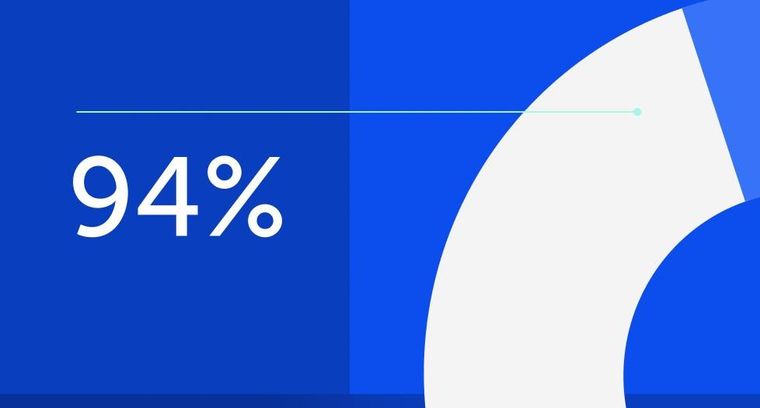
94% of researchers rate our articles as excellent or good
Learn more about the work of our research integrity team to safeguard the quality of each article we publish.
Find out more
POLICY AND PRACTICE REVIEWS article
Front. Environ. Sci., 09 March 2022
Sec. Soil Processes
Volume 10 - 2022 | https://doi.org/10.3389/fenvs.2022.821742
This article is part of the Research TopicEurosoil 2021: Sustainable Management of Soil Functions as a Basis to Avoid, Halt, and Reverse Land DegradationView all 14 articles
Soil health-based agricultural management practices are widely promoted to reduce erosion, increase nutrient use efficiency, improve soil structure, and sustain or increase yields. Pest and disease management are less frequently considered as components of a soil health management system. We present a framework for how the crop protection industry can advance soil health by developing systems of crop protection innovation that simultaneously target soil health outcomes, either through direct impact on soil or by enabling practices that promote soil health outcomes. Such an approach could lead to cross-sectoral, integrated agricultural solutions that achieve agronomic, environmental, and economic goals.
The concept of soil health has united farmers, researchers, government agencies, non-profits, and the private sector around the possibility that management of agroecosystems can meaningfully contribute to solving major environmental challenges. Soil health is a multi-dimensional concept that refers to the ability of soil to serve as an ecosystem that sustains plants and animals while supporting human uses such as agriculture and forestry (Lehmann et al., 2020). Decades of evidence has illustrated the agronomic and environmental benefits of agricultural practices such as cover cropping, reduced tillage, and diversified crop rotations (Atwood and Wood, 2021). These soil health practices align with the principles of conservation agriculture: maintain living plant cover, reduce disturbance, and diversify crop rotations. While the benefits of these practices on soil-derived ecosystem services can vary (Palm et al., 2014), there is considerable opportunity to increase the adoption, and thus the benefits, of agronomic practices that reduce erosion and nutrient loss, rebuild soil carbon, and sustain agronomic production.
Pest management is an important component of agriculture, with most farmers relying on multiple pest management practices, including insecticides, fungicides, and herbicides. Many of the available chemical, biological, and genetic crop protection solutions maximize short-term benefits by avoiding pest damage within a season without targeting long-term soil health outcomes creating unintentional trade-offs and highlighting the need for innovation. This includes the drastic rise in biological resistance to chemical crop protection products that results from poor product stewardship and costs farmers billions of U.S. dollars per year (Mortensen et al., 2012; Perotti et al., 2020). Still, the relationship between crop protection innovation and soil health is rarely discussed. An exception to this is weed management, for two reasons: reverting to tillage to manage herbicide resistant weeds would directly reduce soil health (Nunes et al., 2020; Van Deynze et al., 2021) and practices that promote soil health can positively contribute to weed management, e.g., cover cropping (Osipitan et al., 2018).
Historically soil health and crop protection have taken seemingly incompatible approaches, but the future is still unfolding and there is time for evolution within the industry that improves the complementarity between these management strategies and amplifies their agri-environmental benefits. On one hand, the crop protection industry has traditionally taken a more reactive approach to pest threats where management occurs after infestation. Soil health, alternatively, has taken a more preventative approach with a goal of avoiding pest establishment and reducing the need for curative intervention. It is conceivable to envision a future where ecological interactions in the soil are protected and fostered by crop protection innovations, improving soil health and preventing pests from economically damaging crops. This evolution of crop protection is also being supported by remarkable advances in complementary disciplines (e.g., digital technology). Novel imaging-based diagnostic tools, new equipment for precision application of agricultural inputs, and improved predictive algorithms for abiotic and biotic stresses all allow the offer of more targeted crop protection programs (Abit et al., 2018). The transition to this future would also align with and contribute to society’s broader vision for sustainability across sectors, which is outlined in international policies and initiatives like the European Green Deal (European Union, 2019) and the United Nations’ 2030 Agenda for Sustainable Development (United Nations, 2015). Through the establishment of the Coalition of Action 4 Soil Health (CA4SH), the crop protection industry and other relevant stakeholders have demonstrated their intention to act collaboratively to remove barriers to sustainable agricultural systems that promote soil health.
Moving to a system of crop protection that promotes soil health is an underexplored pathway to achieve long-term, agricultural sustainability and productivity. This will require methods of pest management that are compatible with specific soil health practices and also do not degrade the functional capacity of soil communities. To this end, we present three key research and development priorities the crop protection community should pursue simultaneously: 1) innovate products and application methods that avoid or reduce impacts on soil health; 2) innovate products that, alone or in combination with plant genetics, leverage soil functions and communities to enhance pest and disease management and/or biogeochemical nutrient cycling and enable reduced input use; and 3) innovate products that enable management practices that benefit soil health, while minimizing tradeoffs. Achieving these three opportunities requires a fourth innovation: 4) develop new soil health screening and field trial procedures along the crop protection research and development (R&D) stage-gate process (Figure 1). All four of these innovation opportunities are necessary to capitalize on the potential of soil health to deliver long-term agronomic and environmental benefits. We recognize that the economic impacts and feasibility of new technologies developed under this framework will undoubtedly influence adoption, but due to their novelty, the necessary data for economic analyses do not currently exist and remain out of scope of the current discussion.
FIGURE 1. Four key opportunities for the crop protection industry to innovate new technologies around soil health.
The functioning of soil biological communities is a key feature of soil health (Lehmann et al., 2020). Soil-based agroecosystem services important for crop health, like pest management and nutrient cycling, depend on biological interaction (Delgado-Baquerizo et al., 2020). Although the relationship between soil biodiversity and agroecosystem services is both complex and context-dependent (Bradford et al., 2014; Wagg et al., 2014; Gamfeldt and Roger, 2017; Fanin et al., 2018; Delgado-Baquerizo et al., 2020), supporting and protecting soil organisms generally enhances their contributions to agroecosystem services (Tooker et al., 2020).
Microbial and invertebrate biomass and activity, and often biodiversity, tend to increase with soil health practices compared to conventional management practices (Tsiafouli et al., 2015; Atwood and Wood, 2021; Carlos et al., 2021). As the soil environment becomes more competitive, rates of crop infection commonly decrease because pathogenic microbes must increasingly compete for resources and overcome interactions with antagonistic and interfering non-pathogenic microbes (Abawi and Widmer, 2000; de Faria et al., 2021). Additionally, biological pest control generally increases with improved soil health due to appreciable predator-prey interactions among invertebrates (Neher and Barbercheck, 2019; Alyokhin et al., 2020). This increase in biological control can result in direct economic benefits for farmers. In Sweden, for example, it is estimated that soil dwelling predators contribute €41 ha−1 yr−1 by controlling a single pest and increasing spring barley yields 303 kg ha−1 (Östman et al., 2003). There are, however, potential tradeoffs associated with increased soil biodiversity including, but not limited to, increased predation of alternate prey opposed to target pests due to predators’ preferences for alternate prey (Lynch et al., 2022). Such potential tradeoffs are generally outweighed by the agroecosystem service benefits associated with supporting and protecting soil organisms (Tooker et al., 2020).
In many agricultural systems, soil-derived crop protection services are masked, limited, and/or disrupted by management practices, resulting in an increased reliance on pesticides. Simplified crop rotations, frequent or extensive tillage, and the inadequate or inappropriate use of fertilizers and manure have all been correlated with increased incidence or severity of soil soil-borne pathogens and pests (Peters et al., 2003), despite that mechanisms of soil suppressiveness appear to be well conserved across a wide range of soil-pathogen combinations (van Agtmaal et al., 2018). The relationship between soil management and suppressiveness is explained by the fact that the latter is a process that is mediated by soil communities (Weller et al., 2002; Campos et al., 2016) - any practice that directly or indirectly alters soil community composition, diversity, or activity could have an effect on soils’ natural ability to regulate pest populations. Conventional tillage or plowing practices and the removal of crop residues have both been demonstrated to reduce the quantity and quality of soil organic matter and thereby reduce soil suppressiveness to multiple types of crop pests and pathogens (Kremer and Li, 2003; Fang et al., 2012; Bongiorno et al., 2019; Palojärvi et al., 2020). The effects of other practices that are commonly described as beneficial for soil health, such as diversified crop rotations (Congreves et al., 2015), are not as clear. A comprehensive review by Rusch et al. (2010) posited that crop monocultures create environments that cannot support soil-mediated crop protection services due to inadequate resource availability. Still, meta-analysis reveals this may not hold true in up to 48% of scenarios (Rusch et al., 2010), as is the case in the development of soils highly suppressive to Fusarium wilt following the long-term, continuous monoculture of strawberry (Cha et al., 2016). A thorough understanding of the mechanisms of soil-mediated crop protection and their interaction with conservation management strategies is therefore critical to realizing crop protection systems that deliver opportunities (1) and (2) of the proposed framework (Figure 1). Furthermore, there is potential to innovate chemical products or plant genetics (for use alone or in combination with improved soil management) that regenerate soil-derived crop protection services by cultivating and protecting soil community function. Innovations could promote or enhance suppression of invertebrate and weed pests via predator-prey interactions (Sanchez-Morena and Ferris, 2007), suppression of soil-borne pathogens via soil microorganisms (Cha et al., 2016), fortification of plant health with nutrient cycling (Campos et al., 2016; Neher and Barbercheck, 2019), and attraction of predators to pest outbreaks with plant volatiles and exudates (Turlings and Erb, 2018).
The use of plant-derived volatile organic compounds is one example of how the role of chemical signaling in plant and invertebrate interactions could be leveraged for crop protection innovation. Evidence has established that plants have the ability to influence soil communities in beneficial ways (Hiltpold and Turlings, 2012). For instance, herbivory by the cabbage root fly (Delia radicum) elicits the release of a volatile compound, dimethyl disulfide, from cabbage roots (Danner et al., 2012), simultaneously inhibiting the fly’s reproductive behavior and attracting its natural predators (Ferry et al., 2009). Similarly, more than 74 different root exudates have been described as driving factors of host plant location in belowground insect herbivores (Johnson and Nielsen, 2012), either recruiting beneficial species to the rhizosphere (Williams and Vries, 2020) or repelling harmful ones (Xu et al., 2015). Identification of individual compounds contributing to dynamic plant-pest interactions could enable the development and optimization of natural or close semiochemical derivatives for use in novel push-pull systems of pest control (Werle et al., 2019). By definition, push-pull systems capitalize on the abilities of plants to manipulate pests, integrating stimuli that make the crop unattractive to the pest (push) with those that make non-cropped areas attractive (pull) (Cook et al., 2007). Recent research examining the chemical basis of nematode herbivory in Capsicum spp (Kihika et al., 2017) illustrates the potential of using this strategy for belowground pests, though there are many more examples of modulating behavior of pests aboveground through push-pull systems (Cook et al., 2007; Xu et al., 2018; Rivera et al., 2020). Beyond “push-pull” systems there are numerous opportunities to innovate novel methods of pest control–that regulate soil communities using root exudate chemistries (Chaparro et al., 2012), plant-microbe or other symbioses, (Mercado-Blanco and Bakker, 2007; Borghi et al., 2021), mediation of tri-trophic interactions, (Helmberger et al., 2017), and improved cycling of crop limiting nutrients (Moreau et al., 2019)–and reach soil health goals.
While the implications of pesticide driven changes in soil biological communities are still under investigation (Nettles et al., 2016; Storck et al., 2018; Hage-Ahmed et al., 2019) development of new crop protection products should safeguard the continuation of ecosystem services by avoiding or reducing impacts on soil biological communities. The desire to avoid impacts that are not well understood has driven increasing attention within both the policy and R&D communities on technologies that decrease reliance on chemical pest control methods and reduce inputs. This includes strategies to elicit natural plant defenses and immune responses through priming (Worrall et al., 2011), advanced breeding techniques/genetic modification (Bruce, 2012), or plant- or microbe-derived molecules (Wiesel et al., 2014), including plant hormones (De Mesmaeker et al., 2019). One novel approach for eliciting or enhancing plant defenses is through the use of RNA (host-induced sRNA, dsRNA, or RNAi) for targeted gene silencing in fungal pathogens (Niu et al., 2021). RNA-based crop protection technologies can prevent colonization and infection of crop species by fungal pathogens [e.g., of Sclerotinia sclerotiorum, the causal agent of white stem rot in canola (McLoughlin et al., 2018)] and, in some cases, directly reduce the virulence of fungal pathogens [e.g., of Botrytis cinerea, which causes gray mold (Cai et al., 2018)]. However, to determine any technology’s suitability for conserving soil health while simultaneously delivering effective crop protection, the innovation will need to undergo a comprehensive assessment that includes its impacts on soil biological, physical, and chemical properties. Such assessments are therefore a critical first step towards effectively implementing emerging policies that aim to protect and reverse degradation of soil resources for agriculture, nature, and climate, for example, those that comprise the European Union Soil Strategy for 2030. (European Union, 2021).
Structuring new innovations and application methods into an integrated pest management (IPM) approach (i.e., a coordinated management strategy that utilizes a diverse set of prevention, cultural, biological, mechanical, and chemical tactics based on cost-benefit analyses (Kogan, 1998)) will not only help to conserve soil health, but also reduce the incidence of chemical resistance. Indeed, addressing the root causes of the evolution of chemical resistant pest species can be considered a primary principle of IPM (Barzman et al., 2015) and this outcome underpins many existing policy initiatives, such as Directive 2009/128/EC of the European Parliament that outlines the framework for the sustainable use of pesticides within the European Union (European Union, 2009). Even with growing consensus that IPM effectively protects crops, the environment, and soil health and is critical to sustainable agricultural systems (Anderson et al., 2019), it is not yet widely adopted, largely because of perceived risks and intensive knowledge requirements (Parsa et al., 2014; Bakker et al., 2021). By integrating the principles of IPM into the targeting, design and promotion of novel technologies, the crop protection industry can support IPM adoption and ensure the longevity of their innovations.
Regardless of the availability of innovative crop protection products that support soil health, implementation of conservation practices can vary substantially based on location, farm size, and farmer knowledge and perceptions, all of which influence the management approach (Scopel et al., 2013; Hermans et al., 2021). For increased impact on soil health at scale, crop protection innovations should aim to support localized management approaches for farms of all sizes, across a wide variety of cropping systems and environmental contexts. Innovating for a wider range of scenarios would enable the adoption of more diverse or complex cropping systems, while expanding the potential market for new solutions. Encouraging crop diversification benefits the crop protection industry directly because diversified rotations boost biological control and decrease the probability of developing resistant populations (Harker et al., 2016; Isbell et al., 2017), thus protecting the longevity of new products. Technological innovations, including useful decision support tools, are also needed within this context to alleviate the knowledge burden that accompanies management of complex systems. With interest in diversifying rotations growing (Runck et al., 2014), moving beyond monocultures of blockbuster crops towards lesser-researched crops will support the next frontier of sustainable agriculture.
Another opportunity for innovation is to focus on crop protection challenges that consistently occur when soil health practices are implemented. For example, surface residue retention protects soil organisms, maintains soil moisture (Turmel et al., 2015), and suppresses weeds (Mobli and Chauhan, 2020), but can also exacerbate waterlogging and result in lower yields in areas experiencing high rainfall (Rusinamhodzi et al., 2011). If it rains just before or during planting, lowering soil temperatures, seeds may remain quiescent in the soil and be more vulnerable to pathogens and invertebrates (Gamfeldt and Roger, 2017). Prophylactic seed treatments are currently the primary practice for managing this challenge, but potential off-target soil health impacts associated with some insecticide-fungicide seed treatments demonstrate the need for soil health compatible alternatives (Douglas and Tooker, 2015). Relevant innovations could include the development of products that can be used for seed priming or enhance seed or seedling defenses, competitive abilities, or vigor in suboptimal conditions. The types of vulnerabilities that accompany conservation agriculture are prime foci for innovative crop protection products. They also offer an opportunity for innovation in other sectors, such as breeding cover crops that are tailored to expected climate change and creating farm equipment that enables practices like early seeding of cover crops alongside cash crops.
The integration of additional soil health measures into the crop protection R&D pipeline is critical for determining each potential crop protection product’s compatibility with soil health (Figure 2A). This would have two benefits: first, ensuring that new compounds do not directly contribute to the deterioration of important soil community functions; second, providing criteria for optimizing new compounds that target soil health. The need to define effective and feasible screening methods for soil health impacts within crop protection R&D has become even more urgent in light of updates to the European Union’s Chemicals Strategy for Sustainability. (European Union, 2020). An emerging initiative under this strategy, Safe- and Sustainable-by-Design, is intended to serve as a guiding principle for future regulation of chemicals to catalyze the transition towards chemicals, materials, and products that are designed to be inherently safe and sustainable. In the short period since the introduction of Safe- and Sustainable-by-design, it has already become clear that appropriate criteria and assessment tools will be foundational to its success (Mech et al., 2022).
FIGURE 2. Schematic of (A) major activities supporting innovation opportunities along the crop protection research and development (R&D) and lifecycle management timelines and (B) R&D pipeline with opportunities to screen for soil health impacts highlighted with dashed (---) box. The focus of the soil health evaluations will phase from potential direct effects of compounds on soil functions or soil health parameters in the early stages to indirect effects of compounds on soil health in later stages. Number of compounds evaluated in each phase is aspirational and may not reflect actual number of compounds currently undergoing evaluation.
R&D follows a stage-gate process, in which innovations are subjected to evaluations and risk assessments specific to each stage, presenting the potential to integrate soil health measures at multiple scales–from lab assays, to microcosms, to field trials. However, migrating the indicators and evaluations developed for field trials into the lab, or vice versa, is not straightforward. Many relevant soil properties change on the scale of years or are the result of complex biotic and abiotic interactions that are difficult to replicate in controlled environments. Similarly, high-throughput assays are, by nature, simplifications of soil ecosystems and can be challenging to translate to the field-scale. Thus, new methods, indicators, and approaches need to be innovated to align soil health screening with the existing R&D process (Figure 2B).
In particular, biological indicators will be crucial in testing the compatibility of new products with soil health during the stage-gate process. Biological indicators, such as nitrogen- or phosphorus-mineralizing enzyme activity, comprise the smallest proportion of indicators (20%) used in current soil health assessments despite the important role of soil biology in determining the overall health of a soil. (Lehmann et al., 2020). The lack of uptake of biological indicators is largely due to the difficulty in interpreting them, which limits their utility in selecting and monitoring strategies to improve soil health. (Fierer et al., 2021). This presents opportunities to identify new biological indicators of soil health that are both meaningful and compatible with high-throughput screening. Such innovative measures, whether biological or otherwise, should serve as proxies of longer-term dynamics in soil health (Fierer et al., 2021) if they are to be used successfully in early-stage R&D.
The later stages of product development—where experiments are conducted in greenhouse microcosms and fields—are better suited for ecological evaluations because the experimental system can include multiple interacting components (Figure 2B). The opportunity to innovate and test new measures of soil health tailored to different stages means that there will need to be flexibility built into R&D processes to allow adjustment of procedures based on forthcoming knowledge. This will enable continual improvements in matching the assessments with the desirable function(s) the product intends to support.
Ultimately the acceptance of soil health-oriented crop protection innovations, particularly among practitioners of sustainable agriculture and the environmental community, will depend on the industry’s ability to establish a credible product evaluation process. That is, one with scientifically robust criteria that demonstrate product compatibility with and support of sustainability-focused systems. The implementation of appropriate screening procedures should be complimented by transparent efforts to support voluntary and regulatory efforts that are part of a shift towards more complex agroecosystems that support long-term sustainability. This includes defining processes for collaborating on and sharing learning from the development of criteria and assessment tools with policymakers and other stakeholders to contribute to the refinement of policies like Safe- and Sustainable-by-design.
Increased pesticide resistance has cost millions of dollars in damages, highlighting the need for innovation in the crop protection sector. Efforts to promote soil health and conservation agriculture are largely occurring independent of crop protection innovation. Our framework highlights a way to align the need for crop protection innovation with the broader goals of soil health, revealing potentially fruitful avenues for research and innovation. Achieving this alignment requires new crop protection solutions that benefit soil health directly and indirectly by enabling other soil health practices. Developing these innovations requires new approaches to R&D in the crop protection sector. Greater collaboration between the crop protection sector, the public sector, and civil society can help ensure that agronomic and environmental targets are aligned and achieved together.
Innovative crop protection solutions that deliver agronomic and environmental targets require new approaches to R&D that integrate soil health goals.
Conceptualization: LA, KR, MD, CM, SM, RO, CS, MW, and SW Visualization: LA, KR, and MW Writing – original draft: LA and KR Writing – review and editing: LA, KR, MD, CM, SM, RO, CS, MW and SW.
Authors MD, CM, SM, RO, and CS were employed by Syngenta.
The remaining authors declare that the research was conducted in the absence of any commercial or financial relationships that could be construed as a potential conflict of interest.
All claims expressed in this article are solely those of the authors and do not necessarily represent those of their affiliated organizations or those of the publisher, the editors, and the reviewers. Any product that may be evaluated in this article, or claim that may be made by its manufacturer, is not guaranteed or endorsed by the publisher.
Abawi, G. S., and Widmer, T. L. (2000). Impact of Soil Health Management Practices on Soilborne Pathogens, Nematodes and Root Diseases of Vegetable Crops. Appl. Soil Ecol. 15 (1), 37–47. doi:10.1016/s0929-1393(00)00070-6
Abit, M. J. M., Brian Arnall, D., and Phillips, S. B. (2018). “Environmental Implications of Precision Agriculture,” in Precision Agriculture Basics. Editors D. K. Shannon, D. E. Clay, and N. R. Kitchen (Madison, WI: American Society of Agronomy, Crop Science Society of America, and Soil Science Society of America). doi:10.2134/precisionagbasics.2017.0035
Alyokhin, A., Nault, B., and Brown, B. (2020). Soil Conservation Practices for Insect Pest Management in Highly Disturbed Agroecosystems - a Review. Entomol. Exp. Appl. 168 (1), 7–27. doi:10.1111/eea.12863
Anderson, J. A., Ellsworth, P. C., Faria, J. C., Head, G. P., Owen, M. D. K., Pilcher, C. D., et al. (2019). Genetically Engineered Crops: Importance of Diversified Integrated Pest Management for Agricultural Sustainability. Front. Bioeng. Biotechnol. 7, 24. doi:10.3389/fbioe.2019.00024
Atwood, L. W., and Wood, S. (2021). AgEvidence: Agro-Environmental Responses of Conservation Agricultural Practices in the US Midwest Published from 1980 to 2020. Knowl. Netw. Biocomplexity. doi:10.5063/Z31X15
Bakker, L., Sok, J., Van Der Werf, W., and Bianchi, F. J. J. A. (2021). Kicking the Habit: What Makes and Breaks Farmers' Intentions to Reduce Pesticide Use? Ecol. Econ. 180, 106868. doi:10.1016/j.ecolecon.2020.106868
Barzman, M., Bàrberi, P., Birch, A. N. E., Boonekamp, P., Dachbrodt-Saaydeh, S., Graf, B., et al. (2015). Eight Principles of Integrated Pest Management. Agron. Sustain. Dev. 35, 1199–1215. doi:10.1007/s13593-015-0327-9
Bongiorno, G., Postma, J., Bünemann, E. K., Brussaard, L., de Goede, R. G. M., Mäder, P., et al. (2019). Soil Suppressiveness to Pythium Ultimum in Ten European Long-Term Field Experiments and its Relation with Soil Parameters. Soil Biol. Biochem. 133, 174–187. doi:10.1016/j.soilbio.2019.03.012
Borghi, L., Screpanti, C., Lumbroso, A., Lachia, M., Gubeli, C., and De Mesmaeker, A. (2021). Efficiency and Bioavailability of New Synthetic Strigolactone Mimics with Potential for Sustainable Agronomical Applications. Plant and Soil 465, 109–123. doi:10.1007/s11104-021-04943-8
Bradford, M. A., Wood, S. A., Bardgett, R. D., Black, H. I. J., Bonkowski, M., Eggers, T., et al. (2014). Discontinuity in the Responses of Ecosystem Processes and Multifunctionality to Altered Soil Community Composition. Proc. Natl. Acad. Sci. USA 111 (40), 14478–14483. doi:10.1073/pnas.1413707111
Bruce, T. J. A. (2012). GM as a Route for Delivery of Sustainable Crop protection. J. Exp. Bot. 63 (2), 537–541. doi:10.1093/jxb/err281
Cai, Q., Qiao, L., Wang, M., He, B., Lin, F. M., Palmquist, J., et al. (2018). Plants Send Small RNAs in Extracellular Vesicles to Fungal Pathogen to Silence Virulence Genes. Science 360, 63931126–63931129. doi:10.1126/science.aar4142
Campos, S. B., Lisboa, B. B., Camargo, F. A. O., Bayer, C., Sczyrba, A., Dirksen, P., et al. (2016). Soil Suppressiveness and its Relations with the Microbial Community in a Brazilian Subtropical Agroecosystem under Different Management Systems. Soil Biol. Biochem. 96, 191–197. doi:10.1016/j.soilbio.2016.02.010
Carlos, F. S., Schaffer, N., Marcolin, E., Fernandes, R. S., Mariot, R., Mazzurana, M., et al. (2021). A Long‐term No‐tillage System Can Increase Enzymatic Activity and Maintain Bacterial Richness in Paddy fields. Land Degrad. Dev. 32 (6), 2257–2268. doi:10.1002/ldr.3896
Cha, J.-Y., Han, S., Hong, H.-J., Cho, H., Kim, D., Kwon, Y., et al. (2016). Microbial and Biochemical Basis of a Fusarium Wilt-Suppressive Soil. Isme J. 10, 119–129. doi:10.1038/ismej.2015.95
Chaparro, J. M., Sheflin, A. M., Manter, D. K., and Vivanco, J. M. (2012). Manipulating the Soil Microbiome to Increase Soil Health and Plant Fertility. Biol. Fertil. Soils 48, 489–499. doi:10.1007/s00374-012-0691-4
Congreves, K. A., Hayes, A., Verhallen, E. A., and Van Eerd, L. L. (2015). Long-term Impact of Tillage and Crop Rotation on Soil Health at Four Temperate Agroecosystems. Soil Tillage Res. 152, 17–28. doi:10.1016/j.still.2015.03.012
Cook, S. M., Khan, Z. R., and Pickett, J. A. (2007). The Use of Push-Pull Strategies in Integrated Pest Management. Annu. Rev. Entomol. 52, 375–400. doi:10.1146/annurev.ento.52.110405.091407
Danner, H., Samudrala, D., Cristescu, S. M., and Van Dam, N. M. (2012). Tracing Hidden Herbivores: Time-Resolved Non-invasive Analysis of Belowground Volatiles by Proton-Transfer-Reaction Mass Spectrometry (PTR-MS). J. Chem. Ecol. 38, 785–794. doi:10.1007/s10886-012-0129-3
de Faria, M. R., Costa, L. S. A. S., Chiaramonte, J. B., Bettiol, W., and Mendes, R. (2021). The Rhizosphere Microbiome: Functions, Dynamics, and Role in Plant Protection. Trop. Plant Pathol. 46 (1), 13–25. doi:10.1007/s40858-020-00390-5
De Mesmaeker, A., Screpanti, C., Fonné-Pfister, R., Lachia, M., Lumbroso, A., and Bouwmeester, H. (2019). Design, Synthesis and Biological Evaluation of Strigolactone and Strigolactam Derivatives for Potential Crop Enhancement Applications in Modern Agriculture. Chimia (Aarau) 73 (7-8), 549–560. doi:10.2533/chimia.2019.549
Delgado-Baquerizo, M., Reich, P. B., Trivedi, C., Eldridge, D. J., Abades, S., Alfaro, F. D., et al. (2020). Multiple Elements of Soil Biodiversity Drive Ecosystem Functions across Biomes. Nat. Ecol. Evol. 4, 210–220. doi:10.1038/s41559-019-1084-y
Douglas, M. R., and Tooker, J. F. (2015). Large-Scale Deployment of Seed Treatments Has Driven Rapid Increase in Use of Neonicotinoid Insecticides and Preemptive Pest Management in U.S. Field Crops. Environ. Sci. Technol. 49, 5088–5097. doi:10.1021/es506141g
European Union (2021). Communication from the Commission to the European Parliament, the Council, the European Economic and Social Committee and the Committee of the Regions EU Soil Strategy for 2030 Reaping the Benefits of Healthy Soils for People, Food, Nature and Climate. COM/2021/699 Final. Brussels: European Union.
European Union (2020). Communication from the Commission to the European Parliament, the European Council, the Council, the European Economic and Social Committee and the Committee of the Regions A New Industrial Strategy for Europe. COM/2020/102 Final. Brussels: European Union.
European Union (2019). Communication from the Commission to the European Parliament, the European Council, the Council, the European Economic and Social Committee and the Committee of the Regions the European Green Deal. COM/2019/640 Final. Brussels: European Union.
European Union (2009). Directive 2009/128/EC of the European Parliament and of the Council of 21 October 2009 Establishing a Framework for Community Action to Achieve the Sustainable Use of Pesticides. Off. J. Eur. Union 309, 71.
Fang, X., You, M. P., and Barbetti, M. J. (2012). Reduced Severity and Impact of Fusarium Wilt on Strawberry by Manipulation of Soil pH, Soil Organic Amendments and Crop Rotation. Eur. J. Plant Pathol. 134, 619–629. doi:10.1007/s10658-012-0042-1
Fanin, N., Gundale, M. J., Farrell, M., Ciobanu, M., Baldock, J. A., Nilsson, M.-C., et al. (2018). Consistent Effects of Biodiversity Loss on Multifunctionality across Contrasting Ecosystems. Nat. Ecol. Evol. 2 (2), 269–278. doi:10.1038/s41559-017-0415-0
Ferry, A., Le Tron, S., Dugravot, S., and Cortesero, A. M. (2009). Field Evaluation of the Combined Deterrent and Attractive Effects of Dimethyl Disulfide on Delia Radicum and its Natural Enemies. Biol. Control. 49, 219–226. doi:10.1016/j.biocontrol.2009.01.013
Fierer, N., Wood, S. A., and Bueno de Mesquita, C. P. (2021). How Microbes Can, and Cannot, Be Used to Assess Soil Health. Soil Biol. Biochem. 153, 108111. doi:10.1016/j.soilbio.2020.108111
Gamfeldt, L., and Roger, F. (2017). Revisiting the Biodiversity-Ecosystem Multifunctionality Relationship. Nat. Ecol. Evol. 1 (7), 168. doi:10.1038/s41559-017-0168
Hage-Ahmed, K., Rosner, K., and Steinkellner, S. (2019). Arbuscular Mycorrhizal Fungi and Their Response to Pesticides. Pest Manag. Sci. 75, 583–590. doi:10.1002/ps.5220
Harker, K. N., O'Donovan, J. T., Turkington, T. K., Blackshaw, R. E., Lupwayi, N. Z., Smith, E. G., et al. (2016). Diverse Rotations and Optimal Cultural Practices Control Wild Oat (Avena Fatua). Weed Sci. 64 (1), 170–180. doi:10.1614/ws-d-15-00133.1
Helmberger, M. S., Shields, E. J., and Wickings, K. G. (2017). Ecology of Belowground Biological Control: Entomopathogenic Nematode Interactions with Soil Biota. Appl. Soil Ecol. 121, 201–213. doi:10.1016/j.apsoil.2017.10.013
Hermans, T. D. G., Dougill, A. J., Whitfield, S., Peacock, C. L., Eze, S., and Thierfelder, C. (2021). Combining Local Knowledge and Soil Science for Integrated Soil Health Assessments in Conservation Agriculture Systems. J. Environ. Manage. 286, 112192. doi:10.1016/j.jenvman.2021.112192
Hiltpold, I., and Turlings, T. C. J. (2012). Manipulation of Chemically Mediated Interactions in Agricultural Soils to Enhance the Control of Crop Pests and to Improve Crop Yield. J. Chem. Ecol. 38, 641–650. doi:10.1007/s10886-012-0131-9
Isbell, F., Adler, P. R., Eisenhauer, N., Fornara, D., Kimmel, K., Kremen, C., et al. (2017). Benefits of Increasing Plant Diversity in Sustainable Agroecosystems. J. Ecol. 105, 871–879. doi:10.1111/1365-2745.12789
Johnson, S. N., and Nielsen, U. N. (2012). Foraging in the Dark - Chemically Mediated Host Plant Location by Belowground Insect Herbivores. J. Chem. Ecol. 38, 604–614. doi:10.1007/s10886-012-0106-x
Kihika, R., Murungi, L. K., Coyne, D., Ng’ang’a, M., Hassanali, A., Teal, P. E. A., et al. (2017). Parasitic Nematode Meloidogyne incognita Interactions with Different Capsicum Annum Cultivars Reveal the Chemical Constituents Modulating Root Herbivory. Sci. Rep. 7, 2903. doi:10.1038/s41598-017-02379-8
Kogan, M. (1998). Integrated Pest Management: Historical Perspectives and Contemporary Developments. Annu. Rev. Entomol. 43 (1), 243–270. doi:10.1146/annurev.ento.43.1.243
Kremer, R. J., and Li, J. (2003). Developing weed-suppressive Soils through Improved Soil Quality Management. Soil Tillage Res. 72, 193–202. doi:10.1016/s0167-1987(03)00088-6
Lehmann, J., Bossio, D. A., Kögel-Knabner, I., and Rillig, M. C. (2020). The Concept and Future Prospects of Soil Health. Nat. Rev. Earth Environ. 1, 544–553. doi:10.1038/s43017-020-0080-8
Lynch, C. A., Olivia, M. S., Chapman, E. G., Crossley, M. S., Crowder, D. W., Fu, Z., et al. (2022). Alternative Prey and Farming System Mediate Predation of Colorado Potato Beetles by Generalists. Pest Manage. Sci. doi:10.1002/ps.6553
McLoughlin, A. G., Wytinck, N., Walker, P. L., Girard, I. J., Rashid, K. Y., de Kievit, T., et al. (2018). Identification and Application of Exogenous dsRNA Confers Plant protection against Sclerotinia sclerotiorum and Botrytis Cinerea. Sci. Rep. 8, 7320. doi:10.1038/s41598-018-25434-4
Mech, A., Gottardo, S., Amenta, V., Amodio, A., Belz, S., Bøwadt, S., et al. (2022). Safe- and Sustainable-By-Design: The Case of Smart Nanomaterials. A Perspective Based on a European Workshop. Regul. Toxicol. Pharmacol. 128, 105093. doi:10.1016/j.yrtph.2021.105093
Mercado-Blanco, J., and Bakker, P. A. H. M. (2007). Interactions between Plants and Beneficial Pseudomonas spp.: Exploiting Bacterial Traits for Crop protection. Antonie van Leeuwenhoek 92 (4), 367–389. doi:10.1007/s10482-007-9167-1
Mobli, A., and Chauhan, B. S. (2020). Crop Residue Retention Suppresses Seedling Emergence and Biomass of winter and Summer Australian weed Species. Weed Biol. Manag. 20 (3), 118–128. doi:10.1111/wbm.12208
Moreau, D., Bardgett, R. D., Finlay, R. D., Jones, D. L., and Philippot, L. (2019). A Plant Perspective on Nitrogen Cycling in the Rhizosphere. Funct. Ecol. 33, 540–552. doi:10.1111/1365-2435.13303
Mortensen, D. A., Egan, J. F., Maxwell, B. D., Ryan, M. R., and Smith, R. G. (2012). Navigating a Critical Juncture for Sustainable weed Management. BioScience 62 (1), 75–84. doi:10.1525/bio.2012.62.1.12
Neher, D., and Barbercheck, M. (2019). Soil Microarthropods and Soil Health: Intersection of Decomposition and Pest Suppression in Agroecosystems. Insects 10 (12), 414. doi:10.3390/insects10120414
Nettles, R., Watkins, J., Ricks, K., Boyer, M., Licht, M., Atwood, L. W., et al. (2016). Influence of Pesticide Seed Treatments on Rhizosphere Fungal and Bacterial Communities and Leaf Fungal Endophyte Communities in maize and Soybean. Appl. Soil Ecol. 102, 61–69. doi:10.1016/j.apsoil.2016.02.008
Niu, D., Hamby, R., Sanchez, J. N., Cai, Q., Yan, Q., and Jin, H. (2021). RNAs - a New Frontier in Crop protection. Curr. Opin. Biotechnol. 70, 204–212. doi:10.1016/j.copbio.2021.06.005
Nunes, M. R., Karlen, D. L., Veum, K. S., Moorman, T. B., and Cambardella, C. A. (2020). Biological Soil Health Indicators Respond to Tillage Intensity: A US Meta-Analysis. Geoderma 369, 114335. doi:10.1016/j.geoderma.2020.114335
Osipitan, O. A., Dille, J. A., Assefa, Y., and Knezevic, S. Z. (2018). Cover Crop for Early Season Weed Suppression in Crops: Systematic Review and Meta‐Analysis. Agron.j. 110, 2211–2221. doi:10.2134/agronj2017.12.0752
Östman, Ö., Ekbom, B., and Bengtsson, J. (2003). Yield Increase Attributable to Aphid Predation by Ground-Living Polyphagous Natural Enemies in spring Barley in Sweden. Ecol. Econ. 451, 149–158. doi:10.1016/s0921-8009(03)00007-7
Palm, C., Blanco-Canqui, H., DeClerck, F., Gatere, L., and Grace, P. (2014). Conservation Agriculture and Ecosystem Services: An Overview. Agric. Ecosyst. Environ. 187, 87–105. doi:10.1016/j.agee.2013.10.010
Palojärvi, A., Kellock, M., Parikka, P., Jauhiainen, L., and Alakukku, L. (2020). Tillage System and Crop Sequence Affect Soil Disease Suppressiveness and Carbon Status in Boreal Climate. Front. Microbiol. 11, 534786. doi:10.3389/fmicb.2020.534786
Parsa, S., Morse, S., Bonifacio, A., Chancellor, T. C. B., Condori, B., Crespo-Pérez, V., et al. (2014). Obstacles to Integrated Pest Management Adoption in Developing Countries. Proc. Natl. Acad. Sci. USA 111 (10), 3889–3894. doi:10.1073/pnas.1312693111
Perotti, V. E., Larran, A. S., Palmieri, V. E., Martinatto, A. K., and Permingeat, H. R. (2020). Herbicide Resistant Weeds: A Call to Integrate Conventional Agricultural Practices, Molecular Biology Knowledge and New Technologies. Plant Sci. 290, 110255. doi:10.1016/j.plantsci.2019.110255
Peters, R. D., Sturz, A. V., Carter, M. R., and Sanderson, J. B. (2003). Developing Disease-Suppressive Soils through Crop Rotation and Tillage Management Practices. Soil Tillage Res. 72, 181–192. doi:10.1016/s0167-1987(03)00087-4
Rivera, M. J., Martini, X., Conover, D., Mafra-Neto, A., Carrillo, D., and Stelinski, L. L. (2020). Evaluation of Semiochemical Based Push-Pull Strategy for Population Suppression of Ambrosia Beetle Vectors of laurel Wilt Disease in Avocado. Sci. Rep. 10, 2670. doi:10.1038/s41598-020-59569-0
Runck, B. C., Kantar, M. B., Jordan, N. R., Anderson, J. A., Wyse, D. L., Eckberg, J. O., et al. (2014). The Reflective Plant Breeding Paradigm: a Robust System of Germplasm Development to Support Strategic Diversification of Agroecosystems. Crop Sci. 54 (5), 1939–1948. doi:10.2135/cropsci2014.03.0195
Rusch, A., Valantin-Morison, M., Sarthou, J. P., and Roger-Estrade, J. (2010). “Integrating Crop and Landscape Management into New Crop protection Strategies to Enhance Biological Control of Oilseed Rape Insect Pests,” in Biocontrol-based Integrated Management of Oilseed Rape Pests (Dordrecht: Springer), 415–448. doi:10.1007/978-90-481-3983-5_17
Rusinamhodzi, L., Corbeels, M., van Wijk, M. T., Rufino, M. C., Nyamangara, J., and Giller, K. E. (2011). A Meta-Analysis of Long-Term Effects of Conservation Agriculture on maize Grain Yield under Rain-Fed Conditions. Agron. Sustain. Dev. 31, 657–673. doi:10.1007/s13593-011-0040-2
Sanchez-Morena, S., and Ferris, H. (2007). Suppressive Service of the Soil Food Web: Effects of Environmental Management. Agric. Ecosyst. Environ. 119, 75–87. doi:10.1016/j.agee.2006.06.012
Scopel, E., Triomphe, B., Affholder, F., Da Silva, F. A. M., Corbeels, M., Humberto, J., et al. (2013). Conservation Agriculture Cropping Systems in Temperate and Tropical Conditions, Performances and Impacts: A Review. Agron. Sust. Dev. 33 (1), 113–130. doi:10.1007/s13593-012-0106-9
Storck, V., Nikolaki, S., Perruchon, C., Chabanis, C., Sacchi, A., Pertile, G., et al. (2018). Lab to Field Assessment of the Ecotoxicological Impact of Chlorpyrifos, Isoproturon, or Tebuconazole on the Diversity and Composition of the Soil Bacterial Community. Front. Microbiol. 9, 1412. doi:10.3389/fmicb.2018.01412
Tooker, J. F., O'Neal, M. E., and Rodriguez-Saona, C. (2020). Balancing Disturbance and Conservation in Agroecosystems to Improve Biological Control. Annu. Rev. Entomol. 65, 81–100. doi:10.1146/annurev-ento-011019-025143
Tsiafouli, M. A., Thébault, E., Sgardelis, S. P., de Ruiter, P. C., van der Putten, W. H., Birkhofer, K., et al. (2015). Intensive Agriculture Reduces Soil Biodiversity across Europe. Glob. Change Biol. 21 (2), 973–985. doi:10.1111/gcb.12752
Turlings, T. C. J., and Erb, M. (2018). Tritrophic Interactions Mediated by Herbivore-Induced Plant Volatiles: Mechanisms, Ecological Relevance, and Application Potential. Annu. Rev. Entomol. 63 (1), 433–452. doi:10.1146/annurev-ento-020117-043507
Turmel, M.-S., Speratti, A., Baudron, F., Verhulst, N., and Govaerts, B. (2015). Crop Residue Management and Soil Health: a Systems Analysis. Agric. Syst. 134, 6–16. doi:10.1016/j.agsy.2014.05.009
United Nations (2015). General Assembly Resolution A/RES/70/1. Transforming Our World, the 2030 Agenda for Sustainable Development. Distr.: General 70/1. New York, NY: United Nations.
van Agtmaal, M., Straathof, A. L., Termorshuizen, A., Lievens, B., Hoffland, E., and de Boer, W. (2018). Volatile-mediated Suppression of Plant Pathogens Is Related to Soil Properties and Microbial Community Composition. Soil Biol. Biochem. 117, 164–174. doi:10.1016/j.soilbio.2017.11.015
Van Deynze, B., Swinton, S. M., and Hennessy, D. A. (2021). Are Glyphosate-Resistant Weeds a Threat to Conservation Agriculture? Evidence from Tillage Practices in Soybean. Am. J. Agric. Econ. 104 (2), 1–28. doi:10.1111/ajae.12243
Wagg, C., Bender, S. F., Widmer, F., and van der Heijden, M. G. A. (2014). Soil Biodiversity and Soil Community Composition Determine Ecosystem Multifunctionality. Proc. Natl. Acad. Sci. 111 (14), 5266–5270. doi:10.1073/pnas.1320054111
Weller, D. M., Raaijmakers, J. M., Gardener, B. B. M., and Thomashow, L. S. (2002). Microbial Populations Responsible for Specific Soil Suppressiveness to Plant Pathogens. Annu. Rev. Phytopathol. 40, 309–348. doi:10.1146/annurev.phyto.40.030402.110010
Werle, C. T., Ranger, C. M., Schultz, P. B., Reding, M. E., Addesso, K. M., Oliver, J. B., et al. (2019). Integrating Repellent and Attractant Semiochemicals into a Push-Pull Strategy for Ambrosia Beetles (Coleoptera: Curculionidae). J. Appl. Entomol. 143, 333–343. doi:10.1111/jen.12594
Wiesel, L., Newton, A. C., Elliott, I., Booty, D., Gilroy, E. M., Birch, P. R. J., et al. (2014). Molecular Effects of Resistance Elicitors from Biological Origin and Their Potential for Crop protection. Front. Plant Sci. 5, 655. doi:10.3389/fpls.2014.00655
Williams, A., and Vries, F. T. (2020). Plant Root Exudation under Drought: Implications for Ecosystem Functioning. New Phytol. 225, 1899–1905. doi:10.1111/nph.16223
Worrall, D., Holroyd, G. H., Moore, J. P., Glowacz, M., Croft, P., Taylor, J. E., et al. (2011). Treating Seeds with Activators of Plant Defence Generates Long‐lasting Priming of Resistance to Pests and Pathogens. New Phytol. 193, 770–778. doi:10.1111/j.1469-8137.2011.03987.x
Xu, Q., Hatt, S., Lopes, T., Zhang, Y., Bodson, B., Chen, J., et al. (2018). A Push-Pull Strategy to Control Aphids Combines Intercropping with Semiochemical Releases. J. Pest Sci. 91, 93–103. doi:10.1007/s10340-017-0888-2
Keywords: soil health, crop protection, research and development, sustainable agriculture, innovation framework, ecological pest management
Citation: Atwood LW, Racette KA, Diggelmann M, Masala CA, Maund S, Oliver R, Screpanti C, Wironen M and Wood SA (2022) Soil Health: New Opportunities to Innovate in Crop Protection Research and Development. Front. Environ. Sci. 10:821742. doi: 10.3389/fenvs.2022.821742
Received: 24 November 2021; Accepted: 09 February 2022;
Published: 09 March 2022.
Edited by:
Lucy Crockford, Harper Adams University, United KingdomReviewed by:
Cristina Lazcano, University of California, Davis, United StatesCopyright © 2022 Atwood, Racette, Diggelmann, Masala, Maund, Oliver, Screpanti, Wironen and Wood. This is an open-access article distributed under the terms of the Creative Commons Attribution License (CC BY). The use, distribution or reproduction in other forums is permitted, provided the original author(s) and the copyright owner(s) are credited and that the original publication in this journal is cited, in accordance with accepted academic practice. No use, distribution or reproduction is permitted which does not comply with these terms.
*Correspondence: K. A. Racette, a2VsbHkucmFjZXR0ZUB0bmMub3Jn; S. Maund, c3RldmUubWF1bmRAc3luZ2VudGEuY29t
†These authors share first authorship
Disclaimer: All claims expressed in this article are solely those of the authors and do not necessarily represent those of their affiliated organizations, or those of the publisher, the editors and the reviewers. Any product that may be evaluated in this article or claim that may be made by its manufacturer is not guaranteed or endorsed by the publisher.
Research integrity at Frontiers
Learn more about the work of our research integrity team to safeguard the quality of each article we publish.