- 1Department of Microbiology, Ramón y Cajal Institute for Health Research (IRYCIS), Ramón y Cajal University Hospital, Madrid, Spain
- 2Network Center for Research in Epidemiology and Public Health (CIBERESP), Madrid, Spain
- 3Network Center for Research Infectious Diseases (CIBERINFEC), Madrid, Spain
- 4Department of Systems Biology, National Center for Biotechnology (CNB-CSIC), Madrid, Spain
Water and soil contain a multiplicity of particulate material coated with bacterial populations and communities. Microbiotic particles are any type of small particle (measuring less than 2 mm) to which bacteria and other microbes attach, resulting in medium to long-term colonization. This study reviews the interactions of ecologically distant bacterial organisms on microbiotic particles in soil and water as a method for explaining the evolution and spread of antibiotic resistance traits. These particles include bacteria-bacteria aggregates, which can merge with particles from fungi, protozoa, phytoplankton, zooplankton, and biodetritus resulting from animal and vegetal decomposition, humus, mineral particles (clay, carbonates, silicates), and anthropogenic particles (including wastewater particles and microplastics). In turn, these complex particles can interact and coalesce. Natural phenomena (waterflow, tides, tsunamis, currents, and strong winds) and anthropogenic activity (agriculture, waste-water management, mining, excavation/construction) favor the interaction and merging of microbiotic particles in soil and water, resulting in enhanced recombinant communities capable of exchanging genetic material, including antimicrobial resistance genes, particularly in antimicrobial-polluted environments. In this review, we propose that the worldwide spread of antimicrobial resistance might be related to the environmental dynamics of microbiotic particles, and we discuss possible methods for reducing this problem that threatens One Health and Planetary Health.
Introduction
Bacteria are attracted to surfaces (Ardré et al., 2019) whose presence entails a reduction or loss in environment homogeneity, giving rise to differentiated patches and spatially structured compartments. Surfaces provide spatial compartments in which organic molecules, microorganisms, and pollutants can concentrate, fragmenting the apparent homogeneity of water bodies. Biotic and abiotic particulate matter are a major source of surfaces, providing cell surfaces and surfaces created by exopolymerization processes (Ardré et al., 2019). Such compartmentalization of particulate matter contributes to the subdivision of bacterial populations colonizing the various patches (metapopulations), giving rise to phenotypic and genotypic differentiation (Rainey and Travisano 1998). The tendency of bacterial clones and populations to differentiate and increase in diversity and complexity might be the result of random events (McShea and Brandon 2010; Wang et al., 2016); however, compartmentalized, heterogeneous environments are likely essential for selecting bacterial variants adapted to the local conditions. The random bacterial occupation of microcompartments might also favor drift evolution, providing opportunities for minority populations with low fixation possibilities in fully homogeneous environments. Consequently, the framework for evolution consists not only of time but of space. In fact, time is required to gain space, which in turn is needed to gain time. This biological “hunger for time” (reproduction, survival) is a relevant philosophical issue (Baquero 2005; Arenhart 2019). In evolutionary dynamics, differentiated spaces/compartments are as critical as time. Organisms that expand into these spaces improve their evolutionary capabilities.
This review proposes that the global spread of antimicrobial resistance is related to the environmental dynamics of microbiotic particles, forming compartmentalized spaces where bacterial cells of differing origin (and frequently of different species) coaggregate and can genetically and phenotypically interact. Such coalescent spaces constitute a wealth of “evolutionary reactors” for the evolution of antimicrobial resistance (Baquero et al., 2008). In more practical terms, the spatial heterogeneity created by microbiotic particles influences sampling, making it difficult or impossible to accurately predict local the microbial densities of dangerous microorganisms and, consequently, the assessment of environmental health risks (Burkart 2000).
Surfaces, Particles, and Compartmentalized Biospaces
In addition to their attraction towards surfaces, living organisms provide surfaces. The external surface of the average bacterial cell extends approximately 5–10 times the size of the surface where the cell was deposited. Water systems provide a wealth of particles with attachment surfaces for microorganisms, surfaces that serve as “condenser nodes” (aggregation nodes) for numerous bacterial species, thereby creating biospaces, which are essentially a type of nest with an interwoven scaffolding structure determined by physical-chemical attractants that promote bacterial adhesion to surfaces. These attachment surfaces are biotic and abiotic in nature and result in the formation of biotic particles. Adhesion surfaces for microorganisms in water and soil environments include the surfaces of prokaryotic and eukaryotic organisms (Simon et al., 2002). Bacterial attachment to surfaces is frequently followed by the construction of biofilms, which result from the release of extracellular polymers, such as polysaccharides. In this review, we will focus on microbiotic particles ranging in mean size from 5 μm to 5 mm in diameter and composed of or carrying bacterial organisms. Emphasis will be placed on proteobacteria (given that gammaproteobacteria [γ-proteobacteria] includes most human pathogens) and the organisms in which antibiotic resistance genes of clinical significance are more frequently found. However, genes from γ-proteobacteria might have their origin or can spread to other branches of the phylum (Kloesges et al., 2011).
Bacteria-Bacteria Microbiotic Particles
Bacterial surfaces promote interbacterial homogenic and heterogenic adhesion, which involve the same or different species. Autoaggregation occurs within the same clonal population, forming spontaneous aggregates in the growing process. In Gram-positive organisms, numerous genera form spontaneous aggregates in liquid media (classical examples include Staphylococcus, Streptococcus, Micrococcus, Enterococcus, Sarcina, Bacillus, Listeria, Corynebacterium, Mycobacterium, and Streptomyces). Aggregates are also produced in Gram-negative bacteria, typically mediated by trimeric autotransporter adhesins in the outer membrane (Bassler et al., 2015; Adlakha et al., 2019). Autoaggregation (same species) mechanisms have recently been reviewed (Nwoko and Okeke 2021). In water environments, bacterial flocs (a type of microbiotic aggregate) can be integrated by one or more species, have being designed “suspended bacterial aggregates” (Cai 2020), and constitute an intermediary step in the adhesion to other biotic and abiotic surfaces, forming colonies and biofilms (Vlamakis et al., 2013). Homogeneous biofilms also provide “surfaces” that can adhere to bacterial cells. Coexistence facilitates interspecific biofilm formation in complex microbial communities, giving rise to multimicrobial compartmentalized consortia, complex biofilms (Katharios-Lanwermeyer et al., 2014; Madsen et al., 2016). Complex biofilms have an internal 3-D compartmentalized structure derived from the combination of microcolonies, extracellular polymeric substances, and channels (Wimpenny et al., 2000). This three-dimensional structure favors the detachment of biofilm particles, particularly due to the internal structural stress from liquid flow (Picioreanu et al., 2004). The result of this process is a type of complex biofilm reproduction, and bacterial complex coaggregates could evolve to become an biological “unit of selection”, i.e., an evolutionary individual (Okasha 2006; Baquero et al., 2021). This unit is tightened by a permanent assembly of organisms linked by cooperative or mutualistic interactions (Røder et al., 2020), leading to synergistic integrated functions in the ecosystems.
Microalgae-Bacteria Microbiotic Particles
Eukaryotic microalgae cells, a main constituent of phytoplankton, are surrounded by a chemical phycosphere (a microscale mucus region rich in organic matter) (Bell and Mitchell 1972), which can be colonized by microorganisms, ensuring long-term spatial coexistence with bacterial groups, based on a complex interactive network of metabolites and signaling molecules (Seymour et al., 2017; Cirri and Pohnert 2019), which includes bacterial quorum sensing signals (such as N-acyl-homoserine lactones) promoting microalgae-bacteria aggregation (Zhou et al., 2017). Most bacteria associated with microalgae (typically diatoms) are Proteobacteria and Bacteroidetes, which are also frequently linked to green algae (Amin et al., 2012; Ramanan et al., 2016). During cyanobacterial blooms, Firmicutes and Proteobacteria increase in frequency (Zhang et al., 2019). Anthropogenic interventions might affect these interactions; for instance, technologies based on microalgae-bacteria aggregates are increasingly considered an alternative to activated sludge treatments of wastewater (Quijano et al., 2017; Lee and Lei 2019).
Microfungi-Bacteria Microbiotic Particles
Fungi have classically been considered infrequent in water, although they have been detected in deep sea sediments (Damare and Raghukumar 2008). In most cases, these fungal species also occur in soil and freshwater environments, suggesting a terrestrial origin (Rédou et al., 2015). However, more recent studies have indicated a high fungal biomass in the oceans and the Arctic sea, approaching the bacterial biomass (Hassett et al., 2019). Many of these microfungi (such as Chytridiomycota) are also found in soil and freshwater. In fact, microscopic fungi are frequently present in soil and are particularly associated with the plant rhizosphere, where they closely and permanently interact with specific bacterial communities. Incidentally, these communities might play a role in triggering the germination of fungal spores (Scherlach et al., 2013). Such aggregates modulate mycorrhizal symbiosis, a type of positive interaction reflected in the concept of mycorrhiza helper bacteria (Frey-Klett et al., 2007). Kin recognition and cooperation among bacteria occurs in spatially structured Rhizobium populations (Zee and Bever 2014). During periodic or accidental merging of soil and water bodies, these functional fungal-bacterial particles can enter the water environment. When outside the mycorrhizal space, fungal-bacterial interactions in the soil are frequently antagonistic (Bahram et al., 2018), probably preventing permanent particulate coaggregates. Macrofungi such as Ascomycota and Basidiomycota, which appear to be important ecological players in all aquatic ecosystems (Grossart et al., 2019), contribute to the formation of bacterial-colonizable particles such as those resulting from leaf litter decomposition (Zhao et al., 2021). In short, the importance of the rhizosphere in the development of microbiotic particles is derived from the massive extent of this ecological compartment, considered the most important microbial site in the soil and in terrestrial ecosystems (Kuzyakov and Razavi 2019).
Protozoa-Bacterial Microbiotic Particles
Amoeba are frequent protists in soil and water, usually grazing bacteria but ultimately preserving them, using a kind of “primitive farming” behavior (Brock et al., 2011). Social amoeba, such as Dictyostelium discoideum, can form multicellular aggregates, and these particles incorporate living bacteria. In fact, bacterial organisms can also kill and grow at the expense of dead amoebas (Pukatzki et al., 2002; Bahram et al., 2018; Nguyen et al., 2020). Slime molds (myxomycetes) are composed of aggregates of amoebal organisms fused in a large cell (plasmodia) by the centripetal attraction of a signaling agent, acrasin. Many types of bacteria, mostly belonging to Proteobacteria, have been found to be associated with these plasmodia (Li et al., 2018).
Zooplankton-Bacterial Microbiotic Particles
Similarly, zooplankton constitute a large compartmentalized biospace harboring permanent bacterial communities; for example, colonizing the exoskeleton and gut of crustacean plankton (de Corte et al., 2018). The microbiota associated with Daphnia consists of ß-proteobacteria, γ-proteobacteria, and Flavobacteria (Cooper and Cressler 2020). However, individual zooplankton species can vary in their ability to host bacterial communities (Wang et al., 2021).
Biodetritus-Bacterial Microbiotic Particles
In soil, bacterial aggregates can be established on biodetritus, which is physically unbound (not bound to soil mineral particles), dead particulate organic matter, including partially decomposed vegetables and animals (such as nematodes, tracheids, and pot worms) (Carter and Gregorich 2007). Biodetritus, which forms a “detritusphere”, frequently overlaps the rhizosphere. The abundant nutrients released by litter decomposition create a dynamic structure somewhat analogous to the trophic-dynamic aspect of biota, thereby affecting biodetritus-attached bacteria (Rich and Wetzel 1978; Kuzyakov and Razavi 2019). In seawater, diatom detritus is heavily colonized by γ-proteobacteria, α-proteobacteria and Flavobacteria (Abell and Bowman 2005). Phytoplankton biodetritus creates a dynamic detritusphere, with microbial processes of aggregation and degradation involving bacterial and protozoan succession (Biddanda and Pomeroy, 1988). Detritus originating from seaweed and carrying bacteria such as Proteobacteria, Bacteroidetes, Firmicutes, Cyanobacteria, Planctomycetes, Actinobacteria and Verrucomicrobia might also play a role in the spread of microbiotic particles (Selvarajan et al., 2019). Water farming (e.g., for raising salmon) creates conditions favoring the production of microbiotic particles near the farming facilities (Poirier et al., 2020).
Humus-Bacterial Microbiotic Particles
Humus is the organic dark material in soil resulting from the decomposition of plant and animal matter. The humic material can be of colloidal size (humic and fulvic acids) or larger and insoluble (humins) and can degrade, eventually resulting in soluble compounds. Studies with atomic force microscopy have characterized the interactions between natural organic material and bacteria, showing that adhesion was proportional to the material’s molecular weight, particle size, and charge density. The charge of the bacterial surface also affects adhesion (Abu-Lail et al., 2007). Humus forms flocculated complexes with clay particles (see below).
Mineral-Bacterial Microbiotic Particles
Organo-mineral assemblages are microaggregates involving bacteria, which are facilitated by bacterial extracellular polymeric substances. Pre-existing soil aggregate bacterial communities are incorporated into water environments under soil-water merging circumstances. However, most aggregate bacterial communities and therefore most microbiome interactions are established in small (less than 2 mm) soil mineral aggregates of heterogeneous origins (such as clay minerals, i.e., layered aluminum silicates, and carbonates), which are extremely resilient to physical-mechanical disruptions and water effects and can form “microbial villages” (Wilpiszeski et al., 2019). Most importantly, microbes contribute to mineral (such as clay and carbonates) authigenesis, which is the process of in situ formation of mineral particles in sediments. The microbial exploitation of suspended clay particles in liquid environments promotes their microaggregation (Watts et al., 2005). Microbial biofilms constitute a reactive exopolysaccharide matrix that binds soluble chemical components and forms solid inorganic particles (Konhauser and Urrutia 1999; Wei et al., 2021). Structurally, the clay leaflets can be arranged in the form of a “house of cards”. In fact, aggregates resemble hutches housing bacterial cells (Lünsdorf et al., 2000). Electrochemically-active microbial populations and communities surrounded by exopolymer substances (frequently involving Geobacter and Methanosaeta species) are capable of extracellular electron transfer. These communities tend to aggregate on minerals such as manganese dioxide and iron oxide (Virdis et al., 2012; Kiran and Patil 2019; Xie et al., 2021). These organisms therefore breathe the mineral they grow on as part of their regular cellular metabolism. The mineral-attached biofilm and the increased electrostatic interactions between clay particles are critical for the flocculation process, which forms bridges between clay particles and leading to floc formation (Mueller 2015).
Anthropogenic Litter and Bacterial Microbiotic Particles
A characteristic example of anthropogenic litter is microplastic particles, which are millimeter-sized plastics that have been detected in aquatic ecosystems worldwide. Numerous microbial organisms adhere to microplastics, forming biofilms (the microbial plastisphere) (Reisser et al., 2014; Galafassi et al., 2021). In Chinese rivers, bacteria such as Flavobacterium, Pseudomonas (γ-proteobacteria), Rhodoferax (β-proteobacteria) Janthinobacterium (β-proteobacteria) are enriched on microplastics when compared with water and sediment without microplastics (Hu et al., 2021). The growing ensemble of microplastics in water likely constitutes a novel ecological niche, which is exploited by bacterial biofilms hosting specific communities that differ from the surrounding planktonic ones (Sathicq et al., 2021).
Interactions Among Microbiotic Particles in Soil-Water Environments: Bacterial Coalescence and Dispersal
All of the previously mentioned microbiotic particles of varying origins can interact, aggregate, and eventually exchange microbial populations (Figure 1). The frequency of interparticle interactions depends both on the particle’s local density and the dynamics of the local environment (Rahlff et al., 2021). The natural and anthropogenic areas where soil and water coalesce (such as coastal waters, particularly those with significant tides, rivers and estuaries, the sea bottom, lakes and river beds, agricultural irrigation channels, mining and tunnelling water and mud flows, snow break streams, and waterfalls) constitute the main source of soil particles where bacteria find opportunities to establish compartmentalized biospaces (Figure 2). This soil-water coalescence can be stimulated by occasional events, such as flooding, tsunamis, mud-tsunamis, accelerated deep sea currents, and heavy winds of terrestrial origin, including dust storms that seed soil particles onto bodies of water (Behzad et al., 2018; Choufany et al., 2021; Pérez-Valdespino et al., 2021; Suhadolnik et al., 2021).
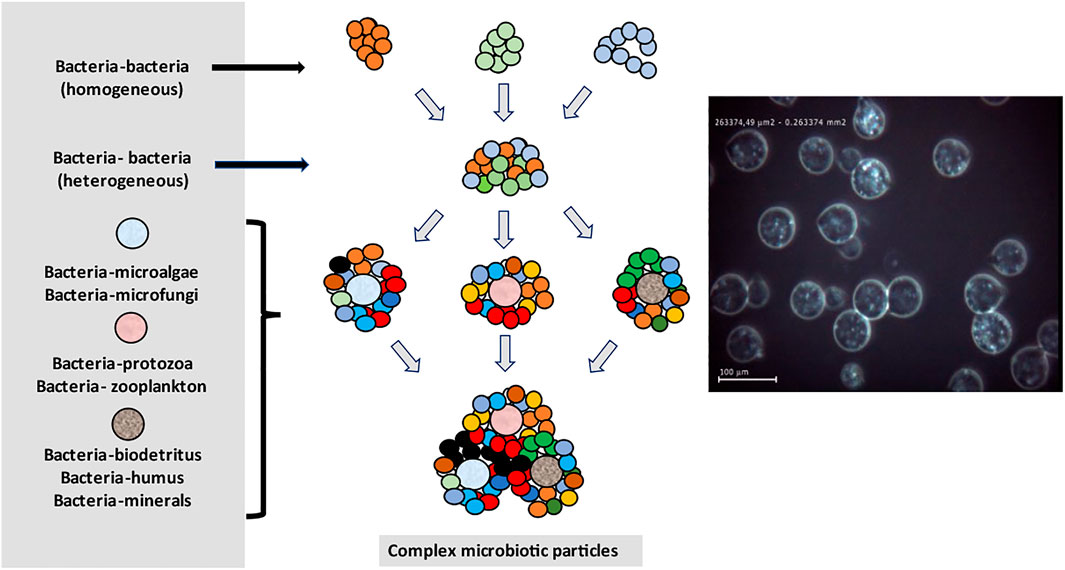
FIGURE 1. Formation of microbiotic particles. Interbacterial clumps are formed by the aggregation of cells of the same population (homogeneous clumps) or coaggregation of cells from different species (heterogeneous clumps). Particles can be formed from the bacteria’s (or bacterial clumps) interaction in water and soil with microalgae, microfungi, protozoa, zooplankton, biodetritus, humus and mineral particles. Complex coaggregates result from the merging of these particles. The circles in the figure represent various bacterial species and populations. The black circles represent antibiotic-resistant bacterial populations that can make other populations antibiotic-resistant by genetic transfer, which in turn can make their neighbors resistant, eventually including pathogenic microorganisms. The image on the right side of the figure shows alginate-chitosan beads microencapsulating natural clumps of microorganisms measuring less than 100 nm in width, which are present in diluted human intestinal microbiota. Alginate bead technology might be useful for trapping, isolating, and studying microbiotic particles of particular sizes.
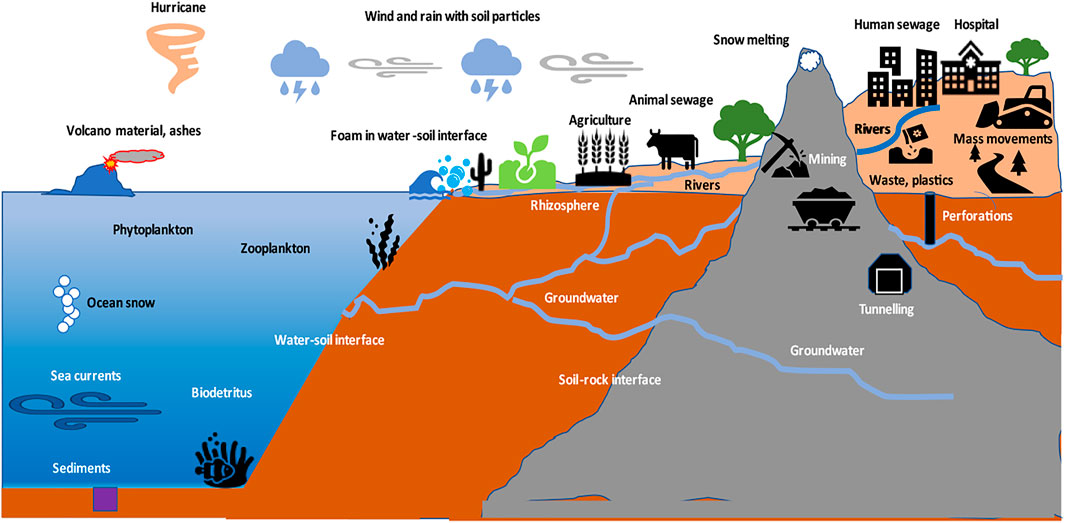
FIGURE 2. The origin and flow of microbiotic particles. Particles composed of or aggregating bacterial populations are generated in biological microparticles in water and soil (including phytoplankton, zooplankton, soil particles and biodetritus), which coalesce in soil-water interfaces. Soil-water interactions among particles are favored by natural events (such as floods, tsunamis, volcanic activity, rain, melting snow, heavy winds and material transport by seas, rivers, and groundwater) and anthropogenic activities (human wastewater, agriculture, farming, mining, tunnelling, mass transportation by roads and urbanization activities). The coalescence of microbiotic particles from different origins favors heterogeneous bacterial coaggregation, resulting in the potential genetic transfer of antibiotic resistance genes.
Landslides are a significant source of particles and include mobile intrusions produced by rapid snowmelt, intense rainfall, earthquakes, volcanic eruption, storm waves, rapid erosion, and submarine landslides (Walker and Shiels 2012). Microbial biodiversity in water is clearly increased by the insertion of microbes from soil (Crump et al., 2012). Soil particles can mix with water during extended periods (mud formation), leading to increased microbial activity, reproduction, and community mixing (Parvathi et al., 2019). Eruptions of mud and slurry (mud volcanoes) drastically affect bacterial and microeukaryotic communities (Coelho et al., 2016), while volcanic ash falling into the sea supports a diverse bacterial community (Witt et al., 2017). The role of anthropogenic soil-mass movements (including agricultural and mining activities, urbanization, and road and park construction) in dispersing bacterial-colonizable particles should not be underestimated, (Jaboyedoff et al., 2016).
The effect of climate change on these water-soil-air exchange processes is an important issue. Warmer and drier periods cause the retention of soil microbioparticles that can then be dispersed by more frequent air and water-runoff events (Fröhlich-Nowoisky et al., 2016). Airborne particles originate mostly from soil, and many of them contain microorganisms, which form the so-called “aerobiome” (de Groot et al., 2021). Microbes in these particles have a strong interaction with those of water bacterioneuston, the community of bacteria located in the thin layer between water and air (Cunliffe et al., 2009; Hervas and Casamayor 2009), particularly in foam interphases, favored by surface-active substances. Marine foam can contain a high abundance of Proteobacteria including bacterioneuston organisms such as the γ-proteobacteria Pseudoalteromonas and Vibrio (Rahlff et al., 2021).
Most particles of sufficient size (“large villages”) have a stratified bacterial community structure, with deep, highly stable residents and more mobile (exchangeable) populations on the surface (Bailey et al., 2013). Such mobility is certainly reduced under dry conditions, and significant variations in moisture can occur periodically or intermittently. Not much is known about the consequences of the drying process; in principle, however, Gram-positive bacteria should retain viability, whereas Gram-negative bacteria, such as Enterobacterales, which have a lower salt tolerance (Brown 1976), will be subjected to water stress and can decline in number. However, certain Gram-negative bacteria have adapted to periodic water stress by excreting glycoproteins and polysaccharides. The exopolysaccharide matrix surrounds numerous cells in a biofilm, which can buffer cells against either desiccation or rapid changes in water potential (Decho and Gutierrez 2017) This adaptation is probably one of the main functions of alginate, which can retain moisture and ensure viability in dry conditions (Gacesa 1998; Marshall et al., 2019), resulting in the formation of a biofilm under which coexisting bacteria might be spared from extinction. Alginate also provides osmotolerance, probably by intracellular accumulation (Sá et al., 2019).
Most importantly, synthase-dependent exopolysaccharides such as cellulose, acetylated cellulose, poly-β-1,6-N-acetyl-D-glucosamine, and Pel contribute to the coating of colonized soil particles, facilitating the formation of interparticle aggregates where subsequent incoming bacterial populations and communities can attach (Kindler et al., 2006; Vu et al., 2009; Colica et al., 2014; Limoli et al., 2015; Decho and Gutierrez 2017; Costa et al., 2018). Biofilm formation on particles facilitates genetic exchange between organisms originating from different environments. Genes encoding exopolymer substances such as alginate (also Pel) can be shared by phylogenetically (and ecologically) different Proteobacteria organisms, probably resulting from horizontal gene transfer in a shared biofilm (Bundalovic-Torma et al., 2020).
Particular attention should be paid to predicting “microbial hotspots” in soil, where plant-associated microbiotic particles can bloom within short periods (Kuzyakov and Blagodatskaya 2015). These ephemeral hotspots also occur in foam particles (consisting of foam beads) on the sea surface (Rahlff et al., 2021). It would be useful to construct a “grammar of interactions” among microbiotic particles, which should consider not only the study of classic species prototype strains, but the local ecotypes or functionally equivalent organisms that help stabilize the populational structure of the microbial community to preserve the same role despite environmental changes (García-García et al., 2019).
Microbiotic Particles Dispersal by Marine Snow
In water systems, the cumulative process of microbiotic particle aggregation is depicted by macroscopic aggregates (from 0.5 mm to a few centimeters in diameter), also known as marine snow (or lake snow), which sink in water at variable speeds (Alldredge and Silver 1988). Microbiotic particles in marine snow frequently include fungi (Bochdansky et al., 2017). In fact, marine snow is a complex microhabitat containing a diversity of bacterial lineages such Planctomycetes, Firmicutes, Bacteroides, and the α, γ δ, and ε classes of Proteobacteria (Rath et al., 1998). This biological richness influences the processes of microbial photosynthesis, decomposition, and nutrient regeneration, constituting an actual snow microcosm (Azam and Long 2001). Typically, the microorganisms contained in marine snow are subjected to successional changes derived from earlier processes, eventually leading to disaggregation and sinking kinetics (Alldredge et al., 1990). Bacterial succession can also result from the specific amensalism mediated by antimicrobial substances (Grossart et al., 2003), as occurs in other natural microbiota (Baquero et al., 2019). In addition to the vertical migration of marine snow (Mestre et al., 2018; Sanz-Sáez et al., 2020) and suspended sediment aggregates, horizontal migration associated with currents and water flow (Simon et al., 2002) also occurs, contributing to the dispersal of the constituent microbiotic particles and to the new potential associations with other microbial communities (Droppo 2001). The end result is the dissemination of microorganisms to distant environments (Hooper et al., 2008) (Figures 1, 2).
Micro and macroaggregation processes determine the bacterial content of sediment in water, particularly of the benthic zone, where soil and water coalesce (Simon et al., 2002; Wotton 2007). In fact, particle-attached bacterial communities in the deep ocean have a particular lifestyle as compared with free-living organisms (Acinas et al., 2021).
Evolution in Compartmentalized Water Biospaces
Several models have been established to study evolution in compartmentalized biospaces. A number of particulate biotic interactions tend to be fixed by evolution, such as algae-bacteria interactions (Ramanan et al., 2016). Experiments with artificial microcapsules, made with an alginate-chitosan “membrane” (Figure 1) have shown that compartmentalization in particles can contribute to the preservation of diversity (Zadorin et al., 2019), evading the dominance of a single organism or genotype resulting from periodic selection that occurs in homogeneous environments (Atwood et al., 1951). The preservation of diversity in particles promotes genetic drift and therefore maintains potentially adaptive changes that are lost in mixed planktonic environments (Baquero et al., 2021). In addition, particulation of communities ensures that various genotypes can be subjected to environmental stress, contributing to the overall evolvability of natural populations (Baquero 2009; Rocca et al., 2019). Cells of the same species can have different adaptive needs in different ecological subcompartments. Connectivity with particles coated with communities of local organisms facilitates adaptive evolution; as an example, the evolution (diversification) of Shewanella differs between the upper ocean and the abyssal zones (Tang et al., 2021).
Bacterial populations tend to form closed compartments, such as colonies and biofilms attached to biotic and abiotic surfaces. There are likely surface recognition signals leading to these multicellular structures (Troselj et al., 2018; Kimkes and Heinemann 2020). Such dense population organizations have an internal structure with layers of cells in various physiological stages, “compartments within compartments” (You et al., 2019), which can release organisms outside the compartment, as occurs when planktonic cells are released from sessile populations in biofilms (Bester et al., 2009). It has been proposed that evolution in biofilms generates greater genetic diversity than mixed planktonic environments, and this enhanced diversity leads to differing pathways of antibiotic resistance (Santos-Lopez et al., 2019). In fact, the biology of surface microbes frequently favors local differentiation, including the emergence of antibiotic-resistant mutations (Rainey and Travisano 1998; Oliver et al., 2000).
Compartments can be occupied by multiple species, and there are multispecies biofilms in natural environments (Yang et al., 2011), frequently resulting from spontaneous coaggregation (Rickard et al., 2003). The mechanisms by which densely populated compartments, such as biofilms, are invaded by external microorganisms (which can become part of the consortium) is an interesting topic that has recently been modeled, showing the importance of species concentrations on the biofilm’s free boundary (D’Acunto et al., 2014). In any case, such dense aggregation of diverse populations facilitates horizontal gene transfer (including the transfer of antibiotic resistance genes), and it has been proposed that biofilm communities in water environments are hot spots for gaining adaptive traits (Zadorin et al., 2019; Abe et al., 2020).
Particulation by Microbial Coaggregation: Functional Ensembles?
Coaggregation refers to a process by which individual microbial cells, either from a single clonal lineage or species or from different species, recognize and attach to one another (London and Kolenbrander 1996; Rickard et al., 2003). The clues for recognition are not yet fully understood but most probably are the result of natural selection and should involve a type of surface recognition code, probably involving lectins and polysaccharide interactions, resembling the DNA codes used in protein synthesis (Baquero 2014). Clumps or coaggregates involving two types of cells are formed immediately upon mixing the partner populations, a phenomenon particularly studied in oral plaque microbial consortia (Kolenbrander et al., 1993). Various methods have been evaluated to measure coaggregation (Kinder and Holt 1994). Quantitative spectrophotometry (based on flocculation) has shown that coaggregation occurs weakly among bacteria from different sites, such as oral and intestinal species (Ledder et al., 2008). A complete “grammar of interactions” is not yet available but will be critical for understanding the niche’s coalescence and the resulting merging of the microbiome, which could be described as a multidimensional network (Baquero et al., 2019, Baquero et al., 2021). Certain species serve as bridging “nodes” to which other species attach, as has been shown with Acinetobacter species in water bodies, from activated sludge (Malik et al., 2003) to drinking water (Simões et al., 2008), and with Blastomonas in fresh water (Rickard et al., 2002; Afonso et al., 2021). Extracellular polymers play an important role in the coaggregation of aquatic biofilms (Hede and Khandeparker 2020). There is an “ecology of coaggregation”, such that the process can be modified by variations in external physical and chemical factors (Oki et al., 2018). In general, however, coaggregation ensures the permanence (resilience) of species-species interactions in fluctuating environments.
Does a reproducible coaggregate act as a single individual biological unit? Are stable coaggregates endowed with particular organism-like traits? There are certainly chemical interactions among members of multispecies biofilms (Yang et al., 2011; Burmølle et al., 2014; Liu et al., 2016). It has been shown that gene expression in the partner species can be modified by coaggregation (Jakubovics et al., 2008), and the coaggregate can therefore be a source of emerging properties from a social individuality (Sadiq et al., 2021). The main difficulty in predicting the composition of microbiotic particles is due to the multistability in multispecies communities, combined with ecological noise (Wright et al., 2021).
Microbiotic Aggregates and Antimicrobial Resistance
Antibiotics and antibiotic resistance genes originate and are present in water and soil environments (Martínez 2008; Cabello and Godfrey 2018). In recent decades (1970’s–2000’s) the abundance of antibiotic resistance genes in European archived soils have increased 10-fold (Knapp et al., 2010). Microbiotic aggregates in soil and water environments have a considerable influence on the emergence and evolution of antimicrobial resistance (Baquero et al., 2021) for several reasons:
(1) Efficient antibiotic interactions require close cell-cell physical neighborhoods (Burmølle et al., 2014), even cell-to-cell contact (Lemonnier et al., 2008).
(2) Non-aggregated antibiotic producers do not reach the critical density to ensure antibiosis, and non-aggregated susceptible organisms do not reach a number that ensures the acquisition of mutations or the acquisition of foreign resistance genes, which in some cases respond to quorum sensing.
(3) Cell-to-cell contact facilitates the interbacterial horizontal gene transfer of antibiotic resistance genes, including transformation, conjugation (plasmids, integrative-conjugative elements) and, particularly in soils and marine habitats, DNA-packing extracellular vesicles and DNA transfer through intercellular nanotubes (Woegerbauer et al., 2020).
(4) “Functionally equivalent” bacterial species tend to cluster in the same type of aggregates. Moreover, kin recognition favors the horizontal transfer of antibiotic resistance genes (Baquero et al., 2019). Most microbial organisms present in water and soil aggregates and, significantly, species from all branches of the Proteobacteria phylum (most antibiotic-resistant Gram-negative pathogens belong to γ-proteobacteria) can exchange genes, including antibiotic resistance and metal resistance genes (Kloesges et al., 2011; Pohl et al., 2014). Horizontal gene transfer is frequent in the ocean (McDaniel et al., 2010; Hemme et al., 2016).
(5) Most organisms producing antibiotics have a soil or water origin. The genus Streptomyces (Actinobacteria in general) is one of the self-aggregated bacterial organisms most frequently found in soil and water. Interestingly, this class of organisms is the main source (at least two-thirds) of the antibiotic groups used for treating infections, including aminoglycosides, beta-lactams and beta-lactamase inhibitors, tetracyclines, macrolides, lincosamides, streptogramins, phenicols, rifamycins, fosfomycins, glycopeptides, novobiocin, daptomycin, and platensimycin. These antibiotics are only the tip of the iceberg when it comes to detected antimicrobial compounds (Mast and Stegmann 2019). Auto-aggregation of dividing cells is probably an evolutionary strategy to produce locally sufficient concentrations of bioactive compounds as antibiotics, either acting as antimicrobials or as intermicrobial signaling agents (Linares et al., 2006). In addition, there is always the possibility of transferring antibiotic resistance genes from antibiotic producers to pathogens (Jiang et al., 2017). In water, organisms such as Shewanella are frequently part of coaggregates and might be involved in the spread of antibiotic resistance (Rickard et al., 2003; Cabello and Godfrey 2018).
(6) Antimicrobials of anthropogenic origin extensively pollute soil and water environments. They tend to accumulate in particulate material (Baquero et al., 2008; Rodriguez-Mozaz et al., 2020; Huang et al., 2021), retaining their antibacterial activities and consequently selecting, even at very low concentrations, for antibiotic resistant bacteria (Chander et al., 2005).
(7) The most dangerous type of microbiotic particles involved in antibiotic resistance are those resulting from human and animal fecal pollution of water and soil, including those originating in waste water treatment plants (Karkman et al., 2018; Pärnänen et al., 2019). Large-scale wastewater treatment plants discharge hundreds of tons of total suspended particles into bodies of water every year, and antibiotic resistance genes are perpetuated in the sediments (Brown et al., 2018). However, a significant decay of resistance genes can occur over time in some environments (Brown et al., 2020). Microbiotic particles based on microplastics contribute to the emergence and spread of antibiotic resistance (Wang et al., 2020; Hu et al., 2021). However, the relative importance of microplastic biotic particles is dependent on the inoculation environment and the weight of their contributions can vary by location (Galafassi et al., 2021).
The effect of antibiotic anthropogenic pollution has penetrated into the deepest region of the oceans, such as the Mariana Trench, where antibiotic resistance genes of possible human or animal origin have been detected (Yang et al., 2021). In fact, an ocean resistome, with an ensemble of antibiotic resistance genes, is now available, which includes genes conferring resistance to some of the most relevant clinical antibiotics, some of which are particularly abundant in specific geographic locations (Cuadrat et al., 2020). Further research is needed to correlate these findings with the density of the various microbiotic particles.
Counteracting Antibiotic Resistance by Controlling Microbiotic Particles
How can we counteract the dangerous spread of antibiotic resistance mediated by water-soil particles? It appears to be an almost impossible task at the global scale, but some local interventions can be effective. The density and distribution of the particles might bias surveillance results focusing on bacterial fecal pollution. The density of suspended particulate matter influences the recovery of fecal indicator bacteria, and this “local factor” should be taken into account (Perkins et al., 2016). Contamination of water bodies with human and animal microbiotic particles containing antibiotic resistance is highly dependent on the socioeconomic status of the country. This access to particles, based on a lack of proper sanitation procedures, is probably more important than antibiotic consumption in shaping the local rate of resistance in human and animal pathogens (Collignon et al., 2018).
Removal of water microbiotic particles is an essential step to decontaminating the environment from antibiotic resistance (Kumar and Pal 2018; Liang et al., 2021). Various procedures have been proposed (Lawler 1986) or are under investigation for removal or deactivation of particles in water. In addition to the classic sedimentation, flocculation, coagulation, or disinfection process, filtration and ultrafiltration, as well as cold atmospheric plasma technology (Kim and Dempsey 2008), hydrodynamic vortex separators (Gronowska-Szneler and Sawicki 2014); dissolved air flotation (Han et al., 2007; El-Kalliny et al., 2021) and other procedures will be necessary. Nanoparticle-based biotechnology is a promising field. It includes “insertion” in the natural process of aggregation of nanoparticles that recognize particular microorganisms (even in biofilms) and kill them, such as those synthesized from natural organic matter (mostly composed of humic and fulvic acids) and silver or gold particles, which are then released into the environment. The use of nanoparticles with zinc oxide and titanium dioxide in combination with halophilic bacteria (which reduce nutrients) has been proposed to reduce the biological part of microbiotic particles (Weber et al., 2021). However, the environmental safety of nanoparticles remains under discussion (Hajipour et al., 2021). Other suggested approaches include the use of “environmental probiotics” such as Pseudoalteromonas, with antibiofilm activity (Dheilly et al., 2010) or particular types of natural clay minerals with antimicrobial and antibiofilm effects (Behroozian et al., 2020).
Final Remarks on Microbiotic Particles in Planetary Health
The preservation of a healthy equilibrium among biological and chemical constituents of Earth and human populations is a main proposition of the One Health and Global Health approaches; and in fact, of Planetary Health (Myers 2017; Hernando-Amado et al., 2019). Such equilibrium can be altered by human interventions, as can be observed by the deleterious changes in the chemical and biological composition of the oceans (Pedrós-Alió et al., 2021). Therefore, robust counter-interventions are required to ensure that Earth’s organisms are maintained in a homeostatic, constant internal state despite perturbations from their surroundings (Tang and Mcmillen 2016). From a Planetary Health perspective, we can propose that the dynamic network of interactions among microbiotic particles in the soil and water constitute a linking material, a kind of cement for a unified life-holobiont, where everything depends on everything else (Davies 2009). The analysis and characterization of such a system requires further research, which should lead to suitable corrective interventions to ensure the future of our common well-being.
Author Contributions
All authors listed have made a substantial, direct, and intellectual contribution to the work and approved it for publication.
Funding
This work was supported by the European Commission (JPIAMR 2021-13th call, MISTAR project) and funded by Instituto de Salud Carlos III (ISCIII), PI18/01942, PI21/02027, AC21_2/0004, CIBERINFEC (CB21/13/00084), CIBERESP (CB06/02/0053), and the Regional Government of Madrid (InGeMICS-B2017/BMD-3691); and co-funded by the European Union (Next Generation EU) and European Regional Development Fund “A way to make Europe”. NG was supported by a contract associated with PI18/01942.
Conflict of Interest
The authors declare that the research was conducted in the absence of any commercial or financial relationships that could be construed as a potential conflict of interest.
Publisher’s Note
All claims expressed in this article are solely those of the authors and do not necessarily represent those of their affiliated organizations, or those of the publisher, the editors, and the reviewers. Any product that may be evaluated in this article, or claim that may be made by its manufacturer, is not guaranteed or endorsed by the publisher.
References
Abe, K., Nomura, N., and Suzuki, S. (2020). Biofilms: Hot Spots of Horizontal Gene Transfer (HGT) in Aquatic Environments, with a Focus on a New HGT Mechanism. FEMS Microbiol. Ecol. 96, 31. doi:10.1093/FEMSEC/FIAA031
Abell, G. C. J., and Bowman, J. P. (2005). Colonization and Community Dynamics of Class Flavobacteria on Diatom Detritus in Experimental Mesocosms Based on Southern Ocean Seawater. FEMS Microbiol. Ecol. 53, 379–391. doi:10.1016/J.FEMSEC.2005.01.008
Abu-Lail, L. I., Liu, Y., Atabek, A., and Camesano, T. A. (2007). Quantifying the Adhesion and Interaction Forces between Pseudomonas aeruginosa and Natural Organic Matter. Environ. Sci. Technol. 41, 8031–8037. Available at: https://www.academia.edu/6978358/Quantifying_the_Adhesion_and_Interaction_Forces_Between_Pseudomonas_aeruginosa_and_Natural_Organic_Matter (Accessed November 22, 2021). doi:10.1021/es071047o
Acinas, S. G., Sánchez, P., Salazar, G., Cornejo-Castillo, F. M., Sebastián, M., Logares, R., et al. (2021). Deep Ocean Metagenomes Provide Insight into the Metabolic Architecture of Bathypelagic Microbial Communities. Commun. Biol. 4. doi:10.1038/S42003-021-02112-2
Adlakha, J., Karamichali, I., Sangwallek, J., Deiss, S., Bär, K., Coles, M., et al. (2019). Characterization of MCU-Binding Proteins MCUR1 and CCDC90B - Representatives of a Protein Family Conserved in Prokaryotes and Eukaryotic Organelles. Structure 27, 464–475. e6. doi:10.1016/J.STR.2018.11.004
Afonso, A. C., Gomes, I. B., Saavedra, M. J., Giaouris, E., Simões, L. C., and Simões, M. (2021). Bacterial Coaggregation in Aquatic Systems. Water Res. 196, 117037. doi:10.1016/j.watres.2021.117037
Alldredge, A. L., Granata, T. C., Gotschalk, C. C., and Dickey, T. D. (1990). The Physical Strength of marine Snow and its Implications for Particle Disaggregation in the Ocean. Limnol. Oceanogr. 35, 1415–1428. doi:10.4319/LO.1990.35.7.1415
Alldredge, A. L., and Silver, M. W. (1988). Characteristics, Dynamics and Significance of marine Snow. Prog. Oceanography 20, 41–82. doi:10.1016/0079-6611(88)90053-5
Amin, S. A., Parker, M. S., and Armbrust, E. V. (2012). Interactions between Diatoms and Bacteria. Microbiol. Mol. Biol. Rev. 76, 667–684. doi:10.1128/MMBR.00007-12
Ardré, M., Dufour, D., and Rainey, P. B. (2019). Causes and Biophysical Consequences of Cellulose Production by Pseudomonas fluorescens SBW25 at the Air-Liquid Interface. J. Bacteriol. 201 (18), e00110–19.
Arenhart, J. R. B. (2019). “Bridging the gap between Science and Metaphysics, with a Little Help from Quantum Mechanics,” in Proceedings of the 3rd Filomena Workshop, Natal (Brazil), August 21–23, 2017. Available at: https://www.academia.edu/39755699/Bridging_the_gap_between_science_and_metaphysics_with_a_little_help_from_quantum_mechanics (Accessed November 20, 2021).
Atwood, k. C., Schneider, l. K., and Ryan, f. J. (1951). Periodic Selection in Escherichia Coli. Proc. Natl. Acad. Sci. U.S.A. 37, 146–155. doi:10.1073/PNAS.37.3.146
Bahram, M., Hildebrand, F., Forslund, S. K., Anderson, J. L., Soudzilovskaia, N. A., Bodegom, P. M., et al. (2018). Structure and Function of the Global Topsoil Microbiome. Nature 560, 233–237. doi:10.1038/S41586-018-0386-6
Bailey, V. L., McCue, L. A., Fansler, S. J., Boyanov, M. I., DeCarlo, F., Kemner, K. M., et al. (2013). Micrometer-scale Physical Structure and Microbial Composition of Soil Macroaggregates. Soil Biol. Biochem. 65, 60–68. doi:10.1016/J.SOILBIO.2013.02.005
Baquero, F., Coque, T. M., Martínez, J.-L., Aracil-Gisbert, S., and Lanza, V. F. (2019). Gene Transmission in the One Health Microbiosphere and the Channels of Antimicrobial Resistance. Front. Microbiol. 10, 2892. doi:10.3389/FMICB.2019.02892/BIBTEX
Baquero, F. (2009). Environmental Stress and Evolvability in Microbial Systems. Clin. Microbiol. Infect. 15, 5–10. doi:10.1111/J.1469-0691.2008.02677.X
Baquero, F. (2005). Evolution and the Nature of Time. Int. Microbiol. 8, 81–91. PubMed. Available at: https://pubmed.ncbi.nlm.nih.gov/16052456/(Accessed November 20, 2021).
Baquero, F. (2014). “Genetic Hyper-Codes and Multidimensional Darwinism: Replication Modes and Codes in Evolutionary Individuals Od the Bacteria World,” in Why Does Evolution Matter?:the Importance of Understanding Evolution. Editor G. Trueba (Newcastle Upon Tyne, United Kingdom: Cambridge Scholars Publishing), 165–180.
Baquero, F., Martínez, J.-L., and Cantón, R. (2008). Antibiotics and Antibiotic Resistance in Water Environments. Curr. Opin. Biotechnol. 19, 260–265. doi:10.1016/J.COPBIO.2008.05.006
Baquero, F., Martínez, J. L., Lanza, V. F., Rodríguez-Beltrán, J., Galán, J. C., San Millán, A., et al. (2021). Evolutionary Pathways and Trajectories in Antibiotic Resistance. Clin. Microbiol. Rev. 34, e0005019. doi:10.1128/CMR.00050-19
Bassler, J., Hernandez Alvarez, B., Hartmann, M. D., and Lupas, A. N. (2015). A Domain Dictionary of Trimeric Autotransporter Adhesins. Int. J. Med. Microbiol. 305, 265–275. doi:10.1016/J.IJMM.2014.12.010
Behroozian, S., Svensson, S. L., Li, L. Y., and Davies, J. E. (2020). Broad-Spectrum Antimicrobial and Antibiofilm Activity of a Natural Clay Mineral from British Columbia, Canada. mBio 11, 1–14. doi:10.1128/MBIO.02350-20
Behzad, H., Mineta, K., and Gojobori, T. (2018). Global Ramifications of Dust and Sandstorm Microbiota. Genome Biol. Evol. 10, 1970–1987. doi:10.1093/GBE/EVY134
Bell, W., and Mitchell, R. (1972). Chemotactic and Growth Responses of marine Bacteria to Algal Extracellular Products. Biol. Bull. 143, 265–277. doi:10.2307/1540052
Bester, E., Edwards, E. A., and Wolfaardt, G. M. (2009). Planktonic Cell Yield Is Linked to Biofilm Development. Can. J. Microbiol. 55, 1195–1206. doi:10.1139/W09-075
Biddanda, B., and Pomeroy, L. (1988). Microbial Aggregation and Degradation of Phytoplankton-Derived Detritus in Seawater. I. Microbial Succession. Mar. Ecol. Prog. Ser. 42, 79–88. doi:10.3354/MEPS042079
Bochdansky, A. B., Clouse, M. A., and Herndl, G. J. (2017). Eukaryotic Microbes, Principally Fungi and Labyrinthulomycetes, Dominate Biomass on Bathypelagic marine Snow. ISME J. 11, 362–373. doi:10.1038/ISMEJ.2016.113
Brock, D. A., Douglas, T. E., Queller, D. C., and Strassmann, J. E. (2011). Primitive Agriculture in a Social Amoeba. Nature 469 (7330), 393–396. doi:10.1038/nature09668
Brown, A. D. (1976). Microbial Water Stress. Bacteriol. Rev. 40, 803–846. doi:10.1128/BR.40.4.803-846.1976
Brown, P. C., Borowska, E., Peschke, R., Schwartz, T., and Horn, H. (2020). Decay of Elevated Antibiotic Resistance Genes in Natural River Sediments after Sedimentation of Wastewater Particles. Sci. Total Environ. 705, 135861. doi:10.1016/J.Scitotenv.2019.135861
Brown, P. C., Borowska, E., Schwartz, T., and Horn, H. (2019). Impact of the Particulate Matter from Wastewater Discharge on the Abundance of Antibiotic Resistance Genes and Facultative Pathogenic Bacteria in Downstream River Sediments. Sci. Total Environ. 649, 1171–1178. doi:10.1016/j.scitotenv.2018.08.394
Bundalovic-Torma, C., Whitfield, G. B., Marmont, L. S., Howell, P. L., and Parkinson, J. (2020). A Systematic Pipeline for Classifying Bacterial Operons Reveals the Evolutionary Landscape of Biofilm Machineries. Plos Comput. Biol. 16 (4), e1007721. doi:10.1371/journal.pcbi.1007721
Burkart, W. (2000). Compartmentalization in Environmental Science and the Perversion of Multiple Thresholds. Sci. Total Environ. 249, 63–72. doi:10.1016/S0048-9697(99)00511-2
Burmølle, M., Ren, D., Bjarnsholt, T., and Sørensen, S. J. (2014). Interactions in Multispecies Biofilms: Do They Actually Matter? Trends Microbiol. 22, 84–91. doi:10.1016/J.TIM.2013.12.004
Cabello, F. C., and Godfrey, H. P. (2018). Aquaculture, Exaptation, and the Origin of Mcr -Positive Colistin Resistance. Antimicrob. Agents Chemother. 62. doi:10.1128/AAC.01903-18
Cai, Y. M. (2020). Non-surface Attached Bacterial Aggregates: A Ubiquitous Third Lifestyle. Front. Microbiol 11, 3106. doi:10.3389/FMICB.2020.557035/BIBTEX
Carter, M. R., and Gregorich, E. G. (2007). Soil Sampling and Methods of Analysis. Boca Ratón, FL: CRC Press, Taylor and Francis. doi:10.1201/9781420005271
Chander, Y., Kumar, K., Goyal, S. M., and Gupta, S. C. (2005). Antibacterial Activity of Soil-Bound Antibiotics. J. Environ. Qual. 34, 1952–1957. doi:10.2134/JEQ2005.0017
Choufany, M., Martinetti, D., Soubeyrand, S., and Morris, C. E. (2021. Inferring Long-Distance Connectivity Shaped by Air-Mass Movement for Improved Experimental Design in Aerobiology. Sci. Rep. 11, 1–10. doi:10.1038/s41598-021-90733-2
Cirri, E., and Pohnert, G. (2019). Algae−bacteria Interactions that Balance the Planktonic Microbiome. New Phytol. 223, 100–106. doi:10.1111/NPH.15765
Coelho, F. J. R. C., Louvado, A., Domingues, P. M., Cleary, D. F. R., Ferreira, M., Almeida, A., et al. (2016). Integrated Analysis of Bacterial and Microeukaryotic Communities from Differentially Active Mud Volcanoes in the Gulf of Cadiz. Sci. Rep. 6, 35272. doi:10.1038/SREP35272
Colica, G., Li, H., Rossi, F., Li, D., Liu, Y., and De Philippis, R. (2014). Microbial Secreted Exopolysaccharides Affect the Hydrological Behavior of Induced Biological Soil Crusts in Desert sandy Soils. Soil Biol. Biochem. 68, 62–70. doi:10.1016/j.soilbio.2013.09.017
Collignon, P., Beggs, J. J., Walsh, T. R., Gandra, S., and Laxminarayan, R. (2018). Anthropological and Socioeconomic Factors Contributing to Global Antimicrobial Resistance: a Univariate and Multivariable Analysis. Lancet Planet. Health 2, e398–e405. doi:10.1016/S2542-5196(18)30186-4/ATTACHMENT/7900DD52-E610-4B3F-85A0-581FE7E097B2/MMC1.PDF
Cooper, R. O., and Cressler, C. E. (2020). Characterization of Key Bacterial Species in the Daphnia magna Microbiota Using Shotgun Metagenomics. Sci. Rep. 10, 1–11. doi:10.1038/s41598-019-57367-x
Costa, O. Y. A., Raaijmakers, J. M., and Kuramae, E. E. (2018). Microbial Extracellular Polymeric Substances: Ecological Function and Impact on Soil Aggregation. Front. Microbiol. 9, 1636. doi:10.3389/fmicb.2018.01636
Crump, B. C., Amaral-Zettler, L. A., and Kling, G. W. (2012). Microbial Diversity in Arctic Freshwaters Is Structured by Inoculation of Microbes from Soils. ISME J. 6, 1629–1639. doi:10.1038/ismej.2012.9
Cuadrat, R. R. C., Sorokina, M., Andrade, B. G., Goris, T., and Dávila, A. M. R. (2020). Global Ocean Resistome Revealed: Exploring Antibiotic Resistance Gene Abundance and Distribution in TARA Oceans Samples. GigaScience 9, 1–12. doi:10.1093/GIGASCIENCE/GIAA046
Cunliffe, M., Whiteley, A. S., Newbold, L., Oliver, A., Schäfer, H., and Murrell, J. C. (2009). Comparison of Bacterioneuston and Bacterioplankton Dynamics during a Phytoplankton Bloom in a Fjord Mesocosm. Appl. Environ. Microbiol. 75, 7173–7181. doi:10.1128/AEM.01374-09
D’Acunto, B., Frunzo, L., Klapper, I., and Mattei, M. R. (2015). Modeling Multispecies Biofilms Including New Bacterial Species Invasion. Math. Biosciences 259, 20–26. doi:10.1016/j.mbs.2014.10.009
Damare, S., and Raghukumar, C. (2008). Fungi and Macroaggregation in Deep-Sea Sediments. Microb. Ecol. 56, 168–177. doi:10.1007/S00248-007-9334-Y
Davies, J. (2009). Everything Depends on Everything Else. Clin. Microbiol. Infect. 15, 1–4. doi:10.1111/j.1469-0691.2008.02682.x
de Corte, D., Srivastava, A., Koski, M., Garcia, J. A. L., Takaki, Y., Yokokawa, T., et al. (2018). Metagenomic Insights into Zooplankton-Associated Bacterial Communities. Environ. Microbiol. 20, 492–505. doi:10.1111/1462-2920.13944
de Groot, G. A., Geisen, S., Wubs, E. R. J., Meulenbroek, L., Laros, I., Snoek, L. B., et al. (2021). The Aerobiome Uncovered: Multi-Marker Metabarcoding Reveals Potential Drivers of Turn-Over in the Full Microbial Community in the Air. Environ. Int. 154, 106551. doi:10.1016/J.ENVINT.2021.106551
Decho, A. W., and Gutierrez, T. (2017). Microbial Extracellular Polymeric Substances (EPSs) in Ocean Systems. Front. Microbiol. 8, 922. doi:10.3389/fmicb.2017.00922
Dheilly, A., Soum-Soutéra, E., Klein, G. L., Bazire, A., Compère, C., Haras, D., et al. (2010). Antibiofilm Activity of the marine Bacterium Pseudoalteromonas Sp. Strain 3J6. Appl. Environ. Microbiol. 76, 3452–3461. doi:10.1128/AEM.02632-09
Droppo, I. G. (2001). Rethinking what Constitutes Suspended Sediment. Hydrol. Process. 15, 1551–1564. doi:10.1002/HYP.228
El‐Kalliny, A. S., Abd‐Elmaksoud, S., El‐Liethy, M. A., Abu Hashish, H. M., Abdel‐Wahed, M. S., Hefny, M. M., et al. (2021). Efficacy of Cold Atmospheric Plasma Treatment on Chemical and Microbial Pollutants in Water. ChemistrySelect 6, 3409–3416. doi:10.1002/SLCT.202004716
Frey‐Klett, P., Garbaye, J., and Tarkka, M. (2007). The Mycorrhiza Helper Bacteria Revisited. New Phytol. 176, 22–36. doi:10.1111/J.1469-8137.2007.02191.X
Fröhlich-Nowoisky, J., Kampf, C. J., Weber, B., Huffman, J. A., Pöhlker, C., Andreae, M. O., et al. (2016). Bioaerosols in the Earth System: Climate, Health, and Ecosystem Interactions. Atmos. Res. 182, 346–376. doi:10.1016/J.ATMOSRES.2016.07.018
Gacesa, P. (1998). Bacterial Alginate Biosynthesis - Recent Progress and Future Prospects. Microbiology 144, 1133–1143. doi:10.1099/00221287-144-5-1133/CITE/REFWORKS
Galafassi, S., Sabatino, R., Sathicq, M. B., Eckert, E. M., Fontaneto, D., Dalla Fontana, G., et al. (2021). Contribution of Microplastic Particles to the Spread of Resistances and Pathogenic Bacteria in Treated Wastewaters. Water Res. 201, 117368. doi:10.1016/J.WATRES.2021.117368
García-García, N., Tamames, J., Linz, A. M., Pedrós-Alió, C., and Puente-Sánchez, F. (2019). Microdiversity Ensures the Maintenance of Functional Microbial Communities under Changing Environmental Conditions. Isme J. 13, 2969–2983. doi:10.1038/S41396-019-0487-8
Gronowska-Szneler, M. A., and Sawicki, J. M. (2014). Simple Design Criteria and Efficiency of Hydrodynamic Vortex Separators. Water Sci. Technl.: A Journal Int. Assoc. Water Pollut. Res. 70, 457–463. doi:10.2166/WST.2014.245
Grossart, H.-P., Kiørboe, T., Tang, K., and Ploug, H. (2003). Bacterial Colonization of Particles: Growth and Interactions. Appl. Environ. Microbiol. 69, 3500–3509. doi:10.1128/AEM.69.6.3500-3509.2003
Grossart, H.-P., van den Wyngaert, S., Kagami, M., Wurzbacher, C., Cunliffe, M., and Rojas-Jimenez, K. (2019). Fungi in Aquatic Ecosystems. Nat. Rev. Microbiol. 17, 339–354. doi:10.1038/s41579-019-0175-8
Hajipour, M. J., Saei, A. A., Walker, E. D., Conley, B., Omidi, Y., Lee, K. B., et al. (2021). Nanotechnology for Targeted Detection and Removal of Bacteria: Opportunities and Challenges. Adv. Sci. 8, 2100556. doi:10.1002/ADVS.202100556
Han, M., Kim, T.-i., and Kim, J. (2007). Effects of Floc and Bubble Size on the Efficiency of the Dissolved Air Flotation (DAF) Process. Water Sci. Technol. A Journal Int. Assoc. Water Pollut. Res. 56, 109–115. doi:10.2166/WST.2007.779
Hassett, B. T., Borrego, E. J., Vonnahme, T. R., Rämä, T., Kolomiets, M. v., and Gradinger, R. (2019). Arctic marine Fungi: Biomass, Functional Genes, and Putative Ecological Roles. Isme J. 13, 1484–1496. doi:10.1038/S41396-019-0368-1
Hede, N., and Khandeparker, L. (2020). Extracellular Polymeric Substances Mediate the Coaggregation of Aquatic Biofilm-Forming Bacteria. Hydrobiologia 847, 4249–4272. doi:10.1007/S10750-020-04411-X
Hemme, C. L., Green, S. J., Rishishwar, L., Prakash, O., Pettenato, A., Chakraborty, R., et al. (2016). Lateral Gene Transfer in a Heavy Metal-Contaminated-Groundwater Microbial Community. mBio 7, e02234–15. doi:10.1128/MBIO.02234-15/SUPPL_FILE/MBO001162717ST3.DOCX
Hernando-Amado, S., Coque, T. M., Baquero, F., and Martínez, J. L. (2019). Defining and Combating Antibiotic Resistance from One Health and Global Health Perspectives. Nat. Microbiol. 4, 1432–1442. doi:10.1038/S41564-019-0503-9
Hervas, A., and Casamayor, E. O. (2009). High Similarity between Bacterioneuston and Airborne Bacterial Community Compositions in a High mountain lake Area. FEMS Microbiol. Ecol. 67, 219–228. doi:10.1111/J.1574-6941.2008.00617.X
Hooper, S. D., Raes, J., Foerstner, K. U., Harrington, E. D., Dalevi, D., and Bork, P. (2008). A Molecular Study of Microbe Transfer between Distant Environments. PloS one 3, e2607. doi:10.1371/JOURNAL.PONE.0002607
Hu, H., Jin, D., Yang, Y., Zhang, J., Ma, C., and Qiu, Z. (2021). Distinct Profile of Bacterial Community and Antibiotic Resistance Genes on Microplastics in Ganjiang River at the Watershed Level. Environ. Res. 200, 111363. doi:10.1016/J.ENVRES.2021.111363
Huang, J., Zhu, J., Liu, S., Luo, Y., Zhao, R., Guo, F., et al. (2022). Estuarine Salinity Gradient Governs Sedimentary Bacterial Community but Not Antibiotic Resistance Gene Profile. Sci. Total Environ. 806, 151390. doi:10.1016/J.SCITOTENV.2021.151390
Jaboyedoff, M., Michoud, C., Derron, M.-H., Voumard, J., Leibundgut, G., Sudmeier-Rieux, K., et al. (2018). “Human-Induced Landslides: Toward the Analysis of Anthropogenic Changes of the Slope Environment,” in Landslides and Engineered Slopes. Experience, Theory and Practice (London, United Kingdom: CRC Press), 217–232. doi:10.1201/9781315375007-12
Jakubovics, N. S., Gill, S. R., Iobst, S. E., Vickerman, M. M., and Kolenbrander, P. E. (2008). Regulation of Gene Expression in a Mixed-Genus Community: Stabilized Arginine Biosynthesis in Streptococcus Gordonii by Coaggregation with Actinomyces Naeslundii. J. Bacteriol. 190, 3646–3657. doi:10.1128/JB.00088-08
Jiang, X., Ellabaan, M. M. H., Charusanti, P., Munck, C., Blin, K., Tong, Y., et al. (2017). Dissemination of Antibiotic Resistance Genes from Antibiotic Producers to Pathogens. Nat. Commun. 8, 1–7. doi:10.1038/ncomms15784
Karkman, A., Do, T. T., Walsh, F., and Virta, M. P. J. (2018). Antibiotic-Resistance Genes in Waste Water. Trends Microbiol. 26, 220–228. doi:10.1016/J.TIM.2017.09.005
Katharios-Lanwermeyer, S., Xi, C., Jakubovics, N. S., and Rickard, A. H. (2014). Mini-review: Microbial Coaggregation: Ubiquity and Implications for Biofilm Development. Biofounling 30, 1235–1251. doi:10.1080/08927014.2014.976206
Kim, H.-C., and Dempsey, B. A. (2008). Effects of Wastewater Effluent Organic Materials on Fouling in Ultrafiltration. Water Res. 42, 3379–3384. Available at: https://www.academia.edu/14831816/Effects_of_wastewater_effluent_organic_materials_on_fouling_in_ultrafiltration (Accessed November 22, 2021). doi:10.1016/j.watres.2008.04.021
Kimkes, T. E. P., and Heinemann, M. (2020). How Bacteria Recognise and Respond to Surface Contact. FEMS Microbiol. Rev. 44, 106–122. doi:10.1093/FEMSRE/FUZ029
Kinder, S. A., and Holt, S. C. (1994). Coaggregation between Bacterial Species. Methods Enzymol. 236, 254–270. doi:10.1016/0076-6879(94)36020-0
Kindler, R., Miltner, A., Richnow, H., and Kastner, M. (2006). Fate of Gram-Negative Bacterial Biomass in Soil-Mineralization and Contribution to SOM. Soil Biol. Biochem. 38, 2860–2870. doi:10.1016/J.SOILBIO.2006.04.047
Kiran, R., and Patil, S. A. (2019). “Microbial Electroactive Biofilms,” in Introduction to Biofilm Engineering (Oxford, United Kingdom: Oxford University Press), 159–186. doi:10.1021/bk-2019-1323.ch008
Kloesges, T., Popa, O., Martin, W., and Dagan, T. (2011). Networks of Gene Sharing Among 329 Proteobacterial Genomes Reveal Differences in Lateral Gene Transfer Frequency at Different Phylogenetic Depths. Mol. Biol. Evol. 28, 1057–1074. doi:10.1093/MOLBEV/MSQ297
Knapp, C. W., Dolfing, J., Ehlert, P. A. I., and Graham, D. W. (2010). Evidence of Increasing Antibiotic Resistance Gene Abundances in Archived Soils since 1940. Environ. Sci. Technol. 44, 580–587. doi:10.1021/ES901221X
Kolenbrander, P. E., Ganeshkumar, N., Cassels, F. J., and Hughes, C. v. (1993). Coaggregation: Specific Adherence Among Human Oral Plaque Bacteria. FASEB j. 7, 406–413. doi:10.1096/FASEBJ.7.5.8462782
Konhauser, K. O., and Urrutia, M. M. (1999). Bacterial clay Authigenesis: a Common Biogeochemical Process. Chem. Geology. 161, 399–413. Available at: www.elsevier.comrlocaterchemgeo (Accessed November 22, 2021). doi:10.1016/s0009-2541(99)00118-7
Kumar, A., and Pal, D. (2018). Antibiotic Resistance and Wastewater: Correlation, Impact and Critical Human Health Challenges. J. Environ. Chem. Eng. 6, 52–58. doi:10.1016/J.JECE.2017.11.059
Kuzyakov, Y., and Blagodatskaya, E. (2015). Microbial Hotspots and Hot Moments in Soil: Concept & Review. Soil Biol. Biochem. 83, 184–199. doi:10.1016/J.SOILBIO.2015.01.025
Kuzyakov, Y., and Razavi, B. S. (2019). Rhizosphere Size and Shape: Temporal Dynamics and Spatial Stationarity. Soil Biol. Biochem. 135, 343–360. doi:10.1016/j.soilbio.2019.05.011
Lawler, D. F. (1986). Removing Particles in Water and Wastewater. Environ. Sci. Technol. 20, 856–861. doi:10.1021/ES00151A002
Ledder, R. G., Timperley, A. S., Friswell, M. K., Macfarlane, S., and McBain, A. J. (2008). Coaggregation between and Among Human Intestinal and Oral Bacteria. FEMS Microbiol. Ecol. 66, 630–636. doi:10.1111/J.1574-6941.2008.00525.X
Lee, Y.-J., and Lei, Z. (2019). Microalgal-bacterial Aggregates for Wastewater Treatment: a Mini-Review. Bioresour. Techn. Rep. 8, 100199. doi:10.1016/j.biteb.2019.100199
Lemonnier, M., Levin, B. R., Romeo, T., Garner, K., Baquero, M.-R., Mercante, J., et al. (2008). The Evolution of Contact-dependent Inhibition in Non-growing Populations of Escherichia Coli. Proc. R. Soc. B. 275, 3–10. doi:10.1098/RSPB.2007.1234
Liang, C., Wei, D., Zhang, S., Ren, Q., Shi, J., and Liu, L. (2021). Removal of Antibiotic Resistance Genes from Swine Wastewater by Membrane Filtration Treatment. Ecotoxicology Environ. Saf. 210, 111885. doi:10.1016/J.ECOENV.2020.111885
Limoli, D. H., Jones, C. J., and Wozniak, D. J. (2015). Bacterial Extracellular Polysaccharides in Biofilm Formation and Function. Microbiol. Spectr. 3 (3), 1–29. doi:10.1128/microbiolspec.MB-0011-2014
Linares, J. F., Gustafsson, I., Baquero, F., and Martinez, J. L. (2006). Antibiotics as Intermicrobial Signaling Agents Instead of Weapons. Proc. Natl. Acad. Sci. U.S.A. 103, 19484–19489. doi:10.1073/PNAS.0608949103
Li, S., Peng, X., Qi, B., Wang, W., Wang, Q., and Li, Y. (2018). Diversity of Bacteria in Plasmodia of Myxomycetes Estimated by 16S rDNA Sequence Analysis. J. Jilin Agric. Univ. 40, 185–189.
Liu, W., Røder, H. L., Madsen, J. S., Bjarnsholt, T., Sørensen, S. J., and Burmølle, M. (2016). Interspecific Bacterial Interactions Are Reflected in Multispecies Biofilm Spatial Organization. Front. Microbiol. 7, 1366. doi:10.3389/FMICB.2016.01366/BIBTEX
London, J., and Kolenbrander, P. (1996). “Coaggregation,” in Bacterial Adhesion : Molecular and Ecological Diversity. Editor M. Fletcher (Hoboken, NJ: Wiley Series in Ecological and Applied Microbiology, Wiley-Liss), 19, 249.
Lunsdorf, H., Erb, R. W., Abraham, W. R., and Timmis, K. N. (2000). 'Clay Hutches': a Novel Interaction between Bacteria and clay Minerals. Environ. Microbiol. 2, 161–168. doi:10.1046/J.1462-2920.2000.00086.X
Madsen, J. S., Røder, H. L., Russel, J., Sørensen, H., Burmølle, M., and Sørensen, S. J. (2016). Coexistence Facilitates Interspecific Biofilm Formation in Complex Microbial Communities. Environ. Microbiol. 18, 2565–2574. doi:10.1111/1462-2920.13335
Malik, A., Sakamoto, M., Hanazaki, S., Osawa, M., Suzuki, T., Tochigi, M., et al. (2003). Coaggregation Among Nonflocculating Bacteria Isolated from Activated Sludge. Appl. Environ. Microbiol. 69, 6056–6063. doi:10.1128/AEM.69.10.6056-6063.2003
Marshall, D. C., Arruda, B. E., and Silby, M. W. (2019). Alginate Genes Are Required for Optimal Soil Colonization and Persistence by Pseudomonas fluorescens Pf0-1. Access Microbiol. 1, e000021. doi:10.1099/ACMI.0.000021
Martínez, J. L. (2008). Antibiotics and Antibiotic Resistance Genes in Natural Environments. Science 321, 365–367. doi:10.1126/SCIENCE.1159483
Mast, Y., and Stegmann, E. (2019). Actinomycetes: The Antibiotics Producers. Antibiotics 8, 105. doi:10.3390/ANTIBIOTICS8030105
McDaniel, L. D., Young, E., Delaney, J., Ruhnau, F., Ritchie, K. B., and Paul, J. H. (2010). High Frequency of Horizontal Gene Transfer in the Oceans. Science 330, 50. doi:10.1126/SCIENCE.1192243
McShea, D. W., and Brandon, R. N. (2010). Biology’s First Law: The Tendency for Diversity and Complexity to Increase in Evolutionary Systems. (Chicago, IL: University of Chicago Press) , 170.
Mestre, M., Ruiz-González, C., Logares, R., Duarte, C. M., Gasol, J. M., and Sala, M. M. (2018). Sinking Particles Promote Vertical Connectivity in the Ocean Microbiome. Proc. Natl. Acad. Sci. U S A. 115, E6799–E6807. doi:10.1073/PNAS.1802470115/-/DCSUPPLEMENTAL
Mueller, B. (2015). Experimental Interactions between Clay Minerals and Bacteria: A Review. Pedosphere 25, 799–810. doi:10.1016/S1002-0160(15)30061-8
Myers, S. S. (2017). Planetary Health: Protecting Human Health on a Rapidly Changing Planet. The Lancet 390, 2860–2868. doi:10.1016/S0140-6736(17)32846-5
Nguyen, B.-A. T., Chen, Q.-L., He, J.-Z., and Hu, H.-W. (2020). Microbial Regulation of Natural Antibiotic Resistance: Understanding the Protist-Bacteria Interactions for Evolution of Soil Resistome. Sci. Total Environ. 705, 135882. doi:10.1016/J.SCITOTENV.2019.135882
Nwoko, E.-s. Q. A., and Okeke, I. N. (2021). Bacteria Autoaggregation: How and Why Bacteria Stick Together. Biochem. Soc. Trans. 49, 1147–1157. doi:10.1042/BST20200718
Okasha, S. (2006). Evolution and the Levels of Selection. Oxford, United Kingdom: Oxford University Press.
Oki, S., Iwashita, K., Kimura, M., Kano, H., and Shiraki, K. (2018). Mechanism of Co-aggregation in a Protein Mixture with Small Additives. Int. J. Biol. Macromolecules 107, 1428–1437. doi:10.1016/J.IJBIOMAC.2017.10.004
Oliver, A., Cantón, R., Campo, P., Baquero, F., and Blázquez, J. (2000). High Frequency of Hypermutable Pseudomonas aeruginosa in Cystic Fibrosis Lung Infection. Science 288, 1251–1253. doi:10.1126/SCIENCE.288.5469.1251
Pärnänen, K. M. M., Narciso-Da-Rocha, C., Kneis, D., Berendonk, T. U., Cacace, D., Do, T. T., et al. (2019). Antibiotic Resistance in European Wastewater Treatment Plants Mirrors the Pattern of Clinical Antibiotic Resistance Prevalence. Sci. Adv. 5, eaau9124. doi:10.1126/SCIADV.AAU9124
Parvathi, A., Jasna, V., Aswathy, V. K., Nathan, V. K., Aparna, S., and Balachandran, K. K. (2019). Microbial Diversity in a Coastal Environment with Co-existing Upwelling and Mud-banks along the South West Coast of India. Mol. Biol. Rep. 46, 3113–3127. doi:10.1007/S11033-019-04766-Y
Pedrós-Alió, C., Gasol i Piqué, J. M., and Simó i Martorell, R. (2021). Oceans. The Impact of Global Change on the Sea. Mètode 11, 162–217. doi:10.7203/METODE.11.19797
Pérez-Valdespino, A., Pircher, R., Pérez-Domínguez, C. Y., and Mendoza-Sanchez, I. (2021). Impact of Flooding on Urban Soils: Changes in Antibiotic Resistance and Bacterial Community after Hurricane Harvey. Sci. Total Environ. 766, 142643. doi:10.1016/J.SCITOTENV.2020.142643
Perkins, T. L., Perrow, K., Rajko-Nenow, P., Jago, C. F., Jones, D. L., Malham, S. K., et al. (2016). Decay Rates of Faecal Indicator Bacteria from Sewage and Ovine Faeces in Brackish and Freshwater Microcosms with Contrasting Suspended Particulate Matter Concentrations. Sci. Total Environ. 572, 1645–1652. doi:10.1016/J.SCITOTENV.2016.03.076
Picioreanu, C., Kreft, J.-U., and Van Loosdrecht, M. C. M. (2004). Particle-based Multidimensional Multispecies Biofilm Model. Appl. Environ. Microbiol. 70, 3024–3040. doi:10.1128/aem.70.5.3024-3040.2004
Pohl, S., Klockgether, J., Eckweiler, D., Khaledi, A., Schniederjans, M., Chouvarine, P., et al. (2014). The Extensive Set of Accessory Pseudomonas aeruginosa genomic Components. FEMS Microbiol. Lett. 356, 235–241. doi:10.1111/1574-6968.12445
Poirier, I., Benhaïm, D., Poizot, E., Gallon, R. K., Cauvin, E., Lemarchand, A., et al. (2020). Marine Aggregates in North Atlantic Coast: Microbial Characteristics and Potential Interactions with Farmed Atlantic salmon (Salmo salar). Mar. Environ. Res. 157, 104864. doi:10.1016/J.MARENVRES.2019.104864
Pukatzki, S., Kessin, R. H., and Mekalanos, J. J. (2002). The Human Pathogen Pseudomonas aeruginosa Utilizes Conserved Virulence Pathways to Infect the Social Amoeba Dictyostelium discoideum. Proc. Natl. Acad. Sci. U.S.A. 99, 3159–3164. doi:10.1073/PNAS.052704399
Quijano, G., Arcila, J. S., and Buitrón, G. (2017). Microalgal-bacterial Aggregates: Applications and Perspectives for Wastewater Treatment. Biotechnol. Adv. 35 (6), 772–781. doi:10.1016/j.biotechadv.2017.07.003
Rahlff, J., Stolle, C., Giebel, H.-A., Mustaffa, N. I. H., Wurl, O., and P. R. Herlemann, D. (2021). Sea Foams Are Ephemeral Hotspots for Distinctive Bacterial Communities Contrasting Sea-Surface Microlayer and Underlying Surface Water. FEMS Microbiol. Ecol. 97, 35. doi:10.1093/FEMSEC/FIAB035
Rainey, P. B., and Travisano, M. (1998). Adaptive Radiation in a Heterogeneous Environment. Nature 394, 69–72. doi:10.1038/27900
Ramanan, R., Kim, B.-H., Cho, D.-H., Oh, H.-M., and Kim, H.-S. (2016). Algae-bacteria Interactions: Evolution, Ecology and Emerging Applications. Biotechnol. Adv. 34, 14–29. doi:10.1016/J.BIOTECHADV.2015.12.003
Rath, J., Wu, K., Herndl, G., and DeLong, E. (1998). High Phylogenetic Diversity in a marine-snow-associated Bacterial Assemblage. Aquat. Microb. Ecol. 14, 261–269. doi:10.3354/AME014261
Rédou, V., Navarri, M., Meslet-Cladière, L., Barbier, G., and Burgaud, G. (2015). Species Richness and Adaptation of Marine Fungi from Deep-Subseafloor Sediments. Appl. Environ. Microbiol. 81, 3571–3583. doi:10.1128/AEM.04064-14
Reisser, J., Shaw, J., Hallegraeff, G., Proietti, M., Barnes, D. K. A., Thums, M., et al. (2014). Millimeter-Sized Marine Plastics: A New Pelagic Habitat for Microorganisms and Invertebrates. PLOS ONE 9, e100289. doi:10.1371/JOURNAL.PONE.0100289
Rich, P. H., and Wetzel, R. G. (1978). Detritus in the lake Ecosystem. The Am. Naturalist 112, 57–71. doi:10.2307/2460137
Rickard, A. H., Gilbert, P., High, N. J., Kolenbrander, P. E., and Handley, P. S. (2003). Bacterial Coaggregation: An Integral Process in the Development of Multi-Species Biofilms. Trends Microbiol. 11, 94–100. doi:10.1016/S0966-842X(02)00034-3
Rickard, A. H., Leach, S. A., Hall, L. S., Buswell, C. M., High, N. J., and Handley, P. S. (2002). Phylogenetic Relationships and Coaggregation Ability of Freshwater Biofilm Bacteria. Appl. Environ. Microbiol. 68, 3644–3650. doi:10.1128/AEM.68.7.3644-3650.2002
Rocca, J. D., Simonin, M., Blaszczak, J. R., Ernakovich, J. G., Gibbons, S. M., Midani, F. S., et al. (2019). The Microbiome Stress Project: Toward a Global Meta-Analysis of Environmental Stressors and Their Effects on Microbial Communities. Front. Microbiol. 9, 3272. doi:10.3389/FMICB.2018.03272/BIBTEX
Røder, H. L., Olsen, N. M., Whiteley, M., and Burmølle, M. (2020). Unravelling Interspecies Interactions across Heterogeneities in Complex Biofilm Communities. Environ. Microbiol. 22 (1), 5–16. doi:10.1111/1462-2920.14834
Rodriguez-Mozaz, S., Vaz-Moreira, I., Varela Della Giustina, S., Llorca, M., Barceló, D., Schubert, S., et al. (2020). Antibiotic Residues in Final Effluents of European Wastewater Treatment Plants and Their Impact on the Aquatic Environment. Environ. Int. 140, 105733. doi:10.1016/J.ENVINT.2020.105733
Sá, C., Cardoso, P., and Figueira, E. (2019). Alginate as a Feature of Osmotolerance Differentiation Among Soil Bacteria Isolated from Wild Legumes Growing in Portugal. Sci. Total Environ. 681, 312–319. doi:10.1016/J.SCITOTENV.2019.05.050
Sadiq, F. A., Burmølle, M., Heyndrickx, M., Flint, S., Lu, W., Chen, W., et al. (2021). Community-wide Changes Reflecting Bacterial Interspecific Interactions in Multispecies Biofilms. Crit. Rev. Microbiol. 47, 338–358. doi:10.1080/1040841X.2021.1887079
Santos-Lopez, A., Marshall, C. W., Scribner, M. R., Snyder, D. J., and Cooper, V. S. (2019). Evolutionary Pathways to Antibiotic Resistance Are Dependent upon Environmental Structure and Bacterial Lifestyle. eLife 8, e47612. doi:10.7554/ELIFE.47612
Sanz-Sáez, I., Salazar, G., Sánchez, P., Lara, E., Royo-Llonch, M., Sà, E. L., et al. (2020). Diversity and Distribution of marine Heterotrophic Bacteria from a Large Culture Collection. BMC Microbiol. 20, 1–16. doi:10.1186/S12866-020-01884-7/TABLES/3
Sathicq, M. B., Sabatino, R., Corno, G., and di Cesare, A. (2021). Are Microplastic Particles a Hotspot for the Spread and the Persistence of Antibiotic Resistance in Aquatic Systems? Environ. Pollut. 279, 116896. doi:10.1016/J.ENVPOL.2021.116896
Scherlach, K., Graupner, K., and Hertweck, C. (2013). Molecular Bacteria-Fungi Interactions: Effects on Environment, Food, and Medicine. Annu. Rev. Microbiol. 67, 375–397. doi:10.1146/ANNUREV-MICRO-092412-155702
Selvarajan, R., Sibanda, T., Venkatachalam, S., Ogola, H. J. O., Christopher Obieze, C., and Msagati, T. A. (2019). Distribution, Interaction and Functional Profiles of Epiphytic Bacterial Communities from the Rocky Intertidal Seaweeds, South Africa. Sci. Rep. 9, 1–13. doi:10.1038/s41598-019-56269-2
Seymour, J. R., Amin, S. A., Raina, J.-B., and Stocker, R. (2017). Zooming in on the Phycosphere: the Ecological Interface for Phytoplankton-Bacteria Relationships. Nat. Microbiol. 2, 1–12. doi:10.1038/nmicrobiol.2017.65
Simon, M., Grossart, H., Schweitzer, B., and Ploug, H. (2002). Microbial Ecology of Organic Aggregates in Aquatic Ecosystems. Aquat. Microb. Ecol. 28, 175–211. doi:10.3354/AME028175
Simões, L. C., Simões, M., and Vieira, M. J. (2008). Intergeneric Coaggregation Among Drinking Water Bacteria: Evidence of a Role for Acinetobacter Calcoaceticus as a Bridging Bacterium. Appl. Environ. Microbiol. 74, 1259–1263. doi:10.1128/AEM.01747-07
Suhadolnik, M. L. S., Costa, P. S., Paiva, M. C., Salim, A. C. d. M., Barbosa, F. A. R., Lobo, F. P., et al. (2022). Spatiotemporal Dynamics of the Resistome and Virulome of Riverine Microbiomes Disturbed by a Mining Mud Tsunami. Sci. Total Environ. 806, 150936. doi:10.1016/J.SCITOTENV.2021.150936
Tang, X., Yu, L., Yi, Y., Wang, J., Wang, S., Meng, C., et al. (2021). Phylogenomic Analysis Reveals a Two‐stage Process of the Evolutionary Transition of Shewanella from the Upper Ocean to the Hadal Zone. Environ. Microbiol. 23, 744–756. doi:10.1111/1462-2920.15162
Tang, Z. F., and Mcmillen, D. R. (2016). Design Principles for the Analysis and Construction of Robustly Homeostatic Biological Networks. J. Theor. Biol. 408, 274–289. doi:10.1016/j.jtbi.2016.06.036
Troselj, V., Cao, P., and Wall, D. (2018). Cell-cell Recognition and Social Networking in Bacteria. Environ. Microbiol. 20, 923–933. doi:10.1111/1462-2920.14005
Virdis, B., Harnisch, F., Batstone, D. J., Rabaey, K., and Donose, B. C. (2012). Non-invasive Characterization of Electrochemically Active Microbial Biofilms Using Confocal Raman Microscopy. Energy Environ. Sci. 5, 7017–7024. doi:10.1039/c2ee03374g
Vlamakis, H., Chai, Y., Beauregard, P., Losick, R., and Kolter, R. (2013). Sticking Together: Building a Biofilm the Bacillus subtilis Way. Nat. Rev. Microbiol. 11, 157–168. doi:10.1038/NRMICRO2960
Vu, B., Chen, M., Crawford, R., and Ivanova, E. (2009). Bacterial Extracellular Polysaccharides Involved in Biofilm Formation. Molecules 14, 2535–2554. doi:10.3390/MOLECULES14072535
Walker, L., and Shiels, A. (2012). Landslide Ecology. Cambridge, United Kingdom: Cambridge University Press. Available at: https://digitalcommons.unl.edu/icwdm_usdanwrc/1644 (Accessed November 22, 2021).
Wang, G., Sun, S., and Zhang, Z. (2016). Randomness in Sequence Evolution Increases over Time. PLOS ONE 11, e0155935. doi:10.1371/JOURNAL.PONE.0155935
Wang, J., Qin, X., Guo, J., Jia, W., Wang, Q., Zhang, M., et al. (2020). Evidence of Selective Enrichment of Bacterial Assemblages and Antibiotic Resistant Genes by Microplastics in Urban Rivers. Water Res. 183, 116113. doi:10.1016/J.WATRES.2020.116113
Wang, Q., Hao, Z., Ding, R., Li, H., Tang, X., and Chen, F. (2021). Host Dependence of Zooplankton-Associated Microbes and Their Ecological Implications in Freshwater Lakes. Water 13, 2949. doi:10.3390/W13212949
Watts, C. W., Whalley, W. R., Brookes, P. C., Devonshire, B. J., and Whitmore, A. P. (2005). Biological and Physical Processes that Mediate Micro-aggregation of Clays. Soil Sci. 170, 573–583. doi:10.1097/01.ss.0000178206.74040.0c
Weber, V., Kamika, I., and Momba, M. N. B. (2021). Comparing the Effect of Zinc Oxide and Titanium Dioxide Nanoparticles on the Ability of Moderately Halophilic Bacteria to Treat Wastewater. Sci. Rep. 11. doi:10.1038/S41598-021-96413-5
Wei, Z., Xu, T., Shang, S., Tian, H., Cao, Y., Wang, J., et al. (2021). Laboratory Experimental Study on the Formation of Authigenic Carbonates Induced by Microbes in Marine Sediments. Jmse 9, 479. doi:10.3390/JMSE9050479
Wilpiszeski, R. L., Aufrecht, J. A., Retterer, S. T., Sullivan, M. B., Graham, D. E., Pierce, E. M., et al. (2019). Soil Aggregate Microbial Communities: Towards Understanding Microbiome Interactions at Biologically Relevant Scales. Appl. Environ. Microbiol. 85, e00324–19. doi:10.1128/AEM.00324-19/ASSET/344BEADD-2957-421A-AC49-D1B9EF8427A0/ASSETS/GRAPHIC/AEM.00324-19-F0003.JPEG
Wimpenny, J., Manz, W., and Szewzyk, U. (2000). Heterogeneity in Biofilms: Table 1. FEMS Microbiol. Rev. 24, 661–671. doi:10.1111/j.1574-6976.2000.tb00565.x
Witt, V., Ayris, P. M., Damby, D. E., Cimarelli, C., Kueppers, U., Dingwell, D. B., et al. (2017). Volcanic Ash Supports a Diverse Bacterial Community in a marine Mesocosm. Geobiology 15, 453–463. doi:10.1111/GBI.12231
Woegerbauer, M., Bellanger, X., and Merlin, C. (2020). Cell-Free DNA: An Underestimated Source of Antibiotic Resistance Gene Dissemination at the Interface between Human Activities and Downstream Environments in the Context of Wastewater Reuse. Front. Microbiol. 11, 671. doi:10.3389/FMICB.2020.00671/BIBTEX
Wotton, R. S. (2007). Do benthic Biologists Pay Enough Attention to Aggregates Formed in the Water Column of Streams and Rivers? J. North Am. Benthological Soc. 26, 1–11. doi:10.1899/0887-3593(2007)26[1:dbbpea]2.0.co;2
Wright, E. S., Gupta, R., and Vetsigian, K. H. (2021). Multi-stable Bacterial Communities Exhibit Extreme Sensitivity to Initial Conditions. FEMS Microbiol. 97, fiab073. doi:10.1093/FEMSEC/FIAB073
Xie, Q., Lu, Y., Tang, L., Zeng, G., Yang, Z., Fan, C., et al. (2021). The Mechanism and Application of Bidirectional Extracellular Electron Transport in the Field of Energy and Environment. Crit. Rev. Environ. Sci. Techn. 51, 1924–1969. doi:10.1080/10643389.2020.1773728
Yang, H., Liu, R., Liu, H., Wang, C., Yin, X., Zhang, M., et al. (2021). Evidence for Long-Term Anthropogenic Pollution: The Hadal Trench as a Depository and Indicator for Dissemination of Antibiotic Resistance Genes. Environ. Sci. Technol. 55, 15136–15148. doi:10.1021/ACS.EST.1C03444
Yang, L., Liu, Y., Wu, H., Høiby, N., Molin, S., and Song, Z. J. (2011). Current Understanding of Multi‐species Biofilms. Int. J. Oral Sci. 3, 74–81. doi:10.4248/IJOS11027
You, Z., Pearce, D. J. G., Sengupta, A., and Giomi, L. (2019). Mono- to Multilayer Transition in Growing Bacterial Colonies. Phys. Rev. Lett. 123, 178001. doi:10.1103/PHYSREVLETT.123.178001/FIGURES/4/MEDIUM
Zadorin, A. S., Rondelez, Y., and Yannick, R. (2019). Natural Selection in Compartmentalized Environment with Reshuffling. J. Math. Biol. 79, 1401–1454. doi:10.1007/s00285-019-01399-4ï
Zee, P. C., and Bever, J. D. (2014). Joint Evolution of Kin Recognition and Cooperation in Spatially Structured Rhizobium Populations. PLOS ONE 9, e95141. doi:10.1371/JOURNAL.PONE.0095141
Zhang, W., Gu, P., Wang, N., Zhu, W., Jiang, M., He, J., et al. (2019). Effects of Cyanobacterial Blooms on Microbial Community Distribution in Surface Sediments in Lakes. J. Bioremediat. Biodegrad. 10, 461.
Zhao, B., Xing, P., and Wu, Q. L. (2021). Interactions between Bacteria and Fungi in Macrophyte Leaf Litter Decomposition. Environ. Microbiol. 23, 1130–1144. doi:10.1111/1462-2920.15261
Keywords: microbiotic particles, antimicrobial resistance, soil particles, particles coalescence, water-bodies particles
Citation: Baquero F, Coque T, Guerra-Pinto N, Galán J, Jiménez-Lalana D, Tamames J and Pedrós-Alió C (2022) The Influence of Coalescent Microbiotic Particles From Water and Soil on the Evolution and Spread of Antimicrobial Resistance. Front. Environ. Sci. 10:824963. doi: 10.3389/fenvs.2022.824963
Received: 29 November 2021; Accepted: 23 March 2022;
Published: 21 April 2022.
Edited by:
Luther King Abia Akebe, University of KwaZulu-Natal, South AfricaReviewed by:
Arnaud Dechesne, Technical University of Denmark, DenmarkYunpeng Gai, Zhejiang University, China
Mohd Sayeed Akhtar, M. J. P. Rohilkhand University, India
Eustace Fernando, Rajarata University of Sri Lanka, Sri Lanka
Copyright © 2022 Baquero, Coque, Guerra-Pinto, Galán, Jiménez-Lalana, Tamames and Pedrós-Alió. This is an open-access article distributed under the terms of the Creative Commons Attribution License (CC BY). The use, distribution or reproduction in other forums is permitted, provided the original author(s) and the copyright owner(s) are credited and that the original publication in this journal is cited, in accordance with accepted academic practice. No use, distribution or reproduction is permitted which does not comply with these terms.
*Correspondence: F. Baquero, bWFpbHRvOmJhcXVlcm9AYml0bWFpbGVyLm5ldA==