure to Cr (VI) by the inhalation route has been associated with lung cancer (Kotas and Stasicka 2000) and the IARC has classified Cr (VI) in Group 1 human carcinogen and Cr (III) in Group 3 (IARC, 2012). Mo is an essential element for human health and only rare cases of toxicity in humans have been reported, it is however known to be very toxic to some animals, especially ruminants (Novotny and Peterson, 2018).
The present study was preceded by an evaluation of the uptake of PHEs by vegetables grown within the same study sites and an assessment of the potential risk posed by their bioaccumulation in the edible parts (Meck et al., 2020). That study identified Pb, As, and Cd levels well above safe limits for human health in common vegetables grown in from the study site. The city of Kadoma and its residential suburbs that include, Eiffel Flats, Sabonabona, Ngezi, and Rimuka all fall within the KAD study site together with several residential sites some of which were former mine staff compounds that have continued to grow into informal settlements. Small and medium scale horticultural plots produce vegetables that are sold to the city and beyond with some active livestock farming producing meat and milk products. Fish from rivers and the main dam Claw Dam also form part of the dietary intake and comparison with other studies have shown PHE concentrations PLI values obtained in KAD to be of levels of concern for biomagnification. Fish consumption is a main entry for aquatic pollutants such as toxic metals (e.g., Hg and Cd) whose bioaccumulation and biomagnification are known to occur along the food chain, and can result in a whole array of systemic illnesses (See, e.g., Isangedighi and Samuel David, 2019). Subsistence farming is very common and many families rely on food crops grown in this setting for their daily consumption.
In the light of the evaluation of the degree of contamination by PHEs in this study, there is a risk of exposure through both dietary and non-dietary intake which would warrant further investigation by government authorities and environmental agencies.
This study has evaluated the degree of contamination in soils and sediments using geochemical indices at two sites within the Sanyati Catchment, KAD being an auriferous site from which gold has been extracted for over a century and HUR bereft of economic metal or mineral deposits. It was deduced that though As, Sb, Cr, and Mo are the main contaminant PHEs in the KAD site, potential ecological risk resulted from Sb, Hg, and As, posing between moderate and very high ecological risk in 71% of soil and 53% of the sediment samples. The HUR site results showed a low RI for all soils and sediment samples.
The geochemical distribution of PHEs in soils over the KAD and HUR terranes has shown varying trends, reflecting the influence of differences in the lithological composition of the two sites attributed to their geological setting. The PHE distribution levels in sediments of the sites were, however, markedly similar, indicating the predominant role of the PHE dispersion process wrought by sediment transport through sub-catchments from upper reaches.
The correlation analysis showed the influence of lithology and mineralisation on the natural background variation and hence the geochemical signatures of soils and sediments. Any remediation plans would need to be carefully targeted at specific points in the catchment where higher concentrations may be found, however, total removal would not be possible. Land use planning would need to be well informed by the geochemistry of each locality.
The study showed the importance of the application of a variety of geochemical indices in the evaluation of the degree of PHE contamination in soils and sediments of an area particularly during the hazard identification phase of the risk assessment as well as for environmental management and monitoring. The importance of separately evaluating the different surficial media of soils and sediments was also evidenced particularly in the variations noted in soil and sediment contamination at the HUR site especially when the practices of the use of floodplains in the growing of crops. The study also revealed how including toxicological risk factors in evaluating health risks within the system aids potential risk evaluation, as contamination may not denote pollution. It is hoped that the results from this study may be used as a model by environmental practitioners and in the design of experiments to elucidate the role of parameters such as chemical form and bioavailability that engender the transfer of PHEs into the food chain and their subsequent metabolic reactivity that produces toxicity and disease.
The raw data from the chemical analysis and all field measurements supporting the conclusions of this article will become available from the corresponding author once all research has work been completed and published.
Conceptualisation: DM, MM, TD, and DT; Methodology: DM, MM, and TD; Data Collection and Analysis: DM and MM; Formal Analysis: DM; Writing-Original Draft: DM; Writing-Review and Editing: DM, MM, TD, and DT; Supervision: MM, TD, and DT.
The authors declare that the research was conducted in the absence of any commercial or financial relationships that could be construed as a potential conflict of interest.
All claims expressed in this article are solely those of the authors and do not necessarily represent those of their affiliated organizations, or those of the publisher, the editors and the reviewers. Any product that may be evaluated in this article, or claim that may be made by its manufacturer, is not guaranteed or endorsed by the publisher.
The authors thank UNESCO/SIDA for funding under the Mapping and Assessing the Environmental and Health Impacts of Abandoned Mines in Sub-Saharan African Countries Project. We thank the German Academic Exchange Services (DAAD) for the In-Country scholarship and the UNESCO/Sida ANESI Visiting Fellowship Programme for support extended to DM during this research. We extend our appreciation to Professor Bernhard Wehrli and ETH Zürich through the research collaboration with the University of Zimbabwe for access to grant funding towards open access publication.
The Supplementary Material for this article can be found online at: https://www.frontiersin.org/articles/10.3389/fenvs.2022.829900/full#supplementary-material
Ander, E. L., Johnson, C. C., Cave, M. R., Palumbo-Roe, B., Nathanail, C. P., and Lark, R. M. (2013). Methodology for the Determination of Normal Background Concentrations of Contaminants in English Soil. Sci. Total Environ. 454-455, 604–618. doi:10.1016/j.scitotenv.2013.03.005
Anhaeusser, C. R. (2014). Archaean Greenstone Belts and Associated Granitic Rocks - A Review. J. Afr. Earth Sci. 100, 684–732. doi:10.1016/j.jafrearsci.2014.07.019
Antoniadis, V., Golia, E. E., Liu, Y.-T., Wang, S.-L., Shaheen, S. M., and Rinklebe, J. (2019). Soil and Maize Contamination by Trace Elements and Associated Health Risk Assessment in the Industrial Area of Volos, Greece. Environ. Int. 124, 79–88. doi:10.1016/j.envint.2018.12.053
Ashton, P., Love, D., Mahachi, H., and Dirks, P. (2001). An Overview of the Impact of Mining and Mineral Processing Operations on Water Resources and Water Quality in the Zambezi, Limpopo and Olifants Catchments in Southern Africa. Harare, Zimbabwe, South Africa: Geology Department, University of Zimbabwe.
Balkhair, K. S., and Ashraf, M. A. (2016). Field Accumulation Risks of Heavy Metals in Soil and Vegetable Crop Irrigated with Sewage Water in Western Region of Saudi Arabia. Saudi J. Biol. Sci. 23, S32–S44. doi:10.1016/J.SJBS.2015.09.023
Banerjee, S., Kumar, A., Maiti, S. K., and Chowdhury, A. (2016). Seasonal Variation in Heavy Metal Contaminations in Water and Sediments of Jamshedpur Stretch of Subarnarekha River, India. Environ. Earth Sci. 75, 265. doi:10.1007/s12665-015-4990-6
Banerjee, S., Maiti, S. K., and Kumar, A. (2015). Metal Contamination in Water and Bioaccumulation of Metals in the Planktons, Molluscs and Fishes in Jamshedpur Stretch of Subarnarekha River of Chotanagpur Plateau, India. Water Environ. J. 29, 207–213. doi:10.1111/WEJ.12108
Barbieri, M. (2016). The Importance of Enrichment Factor (EF) and Geoaccumulation Index (Igeo) to Evaluate the Soil Contamination. J. Geol. Geophys. 5, 1–4. doi:10.4172/2381-8719.1000237
Becker, J., Bose-O’Reilly, S., Shoko, D., Singo, J., and Steckling-Muschack, N. (2020). Comparing the Self-Reported Health-Related Quality of Life (HRQoL) of Artisanal and Small-Scale Gold Miners and the Urban Population in Zimbabwe Using the EuroQol (EQ-5D-3L+C) Questionnaire: A Cross-Sectional Study. Health Qual. Life Outcomes 18, 253. doi:10.1186/s12955-020-01475-0
Bose-O’Reilly, S., Lettmeier, B., Shoko, D., Roider, G., Drasch, G., and Siebert, U. (2020). Infants and Mothers Levels of Mercury in Breast Milk, Urine and Hair, Data from an Artisanal and Small-Scale Gold Mining Area in Kadoma/Zimbabwe. Environ. Res. 184, 109266. doi:10.1016/j.envres.2020.109266
Boularbah, A., Schwartz, C., Bitton, G., Aboudrar, W., Ouhammou, A., and Morel, J. L. (2006). Heavy Metal Contamination from Mining Sites in South Morocco: 2. Assessment of Metal Accumulation and Toxicity in Plants. Chemosphere 63, 811–817. doi:10.1016/j.chemosphere.2005.07.076
Canales, R. A., Wilson, A. M., Pearce-Walker, J. I., Verhougstraete, M. P., and Reynolds, K. A. (2018). Methods for Handling Left-Censored Data in Quantitative Microbial Risk Assessment. Appl. Environ. Microbiol. 84, e01203–18. doi:10.1128/AEM.01203-18
Centeno, J., Finkelman, R., and Selinus, O. (2016). Medical Geology: Impacts of the Natural Environment on Public Health. Geosciences 6, 8. doi:10.3390/geosciences6010008
Chanza, N., and Gundu-Jakarasi, V. (2020). “Deciphering the Climate Change Conundrum in Zimbabwe: An Exposition,” in Global Warming and Climate Change (London: IntechOpen Ltd.), 1–25. Available at: http://www.intechopen.com/books/trends-in-telecommunications-technologies/gps-total-electron-content-tec- prediction-at-ionosphere-layer-over-the-equatorial-region%0AInTec.
Davies, T. C. (2013). Geochemical Variables as Plausible Aetiological Cofactors in the Incidence of Some Common Environmental Diseases in Africa. J. Afr. Earth Sci. 79, 24–49. doi:10.1016/j.jafrearsci.2012.11.002
Davis, R., Hirji, R., Murwira, A., Owen, R., and Murungweni, Z. (2014). Climate Change and Water Resources Planning, Development, and Management in Zimbabwe. Washington, DC: World Bank. doi:10.1201/9780203020777-17
de Caritat, P., and Mann, A. (2018). An Improved Method for Assessing the Degree of Geochemical Similarity (DOGS2) between Samples from Multi-Element Geochemical Datasets. Geochem. Explor. Environ. Anal. 19, 58–73. doi:10.1144/geochem2018-021
Dissanayake, C. (2005). Of Stones and Health: Medical Geology in Sri Lanka. Science 309, 883–885. doi:10.1126/science.1115174
Du Laing, G., Rinklebe, J., Vandecasteele, B., Meers, E., and Tack, F. M. G. (2009). Trace Metal Behaviour in Estuarine and Riverine Floodplain Soils and Sediments: A Review. Sci. Total Environ. 407, 3972–3985. doi:10.1016/j.envint.2019.02.011
Ekosse, G.-I. E. (2008). Environmental Effects of Nickel-Copper Exploitation on Workers Health Status at Selebi Phikwe Area, Botswana. J. Appl. Sci. 8, 2344–2356. doi:10.3923/JAS.2008.2344.2356
Finkelman, R. B., and Centeno, J. A. (2020). Guizhou Province, China: the Birthplace of Modern Medical Geology. Acta Geochim. 39, 155–159. doi:10.1007/s11631-019-00380-8
Finkelman, R. B., Orem, W. H., Plumlee, G. S., and Selinus, O. (2018). “Applications of Geochemistry to Medical Geology,” in Environmental Geochemistry: Site Characterization, Data Analysis and Case Histories. Second Edition (London: Elsevier), 435–465. doi:10.1016/B978-0-444-63763-5.00018-5
Hakanson, L. (1980). An Ecological Risk Index for Aquatic Pollution control.a Sedimentological Approach. Water Res. 14, 975–1001. doi:10.1016/0043-1354(80)90143-8
Hao, L., Zhao, X., Zhao, Y., Lu, J., and Sun, L. (2014). Determination of the Geochemical Background and Anomalies in Areas with Variable Lithologies. J. Geochem. Explor. 139, 177–182. doi:10.1016/J.GEXPLO.2013.11.007
Heidari, A., Osat, M., and Konyushkova, M. (2022). Geochemical Indices as Efficient Tools for Assessing the Soil Weathering Status in Relation to Soil Taxonomic Classes. CATENA 208, 105716. doi:10.1016/J.CATENA.2021.105716
IARC (2012). “Arsenic, Metals, Fibres, and Dusts. A Review of Human Carcinigens,” in IARC Working Group on the Evaluation of Carcinogenic Risks to Humans (Lyon, France: WHO). Available at: https://www.iarc.fr/.
Isangedighi, A. I, and Samuel David, G. (2019). Heavy Metals Contamination in Fish: Effects on Human Health. J. Aquat. Sci. Mar. Biol. 2, 7–12.
Kanda, A., Ncube, F., Mabote, R. R., Mudzamiri, T., Kunaka, K., and Dhliwayo, M. (2020). Trace Elements in Water, Sediment and Commonly Consumed Fish from a Fish Farm (NE Zimbabwe) and Risk Assessments. SN Appl. Sci. 2, 1–14. doi:10.1007/s42452-020-03291-z
Kanda, A., Ncube, F., and Takura, R. (2017). Potential Ecological Risk Assessment of a Stream in Shamva, Zimbabwe. Environ. Earth Ecol. 1, 67–80. doi:10.24051/eee/68608
Kicińska, A., Turek, K., and Turek, K. (2017). Establishing Geochemical Background of Elements Present in Soil and its Application in the Evaluation of Soil Pollution Based on Data Collected in the Beskid Sądecki Region. Geoinformatica Pol. 16, 87–99. doi:10.4467/21995923gp.17.007.7194
Kotas, J., and Stasicka, Z. (2000). Chromium Occurrence in the Environment and Methods of its Speciation. Environ. Pollut. 107, 263–283. doi:10.1016/s0269-7491(99)00168-2
Kumar Sharma, R., Agrawal, M., and Marshall, F. (2007). Heavy Metal Contamination of Soil and Vegetables in Suburban Areas of Varanasi, India. Ecotoxicol. Environ. Saf. 66, 258–266. doi:10.1016/J.ECOENV.2005.11.007
Kumar, V., Sharma, A., Pandita, S., Bhardwaj, R., Thukral, A. K., and Cerda, A. (2020). A Review of Ecological Risk Assessment and Associated Health Risks with Heavy Metals in Sediment from India. Int. J. Sediment Res. 35, 516–526. doi:10.1016/j.ijsrc.2020.03.012
Lee, H. G., Byun, Y. J., Chun, Y. W., Noh, H. J., Kim, D. J., Kim, H. K., et al. (2021). Identification of Metal Contamination Sources and Evaluation of the Anthropogenic Effects in Soils Near Traffic-Related Facilities. Toxics 9, 278. doi:10.3390/TOXICS9110278/S1
Li, Y., Qu, X., Zhang, M., Peng, W., Yu, Y., and Gao, B. (2018). Anthropogenic Impact and Ecological Risk Assessment of Thallium and Cobalt in Poyang Lake Using the Geochemical Baseline. Water 10, 1703. doi:10.3390/w10111703
Liu, Y., Xiao, T., Perkins, R. B., Zhu, J., Zhu, Z., Xiong, Y., et al. (2017). Geogenic Cadmium Pollution and Potential Health Risks, with Emphasis on Black Shale. J. Geochem. Explor. 176, 42–49. doi:10.1016/j.gexplo.2016.04.004
Lupankwa, K., Love, D., Mapani, B. S., and Mseka, S. (2004). Impact of a Base Metal Slimes Dam on Water Systems, Madziwa Mine, Zimbabwe. Phys. Chem. Earth, Parts A/B/C 29, 1145–1151. doi:10.1016/j.pce.2004.09.017
Mambrey, V., Rakete, S., Tobollik, M., Shoko, D., Moyo, D., Schutzmeier, P., et al. (2020). Artisanal and Small-Scale Gold Mining: A Cross-Sectional Assessment of Occupational Mercury Exposure and Exposure Risk Factors in Kadoma and Shurugwi, Zimbabwe. Environ. Res. 184, 109379. doi:10.1016/j.envres.2020.109379
Mamuse, A., and Watkins, R. (2016). High Fluoride Drinking Water in Gokwe, Northwest Zimbabwe. J. Water Sanit. Hyg. Dev. 6, 55–64. doi:10.2166/washdev.2016.188
Manjengwa, J., Chimhowu, A., Matema, C., and Nyelele, C. (2012). “Poverty Dynamics in Zimbabwe,” in Understanding Poverty , Promoting Wellbeing and Sustainable Development. Editors J. Manjengwa, S. Feresu, and A. Chimhowu (Harare: Institute of Environmental Studies, University of Zimbabwe).
Mao, L., Ye, H., Li, F., Yang, M., Tao, H., and Wen, H. (2019). Enrichment Assessment of Sb and Trace Metals in Sediments with Significant Variability of Background Concentration in Detailed Scale. Environ. Sci. Pollut. Res. 26, 2794–2805. doi:10.1007/s11356-018-3836-7
Mashizha, T. M., Ncube, C., Dzvimbo, A., and Monga, M. (2017). Examining the Impact of Climate Change on Rural Livelihoods and Food Security : Evidence from Sanyati District in Mashonaland West, Zimbabwe. J. Asian Afr. Soc. Sci. Humanit. 3, 56–68. doi:10.11648/j.ijsdr.20170302.12
Meck, M. L. (2013). “Geochemistry for Sustainable Development in Africa: Zimbabwe Case Study,” in Chemistry for Sustainable Development in Africa. Editors A. Gurib-Fakim, and J. N. Eloff (Berlin: Springer-Verlag), 105–122. doi:10.1007/978-3-642-29642-0
Meck, M. L., Mudimbu, D., and Davies, T. C. (2020). Accumulation of Potentially Harmful Elements in Edible Parts of Vegetables Grown on Two Different Geological Substrates in Zimbabwe. J. Geochem. Explor. 208, 106392. doi:10.1016/J.GEXPLO.2019.106392
Meng, Y., Cave, M., and Zhang, C. (2020). Identifying Geogenic and Anthropogenic Controls on Different Spatial Distribution Patterns of Aluminium, Calcium and Lead in Urban Topsoil of Greater London Authority Area. Chemosphere 238, 124541. doi:10.1016/j.chemosphere.2019.124541
Mikkonen, H., Clarke, B., van de Graaff, R., and Reichman, S. (2016). Geochemical Indices Allow Estimation of Heavy Metal Background Concentrations in Soils. London: Elsevier.
Monged, M. H. E., Hassan, H. B., and El-Sayed, S. A. (2020). Spatial Distribution and Ecological Risk Assessment of Natural Radionuclides and Trace Elements in Agricultural Soil of Northeastern Nile Valley, Egypt. Water. Air. Soil Pollut. 231, 1–24. doi:10.1007/s11270-020-04678-9
Mortazavi, S., Tizhoosh, M., and Cheraghi, Z. (2017). Quality Evaluation and Study of Ecological Toxicity of Heavy Metals in Shadegan Wetland. J. Contemp. Urban Aff. 1, 67–72. doi:10.25034/ijcua.2018.3683
Mossop, R. T. (1989). On Living in an Arsenical Atmosphere (Part 2). Clinical Observations, Animal Experiments and Ecological Problems. Cent. Afr. J. Med. 35, 546–551.
Muchuweti, M., Birkett, J. W., Chinyanga, E. A., Zvauya, R., Scrimshaw, M. D., and Lester, J. N. (2006). Heavy Metal Content of Vegetables Irrigated with Mixtures of Wastewater and Sewage Sludge in Zimbabwe: Implications for Human Health. Agric. Ecosyst. Environ. 112, 41–48. doi:10.1016/j.agee.2005.04.028
Nharingo, T., Ndumo, T., and Moyo, M. (2015). Human Health Risks Due to Heavy Metals through Consumption of Wild Mushrooms from Macheke Forest, Rail Block Forest and Muganyi Communal Lands in Zimbabwe. Environ. Monit. Assess. 187, 1–11. doi:10.1007/s10661-015-4974-8
Niu, L., Li, J., Luo, X., Fu, T., Chen, O., and Yang, Q. (2021). Identification of Heavy Metal Pollution in Estuarine Sediments under Long-Term Reclamation: Ecological Toxicity, Sources and Implications for Estuary Management. Environ. Pollut. 290, 118126. doi:10.1016/j.envpol.2021.118126
Novotny, J. A., and Peterson, C. A. (2018). Molybdenum. Adv. Nutr. 9, 272–273. doi:10.1093/advances/nmx001
Phung, D., Connell, D., Rutherford, S., and Chu, C. (2017). Cardiovascular Risk from Water Arsenic Exposure in Vietnam: Application of Systematic Review and Meta-Regression Analysis in Chemical Health Risk Assessment. Chemosphere 177, 167–175. doi:10.1016/j.chemosphere.2017.03.012
Proshad, R., Kormoker, T., Islam, S. M., Hanif, M. A., and Chandra, K. (2018). Chronic Exposure Assessment of Toxic Elements from Agricultural Soils Around the Industrial Areas of Tangail District, Bangladesh. Arch. Agri. Environ. Sci. 3, 317–336. doi:10.26832/24566632.2018.030401
Qu, C.-S., Ma, Z.-W., Yang, J., Liu, Y., Bi, J., and Huang, L. (2012). Human Exposure Pathways of Heavy Metals in a Lead-Zinc Mining Area, Jiangsu Province, China. PLoS One 7, e46793. doi:10.1371/journal.pone.0046793
Radomirović, M., Ćirović, Ž., Maksin, D., Bakić, T., Lukić, J., Stanković, S., et al. (2020). Ecological Risk Assessment of Heavy Metals in the Soil at a Former Painting Industry Facility. Front. Environ. Sci. 8, 177. doi:10.3389/FENVS.2020.560415/BIBTEX
Rahman, S. H., Khanam, D., Adyel, T. M., Islam, M. S., Ahsan, M. A., and Akbor, M. A. (2012). Assessment of Heavy Metal Contamination of Agricultural Soil Around Dhaka Export Processing Zone (DEPZ), Bangladesh: Implication of Seasonal Variation and Indices. Appl. Sci. 2, 584–601. doi:10.3390/app2030584
Rashed, M. N. (2010). Monitoring of Contaminated Toxic and Heavy Metals, From Mine Tailings Through Age Accumulation, in Soil and Some Wild Plants at Southeast Egypt. J. Hazard. Mater. 178, 739–746. doi:10.1016/j.jhazmat.2010.01.147
Ravengai, S., Love, D., Mabvira-Meck, M., Musiwa, K., and Moyce, W. (2005). Water Quality in an Abandoned Gold Mining Belt, Beatrice, Sanyati Valley, Zimbabwe. Phys. Chem. Earth, Parts A/B/C 30, 826–831. doi:10.1016/j.pce.2005.08.026
Rinklebe, J., Antoniadis, V., Shaheen, S. M., Rosche, O., and Altermann, M. (2019). Health Risk Assessment of Potentially Toxic Elements in Soils along the Central Elbe River, Germany. Environ. Int. 126, 76–88. doi:10.1016/j.envint.2019.02.011
RStudio Team (2021). RStudio: Integrated Development Environment for R. Available at: http://www.rstudio.com/ (Accessed February 10, 2022).
Rudnick, R. L., and Gao, S. (2003). “Composition of the Continental Crust,” in Treatise on Geochemistry. Editors K. K. Turekian, and H. D. Holland (Amsterdam: Elsevier), 1–64. doi:10.1016/B0-08-043751-6/03016-4
Salomão, G. N., Farias, D. L., Sahoo, P. K., Dall’Agnol, R., and Sarkar, D. (2021). Integrated Geochemical Assessment of Soils and Stream Sediments to Evaluate Source-Sink Relationships and Background Variations in the Parauapebas River Basin, Eastern Amazon. Soil Syst. 5, 21. doi:10.3390/soilsystems5010021
Sany, S. B. T., Salleh, A., Sulaiman, A. H., Sasekumar, A., Tehrani, G., and Rezayi, M. (2012). Distribution Characteristics and Ecological Risk of Heavy Metals in Surface Sediments of West Port, Malaysia. Environ. Prot. Eng. 38, 139–155. doi:10.5277/EPE12041210.37190/epe120412
Schober, P., Boer, C., and Schwarte, L. A. (2018). Correlation Coefficients. Anesth. Analgesia 126, 1763–1768. doi:10.1213/ANE.0000000000002864
Shoko, D., and Veiga, M. M. (2004). Global Mercury Project: Information about the Project Sites in Zimbabwe. Available at: http://www.globalmercuryproject.org/countries/zimbabwe/docs/Zim project sites.pdf (Accessed March 01, 2013) (Accessed March 20, 2021).
Smith, A. H., Marshall, G., Roh, T., Ferreccio, C., Liaw, J., and Steinmaus, C. (2018). Lung, Bladder, and Kidney Cancer Mortality 40 Years after Arsenic Exposure Reduction. J. Natl. Cancer Inst. 110, 241–249. doi:10.1093/jnci/djx201
Song, Z., Song, G., Tang, W., Yan, D., Zhao, Y., Zhu, Y., et al. (2021). Molybdenum Contamination Dispersion from Mining Site to a Reservoir. Ecotoxicol. Environ. Saf. 208, 111631. doi:10.1016/J.ECOENV.2020.111631
Sorensen, J. P. R., Nayebare, J., Carr, A. F., Lyness, R., Campos, L. C., Ciric, L., et al. (2021). In-Situ Fluorescence Spectroscopy Is a More Rapid and Resilient Indicator of Faecal Contamination Risk in Drinking Water Than Faecal Indicator Organisms. Water Res. 206, 117734. doi:10.1016/J.WATRES.2021.117734
Statistics Solutions (2019). Correlation (Pearson, Kendall, Spearman). Available at: https://www.statisticssolutions.com/correlation-pearson-kendall-spearman/ (Accessed May 20, 2019).
Steckling, N., Bose-O’Reilly, S., Pinheiro, P., Plass, D., Shoko, D., Drasch, G., et al. (2014). The Burden of Chronic Mercury Intoxication in Artisanal Small-Scale Gold Mining in Zimbabwe: Data Availability and Preliminary Estimates. Environ. Health 13, 111. doi:10.1186/1476-069X-13-111
Taylor, S. R., and McLennan, S. M. (1985). The Continental Crust: Its Composition and Evolution. Palo Alto, CA: Blackwell Scientific Publications. Available at: https://www.osti.gov/biblio/6582885.
Tchounwou, P. B., Yedjou, C. G., Patlolla, A. K., and Sutton, D. J. (2012). Heavy Metal Toxicity and the Environment. EXS 101, 133–164. doi:10.1007/978-3-7643-8340-4_6
Tchounwou, P. B., Yedjou, C. G., Udensi, U. K., Pacurari, M., Stevens, J. J., Patlolla, A. K., et al. (2019). State of the Science Review of the Health Effects of Inorganic Arsenic: Perspectives for Future Research. Environ. Toxicol. 34, 188–202. doi:10.1002/tox.22673
Tomlinson, D. L., Wilson, J. G., Harris, C. R., and Jeffrey, D. W. (1980). Problems in the Assessment of Heavy-Metal Levels in Estuaries and the Formation of a Pollution Index. Helgol. Meeresunters 33, 566–575. doi:10.1007/bf02414780
Tunhuma, N. M., Kelderman, P., Love, D., and Uhlenbrook, S. (2004). Environmental Impact Assessment of Small Scale Resource Exploitation: The Case of Gold Panning in Zhulube Catchment. Zimbabwe: Limpopo Basin, 236.
Turekian, K. K., and Wedepohl, K. H. (1961). Distribution of the Elements in Some Major Units of the Earth's Crust. Geol. Soc. Am. Bull. 72, 175–192. doi:10.1130/0016-7606(1961)72[175:doteis]2.0.co;2
US EPA (1999). Appendix E Toxicity Reference Values Screening Level Ecological Risk Assessment Protocol. Available at: https://archive.epa.gov/epawaste/hazard/tsd/td/web/pdf/appx-e.pdf (Accessed November 15, 2021).
US EPA (2021). Regional Screening Levels (RSLs) - Generic Tables. Available at: https://www.epa.gov/risk/regional-screening-levels-rsls-generic-tables (Accessed November 2, 2021).
US EPA (2002). Supplemental Guidance for Developing Soil Screening. Unites States: U.S. Environmental Protection Agency.
Wang, J., and Zuo, R. (2020). Quantifying the Distribution Characteristics of Geochemical Elements and Identifying Their Associations in Southwestern Fujian Province, China. Minerals 10, 183. doi:10.3390/MIN10020183
Wang, S., Zhang, B., Li, T., Li, Z., and Fu, J. (2020). Soil Vanadium(V)-reducing Related Bacteria Drive Community Response to Vanadium Pollution from a Smelting Plant over Multiple Gradients. Environ. Int. 138, 105630. doi:10.1016/J.ENVINT.2020.105630
Wei, B., and Yang, L. (2010). A Review of Heavy Metal Contaminations in Urban Soils, Urban Road Dusts and Agricultural Soils from China. Microchem. J. 94, 99–107. doi:10.1016/j.microc.2009.09.014
Weissmannová, H. D., Mihočová, S., Chovanec, P., and Pavlovský, J. (2019). Potential Ecological Risk and Human Health Risk Assessment of Heavy Metal Pollution in Industrial Affected Soils by Coal Mining and Metallurgy in Ostrava, Czech Republic. Int. J. Environ. Res. Public Health 16. doi:10.3390/ijerph16224495
WHO (2018). Global Health Observatory (GHO) Data NCD Mortality and Morbidity. Geneva: World Health Organization. Available at: http://www.who.int/gho/ncd/mortality_morbidity/en/ (Accessed September 20, 2020).
Wu, J., Teng, Y., Lu, S., Wang, Y., and Jiao, X. (2014). Evaluation of Soil Contamination Indices in a Mining Area of Jiangxi, China. PLoS One 9, e112917. doi:10.1371/journal.pone.0112917.t001
Yu, G., Chen, F., Zhang, H., and Wang, Z. (2021). Pollution and Health Risk Assessment of Heavy Metals in Soils of Guizhou, China. Ecosyst. Health Sustain. 7, 1. doi:10.1080/20964129.2020.1859948
Zhang, C., Appel, E., and Qiao, Q. (2013). Heavy Metal Pollution in Farmland Irrigated with River Water Near a Steel Plant-Magnetic and Geochemical Signature. Geophys. J. Int. 192, 963–974. doi:10.1093/gji/ggs079
Zhao, G., He, F., Dai, X., Zhang, S., and Yu, R. (2013). Ultra-Low Density Geochemical Mapping in Zimbabwe. J. Geochem. Explor. 144, 552–571. doi:10.1016/j.gexplo.2013.11.001
Keywords: potentially harmful elements, spatial analysis, potential ecological risk, Zimbabwe, geochemical indices
Citation: Mudimbu D, Davies TC, Tagwireyi D and Meck ML (2022) Application of Geochemical Indices in Evaluating Potentially Harmful Element Contamination at Mining Centres in the Sanyati Catchment, Zimbabwe. Front. Environ. Sci. 10:829900. doi: 10.3389/fenvs.2022.829900
Received: 06 December 2021; Accepted: 20 April 2022;
Published: 25 May 2022.
Edited by:
Carl Mitchell, University of Toronto Scarborough, CanadaReviewed by:
Joshua Nosa Edokpayi, University of Venda, South AfricaCopyright © 2022 Mudimbu, Davies, Tagwireyi and Meck. This is an open-access article distributed under the terms of the Creative Commons Attribution License (CC BY). The use, distribution or reproduction in other forums is permitted, provided the original author(s) and the copyright owner(s) are credited and that the original publication in this journal is cited, in accordance with accepted academic practice. No use, distribution or reproduction is permitted which does not comply with these terms.
*Correspondence: Daina Mudimbu, ZGVlbXVkaW1idUBnbWFpbC5jb20=
Disclaimer: All claims expressed in this article are solely those of the authors and do not necessarily represent those of their affiliated organizations, or those of the publisher, the editors and the reviewers. Any product that may be evaluated in this article or claim that may be made by its manufacturer is not guaranteed or endorsed by the publisher.
Research integrity at Frontiers
Learn more about the work of our research integrity team to safeguard the quality of each article we publish.