- 1Department of Aquatic and Wetland Management, Bahir Dar University, Bahir Dar, Ethiopia
- 2Department of Biology, Ecology and Biodiversity Research Unit, Vrije Universiteit Brussel, Brussels, Belgium
- 3Laboratory of Aquatic Ecology, Evolution and Conservation, KU Leuven, Leuven, Belgium
- 4Leibniz Institute of Freshwater Ecology and Inland Fisheries, Berlin, Germany
- 5Department of Biology, Bahir Dar University, Bahir Dar, Ethiopia
- 6Department of Biology, Ghent University, Ghent, Belgium
- 7Department of Natural Resource Management, Bahir Dar University, Bahir Dar, Ethiopia
- 8Multidisciplinary Institute for Teacher Education (MILO), Vrije Universiteit Brussel, Brussels, Belgium
Plankton is an integral part of wetland biodiversity and plays a vital role in the functioning of wetlands. Diversity patterns of plankton in wetlands and factors structuring its community composition are poorly understood, albeit important for identifying areas for restoration and conservation. Here we investigate patterns in local and regional plankton richness and taxonomic and functional community composition in riverine papyrus swamps, river mouth wetlands, and lacustrine wetlands in the Lake Tana sub-basin, Ethiopia. Data on phytoplankton, zooplankton, and environmental variables were collected from 12 wetlands during the dry and wet seasons of 2018. Redundancy analysis, and linear mixed effect models, were used to investigate differences in local environmental conditions and variation in plankton community richness and composition between wetland types. We also assessed the ecological uniqueness of the plankton community by calculating the contribution of a single wetland: local contributions to overall beta diversity (LCBD) and contributions of individual species (SCBD) to overall beta diversity (BDTotal). Beta regression models were used to investigate the relationships of LCBD and SCBD to environmental variables, wetland, and taxa characteristics. A total of 85 phytoplankton taxa, distributed among 18 Reynolds functional groups, and 57 zooplankton taxa were observed over the entire set of samples. Local plankton taxon richness was significantly higher in riverine papyrus swamps (mean taxa of 30 phytoplankton and 21 zooplankton) compared to river mouth wetlands (mean taxa of 27 phytoplankton and 13 zooplankton). Several local environmental variables and the composition of the plankton community differed significantly between the three wetland types. The highest phytoplankton ecological uniqueness (LCBD) was detected in lacustrine wetlands, whereas the riverine papyrus swamps had the highest zooplankton ecological uniqueness. Based on our analyses, we recommend protecting the wetlands with high LCBD values and stress the importance of various wetland types for preserving the diverse plankton communities of Lake Tana wetlands.
Introduction
Wetlands are one of the most productive habitats and are of immense socio-economic and ecological importance (De Groot et al., 2012) because they provide a wide range of ecosystem services such as groundwater recharge, water purification, nutrient cycling, shoreline stabilization, cultural, recreational, and educational resources, flood protection, and carbon storage (Everard et al., 2019). Wetlands also host exceptionally high levels of biodiversity, which is attributed to their high spatial and temporal environmental heterogeneity (Keddy et al., 2009). Wetlands are home to numerous organisms including phytoplankton and zooplankton species (hereafter: plankton) (D’Ambrosio et al., 2016; Gogoi et al., 2019), macroinvertebrates (Mereta et al., 2013; Gezie et al., 2019), aquatic plants (Getnet et al., 2021), fish (Aziz et al., 2021), amphibians (Nagel et al., 2021), waterbirds (Aynalem and Bekele, 2008; Chawaka et al., 2018a; Chawaka et al., 2018b), and mammals (Chatterjee and Bhattacharyya, 2021). Phytoplankton form the base of the food chain in aquatic ecosystems and play an important role in overall wetland productivity (Chengxue et al., 2019), while zooplankton are recognized as the main primary consumers. Due to their short life cycle and thus potentially rapid response to anthropogenic disturbance and environmental changes, planktonic organisms are also regarded as ideal bioindicators for assessing the environmental status of wetlands (Wijeyaratne and Nanayakkara, 2020; Chaparro-Herrera et al., 2021).
The plankton community structure differs between tropical and temperate lakes, but research on wetlands is still limited. One of these differences is that smaller zooplankton species (including rotifers) often predominate in tropical lakes, whereas large cladocerans are dominant in temperate lakes (Fernando, 1980; Green, 1994). However, in some Ethiopian tropical highland lakes, the zooplankton communities are dominated by large Cladocerans (Dejen et al., 2004; Fetahi et al., 2011; Haileselasie et al., 2012). In temperate lakes, diatoms often dominate the phytoplankton community during winter and phytoflagellates during the summer (Widdicombe et al., 2010). Chlorophyta and Cyanophyta, as well as diatoms in some cases, generally dominate tropical lakes (Ndebele-Murisa et al., 2010). Wide temperature and light intensity fluctuations are widely regarded as the primary drivers of variation in plankton community structure in temperate systems, whereas hydrological conditions are important in the tropical systems (Loverde-Oliveira et al., 2009; Shatwell et al., 2016).
Hydrological conditions, such as water availability and depth in wetlands affect the interchange of water, sediment, nutrients, organic matter, and organisms between the wetlands and their adjacent water bodies (i.e., river, river-lake, or lake; Chaparro et al., 2018; Feng et al., 2021; Rideout et al., 2021). During high water level periods, inputs from the river, and lake water can also modify local environmental conditions in wetlands by altering dissolved oxygen concentration, conductivity, turbidity, and nutrient levels (Alvarez-mieles et al., 2013; Castillo, 2020). Conversely, during periods of isolation or lower exchange with the river and lake, environmental conditions in the wetlands may be largely driven by autochthonous processes occurring in the water column of the wetland (Cardoso et al., 2012). Thus, variation in hydrological conditions in wetlands results in highly heterogeneous habitats at the wetland scale, ranging from lotic to lentic, turbid to clear water, nutrient-rich to nutrient-poor, frequently disturbed versus relatively stable, and well-vegetated to almost barren conditions (Chaparro et al., 2018). Consequently, plankton communities comprise a huge diversity of life-history traits that vary in response to the environmental conditions in the wetland. These range from small fast-growing phytoplankton taxa adapted to turbulent waters to large slow-growing organisms adapted to more stable conditions (Reynolds, 2002; Padisák et al., 2009; Bhat et al., 2015), and from pelagic filter-feeding to scraping zooplankton taxa associated with vegetation (Gebrehiwot et al., 2017a).
Wetlands can be found in all climatic regions of Ethiopia, with the wetlands of Lake Tana being the second largest in the country after the Gambella wetlands (Menbere and Menbere, 2018). In the Lake Tana sub-basin, wetlands are found mostly along lakeshores, rivers, and stream banks and cover 248 km2 of land (Hunegnaw et al., 2013; Stave et al., 2017; Mengistu, 2018). Previous studies in the Lake Tana sub-basin were focused on the abundance and species richness of lake plankton (Dejen et al., 2004; Imoobe and Akoma, 2008; Gashaye, 2016; Melaku, 2017), fish, waterbirds, and mammals (Aynalem and Bekele, 2008; Mengistu, 2018; Zelelew and Archibald, 2021). Specifically for the wetlands in the sub-basin, benthic invertebrates (Gezie et al., 2017; Gezie et al., 2019), fish (Anteneh et al., 2012), macrophytes (Getnet et al., 2021), and water-birds (Aynalem and Bekele, 2008) have been studied. The capacity of these systems for sediment and nutrient retention has also been investigated (Mucheye et al., 2018). They act as a buffering zone, as nurseries for most of the fish populations in the lake, and as breeding and feeding grounds for waterfowl and mammals (Aynalem and Bekele, 2008; Getahun and Dejen, 2012; Zelelew and Archibald, 2021). Lake Tana and most of these wetlands are also a UNESCO Biosphere Reserve (Kalmbach, 2017) due to their high and unique biodiversity.
This study is focused on river-connected riverine papyrus swamps, river mouth wetlands at the interface between river and lake, and lake-connected lacustrine wetlands (Hunegnaw et al., 2013; Aynalem et al., 2017; Getnet et al., 2021). These wetlands are characterized by seasonal water level variations, which are mostly caused by the unimodal rainfall pattern in the area (Jemberie et al., 2015). The river mouth and lacustrine wetlands are increasingly threatened by livestock grazing and crop cultivation (Wondie, 2018; Abera et al., 2021; Chandrasekharan et al., 2021). Additional environmental pressures for river mouth wetlands are the infestation by the non-native floating water hyacinth (Eichhornia crassipes) (Asmare et al., 2020) and the accumulation of pollutants from nearby cities and human settlements (Abebe and Minale, 2017). Excessive water abstraction for small-scale irrigation, particularly at river mouth wetlands, is particularly common during the dry season (Abebe et al., 2020). Lake water level fluctuations caused by lake water abstractions for major development projects in the sub-basin also had a significant impact on lacustrine wetlands (Alemayehu et al., 2010). These anthropogenic disturbances have been proven to influence the hydrological conditions in other lakes and consequently their plankton diversity (Gownaris et al., 2018; Napiórkowski et al., 2019), and therefore, can be expected to cause both temporal and spatial variation in plankton communities in wetlands of Lake Tana. Generally, plankton communities in wetlands under different hydrological conditions are less studied (but see Cardoso et al., 2012; Rojo et al., 2016; Chaparro et al., 2019). Similarly, plankton communities in Lake Tana wetlands under different hydrological conditions are poorly understood.
In this study, we examined differences in local taxonomic richness and the community composition of plankton, as well as the Reynolds functional phytoplankton composition among wetlands under different hydrological conditions. In addition, we aimed to identify wetlands that support unique plankton communities and therefore require special attention with respect to conservation and restoration. The wetlands studied were (i) riverine papyrus swamps (connected to rivers, R) with substantial papyrus stands, and distant from the lake; (ii) river mouth wetlands at the river-lake interface (connected both to the tributary rivers and the lake, RL), and (iii) lacustrine wetlands (only connected to the lake, L). We hypothesize that plankton communities from wetlands under various hydrological conditions differ in their taxonomic richness and community composition and that relatively undisturbed wetlands (i.e., riverine papyrus swamps) are more diverse and unique than those influenced by human activities.
Material and Methods
Study Area
The Lake Tana sub-basin is located between 10.95◦N to 12.78◦N latitude and from 36.98◦E to 38.25◦E longitude in the highlands of northwestern Ethiopia (Figure 1). The sub-basin covers an area of 15,000 km2, including the Lake, the largest surface water body in the country, with a total surface area of 3,080.8 km2. It is 67 km wide, 84 km long and its mean depth is 9 m (Kebede et al., 2006). The seasonal variation in rainfall is controlled by the northward and southward movement of the intertropical convergence zone resulting in a single rainy season between June and October (Fetene et al., 2018). The hydrogeomorphology of the Lake Tana sub-basin is diverse, with alluvial sediments found in the lower reaches of the tributary rivers (Poppe et al., 2013). Approximately 4.5 million people are living adjacent to the lake and its associated wetlands. More than 500,000 people are directly and indirectly dependent on the lake and its associated wetlands (Vijverberg et al., 2009). The land use in the Lake Tana basin is predominantly cultivated land (7,608.7 km2), water area (2,152.7 km2), bushland (1,438.9 km2), grassland (1,242.1 km2), and forest (546.9 km2) (Tewabe and Fentahun, 2020).
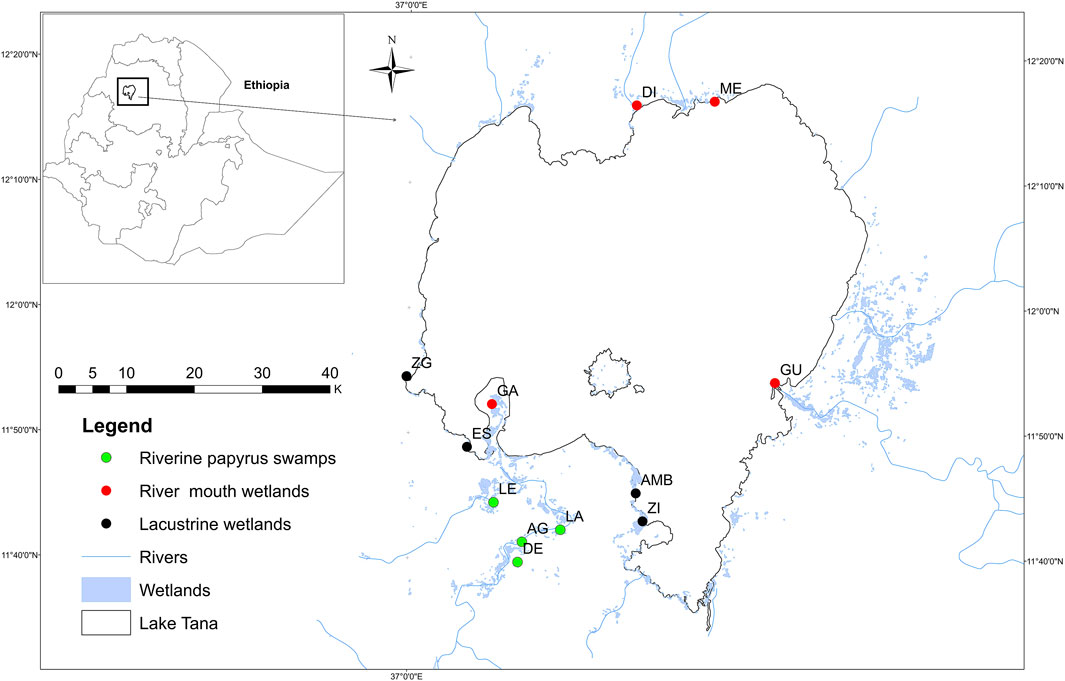
FIGURE 1. Map of Lake Tana and its wetlands; dots show the investigated wetlands divided into the different wetland types (for individual wetland code; see Supplementary Table S1.
The wetlands of Lake Tana are permanent or seasonal with a total area of 248 km2 and distributed from the headwaters of the Guna and Gish-Abay streams to the Fogera and Demba floodplains (Hunegnaw et al., 2013). The majority are located mainly around the lake shores, in the lower reaches of the Gilgel Abay River, and at the river mouths of Gilgel Abay, Ribb, Gumara, and Megech Rivers (Hunegnaw et al., 2013).
The three most common wetland types: riverine papyrus swamps, river mouth wetlands, and lacustrine wetlands in the Lake Tana sub-basin were studied (Figures 1, 2). A total of twelve wetlands (i.e., four wetlands from each wetland type) were selected (Supplementary Table S1). The riverine papyrus swamps sampled are mainly located in the lower reaches of the Gilgel Abay River and are dominated by papyrus (Cyperus papyrus) and other less dominant emergent vegetation (Getnet et al., 2021). With little grazing, settlement, or agricultural activity, these wetlands are characterized with >80% vegetation coverage (Wondie, 2018). These wetlands are permanent swamps with little fluctuation in water level and remain flooded well into the dry season. River mouth wetlands are located at the entrance of the rivers Dirma, Gilgel-Abay, Gumara, and Megech into Lake Tana. The river mouth wetlands are influenced by both lake water levels fluctuation and river flows. During the dry season, most river mouth wetlands have very low water depths due to excessive water abstraction from the inflowing rivers for irrigation by local farmers. River mouth wetlands are characterized by some exotic species and grassy vegetation, with less than 20% cover. The river mouth wetlands included in this study, except the Gilgel Abay wetland, are infested with the non-native water hyacinth (Eichhornia crassipes) (Asmare et al., 2020). Rivers input a large amount of sediment into these wetlands, resulting in the formation of deltas in the majority of them (e.g., in the Gilgel Abay and Gumara wetlands) (Abate et al., 2017; Lemma et al., 2020). Lacustrine wetlands are mostly found on the lake’s southern shores. Lacustrine wetlands have open water-dominated microhabitats with some emergent, submerged, and freely floating macrophyte species with plant cover ranging from 30 to 50%. The river mouth and lacustrine wetlands are home to 27 fish species, which belong to four families: Cichlidae, Clariidae, Nemacheilidae, and Cyprinidae (Dejen et al., 2017).
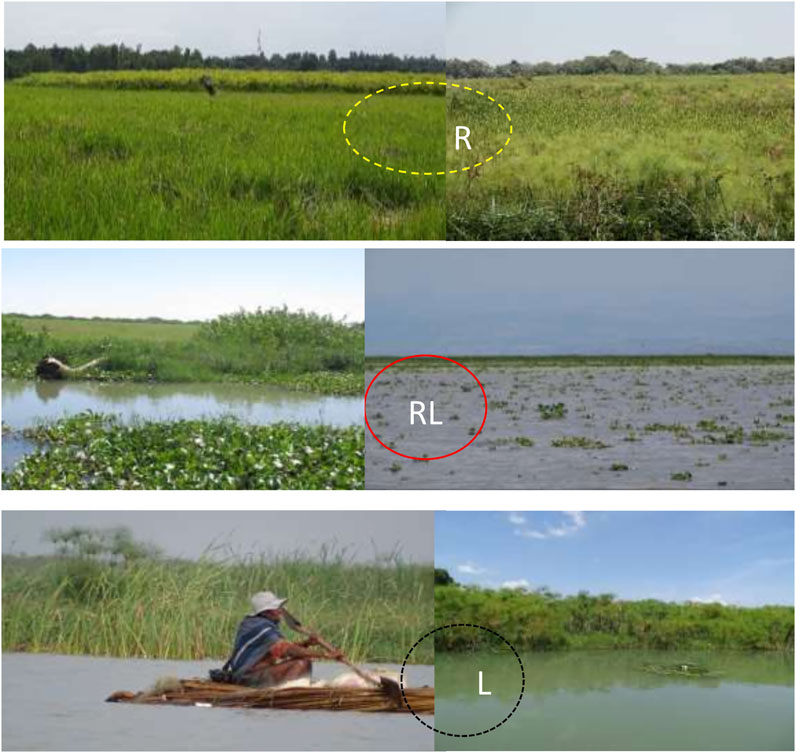
FIGURE 2. Field pictures showing the typical habitats for riverine papyrus swamps (R), river mouth wetlands (RL), and lacustrine wetlands (L).
Field Sampling and Sample Analysis
Sampling for phytoplankton, zooplankton, and environmental variables was carried out from March to May 2018 (in the dry season) and July to October 2018 (in the wet season) in the 12 selected wetlands. A transect line was drawn using Google Earth in the middle part of each wetland ensuring that the entire width of the wetland was covered. In lacustrine and river mouth wetlands, transects started at the edge of the open water and ended at the most outward part of the emergent vegetation that is associated with the wetland. In the riverine papyrus swamp, transects perpendicular to the flow direction were established. Along each transect, 6 plots of 5 × 5 m, at equal distance from each other, were established and marked using GPS (GPSMAP ® ×64). Dissolved oxygen, specific conductance, turbidity, pH, water temperature, water depth, and silt layer (hereafter: sediment depth) were measured from all established plots. Water temperature, pH, specific conductance, and dissolved oxygen were measured in-situ using Multi-probe field meter (YSI 556 MPS). Chlorophyll-a concentration was used as a proxy for phytoplankton biomass and was measured in vivo in the field using a handheld fluorometer (AquaFluor™, Turner Designs). Similarly, turbidity was measured in the field using an aqualytic Turbidimetere (ALT250-IR). Water depth and sediment depth were measured with a wooden stick and a metal measuring tape. For total nitrogen (TN) and total phosphorus (TP), 50 ml water samples were collected from each plot close to the water surface. Samples from different plots along the transect were pooled. The pooled water samples were kept cool in the dark in the field and frozen (−20°C) in the laboratory until further analysis (American Public Health Association, 2005). TN and TP concentrations were measured using a spectrophotometer (HACH, DR/6000) after alkaline persulfate digestion (American Public Health Association, 2005).
Water samples (200 ml) for phytoplankton analysis were collected from the surface of the wetland in each plot. Samples from different plots within each wetland were pooled and subsequently preserved with Lugol’s iodine solution and stored in the dark. In the laboratory, the phytoplankton samples were sedimented for 48–72 h on a heat-free, vibration-free surface, and used for species identification and quantification (Bellinger et al., 2015). A total of 1,190 ml of the supernatant was removed, and the remaining 10 ml concentrated phytoplankton sample was used for further analysis. A 1 ml sub-sample was transferred into the Sedgwick-Rafter cell, which had 1,000 grids, after being fully homogenized by gentle inversion and agitation. Phytoplankton was identified to the species level using taxonomic literature (Tilman et al., 1986; Taylor et al., 2007; Bellinger et al., 2015) and an inverted microscope at a ×1,000 magnification (Krüss, A. Krüss Optronic, Germany). The numbers of phytoplankton cells (single-cell, filament, and colony) of different phytoplankton species in 100 random fields were determined (Hötzel and Croome, 1999).
Samples for zooplankton were collected from the surface of the wetland in each plot by filtering 40 L of water through a 64 µm mesh. Samples from different plots within each wetland were pooled and subsequently preserved in 4% formaldehyde solution in the field and stored at 4°C. For identification and estimation of density, the concentrated initial sample was mixed homogeneously and a 1 ml subsample was taken with a wide opening Stempel pipette and then poured into a Sedgwick–rafter cell (Wetzel and Likens, 2013). This process was replicated until at least 300 rotifer individuals were counted. Additional subsamples were taken for Copepods and Cladocerans when the number of individuals was less than 50 for at least one species (Mack et al., 2012). Zooplankton was identified to the species level under a stereoscopic microscope at ×400 magnification, by using the relevant taxonomic literature (Idris, 1983; Van de Velde, 1984; Koste and Shiel, 1987; Koste and Shiel, 1989; Defaye, 1988; Sinev, 2016). Calanoids and cyclopoids were enumerated according to their developmental stages (adults, copepodites, and nauplii), whereas for rotifers and cladocerans all stages were counted as one age class.
Data Analysis
Data were analyzed using a combination of univariate and multivariate statistical methods. The environmental variables that were measured at several plots within each wetland were averaged for each wetland for the wet and dry seasons separately. Local diversity was calculated for each wetland as the local number of taxa (α - richness) in a given wetland) during the wet and dry seasons separately. Similarly, we determined regional taxon richness (γ-richness) as the total number of taxa across wetlands for the dry and wet seasons separately (Magurran, 2004). The recorded phytoplankton species were classified into Reynolds Functional Groups (RFGs) following Reynolds (2002) and updated by Padisák et al. (2009) to identify dominant functional groups in each wetland.
An ordination plot of a Principal component analysis (PCA) based on standardized and centered environmental data was used to visualize the relationship between environmental variables and wetland types during the dry and wet seasons. The overall effect of wetland type, season, and their interactions on environmental variables, plankton community composition, and Reynolds Functional groups was tested using redundancy analysis (RDA). The significance of RDA models was evaluated with 999 Monte Carlo permutations. The dependency of multiple observations within each wetland type was taken into account by restricting the permutations to blocks (Supplementary Table S5). Prior to these analyses, environmental variables were standardized by subtracting their mean and dividing by their standard deviation, whereas plankton communities data were Hellinger transformed (Legendre and Gallagher, 2001). RDA was also used to test the effect of environmental variables on the composition of plankton communities (ter Braak and Schaffers, 2004).
Linear mixed-effects models (LMMs) were constructed with the package lme4 version 1.1–27.1 (Bates et al., 2011) to test for differences between wetland types and seasons for each environmental variable, local taxonomic richness, and Reynolds Functional groups. Wetland type and season were included as fixed factors in these analyses, while wetland ID was included as a random factor to take into account the temporal dependency of observations from the wet and dry seasons of each wetland. Distributional assumptions of linear models (normality and homoscedasticity of residuals) were checked for each response variable prior to the analyses. Statistical significances of the fixed factors were tested using Satterthwaite’s approximation method of the lmerTest R package version 3.1–3 (Kuznetsova et al., 2017). Posthoc tests were implemented in the lsmeans R package version 2.30–0 (Lenth and Lenth, 2018). The relative importance of fixed and random effects in LMMs was assessed using the marginal R2 and conditional R2 (Nakagawa and Schielzeth, 2013).
To identify wetlands supporting a unique planktonic community, Local Contribution to Beta Diversity in plankton data sets (LCBD; ecological uniqueness of each wetland in their phytoplankton and zooplankton communities) was calculated following the method proposed by Legendre and De Cáceres (2013). Wetlands with higher LCBD values exhibit substantial dissimilarity in species compositions and may have high or low species richness, which should be given more attention in terms of conservation (Legendre and De Cáceres, 2013; da Silva Brito et al., 2020). In addition, calculations were extended to measure variability in plankton communities (BDTotal) and Species Contribution to Beta Diversity (SCBD) (Legendre and De Cáceres, 2013). BDTotal varies from zero (totally similar wetlands) to 1 (dissimilar wetlands). The SCBD value denotes the relative importance of each species in affecting BDTotal patterns. LCBD, SCBD, and BDTotal were calculated for each wetland type and the entire study region from Hellinger transformed plankton abundance data using the beta. div function in the adespatial R package version 0.3–14 (Dray et al., 2018). The significant difference in individual LCBD (each wetland’s contribution to BDTotal) was tested by permutation (999 runs) for the entire study region according to the procedures described in Legendre and De Cáceres (2013). The significant difference in overall LCBD (wetland types’ contribution to BDTotal) was tested using the linear mixed effect model. Wetland type and season were included as fixed factors in these analyses, while wetland ID was included as a random factor to take into account the temporal dependency of observations within each wetland type. We used beta regression as our modeling tool to investigate the relationship between LCBD and environmental variables, LCBD, and species data, SCBD and sampling sites, and SCBD and species data because LCBD and SCBD varied between 0 and 1 (Zeileis et al., 2010). We used beta regression with logit link function from the betareg R package version3.1-4 (Zeileis et al., 2010) for three separate models. First, we related LCBD to species richness, community abundance, and their quadratic terms. Second, we ran beta regression of LCBD using ten environmental variables as predictors (i.e., pH, specific conductance, water temperature, water depth, turbidity, sediment depth, chlorophyll-a, total nitrogen, total phosphorus, and DO). Third, we used beta regression to relate LCBD to the species richness and community abundance (TotAbu). Forth, we used beta regression to relate SCBD to the number of sites occupied (NumSit), the species abundance (SpeAbu), and their quadratic terms.
All basic statistics were conducted in R (CoreTeam, 2020; Version 3.6.3), ggpubr R package version 0.4.0 (Kassambara, 2020). Figures were produced using the ggplot2 R package version 3.3.5 (Wickham, 2016).
Results
Environmental Variables
RDA analyseis revealed that wetland type and season both had a significant effect on the entire set of investigated environmental variables (R2 adj: = 38.6, 4.13%, respectively; Table 1). The first and the second PCA axis jointly explained 54.42% of the overall variation in environmental variables (Figure 3). The first axis explained 33.57% of the variation and was positively associated with water depth, and negatively with TP and pH, generally separating the riverine papyrus swamps from the other wetland types. The second axis explained 20.85% of the variation of the environmental variable and was closely associated with turbidity, specific conductance, and sediment depth. The linear mixed model revealed that, except for chlorophyll-a and total nitrogen, the measured environmental variables differed significantly among wetland types (Supplementary Table S2). The concentration of dissolved oxygen, pH and concentration of total phosphorus were significantly higher in river mouth wetlands compared to riverine papyrus swamps and lacustrine wetlands (Figures 4B, D, I). Water temperature was significantly higher in river mouth wetlands compared to riverine papyrus swamps (Figure 4C). Turbidity was significantly higher in river mouth wetlands compared to riverine papyrus swamps and lacustrine wetlands (Figure 4E). Riverine papyrus swamps had significantly higher sediment depth and electrical conductivity (expressed as specific conductance) compared to lacustrine wetlands (Figures 4F,G). Water depth was significantly higher in riverine papyrus swamps compared to river mouth wetlands (Figure 4H). We also observed considerable differences in water temperature and pH between seasons and the values were higher during the dry season (Supplementary Table S2). Overall, fixed factors (R2 marginal) explained more variance in local environmental conditions than random factors (R2 condition- R2 marginal). However, for chlorophyll-a and total nitrogen concentration, the random factor (Wetland ID) was relatively more than fixed factors (Supplementary Table S2).
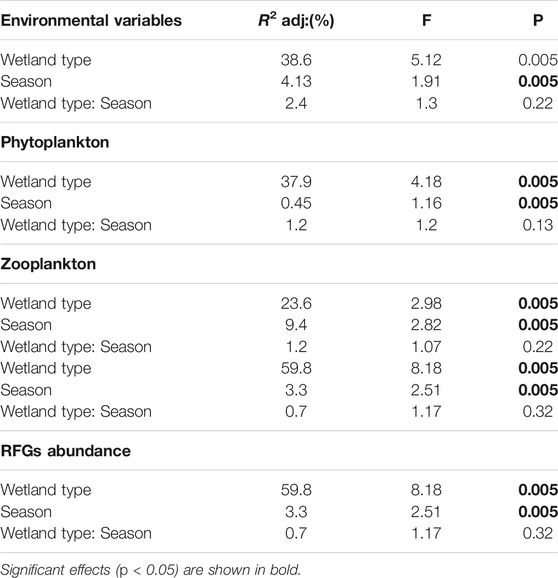
TABLE 1. Results of redundancy analysis (RDA) testing for the effect of wetland types, season, and their interactions on the environmental variables, plankton community composition, and Reynolds functional groups.
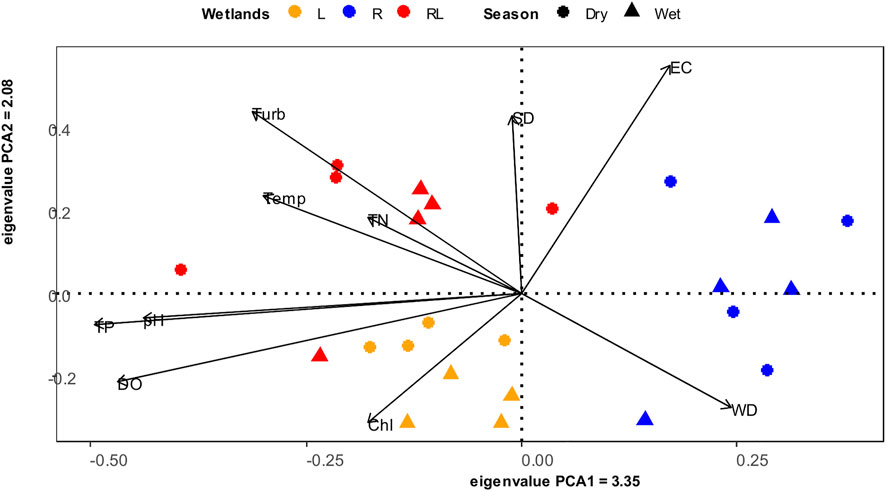
FIGURE 3. Biplots of Principal Component Analysis (PCA) of environmental variables in relation to different wetland types during the dry and the wet seasons. pH, SD sediment depth, WD water depth, Chl chlorophyll-a, EC specific conductance, Turb turbidity, DO dissolve oxygen concentration, and Temp temperature.
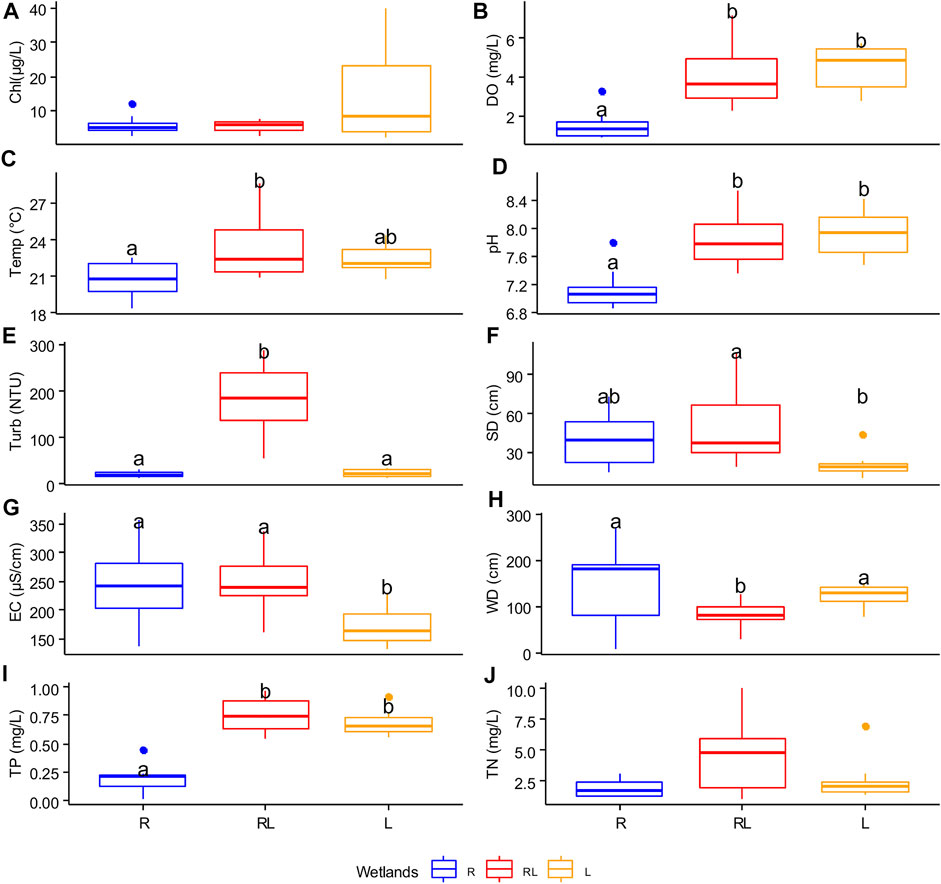
FIGURE 4. Boxplot plots with the median (solid line) for each measured environmental variable (A–J) in R = riverine papyrus swamps, RL = river mouth wetlands, and L = lacustrine wetlands (data were combined from dry and wet seasons). pH, SD sediment depth, WD water depth, Chl chlorophyll-a, EC specific conductance, Turb turbidity, DO dissolve oxygen concentration, and Temp temperature. Boxes that do not have a letter in common are significantly different from each other.
Local, and Regional Taxonomic Richness in Phytoplankton and Zooplankton
A total of 87 phytoplankton taxa belonging to seven main divisions, namely Chlorophyta (43 taxa), Bacillariophyta (25 taxa), Cyanophyta (10 taxa), Euglenophyta (3 taxa), Dinoflagellates (3 taxa), Xanthophyta (2 taxa), and Cryptophyta (1 taxon) were identified as regional taxon richness (Supplementary Table S3). The linear mixed model revealed that phytoplankton local taxonomic richness differed significantly among wetland types (Supplementary Table S2; Figure 5A). Seasonal variation, on the other hand, was insignificant (Supplementary Table S2). The overall mean local taxonomic richness of phytoplankton (data were combined for the dry and the wet seasons) was 29 taxa (25-34 taxa) in the lacustrine wetlands, 30 (20-44 taxa) in the riverine papyrus swamps, and 21 (14-31 taxa) in the river mouth wetlands.
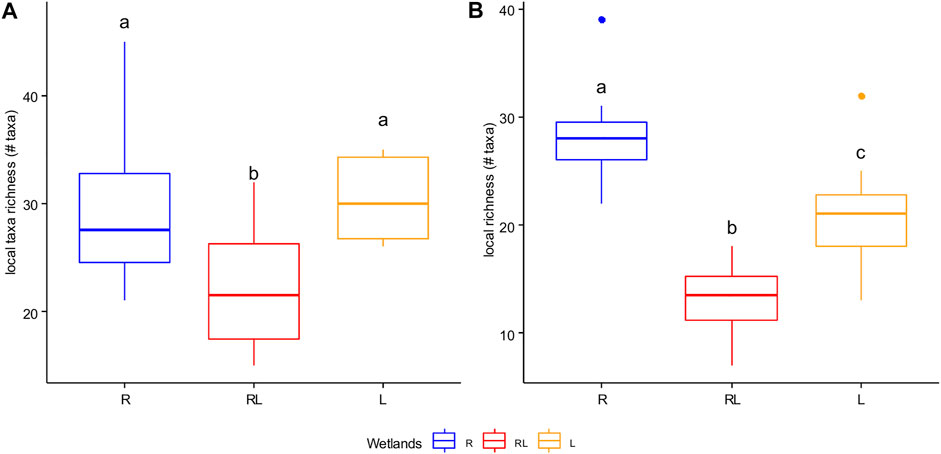
FIGURE 5. Boxplot with the median (solid line) for local taxonomic richness (number of taxa) of phytoplankton (A) and zooplankton (B) in R = riverine papyrus swamps, RL = river mouth wetlands, and in L = lacustrine wetlands. Data were combined for the dry and the wet season. Boxes that have no letter in common significantly differ from each other.
A total of 57 zooplankton taxa representing Cladocerans (24 taxa), Copepods (9 species), and Rotifers (24 taxa) were identified (Supplementary Table S4). Copepods were composed of Cyclopoids and one Calanoid species, i.e., Thermodiaptomus galebi lacustris. The linear mixed model revealed that zooplankton local taxonomic richness differed significantly among wetland types and seasons (Supplementary Table S2; Figure 5B). The overall mean local taxonomic richness of zooplankton (data were combined the dry and the wet seasons) was 21 taxa (13-31 taxa) in the lacustrine wetlands, 27 (25-37 taxa) in the riverine papyrus swamps, and 13 (9-17 taxa) in the river mouth wetlands.
Plankton Community Composition
The redundancy analysis (RDA) revealed a significant variation in plankton community composition and Reynolds Functional groups among wetland types and seasons (Table 1). The RDA analyses revealed a significant effect of wetland types on phytoplankton community composition (R2 adj: = 37.9%; Table 1). The season had a minor impact, despite being statistically significant (R2 adj: = 0.45%). The first and second axes of the RDA based on phytoplankton, explained 36.62% of the total compositional variation in phytoplankton community (Figure 6A). The first axis explained 21.15% of the total variation and mainly separated river mouth wetlands with high turbidity from lacustrine wetlands and riverine papyrus swamps. The second axis, which explained 15.45% of the total variation, separated riverine papyrus swamps with deep water levels from the lacustrine and river mouth wetlands. Although, the majority of phytoplankton taxa showed a positive association with lacustrine wetland types, Aulacoseira italica (AI), Mougeotia laetevirens (ML), Navicula cryptocephala (NAC), Nitzschia minuta (NM), Spirogyra fluviatilis (SF), Synedra ulna (SU), Pediastrum simplex (PS), and Tribonema minus (TM) tended to be slightly more abundant in riverine papyrus swamps. In river mouth wetlands, Cymbella minuta (CM), Gomphonema minutum (GM), Fragilaria capucina (FC), Gomphonema gracile (GG), Nitzschia reversa (NR), Rhoicosphenia abbreviate (RA), and Rhopalodia gibba (RG) were more abundant.
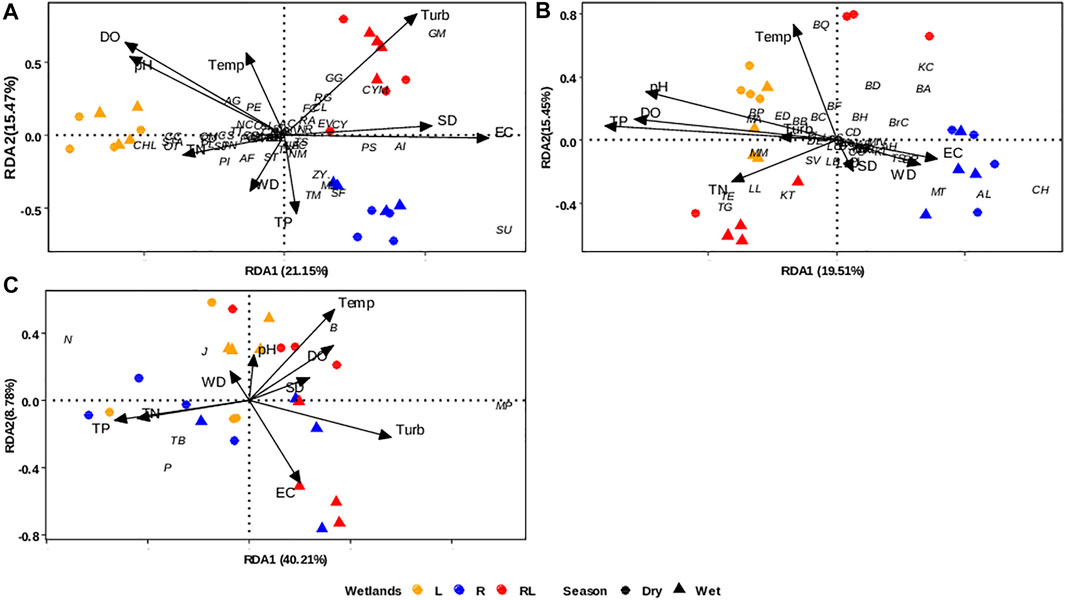
FIGURE.6. Triplots of Redundancy analysis (RDA) for phytoplankton taxa (A), zooplankton taxa (B) and Reynolds Functional Groups (C) in L = lacustrine wetlands (orange color), R = riverine papyrus swamps (blue color), and RL = river mouth wetlands (red color) during the dry season (circle) and the wet season (triangle); pH, SD sediment depth, WD water depth, EC specific conductance, Turb turbidity, DO dissolve oxygen concentration, and Temp temperature. The full names of the plankton taxa indicated as code are given in Supplementary Tables S3, S4.
The phytoplankton species were grouped into 18 Reynolds functional groups (Supplementary Table S3). The functional groups B, MP, N, P, J, and TB accounted for more than 85% of the total phytoplankton abundance. Group B represented by the diatom Aulacoseira italica was the most dominant species comprising 29.4% of the total abundance followed by groups P (15.6%), J (13.71%), MP (12.11%), N (12.02%), and TB (5.97%). Differences in Reynolds functional groups abundance were especially strong between wetland types (R2adj: = 59.8%), whereas season explained a relatively small proportion of variation (R2 adj: = 3.3%; Table 1). The first and the second RDA axis jointly explained 48.99% of the overall variation in Reynolds functional groups abundance (Figure 6C). The first axis comprised 40.21% of the compositional variation in the Reynolds functional groups. The first axis clearly differentiated the river mouth wetlands from the lacustrine wetlands and riverine papyrus swamps (Figure 6C). Groups B and MP were dominant in river mouth wetlands (towards the positive side of the first axis), which was correlated with turbidity. Groups N and P were dominant in lacustrine wetlands (towards the negative side of the first axis), which was correlated with TP and TN concentrations. The second RDA axis accounted for 8.78% of the variation in Reynolds functional groups. Linear mixed models revealed that the abundances of groups B, J, N, MP, and P differ significantly among wetland types, while only group TB significantly varied between seasons (Supplementary Table S2). The group MP was significantly higher in river mouth wetlands compared to lacustrine wetlands (Figure 7A). The group TB did not differ between wetland types (Figure 7B). Group B was significantly higher in riverine papyrus swamps compared to lacustrine wetlands (Figure 7C). The group N was significantly higher in lacustrine wetlands compared to river mouth wetlands (Figure 7D). The groups P and J were slightly higher in lacustrine wetlands compared to the other two wetland types (Figures 7E,F). Except for group B, where wetland identity was more relevant, wetland types and season (Marginal R2) explained more variance in most RFGs (Supplementary Table S2). The habitat preferences, representative taxa, tolerance, and sensitivity of dominant phytoplankton RFGs in the studied wetland types are shown in Table 2.
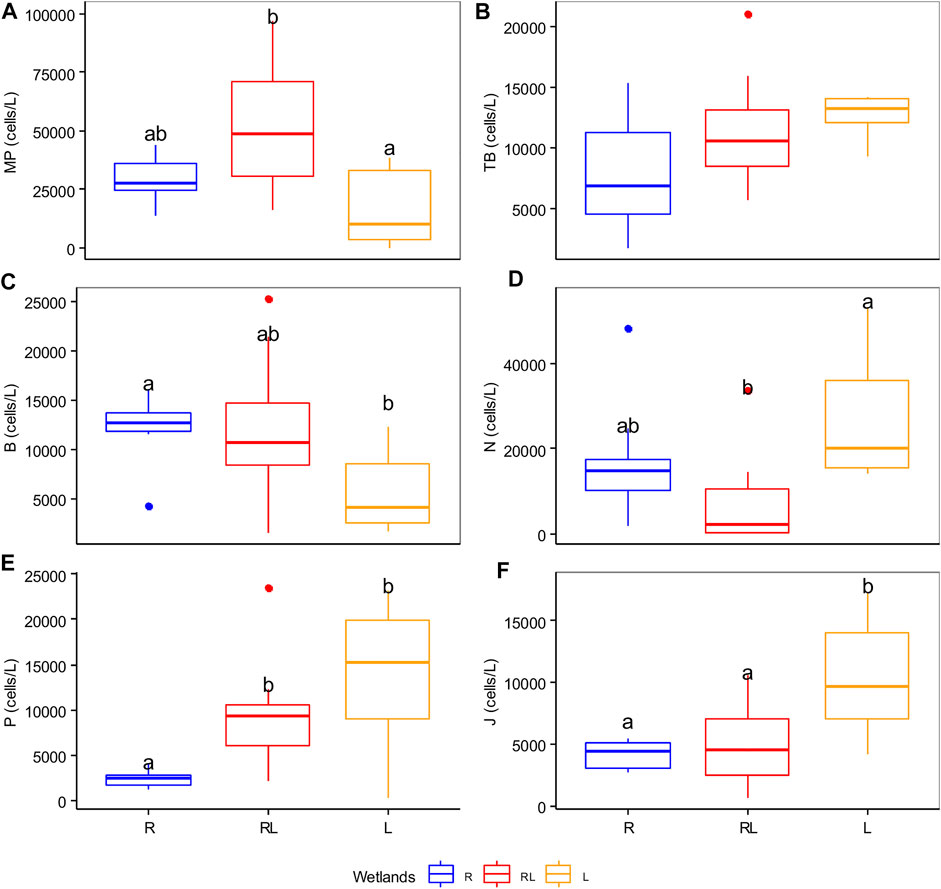
FIGURE 7. Boxplot with the median (solid line) for the abundance of Reynolds functional groups (A–F). Data from the dry and wet seasons were combined. Boxes that have no letter in common significantly differ from each other.
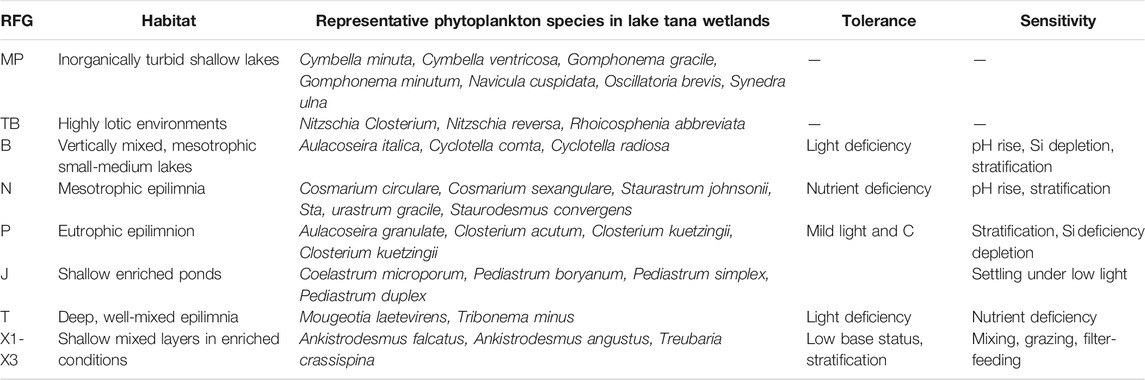
TABLE 2. Description of the main phytoplankton RFGs (with>3% contribution) in wetlands of lake Tana and representative taxa (Reynolds, 2002; Padisák et al., 2009).
Zooplankton community composition differed between wetland types (R2adj: = 23.6%) and season. However, season only explained a relatively small proportion of variation (R2 adj: = 9.4%; Table 1). The first and the second RDA axis jointly explained 34.56% of the overall variation in zooplankton community composition (Figure 6B). The first axis comprised 19.51% of the compositional variation in the zooplankton community and differentiated riverine papyrus swamps from the lacustrine and river mouth wetlands. Zooplankton taxa associated with these riverine papyrus swamps were Acroporus harpae (AH), Alona spp. (AL), Chydorus spp. (CH), Ectocyclops rubescens (ER), Mesocyclops kieferi (MK), Microcyclops varicans (MV), Macrothrix triserialis (MT), and Kurzia longirostris. In river mouth wetlands, Brachionus caudatus (BC), Brachionus diversicornis (BD), Brachionus quadridentatus (BQ), and Branchionus calyciflorus (BrC) tended to be slightly more abundant during the dry season, while Thermocyclops ethiopiensis (TE), Thermodiaptomus galebi (TG), Keratella tropica (KT), Lecane bulla (LB), and Lecane luna were abundant during the wet season.
Local Contribution and Species Contribution to Beta Diversity (BDTotal) of Plankton
The total beta diversity (BDTotal) of phytoplankton for the entire data set was 0.61 and 0.62 during the dry and the wet season, respectively (Supplementary Table S6). LCBD values of phytoplankton for the entire data set ranged between 0.05 and 0.12 during the dry season and between 0.06 and 0.11 during the wet season. Lacustrine wetlands’ contribution to phytoplankton LCBD was significantly higher compared to riverine papyrus swamps (Supplementary Table S2; Figure 8A). For all data, the Zegie-yiganda lacustrine wetland was statistically significant for single wetland contribution (LCBD) of phytoplankton (P.adj = 0.037). The BDTotal of zooplankton for the entire data set was 0.56 and 0.47 for the dry and the wet seasons, respectively (Supplementary Table S6; Figure 8B). LCBD of zooplankton for the entire data set ranged between 0.06 and 0.15 during the dry season and between 0.07 and 0.10 during the wet season. Although three of the four wetlands of riverine papyrus swamps (Amba-giorgis, Dehna-mesenta, and Legdiya) contributed more than the average to zooplankton BDTotal (LCBD), there was no significant difference between wetland types (Supplementary Table S2; Figure 8B). For all data, the Megech river mouth wetland was statistically significant for single wetland contribution (LCBD) of zooplankton (P.adj = 0.003).
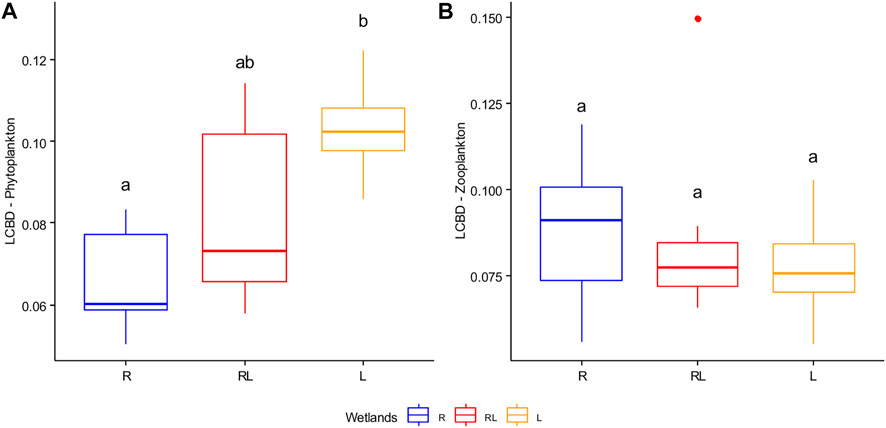
FIGURE 8. Boxplot with the median (solid line) for Local Contribution to beta diversity (LCBD) values for phytoplankton data (A) and zooplankton data (B) in R = riverine papyrus swamps, RL = river mouth wetlands, and L = lacustrine wetlands. Data were combined for the dry and the wet season. Boxes that have no letter in common significantly differ from each other.
The LCBD of phytoplankton data was significantly related to pH (Estimate = 0.27; z = 2.08, p = 0.04), whereas the LCBD of zooplankton data was significantly related to water depth (Estimate = 0.00; z = 2.76, p = 0.005) and sediment depth (Table 3; Estimate = 0.00; z = 2.41, p = 0.02). Also, pseudo R2 values of these models were moderate, 56% of the variation in LCBD of phytoplankton and 41% in LCBD of zooplankton was explained by all environmental variables included (Table 2). However, there was no significant relationship between LCBD values and taxa richness, abundance, or quadratic terms in either of the plankton communities (Supplementary Table S7).
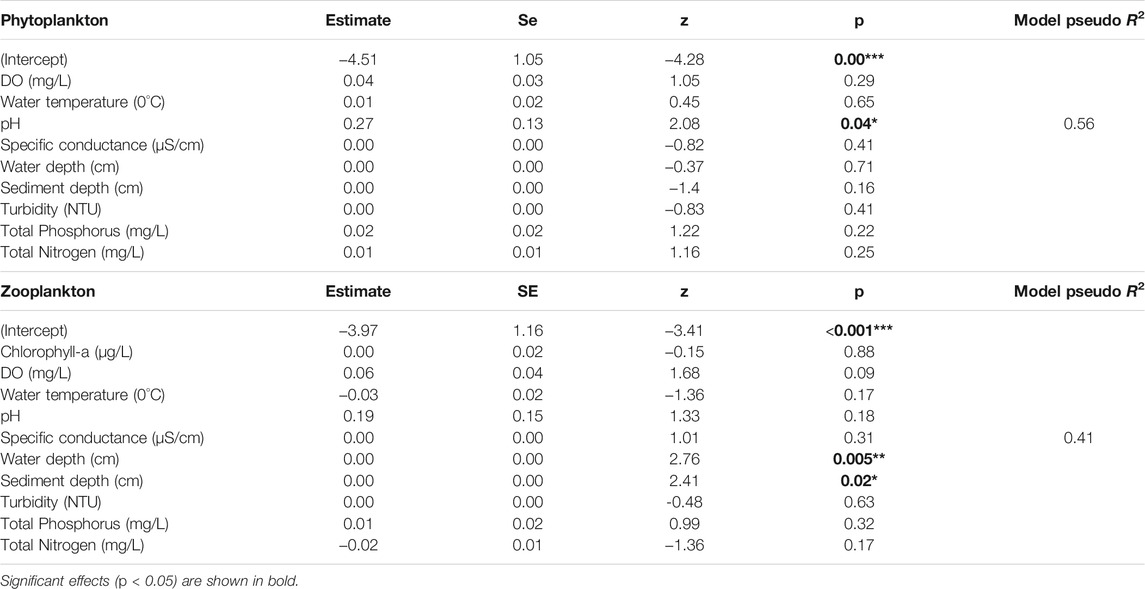
TABLE 3. Results of beta regression analyses when the response variable, local contributions to beta diversity (LCBD), was explained by local environmental variables.
The SCBD values for the entire data set of phytoplankton ranged from 0 to 0.108 and 15 out of 80 phytoplankton taxa had an above average (0.0125) contribution to BDTotal during the dry season. The SCBD for the wet season ranged from 0 to 0.12, with 21 out of 81 phytoplankton taxa contributing more than the average (Supplementary Table S8). Zooplankton SCBD values for the entire data set during the dry season ranged between 0 and 0.08, and 15 out of 55 zooplankton taxa contributed above the mean (0.018) to BDTotal. SCBD during the wet season ranged between 0.00027 and 0.074, and 11 out of 57 zooplankton taxa contributed above the average (0.017) (Supplementary Table S6).
The SCBD of phytoplankton was related to the number of sites occupied and its quadratic term, which accounted for 29% of the variation, whereas abundance and its quadratic term accounted for 62% of SCBD variation of phytoplankton (Table 4). SCBD of Zooplankton was found to be significantly related to the number of sites occupied and its quadratic term, which accounted for 47% of the variation. The SCBD of Zooplankton was also significantly related to abundance and its quadratic term, which accounted for 43% of the variation (Table 4).
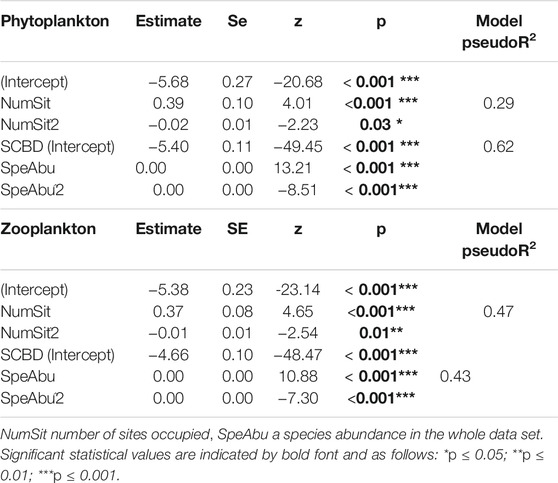
TABLE 4. Results of beta regression analyses when species contributions to beta diversity (SCBD) was explained by species abundance, site occupancy, and their quadratic terms.
Discussion
Wetlands in Lake Tana are heterogeneous, as evidenced by differences in environmental conditions such as the water depth, pH, dissolved oxygen, and turbidity of the water, as well as by the differences in the local richness and composition of the planktonic communities in the different wetland types. These differences could be attributed to differences in local habitat conditions as well as in variation in hydrological conditions between wetlands. In our study, river mouth wetlands were highly turbid, warmer, and shallower, whereas riverine papyrus swamps and lacustrine wetlands were characterized by extended areas of emergent macrophytes, low turbidity, and deeper water levels.
The observed lower phytoplankton local taxa richness in river mouth wetlands compared to riverine papyrus swamps and lacustrine wetlands might be related to the higher turbidity in the former wetland type. Increased turbidity results in lower light availability for wetland phytoplankton (Allende et al., 2009), which in turn might select for a lower number of taxa specifically adapted to low light intensities. This is in line with earlier studies (Sharma and Singh, 2018; Gogoi et al., 2019), which found low phytoplankton richness in turbid aquatic ecosystems. Many authors suggested that water depth is the primary hydrological factor influencing phytoplankton species richness and community composition in wetlands (Casali et al., 2011; Chaparro et al., 2018; Xiao et al., 2020). This is because water depth influences the wetland’s persistence, stability, and size (Chaparro et al., 2018), and local environmental conditions (Xiao et al., 2020), which, in turn, have an impact on phytoplankton communities. Our findings revealed that water depths were shallower in river mouth wetlands than in riverine papyrus swamps and lacustrine wetlands, which might be another reason for the lower phytoplankton richness in the former wetland type. Similarly, phytoplankton species richness was found to be lower during the low water phase than during the high water phase in one of Brazil’s wetlands (Cardoso et al., 2012). This, however, contradicted the broader paradigm, which holds that low water levels in wetlands result in heterogeneous microhabitats as the wetlands become more isolated from one another and disconnected from the main river channel (Tockner et al., 2000). In addition to variation in water depth, the presence of macrophytes in riverine papyrus swamps might have benefited the shade-tolerant species of Bacillariophyta and small-sized species of Chlorophyta that were dominant in these wetlands (Padisák et al., 2009). Macrophytes have also been shown to promote the growth of periphytic phytoplankton species such as Spirogyra fluviatilis, Mougeotia laetevirens, Aulacoseira italica, Gonatozygon monotaenium, and Oedogonium spp. (Rodríguez et al., 2012), which were also dominant in the riverine papyrus swamps in our study.
The high local taxa richness of zooplankton in riverine papyrus swamps might be attributed to the varying water depth (30–300 cm depth) observed in these wetlands. In general, variation in water depth within wetlands promotes different niches that support many zooplankton species (Adamczuk, 2014; Chaparro et al., 2018). Thus, various species can be seen along a water depth gradient, which can be related to UV exposure, thermal properties, and food resources associated with varying water depths. Here as well, the extensive macrophyte cover in riverine papyrus swamps might also provide a complex habitat (e.g., space and food resources) for zooplankton but especially for epibenthic generalists (Bolduc et al., 2016; Gebrehiwot et al., 2017a; Gogoi et al., 2018) such as Alona spp. Chydorus spp. and Macrothrix triserialis which were dominant zooplankton species in these wetlands. Epibenthic generalists attach to substrates such as underwater stems or leaf surfaces and their low activity makes them less likely to be detected by macrophyte-associated predators compared to planktonic species (Gogoi et al., 2018).
The regional taxonomic richness in the plankton of the Lake Tana wetlands is difficult to compare with other wetlands because the number of species is strongly dependent on the number of samples analyzed and the taxonomic accuracy in the analysis. However, the 85 phytoplankton taxa found was higher than the 36 phytoplankton species in the wetlands of India’s Sundarbans wetlands (Gogoi et al., 2019), 53 species in India’s Chatla wetlands (Laskar and Gupta, 2013), and 36 species in Diyawannawa wetland of Sri Lanka (Wijeyaratne and Nanayakkara, 2020). However, the regional phytoplankton taxa richness in our wetlands was lower than the 360 phytoplankton species in the Bhoj wetland of India (Bhat et al., 2015), 97 species in of Pantanal wetland of South America (Cardoso et al., 2012), and 200 species in Danube Riverine wetlands of Austria (Chaparro et al., 2018). Similarly, the regional taxon richness of zooplankton in our study was higher than the 32 species reported from the wetland of Opa Reservoir (Adebayo et al., 2021), but lower than the 128 species reported from the Yangtze River floodplain (Lu et al., 2021).
In addition to differences in local taxonomic richness, we also found significant differences in plankton community composition, studied at the functional (RFG) and taxonomic level, and the zooplankton communities between the different wetland types. The phytoplankton in riverine papyrus swamps was dominated by Reynolds functional group B, which was represented by Aulacoseira italica; a member of a cosmopolitan genus with a tychoplanktonic live style (Denys et al., 2003; Bicudo et al., 2016). The phytoplankton communities in the river mouth wetlands were dominated by the MP group, which corroborates its presence in inorganically turbid lakes and rivers elsewhere (Padisák et al., 2009; Stević et al., 2013; Udovic et al., 2014). This group was also dominant within the macrophyte stands in Lake Ziway (Gebrehiwot et al., 2017b), a lake that is highly turbid and heavily impacted by intensive agricultural activities and urbanization (Gebrehiwot et al., 2017a; Merga et al., 2020). In addition, species that predominantly contributed to the Reynolds functional group MP, such as Rhopalodia gibba, Gomphonema minutum, Rhoicosphenia abbreviate, Nitzschia reversa, Gomphonema gracile, Cymbella minuta, and Nitzschia closterium have been reported from running waters elsewhere (Leira et al., 2017; Shen et al., 2018). The lacustrine wetlands are dominated by the J, P, and N groups which prefer shallow enriched water bodies with continuous or semi-continuous mixed layers of 2–3 m in thickness (Reynolds, 2002). The zooplankton communities in the riverine papyrus swamps were dominated by the cladoceran functional groups. This is most likely due to the high density of macrophyte stands dominated by Cyperus papyrus, which may have served as a foraging and attachment site for non-active epiphytic zooplankton (e.g., Alona and Chydorus spp.; Choi et al., 2015). This is consistent with what was found in other studies in Lake Ziway (Gebrehiwot et al., 2017a) and Lake Tana (Wudneh 1998). In the present study, a considerably higher diversity of rotifers was recorded in river mouth wetlands. The most abundant species found in these wetlands (B. caudatus, B. diversicornis, B. quadridentatus, B. calyciflorus, K. tropica, L. bulla, and L. luna) were cosmopolitan planktonic species that are generalists that feed on bacteria, detritus, and flagellates (Yin et al., 2018; García-Chicote et al., 2019). Some of these species are also known to be tolerant of differences in temperature, dissolved oxygen, and turbidity (Segers and De Smet, 2007). As a result, some of these species are expected to predominate in river mouth wetlands, which have higher turbidity, higher temperature, and low dissolved oxygen concentrations.
The phytoplankton in the lacustrine wetlands and particularly that in the Zegie-yiganda wetland, with its deep open water and diverse microhabitats, appeared to be relatively unique. The positive correlation between pH and LCBD values for phytoplankton in our study confirms that pH is one of the environmental factors that affect phytoplankton directly or through its influence on the bioavailability of nutrients (Chakraborty et al., 2011). The zooplankton in the Megech river mouth wetland, but particularly the communities in the riverine papyrus swamps, were relatively unique. For the Megech river mouth wetland, this is due to its low richness and severely degraded state and therefore restoration actions are recommended. The zooplankton in the riverine papyrus swamps consisted of unique communities. This, together with the presence of unique taxa in waterbirds in these wetlands (e.g., Zelelew et al., 2020) underlines the need that these wetlands require special protection (Brito et al., 2020). The LCBD values of zooplankton were found to be positively related to sediment and water depth. Indeed, water depth is likely to influence zooplankton community composition by altering the other environmental variables such as turbidity, dissolved oxygen, specific conductance, and nutrient concentrations, as well as the cover and structure of macrophytes in wetlands (Chaki et al., 2021). Water depth in wetlands is known to be directly related to the establishment and survival of different macrophyte types (Rolon et al., 2010), which are very important for zooplankton as a refuge, substrate, and food source, resulting in distinct zooplankton communities (Nevalainen, 2012; Adamczuk, 2014). The positive relation between LCBD and sediment depth might be related to some taxa being present in the riverine papyrus swamps wetlands with deep sediments, such as several bottom-dwelling Cladoceran species (Gogoi et al., 2018).
Finally, the effect of wetland type was more prominent than the effect of season on environmental variables, plankton communities, and RFGs. However, because only one-time sampling per season was used, the insignificance of the seasonal effect could be attributed to our under-sampling efforts.
Conclusion
Understanding local taxon richness, as well as variation in community composition, in combination with ecological uniqueness analysis (LCBD), could aid in the wetland restoration and conservation activities. The observed significant variation in plankton community composition among the three wetland types suggests that all the wetlands included in this study must be conserved, which may be difficult due to limited resources. Therefore, wetlands with a high plankton local taxon richness and a high LCBD (high ecological uniqueness) are worth special attention. Thus, conserving riverine papyrus swamps is critical for preserving the majority of phytoplankton and zooplankton taxa, as well as the most valuable sites. However, the taxa poor, degraded Megech river mouth wetland, which contributed significantly to zooplankton LCBD, requires restoration. Although we believe our findings can serve as a foundation for any conservation and restoration plans, we recommend more research is needed on the diversity, and community composition of other organisms in these wetlands to make better decisions and preserve the Lake Tana regional biodiversity.
Data Availability Statement
The original contributions presented in the study are included in the article/Supplementary Material, further inquiries can be directed to the corresponding author.
Author Contributions
AK contributed to data collection, analysis, and manuscript writing. AK, AW, IS, and PL designed the study and developed a data collection protocol. EV, IS, LT, and PL contributed to the critical revision of the manuscript for important intellectual content. EA, LDM, and MK contributed to the final version of the manuscript.
Funding
This study was financially supported by VLIR-UOS (ET2017IUC036A103).
Conflict of Interest
The authors declare that the research was conducted in the absence of any commercial or financial relationships that could be construed as a potential conflict of interest.
Publisher’s Note
All claims expressed in this article are solely those of the authors and do not necessarily represent those of their affiliated organizations, or those of the publisher, the editors and the reviewers. Any product that may be evaluated in this article, or claim that may be made by its manufacturer, is not guaranteed or endorsed by the publisher.
Acknowledgments
The authors are grateful to Vlaamse Interuniversitaire Raad–Universitaire Ontwikkelingssamenwerking (VLIR-UOS) for financing this study. The first author was a recipient of an IUC (Institutional University Cooperation) Ph.D. scholarship from VLIR (ET2017IUC036A103) to carry out this work. Vrije Universiteit Brussel (VUB-BAS42) and Bahir Dar University are thanked for their financial and logistic support. We are grateful to Demeke Kifle of Addis Ababa University for his help in phytoplankton identification and for allowing us to work in his lab. Jacobus Vijverberg of the Netherlands Institute of Ecology (NIOO-KNAW) deserves special thanks for the zooplankton (the species Cladocerans and Copepods) identification. Two reviewers are thanked for their insightful comments on a previous version of the manuscript.
Supplementary Material
The Supplementary Material for this article can be found online at: https://www.frontiersin.org/articles/10.3389/fenvs.2022.816892/full#supplementary-material
References
Abate, M., Nyssen, J., Moges, M. M., Enku, T., Zimale, F. A., Tilahun, S. A., et al. (2017). Long‐Term Landscape Changes in the Lake Tana Basin as Evidenced by Delta Development and Floodplain Aggradation in Ethiopia. Land Degrad. Develop. 28, 1820–1830. doi:10.1002/ldr.2648
Abebe, W. B., and Minale, A. S. (2017). “Land Use and Watershed Management Practices in Lake Tana Basin,” in Social and Ecological System Dynamics (Springer), 479–521.
Abebe, W. B., Tilahun, S. A., Moges, M. M., Wondie, A., Derseh, M. G., Nigatu, T. A., et al. (2020). Hydrological Foundation as a Basis for a Holistic Environmental Flow Assessment of Tropical Highland Rivers in Ethiopia. Water 12, 547. doi:10.3390/w12020547
Abera, A., Verhoest, N. E. C., Tilahun, S., Inyang, H., and Nyssen, J. (2021). Assessment of Irrigation Expansion and Implications for Water Resources by Using RS and GIS Techniques in the Lake Tana Basin of Ethiopia. Environ. Monit. Assess. 193, 1–17. doi:10.1007/s10661-020-08778-1
Adamczuk, M. (2014). Niche Separation by Littoral-Benthic Chydoridae (Cladocera, Crustacea) in a Deep lake-potential Drivers of Their Distribution and Role in Littoral-Pelagic Coupling. J. Limnol. 73, 490–501. doi:10.4081/jlimnol.2014.884
Adebayo, A. T., Adewole, H. A., Akindele, E. O., and Olaleye, V. F. (2021). Planktonic flora and Fauna of Opa Reservoir Wetlands, Obafemi Awolowo University, Ile-Ife, Nigeria. J. Basic Appl. Zool. 82, 1–10. doi:10.1186/s41936-021-00237-8
Alemayehu, T., McCartney, M., and Kebede, S. (2010). The Water Resource Implications of Planned Development in the Lake Tana Catchment, Ethiopia. Ecohydrology & Hydrobiology 10, 211–221. doi:10.2478/v10104-011-0023-6
Allende, L., Tell, G., Zagarese, H., Torremorell, A., Pérez, G., Bustingorry, J., et al. (2009). Phytoplankton and Primary Production in clear-vegetated, Inorganic-Turbid, and Algal-Turbid Shallow Lakes from the pampa plain (Argentina). Hydrobiologia 624, 45–60. doi:10.1007/s10750-008-9665-9
Alvarez-mieles, G., Irvine, K., GriensvenVan, A. V., Arias-hidalgo, M., Arias-Hidalgo, A., and Mynett, A. E. (2013). Relationships between Aquatic Biotic Communities and Water Quality in a Tropical River-Wetland System (Ecuador). Environ. Sci. Pol. 34, 115–127. doi:10.1016/j.envsci.2013.01.011
American Public Health Association (2005). “Standard Methods for the Examination of Water and Wastewater,” in APHA-AWWA-WEF. Editor D. C. Washington. 21st ed.
Anteneh, W., Dejen, E., and Getahun, A. (2012). Shesher and Welala Floodplain Wetlands (Lake Tana, Ethiopia): Are They Important Breeding Habitats forClarias Gariepinusand the MigratoryLabeobarbusFish Species? Scientific World J. 2012, 1–10. doi:10.1100/2012/298742
Asmare, T., Demissie, B., Nigusse, A. G., and GebreKidan, A. (2020). Detecting Spatiotemporal Expansion of Water Hyacinth (Eichhornia crassipes) in Lake Tana, Northern Ethiopia. J. Indian Soc. Remote Sens. 48, 751–764. doi:10.1007/s12524-020-01107-6
Aynalem, S., and Bekele, A. (2008). Species Composition, Relative Abundance and Distribution of Bird Fauna of Riverine and Wetland Habitats of Infranz and Yiganda at Southern Tip of Lake Tana, Ethiopia. Trop. Ecol. 49, 199.
Aynalem, S., Goshu, G., and Wondie, A. (2017). “Wetlands of the Lake Tana Watershed,” in Social and Ecological System Dynamics (Springer), 245–256. doi:10.1007/978-3-319-45755-0_16
Aziz, M. S. B., Hasan, N. A., Hasan, M. M. R., Alam, M. M., and Haque, M. M. (2021). Decline in Fish Species Diversity Due to Climatic and Anthropogenic Factors in Hakaluki Haor, an Ecologically Critical Wetland in Northeast Bangladesh. Heliyon 7, e05861. e05861. doi:10.1016/j.heliyon.2020.e05861
Bates, D., Maechler, M., Bolker, B., Walker, S., Christensen, R. H. B., Singmann, H., et al. (2011). Package ‘lme4.’ Linear Mix. Model. Using S4 Classes. R Packag. Version 1.
Bellinger, E. G., Sigee, D. C., Edward, B., and David, S. (2015). Freshwater Algae: Identification, Enumeration and Use as Bioindicators. John Wiley & Sons.
Bhat, N. A., Wanganeo, A., and Raina, R. (2015). Seasonal Dynamics of Phytoplankton Community in a Tropical Wetland. Environ. Monit. Assess. 187, 5–12. doi:10.1007/s10661-014-4136-4
Bicudo, D. C., Tremarin, P. I., Almeida, P. D., Zorzal-Almeida, S., Wengrat, S., Faustino, S. B., et al. (2016). Ecology and Distribution ofAulacoseiraspecies (Bacillariophyta) in Tropical Reservoirs from Brazil. Diatom Res. 31, 199–215. doi:10.1080/0269249X.2016.1227376
Bolduc, P., Bertolo, A., and Pinel-Alloul, B. (2016). Does Submerged Aquatic Vegetation Shape Zooplankton Community Structure and Functional Diversity? A Test with a Shallow Fluvial lake System. Hydrobiologia 778, 151–165. doi:10.1007/s10750-016-2663-4
Braak, C. J. F., and Schaffers, A. P. (2004). Co-correspondence Analysis: a New Ordination Method to Relate Two Community Compositions. Ecology 85, 834–846.
Brito, M. T., da, S., Heino, J., Pozzobom, U. M., and Landeiro, V. L. (2020). Ecological Uniqueness and Species Richness of Zooplankton in Subtropical Floodplain Lakes. Aquat. Sci. 82, 43. doi:10.1007/s00027-020-0715-3
Cardoso, S. J., Roland, F., Loverde-Oliveira, S. M., Huszar, V. L., and de, M. (2012). Phytoplankton Abundance, Biomass and Diversity within and between Pantanal Wetland Habitats. Limnologica 42, 235–241. doi:10.1016/j.limno.2012.01.002
Casali, S., Calijuri, M., do, C., Barbarisi, B., Renó, V. F., Affonso, A. G., et al. (2011). Impact of the 2009 Extreme Water Level Variation on Phytoplankton Community Structure in Lower Amazon Floodplain Lakes. Acta limnológica Bras. 23, 260–270.
Castillo, M. M. (2020). Suspended Sediment, Nutrients, and Chlorophyll in Tropical Floodplain Lakes with Different Patterns of Hydrological Connectivity. Limnologica 82, 125767. doi:10.1016/j.limno.2020.125767
Chaki, N., Reid, M., and Nielsen, D. L. (2021). Do temperature and Water Depth Influence Microcrustacean Hatching Responses from Floodplain Wetland Sediments? Mar. Freshw. Res. 72 (11), 1613. doi:10.1071/mf21022
Chakraborty, P., Acharyya, T., Raghunadh Babu, P. V., and Bandyopadhyay, D. (2011). Impact of Salinity and pH on Phytoplankton Communities in a Tropical Freshwater System: An Investigation with Pigment Analysis by HPLC. J. Environ. Monit. 13, 614–620. doi:10.1039/c0em00333f
Chandrasekharan, K. M., Subasinghe, C., and Haileslassie, A. (2021). Mapping Irrigated and Rainfed Agriculture in Ethiopia (2015-2016) Using Remote Sensing Methods. International Water Management Institute (IWMI).
Chaparro, G., Horváth, Z., O'Farrell, I., Ptacnik, R., and Hein, T. (2018). Plankton Metacommunities in Floodplain Wetlands under Contrasting Hydrological Conditions. Freshw. Biol. 63, 380–391. doi:10.1111/fwb.13076
Chaparro, G., O'Farrell, I., and Hein, T. (2019). Multi-scale Analysis of Functional Plankton Diversity in Floodplain Wetlands: Effects of River Regulation. Sci. Total Environ. 667, 338–347. doi:10.1016/j.scitotenv.2019.02.147
Chaparro-Herrera, D., Fuentes-García, R., Hernández-Quiroz, M., Valiente-Riveros, E., Hjort-Colunga, E., and Ponce de Leon-Hill, C. (2021). Comprehensive Health Evaluation of an Urban Wetland Using Quality Indices and Decision Trees. Environ. Monit. Assess. 193, 183. doi:10.1007/s10661-021-08939-w
Chatterjee, A., and Bhattacharyya, S. (2021). Assessing the Threats Facing Wetland Mammals in India Using an Evidence‐based Conservation Approach. Mam Rev. 51, 385–401. doi:10.1111/mam.12242
Chawaka, S. N., Boets, P., Goethals, P. L. M., and Mereta, S. T. (2018a). Does the protection Status of Wetlands Safeguard Diversity of Macroinvertebrates and Birds in Southwestern Ethiopia? Biol. Conservation 226, 63–71. doi:10.1016/j.biocon.2018.07.021
Chawaka, S. N., Boets, P., Mereta, S. T., Ho, L. T., and Goethals, P. L. M. (2018b). Using Macroinvertebrates and Birds to Assess the Environmental Status of Wetlands across Different Climatic Zones in Southwestern Ethiopia. Wetlands 38, 653–665. doi:10.1007/s13157-018-1008-7
Choi, J.-Y., Kim, S.-K., Jeong, K. S., and Joo, G.-J. (2015). Detecting Response Patterns of Zooplankton to Environmental Parameters in Shallow Freshwater Wetlands: Discovery of the Role of Macrophytes as Microhabitat for Epiphytic Zooplankton. J. Ecol. Env. 38, 1–11. doi:10.5141/ecoenv.2015.015
CoreTeam, R. (2020). R: A Language and Environment for Statistical Computing.R Foundation for Statistical Computing. Vienna, Austria. Available at: https://www.R-project.org/.
da Silva Brito, M. T., Heino, J., Pozzobom, U. M., and Landeiro, V. L. (2020). Ecological Uniqueness and Species Richness of Zooplankton in Subtropical Floodplain Lakes. Aquat. Sci. 82, 1–13. doi:10.1007/s00027-020-0715-3
D’Ambrosio, D. S., Claps, M. C., and García, A. (2016). Zooplankton Diversity of a Protected and Vulnerable Wetland System in Southern South America (Llancanelo Area, Argentina). Int. Aquat. Res. 8, 65–80.
De Groot, R., Brander, L., Van Der Ploeg, S., Costanza, R., Bernard, F., Braat, L., et al. (2012). Global Estimates of the Value of Ecosystems and Their Services in Monetary Units. Ecosystem Serv. 1, 50–61. doi:10.1016/j.ecoser.2012.07.005
Defaye, D. (1988). Contribution à la connaissance des Crustacés Copépodes d'Ethiopie. Hydrobiologia 164, 103–147. doi:10.1007/bf00008454
Dejen, E., Anteneh, W., and Vijverberg, J. (2017). The Decline of the Lake Tana (Ethiopia) Fisheries: Causes and Possible Solutions. Land Degrad. Develop. 28, 1842–1851. doi:10.1002/ldr.2730
Dejen, E., Vijverberg, J., Nagelkerke, L. A. J., and Sibbing, F. A. (2004). Temporal and Spatial Distribution of Microcrustacean Zooplankton in Relation to Turbidity and Other Environmental Factors in a Large Tropical lake (L. Tana, Ethiopia). Hydrobiologia 513, 39–49. doi:10.1023/b:hydr.0000018163.60503.b8
Denys, L., Muylaert, K., Krammer, K., Joosten, T., Reid, M., and Rioual, P. (2003). Aulacoseira Subborealis Stat. Nov. (Bacillariophyceae): a Common but Neglected Plankton Diatom. nova_hedwigia 77, 407–427. doi:10.1127/0029-5035/2003/0077-0407
Dilnessa, G. (2016). Spatial and Temporal Phytoplankton Species Diversity in Southern Gulf of Lake Tana, Northwestern Ethiopia. Int. J. Biodivers. Conserv. 8, 224–232. doi:10.5897/IJBC2016.1000
Dray, S., Bauman, D., Guillaume, B., Borcard, D., Clappe, S., and Guenard, G. (2018). Adespatial: Multivariate Multiscale Spatial Analysis.R Package Version 0.3-14. Available at: https://CRAN.R-project.org/package=adespatial.
Everard, M., Kangabam, R., Tiwari, M. K., McInnes, R., Kumar, R., Talukdar, G. H., et al. (2019). Ecosystem Service Assessment of Selected Wetlands of Kolkata and the Indian Gangetic Delta: Multi-Beneficial Systems under Differentiated Management Stress. Wetlands Ecol. Manage. 27, 405–426. doi:10.1007/s11273-019-09668-1
Feng, J., Liang, J., Li, Q., Zhang, X., Yue, Y., and Gao, J. (2021). Effect of Hydrological Connectivity on Soil Carbon Storage in the Yellow River Delta Wetlands of China. Chin. Geogr. Sci. 31, 197–208. doi:10.1007/s11769-021-1185-9
Fernando, C. H. (1980). The Species and Size Composition of Tropical Freshwater Zooplankton with Special Reference to the oriental Region (South East Asia). Int. Revue Ges. Hydrobiol. Hydrogr. 65, 411–426. doi:10.1002/iroh.19800650310
Fetahi, T., Mengistou, S., and Schagerl, M. (2011). Zooplankton Community Structure and Ecology of the Tropical-highland Lake Hayq, Ethiopia. Limnologica 41, 389–397. doi:10.1016/j.limno.2011.06.002
Fetene, Z. A., Weldegerima, T. M., Zeleke, T. T., and Nigussie, M. (2018). Harmonic Analysis of Precipitation Time Series in Lake Tana Basin, Ethiopia. Adv. Meteorology 2018, 1–9. doi:10.1155/2018/1598195
García-Chicote, J., Armengol, X., and Rojo, C. (2019). Zooplankton Species as Indicators of Trophic State in Reservoirs from Mediterranean River Basins. Inland Waters 9, 113–123. doi:10.1080/20442041.2018.1519352
Gebrehiwot, M., Kifle, D., Stiers, I., Triest, L., and Triest, L. (2017b). Phytoplankton Functional Dynamics in a Shallow Polymictic Tropical lake: the Influence of Emergent Macrophytes. Hydrobiologia 797, 69–86. doi:10.1007/s10750-017-3161-z
Gebrehiwot, M., Kifle, D., and Triest, L. (2017a). Emergent Macrophytes Support Zooplankton in a Shallow Tropical Lake: A Basis for Wetland Conservation. Environ. Manage. 60, 1127–1138. doi:10.1007/s00267-017-0935-z
Getnet, H., Mengistou, S., and Warkineh, B. (2021). Macroinvertebrate Community Structure and Diversity in Relation to Environmental Factors in Wetlands of the Lower Gilgel Abay River Catchment, Ethiopia. Afr. J. Aquat. Sci. 46, 1–13. doi:10.2989/16085914.2021.1946383
Gezie, A., Anteneh, W., Dejen, E., and Mereta, S. T. (2017). Effects of Human-Induced Environmental Changes on Benthic Macroinvertebrate Assemblages of Wetlands in Lake Tana Watershed, Northwest Ethiopia. Environ. Monit. Assess. 189, 152. doi:10.1007/s10661-017-5853-2
Gezie, A., Mulat, W. L., Anteneh, W., Dejen, E., Kloos, H., and Mereta, S. T. (2019). Habitat Suitability Modelling of Benthic Macroinvertebrate Community in Wetlands of Lake Tana Watershed, Northwest Ethiopia. Wetlands 40, 1–12. doi:10.1007/s13157-019-01231-1
Gogoi, B., Sousa, F. D. R., and Das, D. N. (2018). Faunal Diversity of Cladocera (Crustacea: Branchiopoda) with Notes on Biogeographically Important Species in the Floodplain Wetlands of the Subansiri River basin, India. Ann. Limnol. - Int. J. Lim. 54, 36. doi:10.1051/limn/2018022
Gogoi, P., Sinha, A., Das Sarkar, S., Chanu, T. N., Yadav, A. K., Koushlesh, S. K., et al. (2019). Seasonal Influence of Physicochemical Parameters on Phytoplankton Diversity and Assemblage Pattern in Kailash Khal, a Tropical Wetland, Sundarbans, India. Appl. Water Sci. 9, 156. doi:10.1007/s13201-019-1034-5
Gownaris, N. J., Rountos, K. J., Kaufman, L., Kolding, J., Lwiza, K. M. M., and Pikitch, E. K. (2018). Water Level Fluctuations and the Ecosystem Functioning of Lakes. J. Great Lakes Res. 44, 1154–1163. doi:10.1016/j.jglr.2018.08.005
Green, J. (1994). The Temperate-Tropical Gradient of Planktonic Protozoa and Rotifera. Hydrobiologia 272, 13–26. doi:10.1007/bf00006509
Haileselasie, T. H., Teferi, M., Dejenie, T., Gerlass, K., Abay, T., Hiluf, S., et al. (2012). Abundance, Species Composition and Spatial Distribution of Zooplankton in Lake Hashengie of Tigray, Northern Ethiopia. Curr. Res. J. Biol. Sci. 4, 389–393. doi:10.5897/ijbc11.274
Hötzel, G., and Croome, R. (1999). A Phytoplankton Methods Manual for Australian Freshwaters. LWRRDC Occasional Paper 22/99. L. Water Resour. Res. Dev. Corp.
Hunegnaw, G., Mengesha, H., Aimro, A., and Ferede, B. (2013). Wetlands Ecosystems Coverage, Status and Threats in the Abbay River Basin. Addis Ababa.
Idris, B. A. G. (1983). Freshwater Zooplankton of Malaysia (Crustacea: Cladocera). Universiti Pertanian Malaysia Press.
Imoobe, T. O. T., and Akoma, O. C. (2008). Assessment of Zooplankton Community Structure of the Bahir Dar Gulf of Lake Tana, Ethiopia. Ethiop. J. Environ. Stud. Manag. 1, 26–34. doi:10.4314/ejesm.v1i2.41577
Jemberie, M. A., Awass, A. A., Melesse, A. M., Ayele, G. T., and Demissie, S. S. (2015). Seasonal Rainfall-Runoff Variability Analysis, Lake Tana Sub-Basin, Upper Blue Nile Basin, Ethiopia. Ethiopia,” in, 341–363. doi:10.1007/978-3-319-18787-7_17
Kalmbach, E. (2017). “Establishment of the Lake Tana Biosphere reserve within the UNESCO World Network of Biosphere Reserves,” in Social and Ecological System Dynamics (Springer), 523–544.
Kassambara, A. (2020). Ggpubr: “Ggplot2” Based Publication Ready Plots.R Package Version 0.4.0.999. Available at: https://rpkgs.datanovia.com/ggpubr/.
Kebede, S., Travi, Y., Alemayehu, T., and Marc, V. (2006). Water Balance of Lake Tana and its Sensitivity to Fluctuations in Rainfall, Blue Nile basin, Ethiopia. J. Hydrol. 316, 233–247. doi:10.1016/j.jhydrol.2005.05.011
Keddy, P. A., Fraser, L. H., Solomeshch, A. I., Junk, W. J., Campbell, D. R., Arroyo, M. T. K., et al. (2009). Wet and Wonderful: The World's Largest Wetlands Are Conservation Priorities. Bioscience 59, 39–51. doi:10.1525/bio.2009.59.1.8
Koste, W., and Shiel, R. J. (1989). Rotifera from Australian Inland Waters. Ill. Euchlanidae, Mytilinidae and Trichotriidae (Rotifera: Monogononta). Trans. R. Soc. S. Aust. 113, 85–114.
Koste, W., and Shiel, R. (1987). Rotifera from Australian Inland Waters. II. Epiphanidae and Brachionidae (Rotifera : Monogononta). Invert. Syst. 1, 949–1021. doi:10.1071/it9870949
Kuznetsova, A., Brockhoff, P. B., and Christensen, R. H. B. (2017). lmerTest Package: Tests in Linear Mixed Effects Models. J. Stat. Softw. 82, 1–26. doi:10.18637/jss.v082.i13
Legendre, P., and De Cáceres, M. (2013). Beta Diversity as the Variance of Community Data: Dissimilarity Coefficients and Partitioning. Ecol. Lett. 16, 951–963. doi:10.1111/ele.12141
Legendre, P., and Gallagher, E. D. (2001). Ecologically Meaningful Transformations for Ordination of Species Data. Oecologia 129, 271–280. doi:10.1007/s004420100716
Leira, M., López-rodríguez, M. C., and Carballeira, R. (2017). Epilithic Diatoms ( Bacillariophyceae ) from Running Waters in NW Iberian Peninsula ( Galicia, Spain ). Del. Jard. Bot. Madrid 74, 1–24. doi:10.3989/ajbm.2421
Lemma, H., Frankl, A., Dessie, M., Poesen, J., Adgo, E., and Nyssen, J. (2020). Consolidated Sediment Budget of Lake Tana, Ethiopia (2012-2016). Geomorphology 371, 107434. doi:10.1016/j.geomorph.2020.107434
Loverde-Oliveira, S. M., Huszar, V. L. M., Mazzeo, N., and Scheffer, M. (2009). Hydrology-driven Regime Shifts in a Shallow Tropical lake. Ecosystems 12, 807–819. doi:10.1007/s10021-009-9258-0
Lu, Q., Liu, X., Qiu, X., Liang, T., Chen, J., Zhao, S., et al. (2021). Changes and Drivers of Zooplankton Diversity Patterns in the Middle Reach of Yangtze River Floodplain Lakes, China. Ecol. Evol 11, 17885–17900. doi:10.1002/ece3.8353
Ma, C., Mwagona, P. C., Yu, H., Sun, X., Liang, L., Mahboob, S., et al. (2019). Seasonal Dynamics of Zooplankton Functional Group and its Relationship with Physico-Chemical Variables in High Turbid Nutrient-Rich Small Xingkai Wetland Lake, Northeast China. J. Freshw. Ecol. 34, 65–79. doi:10.1080/02705060.2018.1443847
Mack, H. R., Conroy, J. D., Blocksom, K. A., Stein, R. A., and Ludsin, S. A. (2012). A Comparative Analysis of Zooplankton Field Collection and Sample Enumeration Methods. Limnol. Oceanogr. Methods 10, 41–53. doi:10.4319/lom.2012.10.41
Melaku, A. (2017). Water Quality and Phytoplankton Community Structure in the Southern Gulf of Lake Tana.MSc Thesis.Ethiopia. Addis Ababa University.
Menbere, I. P., and Menbere, T. P. (2018). Wetland Ecosystems in Ethiopia and Their Implications in Ecotourism and Biodiversity Conservation. J. Ecol. Nat. Environ. 10, 80–96.
Mengistu, W. (2018). A First Directory of Ethiopian Wetlands :Descriptions, Ecosystem Services, Causes of Degradation & Recommendations for Restoration and Sustainability.
Mereta, S. T., Boets, P., De Meester, L., and Goethals, P. L. M. (2013). Development of a Multimetric index Based on Benthic Macroinvertebrates for the Assessment of Natural Wetlands in Southwest Ethiopia. Ecol. Indicators 29, 510–521. doi:10.1016/j.ecolind.2013.01.026
Merga, L., Mengistie, A., Faber, J., and Van den Brink, P. (2020). Trends in Chemical Pollution and Ecological Status of Lake Ziway, Ethiopia: a Review Focussing on Nutrients, Metals and Pesticides. Afr. J. Aquat. Sci. 45, 386–400. doi:10.2989/16085914.2020.1735987
Mucheye, T., Yitaferu, B., and Zenebe, A. (2018). Significance of Wetlands for Sediment and Nutrient Reduction in Lake Tana Sub-Basin, Upper Blue Nile Basin, Ethiopia. Sustain. Water Resour. Manag. 4, 567–572. doi:10.1007/s40899-017-0140-5
Nagel, L. D., McNulty, S. A., Schlesinger, M. D., and Gibbs, J. P. (2021). Species Composition, Relative Abundance and Distribution of Bird Fauna of Riverine and Wetland Habitats of Infranz and Yiganda at Southern Tip of Lake Tana. Wetlands 41, 1–11. doi:10.1007/s13157-021-01404-x
Nakagawa, S., and Schielzeth, H. (2013). A General and Simple Method for obtainingR2from Generalized Linear Mixed-Effects Models. Methods Ecol. Evol. 4, 133–142. doi:10.1111/j.2041-210x.2012.00261.x
Napiórkowski, P., Bąkowska, M., Mrozińska, N., Szymańska, M., Kolarova, N., and Obolewski, K. (2019). The Effect of Hydrological Connectivity on the Zooplankton Structure in Floodplain Lakes of a Regulated Large River (The Lower Vistula, Poland). Water 11, 1924. doi:10.3390/w11091924
Ndebele-Murisa, M. R., Musil, C. F., and Raitt, L. (2010). A Review of Phytoplankton Dynamics in Tropical African Lakes. S. Afr. J. Sci. 106, 13–18. doi:10.4102/sajs.v106i1/2.64
Nevalainen, L. (2012). Distribution of Benthic Microcrustaceans along a Water Depth Gradient in an Austrian Alpine lake - Sedimentary Evidence for Niche Separation. Limnologica 42, 65–71. doi:10.1016/j.limno.2011.08.003
Padisák, J., Crossetti, L. O., and Naselli-Flores, L. (2009). Use and Misuse in the Application of the Phytoplankton Functional Classification: A Critical Review with Updates. Hydrobiologia 621, 1–19. doi:10.1007/s10750-008-9645-0
Petar, Ž., Marija, G. U., Koraljka, K. B., Marija, P.-M., Judit, P., Anđelka, P. M., et al. (2014). Morpho-functional Classifications of Phytoplankton Assemblages of Two Deep Karstic Lakes. Hydrobiologia 740, 147–166. doi:10.1007/s10750-014-1950-1
Poppe, L., Frankl, A., Poesen, J., Admasu, T., Dessie, M., Adgo, E., et al. (2013). Geomorphology of the Lake Tana basin, Ethiopia. J. Maps 9, 431–437. doi:10.1080/17445647.2013.801000
Reynolds, C. S., Huszar, V., Kruk, C., Naselli-Flores, L., and Melo, S. (2002). Towards a Functional Classification of the Freshwater Phytoplankton. J. Plankton Res. 24, 417–428. doi:10.1093/plankt/24.5.417
Rideout, N. K., Compson, Z. G., Monk, W. A., Bruce, M. R., and Baird, D. J. (2021). The Beautiful and the Dammed: Defining Multi-Stressor Disturbance Regimes in an Atlantic River Floodplain Wetland. Front. Ecol. Evol. 9, 400. doi:10.3389/fevo.2021.553094
Rodríguez, P., Vera, M. S., and Pizarro, H. (2012). Primary Production of Phytoplankton and Periphyton in Two Humic Lakes of a South American Wetland. Limnology 13, 281–287. doi:10.1007/s10201-012-0373-9
Rojo, C., Mesquita-Joanes, F., Monros, J. S., Armengol, J., Sasa, M., Bonilla, F., et al. (2016). Hydrology Affects Environmental and Spatial Structuring of Microalgal Metacommunities in Tropical Pacific Coast Wetlands. PLoS One 11, e0149505. doi:10.1371/journal.pone.0149505
Rolon, A. S., Homem, H. F., and Maltchik, L. (2010). Aquatic Macrophytes in Natural and Managed Wetlands of Rio Grande Do Sul State, Southern Brazil. Acta Limnol. Bras. (Online) 22, 133–146. doi:10.1590/s2179-975x2010000200003
Segers, H., and De Smet, W. H. (2007). Diversity and endemism in Rotifera: a review, and Keratella Bory de St Vincent. Protist Divers. Geogr. Distrib., 69–82. doi:10.1007/978-90-481-2801-3_6
Sharma, R., and Singh, S. (2018). Water Quality and Phytoplankton Diversity of High-Altitude Wetland, Dodi Tal of Garhwal Himalaya, India. Biodivers. Int. J. 2, 4493–4894. doi:10.15406/bij.2018.02.00103
Shatwell, T., Adrian, R., and Kirillin, G. (2016). Planktonic Events May Cause Polymictic-Dimictic Regime Shifts in Temperate Lakes. Sci. Rep. 6, 24361–24414. doi:10.1038/srep24361
Shen, R., Ren, H., Yu, P., You, Q., Pang, W., and Wang, Q. (2018). Benthic Diatoms of the Ying River (Huaihe River basin, China) and Their Application in Water Trophic Status Assessment. Water 10, 1013. doi:10.3390/w10081013
Sinev, A. Y. (2016). Key for Identification of Cladocera of the Subfamily Aloninae (Anomopoda: Chydoridae) from South-East Asia. Zootaxa 4200, 451–486. doi:10.11646/zootaxa.4200.4.1
Stave, K., Goshu, G., Aynalem, S., Kopainsky, B., Goshu, G., Aynalem, S., et al. (2017). Social and Ecological System Dynamics. Springer. doi:10.1007/978-3-319-45755-0
Stević, F., Mihaljević, M., and Špoljarić, D. (2013). Changes of Phytoplankton Functional Groups in a Floodplain lake Associated with Hydrological Perturbations. Hydrobiologia 709, 143–158.
Sultana Laskar, H., and Gupta, S. (2013). Phytoplankton Community and Limnology of Chatla Floodplain Wetland of Barak valley, Assam, North-East India. Knowl. Managt. Aquat. Ecosyst., 06–14. doi:10.1051/kmae/2013073
Taylor, J. C., Harding, W. R., and Archibald, C. G. M. (2007). An Illustrated Guide to Some Common Diatom Species from South Africa. Water Research Commission Pretoria.
Tewabe, D., and Fentahun, T. (2020). Assessing Land Use and Land Cover Change Detection Using Remote Sensing in the Lake Tana Basin, Northwest Ethiopia. Cogent Environ. Sci. 6, 1778998. doi:10.1080/23311843.2020.1778998
Tilman, D., Kiesling, R., Sterner, R., Kilham, S. S., and Johnson, F. (1986). Green, Bluegreen and Diatom Algae: Taxonomic Differences in Competitive Ability for Phosphorus, Silicon and Nitrogen. Arch. Hydrobiol. 106, 473–485. Available at: http://www.cedarcreek.umn.edu/biblio/fulltext/t1199.pdf.
Tockner, K., Malard, F., and Ward, J. V. (2000). An Extension of the Flood Pulse Concept. Hydrol. Process. 14, 2861–2883. doi:10.1002/1099-1085(200011/12)14:16/17<2861::aid-hyp124>3.0.co;2-f
Van de Velde, I. (1984). Revision of the African Species of the Genus Mesocyclops Sars, 1914 (Copepoda: Cyclopidae). Hydrobiologia 109, 3–66. doi:10.1007/bf00006297
Vijverberg, J., Sibbing, F. A., and Dejen, E. (2009). “Lake Tana: Source of the Blue Nile, Limnology and Human Use,” in, ed. H. J. Dumont (Dordrecht: Springer Netherlands), 163–192. doi:10.1007/978-1-4020-9726-3_9
Wetzel, R. G., and Likens, G. E. (2013). Limnological Analyses. New York, NY: Springer Science & Business Media. doi:10.1007/978-1-4757-4098-1
Wickham, H. (2016). ggplot2: Elegant Graphics for Data Analysis.Springer-Verlag New York. Available at: https://ggplot2.tidyverse.org.
Widdicombe, C. E., Eloire, D., Harbour, D., Harris, R. P., and Somerfield, P. J. (2010). Long-term Phytoplankton Community Dynamics in the Western English Channel. J. Plankton Res. 32, 643–655. doi:10.1093/plankt/fbp127
Wijeyaratne, W. M. D. N., and Nanayakkara, D. B. M. (2020). Monitoring of Water Quality Variation Trends in a Tropical Urban Wetland System Located within a Ramsar Wetland City: A GIS and Phytoplankton Based Assessment. Environ. Nanotechnology, Monit. Manage. 14, 100323. doi:10.1016/j.enmm.2020.100323
Wondie, A. (2018). Ecological Conditions and Ecosystem Services of Wetlands in the Lake Tana Area, Ethiopia. Ecohydrology & Hydrobiology 18, 231–244. doi:10.1016/j.ecohyd.2018.02.002
Wudneh, T. (1998). Biology and Management of Fish Stocks in Ba- Har Dar Gulf, Lake Tana. Netherlands: Ethiopia. PhD Thesis, Wageningen Agric. Univ. Wageningen.
Xiao, R., Wang, Q., Zhang, M., Pan, W., and Wang, J. J. (2020). Plankton Distribution Patterns and the Relationship with Environmental Gradients and Hydrological Connectivity of Wetlands in the Yellow River Delta. Ecohydrology & Hydrobiology 20, 584–596. doi:10.1016/j.ecohyd.2020.01.002
Yin, L., Ji, Y., Zhang, Y., Chong, L., and Chen, L. (2018). Rotifer Community Structure and its Response to Environmental Factors in the Backshore Wetland of Expo Garden, Shanghai. Aquacult. Fish. 3, 90–97. doi:10.1016/j.aaf.2017.11.001
Zeileis, A., Cribari-Neto, F., Grün, B., and Kos-midis, I. (2010). Beta Regression in R. J. Stat. Softw. 34, 1–24. doi:10.18637/jss.v034.i01
Zelelew, S. A., and Archibald, G. (2021). Nest Characteristics and Morphometry of Black Crowned Cranes Balearica pavonina Ceciliae in Lake Tana Area Wetlands. Afr. Zool., 1–7. doi:10.1080/15627020.2020.1850350
Keywords: Lake Tana, Zooplankton, Phytoplankton, wetlands, community composition, species richness
Citation: Kahsay A, Lemmens P, Triest L, De Meester L, Kibret M, Verleyen E, Adgo E, Wondie A and Stiers I (2022) Plankton Diversity in Tropical Wetlands Under Different Hydrological Conditions (Lake Tana, Ethiopia). Front. Environ. Sci. 10:816892. doi: 10.3389/fenvs.2022.816892
Received: 17 November 2021; Accepted: 16 February 2022;
Published: 09 March 2022.
Edited by:
Albert Chakona, South African Institute for Aquatic Biodiversity, South AfricaReviewed by:
Andrea Funk, University of Natural Resources and Life Sciences Vienna, AustriaMarc Peipoch, Stroud Water Research Center, United States
Copyright © 2022 Kahsay, Lemmens, Triest, De Meester, Kibret, Verleyen, Adgo, Wondie and Stiers. This is an open-access article distributed under the terms of the Creative Commons Attribution License (CC BY). The use, distribution or reproduction in other forums is permitted, provided the original author(s) and the copyright owner(s) are credited and that the original publication in this journal is cited, in accordance with accepted academic practice. No use, distribution or reproduction is permitted which does not comply with these terms.
*Correspondence: Abrehet Kahsay, QWJyZWhldC5LYWhzYXkuTWVoYXJpQHZ1Yi5hYy5iZQ==