- 1Center for Watershed Sciences, University of California, Davis, Davis, CA, United States
- 2Department of Geography, University of New England, Armidale, NSW, Australia
While water resource managers and river scientists recognize the inherent interconnections among hydrology, river structure, biophysical processes and ecological patterns, management of environmental flows still pays insufficient attention to the ecological and geomorphological functionality of particular aspects of the flow regime. Implementation of more natural flow regimes has improved habitat conditions for native species in many moderately impaired rivers but mimicking a natural flow regime in heavily modified riverine landscapes cannot be expected to yield successful ecological outcomes unless such flows trigger functional processes. For example, the restoration of peak flows may not regenerate habitats if the river is starved of sediment or if the river channel is highly confined. High biodiversity is supported when variable flow regimes interact with spatially variable (heterogeneous) river channel and floodplain forms. In contrast, as rivers become homogeneous, biodiversity decreases when these dynamic spatiotemporal interactions are limited by flow alterations, blocked by channel levees, or perturbed by sediment deficit or surplus. Thus, the design of a more natural environmental flow regime without consideration of the implications for sediment transport and implicit recognition of channel–floodplain geomorphology is likely to have limited success in river management and restoration. To enhance the functionality of environmental flows, considerations of physical, biogeochemical, and ecological processes and the inherent heterogeneity of the riverine landscape must be included. A Functional Flows approach enhances the benefits from limited environmental flow allocations by focusing on the ecological and geomorphological functionality of particular aspects of the flow regime, considering geomorphic context, and emphasizing spatiotemporal diversity at key locations in the riverscape, such as adjacent floodplains or tributary junctions. In this paper, we outline and illustrate the concept of Functional Flows using a flow-chain model and provide two case study examples from Australia and the United States, where improvements in channel habitat and reconnection with the floodplain help to achieve the desired functionality of environmental flows.
1 Introduction
Alteration, impairment, and development of river systems for human use is ubiquitous (Lehner et al., 2011; Grill et al., 2019). The increased ability to harness river flows for agriculture, hydropower, industry, and domestic water supply has led to economic growth and prosperity. However, the impacts from the development of freshwater ecosystems have resulted in drastic reductions in freshwater biodiversity and ecosystem services (Vorosmarty et al., 2010; Reid et al., 2019). Recent global biodiversity initiatives explicitly recognize freshwater ecosystems as vital to human sustainability but also highly vulnerable. Thus, activities that target freshwater ecosystem processes, improve water quality, accelerate environmental flow implementation, and protect and restore critical habitats have increased exponentially over the last several decades (Tickner et al., 2020; van Rees et al., 2021). In many locations, actions to improve water quality for human consumption have been successful (e.g., Keiser and Shapiro, 2019), but actions aimed at improving freshwater biodiversity have been limited (Reid et al., 2019).
Environmental flows—the practice of allocating water in river systems for ecological purposes—is a strategy for supporting freshwater dependent ecosystems and improving river health (Horne et al., 2017). The philosophy and practice of what constitutes an environmental flow regime has advanced from prescriptions of static minimum instream flows to protect selected life history stages of aquatic species (e.g., Bovee, 1982) to environmental flow determinations that consider the natural variability of streamflow to which native species have evolved (e.g., Poff et al., 2010; Hall et al., 2011). Associated stream habitat restoration efforts have also changed from construction of an idealized ‘natural channel design’ (e.g., Rosgen, 1996) to emplacement of wood or engineered structures within the stream channel to promote local scour and deposition of sediment to create habitat diversity (e.g., Abbe et al., 2003). However, considerations of flow regimes alone have not always been effective in restoring stream health or increasing biodiversity (Grams et al., 2007). Similarly, considerations of just channel form or physical habitat structure have not always resulted in expected improvements to aquatic species diversity (Simon et al., 2007; Palmer et al., 2010). As a result, resource managers and river scientists have moved towards holistic environmental flow approaches that embrace the importance of physical and ecological processes in supporting riverine habitat and freshwater dependent ecosystems (cf. Beechie et al., 2010; Wohl et al., 2015; Yarnell et al., 2015).
Implementation of holistic environmental flow methods remains complex, especially in highly modified rivers of the Anthropocene (Poff and Matthews, 2013; Tickner et al., 2020). How do we effectively balance water provisions for ecosystem services with water extractions for human uses? The answer is often difficult and elusive to determine, resulting in most environmental flow approaches remaining focused on changes to the flow regime or improvements to physical habitat, rather than integrating these different fields of study. However, holistic approaches that focus on the functionality of flow, where water is prescribed in concert with physical conditions specifically to support discrete geomorphic and ecological processes within the riverscape that are known to support desired ecosystem services (Meitzen et al., 2013; Yarnell et al., 2015; Palmer and Ruhi, 2019), provide a path forward for maximizing benefits from water allocated to the environment.
Here, we provide a conceptual overview of flow functionality in riverine systems illustrated by a flow-chain model and demonstrate how the interactions of flow, sediment, and biophysical processes can be incorporated into environmental flow determinations and river restoration actions with two example case studies. When successful, holistic environmental flow programs can promote river resilience in the face of climate change, thus continuing to provide ecosystem services for societies into the future.
2 The functionality of flow in the riverine landscape
Rivers are diverse landscapes sustained by the interplay of biological, chemical, and physical processes that support high biodiversity and provide multiple ecosystem services for society (Fremier and Strickler, 2010; Gilvear et al., 2016). Identifying and understanding the various biophysical and social drivers, components, processes, and interrelated states of river systems is challenging; however, conceptual frameworks and models can aid in understanding these complex environments (Delong and Thoms, 2016). Flow chain models—a type of conceptual framework—demonstrate interactions between various components at multiple scales within complex adaptive systems (refer to Table 1 for a list of concepts and terms used here to describe Functional Flows in riverine landscapes). Riverine landscapes are complex adaptive systems by virtue of their hierarchical organization and ability to adjust multiple biophysical forms to an array of processes. Flow-chain models have been used to demonstrate the effect of change in physical heterogeneity on food webs in river ecosystems (Thoms et al., 2017) and the ecological concept of disturbance in urban river systems (Grimm et al., 2017). The flow chain of Dollar et al. (2007) is adapted here to provide a conceptual framework for Functional Flows in riverine landscapes.
Flow-chain models have several basic components representing the dynamic interplay of abiotic and biotic characteristics in riverine landscapes (Figure 1). Drivers are the main agents of change governing functions or a series of processes; templates are those states or forms upon which functions act; and, finally there are series of responders. Responders can be sets of organisms or parts of the biophysical environment present across the riverine landscape.
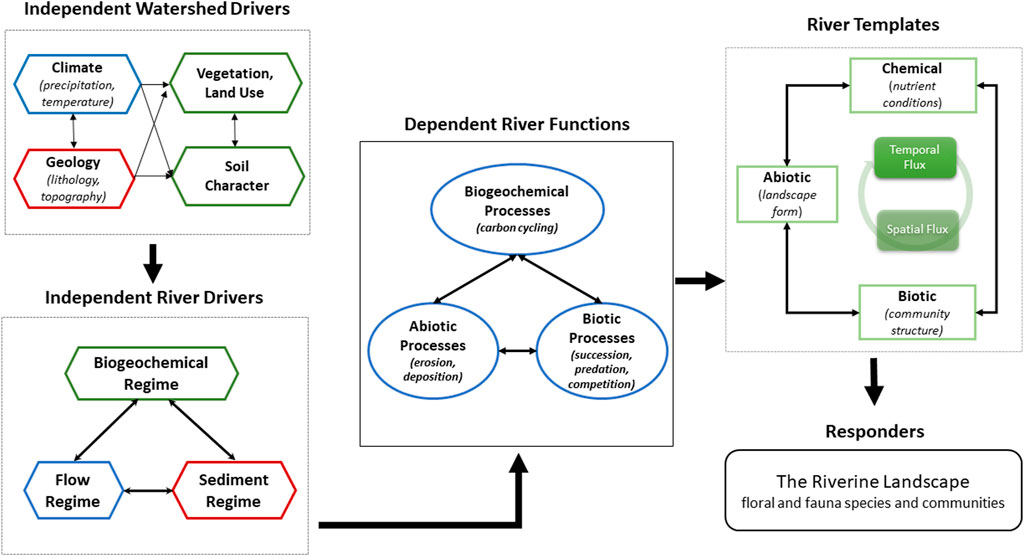
FIGURE 1. A conceptual flow-chain model for Functional Flows in riverine landscapes. The shape of each element reflects the nature of the element (e.g., Driver, Function, or Template), and the arrows indicate interactions between the elements.
The character (spatial heterogeneity) and dynamics (variability over time) of riverine landscapes are not only interrelated but also dependent upon variables operating at multiple scales creating a riverine landscape hierarchy (Frissell et al., 1986; Naiman et al., 2008). In the flow-chain model, first order independent variables such as climate, geology, soils, and vegetation of a watershed determine second order independent river variables such as the flow, sediment, and biogeochemical regimes (Figure 1). These independent and multi-scale variables interact through a series of feedback mechanisms that determine primary dependent river functions. In riverine landscapes, flow, sediment, and biogeochemical regimes are the main independent river drivers, or the primary agents of change, that act upon the dependent river functions, which in turn shape the templates of the riverine landscape—represented by the abiotic, biotic, and biogeochemical forms or states of the riverine landscape. The product of this interaction between the dependent river functions and the river templates is represented by the presence/absence and diversity of aquatic habitats distributed across the riverine landscape in time and space. The life history traits and diversity of plants and animals are responders to this dynamic product and ultimately dependent on watershed-scale and river-scale drivers.
River functions interact with the abiotic and biotic river templates over time and space creating a series of feedbacks within the river templates that modify ecosystem responses across the aquatic habitat mosaic of riverine landscapes. For example, erosion and deposition processes reshape the floodscape allowing for riparian succession processes to create a diverse dynamic vegetation community structure that riparian species respond to. Similarly, predation and competition are two key functions influencing life history traits of aquatic species in the riverscape such that diverse aquatic habitats and adaptation to specialized niches may confer protection from predators or opportunities for competitive advantage (Thoms et al., 2018). Without an understanding of the interplay between riverine functions, templates, and ecological responses, potential impacts of alterations to independent river drivers, like environmental flows, on ecological responses are likely to be uncertain or unexpected.
Holistic approaches to environmental flow management that consider and incorporate geomorphic and biogeochemical processes, as well as spatial and temporal dynamism, will potentially meet with greater success in increasing biodiversity and ecosystem services. In many cases, environmental flow programs fail to achieve expected results due to a singular focus on flow volumes or quantities, or conversely, a singular focus on habitat restoration under a ‘build it and they will come’ perspective (Horne et al., 2017). Lack of consideration of interactions between river drivers, such as the flow and sediment regimes, and functions, like erosion and deposition or lateral connectivity, can only yield limited results. The two case studies discussed here demonstrate the importance of incorporating a biophysical process understanding in developing environmental flows for riverine landscapes. They highlight in particular how an increased geomorphological understanding, as illustrated in our conceptual framework, improves the functionality of managed flow regimes within river landscapes.
3 Case study 1: Restoring geomorphic functionality in floodplains—The Murrumbidgee River and Yanga Floodplain, Australia
Floodplains are heterogeneous landscapes shaped by functions and processes operating at multiple scales. Heterogeneity is defined as spatial variation in the environment or landscape (Thoms et al., 2018). For floodplains, this is represented as the presence and arrangement of different geomorphological features across the abiotic template and associated ecosystem responses. Inundation and the subsequent wetting and drying of different floodplain geomorphological features is an important driver of floodplain ecosystems and the general biodiversity of these landscapes (Thoms, 2003). Hydrological connectivity, established during overbank flow, facilitates exchange of sediments, nutrients, carbon, and organisms between the river channel and adjacent floodplain surfaces (Thoms, 2003; Collins et al., 2005). Wetting also stimulates a multitude of ecosystem functions, like vegetation growth, primary production, and nutrient release, within the various floodplain geomorphological features. Variations in the pattern of inundation, as a result of floodplain topographic heterogeneity during overbank events, creates a dynamic floodplain habitat mosaic that further promotes an elevated floodplain biodiversity compared to the surrounding terrestrial landscape (Ward and Stanford, 1995; Ward et al., 1999; Amoros and Bornette, 2002; Thoms et al., 2006).
Promoting and sustaining landscape biodiversity is an emerging strategy for managing the novel and hybrid landscapes of the Anthropocene (Hobbs et al., 2014). This can be approached structurally by creating an array of physical habitat features across a landscape, and/or functionally via recognizing the interplay between structure, function, and complex feedbacks, as depicted in Figure 1. Functional diversity focuses on managing for process diversity in landscapes and ecosystems. This includes promoting diversity in the response of a landscape or within an ecosystem to management actions (Hulvey et al., 2013), such as the provision of water for floodplains. The idea of ‘Functional Flows’ represents an important long-term strategy for floodplains in the Anthropocene, and one that is cognizant of interactions between floodplain structure and function as well as the interplay between physical and ecological processes.
Heterogeneity is a feature of floodplain surfaces that influences diverse functional responses to flooding (Scown et al., 2016b). For example, floodplain inundation was shown by Thapa et al. (2016; 2020) to drive vegetation productivity responses through an adaptive cycle of wetting (r), wet (K), drying (Ω), and dry (α) phases. Adaptive cycles characterize a diversity of responses as a cycle comprised of four phases: exploitation (r), conservation (K), release (Ω), and renewal (α) (cf. Holling and Gunderson, 2002). The magnitude and duration of vegetation productivity responses depends not only on the duration of each phase of the adaptive cycle but also on the heterogeneity of the floodplain surface—i.e., the more heterogeneous the floodplain surface, the greater the response diversity of different vegetation communities (Thapa et al., 2016; Thapa et al., 2020). Currently, environmental flow allocations to floodplains largely ignore the importance of floodplain heterogeneity and its influence on the diversity of functional responses (Thoms et al., 2020). In this case study, we analyze data previously collected in the Lower Murrumbidgee River floodplain, Australia by Shilpakar (2013) and Scown et al. (2016a, 2016b) to illustrate the importance of floodplain heterogeneity in influencing the diversity of functional floodplain responses to hydrological connections (Figure 1) and highlight the need for understanding the interplay between physical drivers (e.g., flow regime), abiotic processes (e.g., erosion, lateral connectivity), abiotic templates (e.g., floodplain form), and the diversity of ecosystem responses.
3.1 The lower Murrumbidgee Floodplain
Many Australian river systems are set in unconstrained valleys with low gradients, and as such are dominated by extensive floodplains with surface areas up to >10,000 km2 (Thoms and Parsons, 2016). These floodplains are geomorphologically diverse, containing a suite of physical features, including billabongs (bodies of standing water on the floodplain), levees, scrolls, swales, distributary and anabranch channels, benches, palaeo-channels, cutoffs, and flat floodplain surfaces. This geomorphological diversity provides a physical template for a wide variety of animals and plants that underpin the basis of floodplains as “ecosystem control points” (cf. Bernhardt et al., 2017). Monetary values assigned to floodplain ecosystem services—those benefits that people obtain from intact ecosystems—illustrate their importance to society. In Australia, the floodplain landscapes of the Murray-Darling Basin provide an estimated $1.87 billion per annum for their various ecosystem services (Thoms, 2006).
The Yanga National Floodplain Park covers approximately 750 km2 of the lower Murrumbidgee River, SE Australia (Figure 2). The riverine landscape of the region is characteristic of the lowland river systems within the Murray-Darling Basin (Thoms and Sheldon, 2000). Dominated by a large unconfined floodplain (widths up to 40 km), Yanga National Floodplain Park has sedimentary and geomorphological features characteristic of C2 type floodplains (Nanson and Croke, 1992). The lateral instability of the main channel of the Murrumbidgee River has produced a mosaic of geomorphic features and a relatively complex floodplain surface topography. Index of Floodplain Surface Complexity (FSC) values (Scown et al., 2016a), calculated at a resolution of 100 m, range from 0.29 to 0.76 (FSC values range from 0 to 1) for different areas of the Yanga floodplain. FSC is a function of floodplain topographic (surface height and curvature) variance and its spatial organization across the floodplain surface (Scown et al., 2016a).
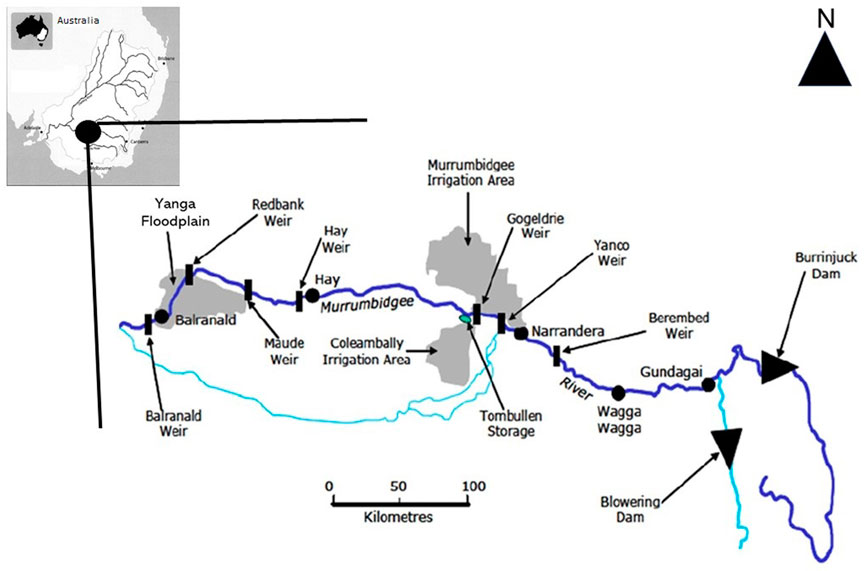
FIGURE 2. The Murrumbidgee River system and Yanga floodplain landscape within the Murray Darling Basin in southeast Australia. Main tributaries and anabranch channels shown in light blue. Significant water resource infrastructure and the Yanga floodplain (Lat: 34.6172°S; Long: 143.6392°E) are also shown.
Inundation of the Yanga floodplain is determined by flows in the Murrumbidgee River. The flow regime in the Murrumbidgee River at Maude gauging station just upstream of the Yanga floodplain is highly variable and unpredictable despite being controlled by several large headwater dams and low-level weirs constructed over 60 years ago for water supply. The long-term (1937–2005) median annual discharge is 16,806 m3 s−1, and annual discharges range from 1,767 to 75,598 m3 s−1 (NSW Office of Water, 2009). Water resource development has reduced the magnitude and frequency of those flood events with average return interval of up to 1 in 5 years by 30 percent (Wen et al., 2009). Inundation of the Yanga floodplain occurs when discharges exceed 232 m3 s−1 at the Maude gauging station (Wen et al., 2009), although management of water levels in the Redbank Weir pool just downstream of Yanga can induce inundations at lower discharges. Gated regulators on some anabranch channels can also control hydrological connections between the river channel and floodplain. Overall, the high variability and unpredictability in river flows in the Murrumbidgee River is inferred to result in a highly dynamic wetting and drying regime of the adjacent floodplain.
The Yanga floodplain landscape supports a complex mosaic of floodplain vegetation communities, which occur in discrete areas of the floodplain (Shilpakar et al., 2021). Dominant floodplain vegetation communities include river red gum (Eucalyptus camaldulensis) forest and woodlands, black box (Eucalyptus largiflorens) woodlands, lignum (Muelenbeckia florulenta) dominated shrubland, spikerush dominated sedgeland, sand hills with sparse vegetation, and dillon bush or salt bush shrubland. River red gum communities cover more than 200 km2 of the Yanga floodplain landscape. In addition, numerous swamps and open water lakes provide important habitat for a number of threatened species of water birds, such as the Australasian bittern (Botaurus poiciloptilus), black-tailed godwit (Limosa), blue-billed duck (Oxyura australis), and freckled duck (Stictonetta naevosa) (Hardwick and Maquire, 2012). This floodplain also supports internationally important migratory bird species including the Caspian tern (Sterna caspia), Latham’s snipe (Gallinago hardwickii), cattle egret (Ardea ibis), curlew sandpiper (Calidris ferruginea), red-necked stint (Calidris ruficollis), and the black-tailed godwit (Limosa) (Maher, 1990; Kingsford and Thomas, 2001; Hardwick and Maquire, 2012). The Yanga floodplain area also hosts the state’s largest known population of the highly endangered southern bell frog (Litoria raniformis) (Wassens et al., 2008).
3.2 Floodplain environmental flows
The Murray-Darling Basin Plan (2012; Australian Commonwealth) provides a coordinated approach to water use across the Murray Darling Basin that balances environmental, social, and economic considerations by setting water use to an environmentally sustainable level. Environmental water is that river water specifically set aside to restore, maintain, and improve the ecological health of rivers, floodplains, and wetlands. Australia’s Commonwealth Environmental Water Holder (CEWH) manages Commonwealth environmental water, which is one of the strategies by which the Australian Government seeks to achieve the Plan’s environmental objectives, of which, floodplain inundation and lateral connectivity are key outcomes.
Environmental water is managed along the lower Murrumbidgee floodplain via releases from headwater dams, water level management through a series of low-level weirs, and the control of regulators in anabranch channels. Headwater dams were originally designed for flood control, while low weirs were constructed as part of water security in drought periods, and in many places were built to serve irrigation districts (Figure 2). Both are now an integral part of downstream water resource management. Water level management at particular discharges in conjunction with the operation of a number of low-level weirs enables flows to be directed onto the floodplain. Controlled diversion flows onto the floodplain can occur at discharges less than bankfull channel capacity, i.e., less than 232 m3 s−1 at Maude Weir and 128–232 m3 s−1 at Redbank Weir (Figure 2). The sole focus of floodplain water management in the study area is to simply get water onto the floodplain rather than understanding the diversity of responses that may occur within the floodplain ecosystem.
3.3 Floodplain inundation
Inundation patterns across the Yanga floodplain have been studied by Shilpakar (2013) using a series of 34 cloud free satellite images to track the expansion and contraction of floodwaters. During three flood events that occurred in 1990, 1991, and 2005, the total inundated floodplain area and the number of distinct wet patches were determined for each remotely sensed image. This enabled the relationship between total wet area and number of wet patches for each flood event to be examined at two scales: the entire floodplain and for eight specific sub-areas of the floodplain with different FSC values. These eight floodplain areas correspond with distinct vegetation communities or vegetation patch types (Shilpakar et al., 2021). This allowed for not only the spatial character of floodplain inundation to be examined during individual flood events but also the association of this spatial character to the complexity of the floodplain surface.
Relationships between floodplain inundated area and the number of wet patches exhibited anticlockwise hysteresis for each flood event in Yanga National Floodplain Park (Shilpakar, 2013; Figure 3A). Thus, the number of wet patches for the same inundated area during the contraction of floodwaters or drying phase of each flood was greater than the expansion of floodwaters or wetting phase. For example, an inundated area of 10,000 ha associated with flood one was associated with >400 wet patches during the contraction or drying phase compared to <100 wet patches during the wetting or expansion phase (Figure 3A). This anticlockwise hysteresis relationship indicates fragmentation of the inundated floodplain during the contraction of floodwaters or drying of the floodplain landscape. Fragmentation of the inundated floodplain landscape promotes an enhanced heterogeneity of surface water resources with wet patches differing in size, shape, and duration of persistence (Shilpakar et al., Forthcoming 2022).
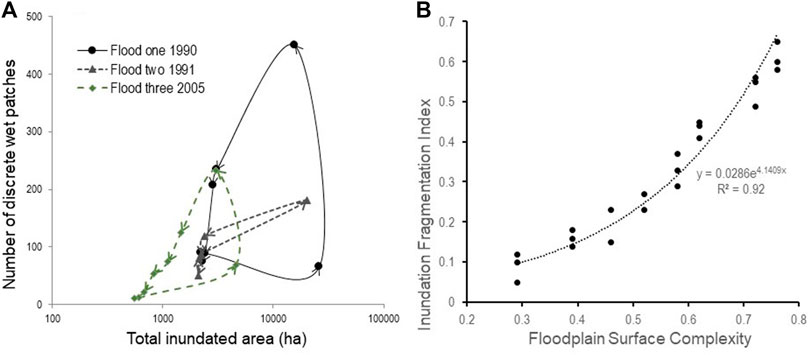
FIGURE 3. Inundation patterns for the Yanga Floodplain. (A) Relationship between total inundated floodplain area (hectares) and number of wet patches for the three flood events. Arrows indicate the consecutive images by image captured date (modified from Shilpakar, 2013). (B) Fragmentation of the inundated floodplain and floodplain surface complexity for the Yanga floodplain.
Similar inundation patterns were recorded among the different floodplain vegetation communities. At this smaller scale, three different relationships were observed between total inundated area and number of wet patches (Table 2). These were a simple linear relationship (L) between inundated area and the number of wet patches, an anti-clockwise (AC) hysteresis relationship, and a complex (Cx) relationship where there was an initial clockwise hysteresis followed by an anticlockwise hysteresis relationship. For the Cx relationship, wet patches were more abundant during the contraction of floodwaters from the floodplain. Overall, anti-clockwise hysteresis patterns were the dominant inundation pattern (n = 15 of the 22 vegetation community landscape inundation sequences), followed by complex patterns (n = 5), and then simple linear relationships (n = 2) (Table 2).
A strong positive linear relationship between degree of hysteresis and floodplain surface complexity—FSC—is evident for the Yanga floodplain (Figure 3B). The degree of hysteresis is a relative measure of fragmentation of floodplain inundation and is determined via normalizing the axes of the total floodplain inundated area to number of wet patches relationship, thus values range from 0 (no fragmentation) to 1 (maximum fragmentation of floodwaters). The positive linear relationship found for the Yanga floodplain suggests those surfaces with a greater inundation heterogeneity experience an enhanced diversity of wet patches or available surface water resources. Given the flow regime is a driver of floodplain process, a diversity of ecosystem responses would be expected. Moreover, enhancing the response to floodplain inundation (i.e., increasing the diversity of wet patches) can be achieved by directing environmental flows to those areas of the floodplain that have a more complex topography.
3.4 Ecosystem response to floodplain inundation
Patterns of vegetation productivity in response to inundation were examined for Yanga Floodplain using the same remotely sensing data used to determine inundation patterns (Shilpakar, 2013). For each remotely sensed image, the Normalized Difference Vegetation Index (NDVI) was calculated. NDVI measures vegetation greenness, which is related to the ability of vegetation to absorb photosynthetically active radiation and is a surrogate for vegetation vigour (Turner et al., 2003). For the Yanga Floodplain study, NDVI was used to examine patterns of vegetation productivity at the same two scales as patterns of inundation were investigated.
Significant differences in the NDVI response were recorded between the inundated and non-inundated sections of the Yanga floodplain. Overall, the inundated floodplain had higher NDVI values compared to the non-inundated floodplain. The mean NDVI value of the inundated floodplain was 0.54 (range: 0.40–0.72) compared to a mean of 0.31 (range: 0.22–0.60) for the non-inundated floodplain (Figure 4). Differences in NDVI were also recorded between flood events for the inundated section of floodplain. Mean NDVI for the inundated floodplain of flood three was 0.60 (range: 0.46–0.72), which was slightly higher than the mean of 0.51 recorded for both flood one (range: 0.40–0.58) and flood two (range: 0.45–0.55) (Figure 4). Thus, inundation enhances the vigour of floodplain vegetation productivity, and the response to inundation does vary between floods.
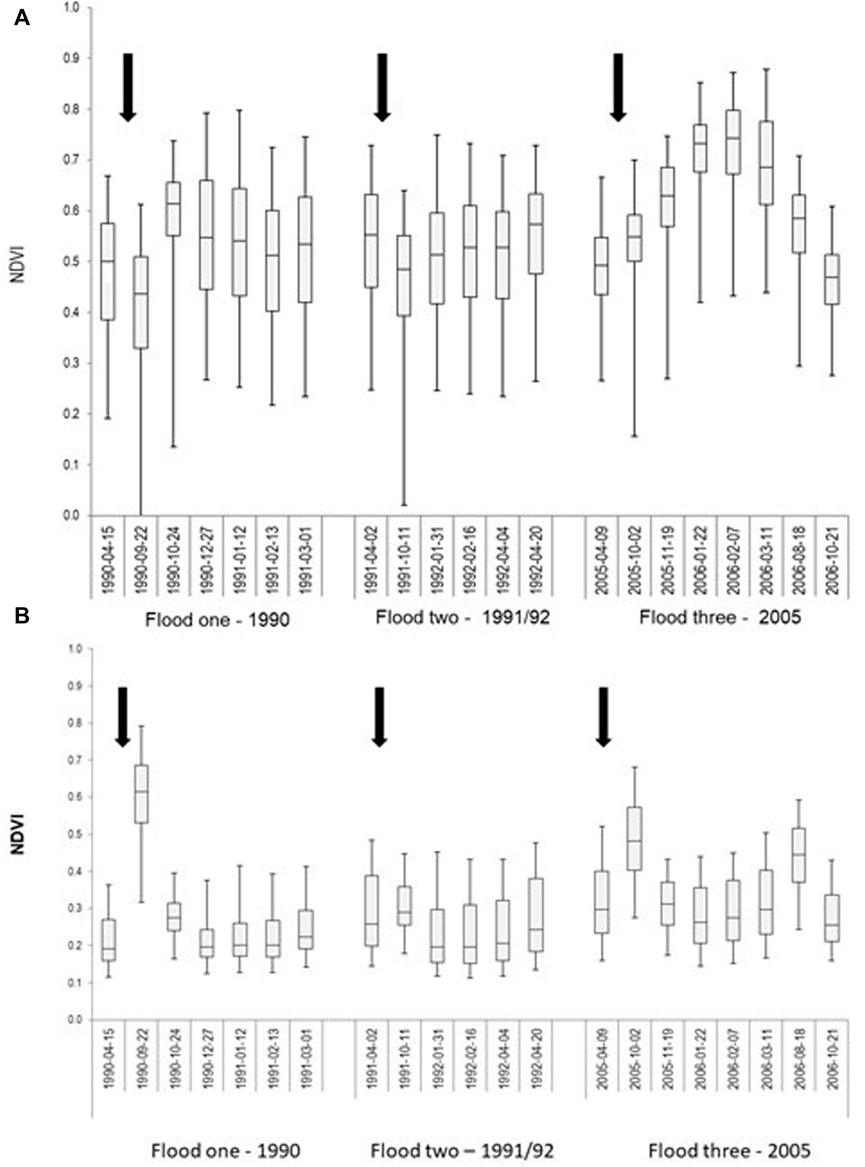
FIGURE 4. Floodplain vegetation (NDVI) response to surface water inundation during three flood events in the (A) inundated floodplain, and (B) non-inundated floodplain. The box whisker diagrams provide the inter-quartile range, mean and median values as well as the 2.5 and 97.5 percentiles. The first image of each sequence is the pre-flood condition, and the arrows indicate the commencement of floodplain inundation (modified from Shilpakar, 2013).
Distinct temporal response patterns in NDVI were recorded for the inundated floodplain (Figure 4A). During flood one, the inundated floodplain had an average NDVI of 0.48 before inundation (April 1990 image). NDVI subsequently decreased to 0.40 immediately after inundation (September 1990) and then peaked at a mean of 0.58 the following month (October 1990). Following the peak, NDVI values gradually declined to a minimum of 0.50 by February 1991. A similar NDVI pattern occurred during flood two (Figure 4A). Prior to floodplain inundation (May 1991), a mean NDVI of 0.53 was recorded, which reduced to 0.45 immediately following inundation (October 1991) and then increased to 0.51 by January 1992 where it remained at this level until April 1992 (Figure 4A). During flood three, the mean NDVI increased from 0.49 before inundation (April 2005) to 0.53 immediately after inundation (October 2005). NDVI then gradually increased to a peak NDVI of 0.72 by February 2006, 4 months after initial inundation (Figure 4A). Following this peak, mean NDVI gradually declined to 0.46 by October 2006. The total increase in mean NDVI following inundation was greater for flood three than floods one and two, but the inter-quartile range indicates there was less variation in NDVI across the inundated floodplain for flood three than for flood one and flood two. By comparison NDVI values for the non-inundated floodplain for the same flood events were not only markedly lower, but also there was no notable NDVI temporal response pattern (Figure 4B). On average, mean NDVI values were 0.25 lower for flood one, 0.33 for flood two, and 0.31 for flood three across the non-inundated floodplain compared to the inundated floodplain.
Differences in the NDVI responses of the eight vegetation communities also occurred, and these variations were related to the topographic complexity (i.e., FSC values) associated with each vegetation community (Table 3). The river red gum gallery forest (FSC = 0.79) had a higher NDVI response (mean = 0.61; range: 0.51–0.72 for the three floods) than all other floodplain vegetation communities. In contrast, lignum dominated shrubland (FSC = 0.29) exhibited the lowest NDVI response among the eight vegetation communities (mean = 0.32; range: 0.28–0.60). Most of the floodplain vegetation communities recorded a relatively higher NDVI response during flood three.
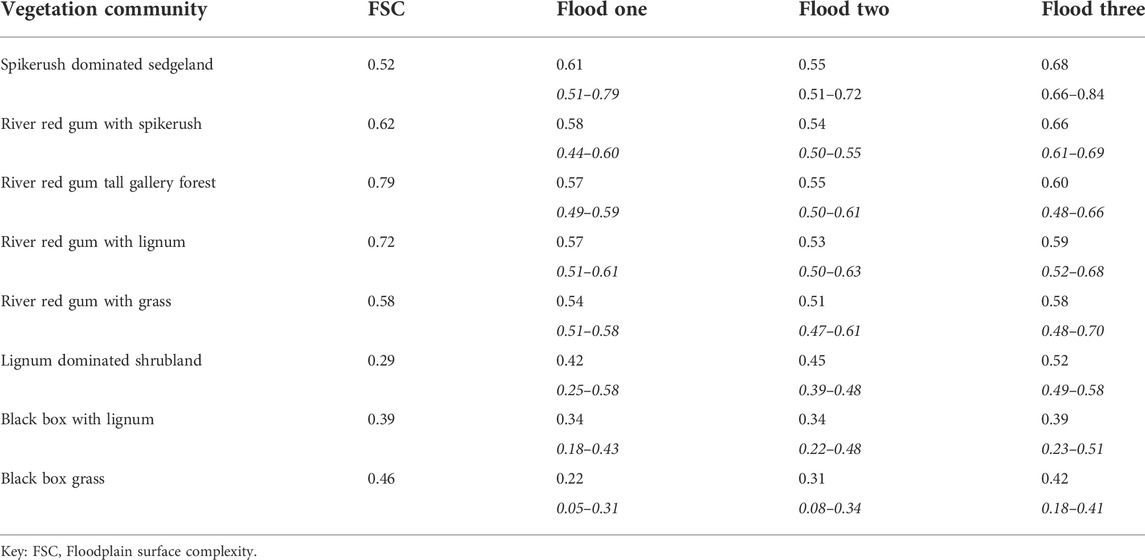
TABLE 3. Floodplain vegetation response to inundation. The mean and range of NDVI values (ranges are in italics) for each vegetation community during the three flood events are provided.
3.5 Functional flows in the floodplain
The science of environmental water management has increased exponentially over the last several decades. However, this knowledge is skewed towards research on environmental flows within river channel environments or the riverscape (Horne et al., 2017). Floodplains are a significant feature of riverine landscapes; current estimates suggest floodplains occupy >3.14 × 106 km2 of the global land surface (Tockner and Stanford, 2002; Thoms and Parsons, 2016), yet efforts to understand floodplain environmental flows and their functional responses to environmental watering is limited. Environmental flows are increasingly being used to restore degraded floodplain vegetation; however, the type of flow regime required for recovery to healthy conditions has varied because of limited knowledge of the interactions between flow, floodplain topography, the state of vegetation, and vegetation responses to flow variability (Campbell et al., 2021). This case study of the Yanga Floodplain, Australia, highlights the importance of floodplain geomorphology, and specifically, the importance of the complexity of the floodplain surface in providing the template upon which the diversity of ecosystem responses is set. Sustainable floodplain management focused on functional responses and promoting diversity of functional responses is an alternative to current environmental flow management of floodplains. This case study also emphasizes the importance of understanding the interplay and influence of geomorphology on floodplain ecosystem responses (Figure 1). Unpacking these relationships will improve upon the current focus of simply getting water onto floodplains. For the Yanga Floodplain, coordinating in-channel water levels with strategic management of the gated regulators can direct flows to those floodplain areas with a greater surface complexity thus enhancing ecosystem response.
4 Case study 2: Restoring geomorphic functionality in River channels—The Trinity River, United States
Hydrogeomorphic and ecological processes in the riverine landscape are not only influenced by active floodplains but also by the balance between the sediment supplied from the watershed and the ability of the river to move the sediment (Lane, 1955). In semi-confined and confined river systems, flow and sediment regimes are tightly coupled creating a variety of channel patterns and forms driven primarily by abiotic processes dictated by the geology and climate of the watershed (Figure 1). As sediment supplies flux with varying flow regimes over time and space, erosion and deposition processes create a diversity of channel habitats that support diverse aquatic communities (Yarnell et al., 2015). In particular, the relationship between sediment supply and flow transport capacity has been shown to be a key factor in determining channel patterns (Wohl et al., 2015), with low supply:capacity ratios creating incised straight channels and high supply:capacity ratios creating braided channels (Montgomery and Buffington, 1997; Kondolf et al., 2002). Although the full range of channel patterns from straight to meandering to braided can be found throughout natural river systems (Schumm, 1985), infrastructure and development, particularly dams, can drastically alter the relationship between sediment supply and flow transport capacity, in turn altering the river template and channel form.
Among other impacts such as altered flow regimes, dams retain coarse sediment, altering the sediment regime and associated supply:capacity ratio downstream (Church, 1995; Kondolf, 1997; Petts and Gurnell, 2013). Elimination of bed material inputs downstream from dams has been shown to lead to channel incision, coarsening of the streambed, decreased bed mobility, and a loss of topographic and habitat diversity (Lisle et al., 1993; Grams et al., 2007). Efforts to improve stream habitat conditions downstream of dams have traditionally focused on setting flow volumes via environmental flows or channel habitat restoration actions such as channel bar creation (Horne et al., 2017); however, consideration of the interactions between flow and sediment regimes and the resulting river functions and processes is needed to effectively manage and improve river ecosystems below dams. In this case study, we summarize and discuss studies completed over the past several decades on the Trinity River in California, United States to illustrate the importance of river drivers, specifically the interactions between flow and sediment, in influencing the diversity of functional responses to abiotic processes (Figure 1) and highlight the need for understanding the interplay between physical drivers, abiotic processes, channel form and aquatic habitat, and species responses.
4.1 The Trinity River
Like many dammed rivers, the Trinity River has experienced long-term channel degradation, loss of habitat heterogeneity, and associated declines in native fish populations downstream of the Lewiston Dam and reservoir. The Trinity River (drainage area of 7,679 km2) is representative of many Mediterranean-montane watersheds with an average annual precipitation of 900–1,900 mm and a highly seasonal mixed rain-snowmelt flow regime (Buffington et al., 2014) (Figure 5). Large winter storms provide high streamflow from October to March, and snowmelt from higher elevations creates a spring snowmelt recession between April and June. Baseflow is sustained through the dry summer months of July-September by receding snowmelt and shallow subsurface flow contributions until precipitation returns the following autumn providing increased streamflow. Much of the gravel-bedded river is partially confined, exhibiting a mixture of alluvial and bedrock-controlled channel morphologies with alluvial forms increasing in the downstream direction (Buffington et al., 2014).
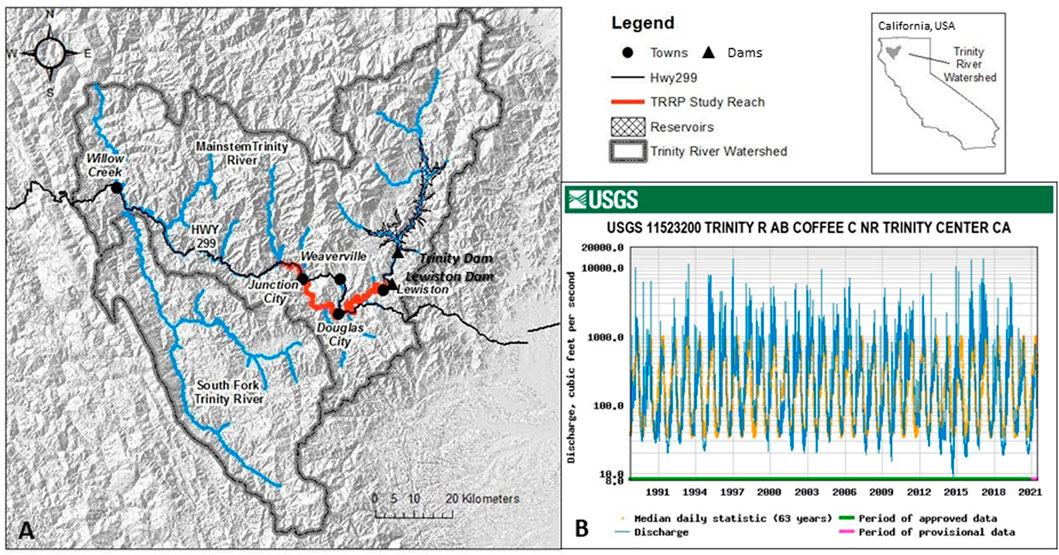
FIGURE 5. Geography and hydrology of the Trinity River. (A) Watershed location in California, United States (Lat: 40.7249°N; Long: 122.7961°W). (B) Unimpaired hydrograph upstream of Lewiston and Trinity Dams. Map from USGS; flow data from USGS gage 11523200 (https://nwis.waterdata.usgs.gov/ca/nwis/); “Median daily statistic” is the average daily flow over the period of record (1953–2021).
Built in 1962, Lewiston Dam and Trinity Dam (located 13 km upstream) currently divert up to 50% of total inflow for agriculture and community water supply uses (decreased from diversions of up to 90% during the first decade following construction) and block virtually 100% of the natural sediment supply to downstream reaches (Kondolf and Minear, 2004). Monthly mean flows prior to the dams were typically 4–115 m3 s−1, with annual peak flows of 140–1,100 m3 s−1 and as high as 2,100 m3 s−1; however, following installation of the dams, monthly mean flows ranged from 4 to 15 m3 s−1 and annual peak flows were reduced to less than 100 m3 s−1, with a high flow event of ∼354 m3 s−1 in 1963 and a high flow event of 408 m3 s−1 in 1974 (Nelson et al., 1987; Kondolf and Minear, 2004). Elimination of the bed material inputs downstream from the dams resulted in channel armor, incision, and riparian encroachment, and drastically changed the riverine habitat with subsequent effects on native fish populations, including several runs of salmon (chinook salmon (Oncorhynchus tshawytscha), coho salmon (Oncorhynchus kisutch), and steelhead trout (Oncorhynchus mykiss)) that no longer can access stream habitat upstream of the dams. By 1980, 80%–90% of salmonid spawning and rearing habitat in the downstream reaches was lost, and salmon returns had decreased by 85% (Nelson et al., 1987; USFWS and HVT (U.S. Fish and Wildlife Service and Hoopa Valley Tribes), 1999).
4.2 Channel restoration activities
A project report by Kondolf and Minear (2004) provides a detailed history of restoration activities in late 20th century. Beginning in the mid-1970s, the loss of gravel and coarse sediment suitable for salmonid spawning was noted as a key factor in reduced salmonid populations, and efforts were made to improve spawning habitat in the stream reach immediately below Lewiston dam. Given the confined nature of the channel and the presence of the Lewiston fish hatchery in the adjacent channel floodplain, gravel augmentation in the form of artificial riffles was utilized to improve spawning habitat. The artificial riffles were comprised of suitably sized spawning gravel fixed into place with lines of boulders or “weirs” lateral to the channel to prevent erosion of the riffle gravel. A series of seven riffles was constructed immediately below the dam in 1976, and another eight riffles were added in 1977 in the next reach downstream, for a total of about 16,820 m3 of gravel augmentation within the river. Following high flow events of approximately 241 m3 s−1 in 1983 and 176 m3 s−1 in 1984, the riffles were visibly degraded through loss of gravel and damage to the boulder weirs. The riffles were ‘ripped’ or scarified and replenished with approximately 1,530 m3 of new gravel following each event. Additional high flow events in 1995–1998 (170–198 m3 s−1) spurred further repair and gravel augmentation to the riffles in 1999–2001. The majority of these gravel augmentation efforts were poorly documented with no monitoring or evaluation of effectiveness, particularly in regard to salmonid use. Rather, riffle repair and gravel additions occurred ad hoc after high flow events had visibly washed a significant portion of gravel downstream. Field surveys in 2004 showed many of the riffle structures were intact, but some had degraded or shifted, and the large boulder weirs intended to stabilize the riffles had shifted or were missing in several locations. When compared with other spawning riffle construction projects in the upper Sacramento, Merced, Tuolumne, and Stanislaus Rivers in northern California completed during the same era, Kondolf and Minear (2004) found that the Trinity River artificial riffle gravels were similarly washed through the downstream river reaches in 1–4 years following high flow events, indicating a pattern of long-term sediment deficit and channel degradation. Although well-intended, these early gravel augmentation efforts were largely unsuccessful in improving fish habitat conditions and fish populations remained in peril.
In 2000, efforts to improve fisheries habitat and riverine conditions in the Trinity River were organized into the Trinity River Restoration Program (TRRP) under a “Record of Decision” by the US Department of the Interior (https://www.trrp.net/program-structure/background/rod/). Despite some early legal challenges, the TRRP was approved and underway by 2004 with a defined restoration strategy that included a variable environmental flow regime designed to mimic more natural flows in spring, treatment of the stream channel with mechanical channel rehabilitation to reshape the channel form to “establish physical processes that will create and maintain fish habitat”, gravel augmentation to increase the supply of spawning gravels below Lewiston Dam, watershed restoration actions to reduce fine sediment inputs to the river, modifications of structures in the floodplain to allow high peak flow events, and an adaptive assessment and monitoring program with environmental compliance and mitigation (www.trrp.net/program-structure/backgroun/rod/). Over the next 10 years, an extensive series of studies, analysis, and discussions were completed to develop conceptual models for the ecology of the Trinity River, determine flow, sediment, and channel morphology needs to improve instream habitat, conduct restoration actions, and evaluate initial results.
Efforts have been focused in the “restoration reach” from the Lewiston Dam to the confluence of the North Fork Trinity River 40 miles (64.4 km) downstream, at which point flow and sediment inputs from tributaries substantially improve river habitat conditions (Buffington et al., 2014) (Figure 6A). Beginning in 2005, a new environmental flow regime was initiated that focused on increased flow variability during spring to provide several different ecological and geomorphic functions specific to salmon life history requirements (Figure 6B). The environmental flow regime was paired with channel rehabilitation actions that included targeted side channel habitat expansion and gravel augmentation via high-flow injection, where gravel is added to the river during high-flow events that are capable of transporting and redistributing the sediment. Collectively, these efforts were intended to not just create fish habitat and channel forms, but to achieve the program goals of supporting geomorphic processes that create a diversity of habitats that exhibit spatial and temporal variability in suitability for various fish life stages (Buffington et al., 2014).
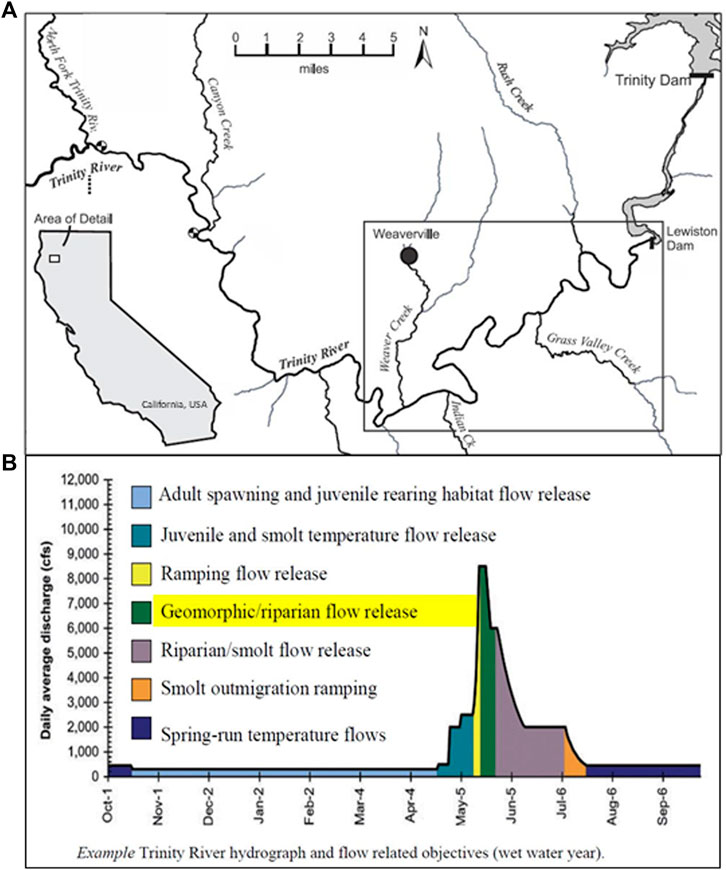
FIGURE 6. (A) Map of the Trinity River between Lewiston Dam and the North Fork Trinity River where restoration activities have been focused since 2004. Gravel augmentation efforts have been focused in the boxed portion of the river, which is shown in greater detail in Figure 5. Reproduced from (Gaeuman, 2020). (B) Example of environmental flow regime and flow-related objectives proposed for “wet” water year types under the Trinity River Restoration Program. Reproduced from (TRRP, 2009).
4.3 Channel sediment augmentation activities
The sediment management program (a sub-group of the TRRP) in particular focused on short- and long-term gravel augmentations to restore and maintain substrate mobility and aquatic habitat quality downstream of Lewiston Dam (Buffington et al., 2014; Gaeuman, 2014). Gravel supplies were augmented in the upper portion of the restoration reach extending from Lewiston Dam to Indian Creek, located 16.4 river miles (26.4 km) downstream (Figure 7A) primarily using high-flow injection methods. During planned high flow releases, gravels of suitable spawning size were dumped into high velocity zones such as the outside of river bends or channel constrictions (for additional details on the augmentation methods, see Gaeuman, 2014). Similar to water year type variations in the annual flow release hydrographs, prescriptions for gravel augmentation varied by water year type, with little or no gravel additions in drier years and large or extremely large augmentation quantities in wetter years (Gaeuman, 2014).
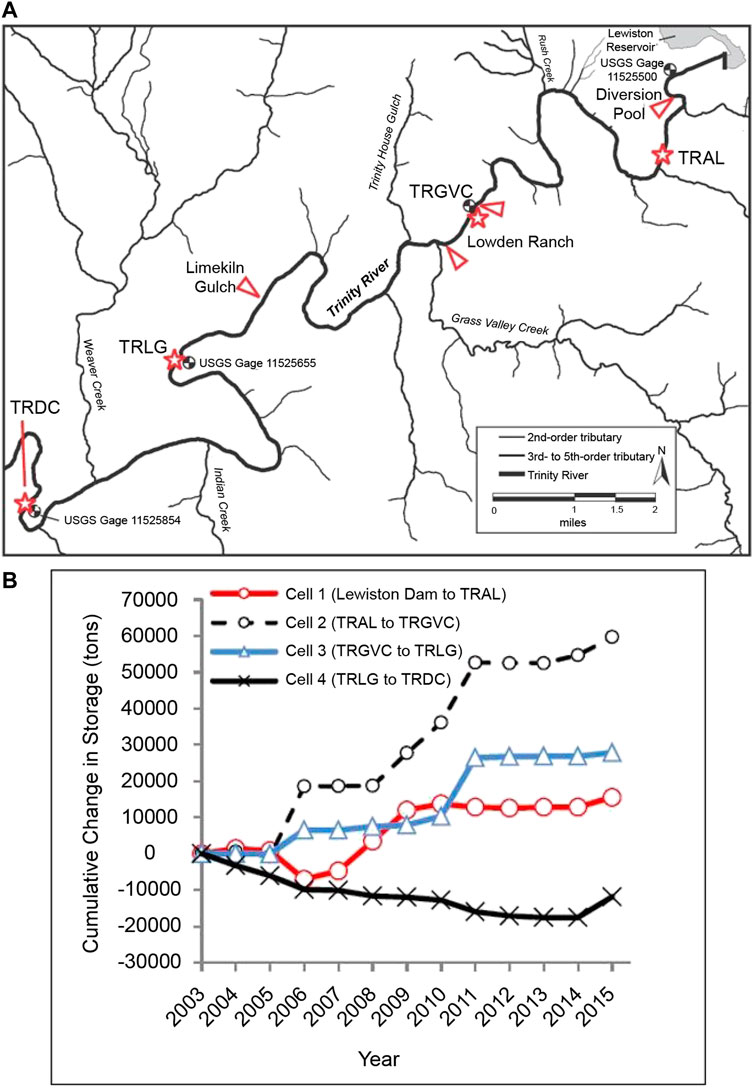
FIGURE 7. (A) Map of the Trinity River between Lewiston Dam and the Trinity River stream gage at Douglas City. Red triangles point to gravel augmentation locations; red stars labelled TRAL, TRGVC, TRLG, and TRDC indicate sediment monitoring locations. Reproduced from (Gaeuman, 2020). (B) Cumulative changes in gravel storage by sediment budget cells located between sediment monitoring locations (TRAL, TRGVC, TRLC, TRDC) for Water Years 2004–2015 with zero budget balance assigned to Water Year 2003. Reproduced from (Gaeuman and Stewart, 2017).
Augmentations were conducted almost annually from 2004–2015 with volumes ranging from 1,055 to 9,490 m3, with the largest injections occurring during wet years from 2008 to 2011 (Gaeuman and Stewart, 2017). This monitoring of coarse sediment transport indicated bedload actively moved through the reach during each high flow event, with higher magnitude flows typically exhibiting higher transport rates. Moderate flows largely increased local gravel storage and dynamically built bedforms and bars near injection sites, while higher flows mobilized and transported coarse material further downstream (Buffington et al., 2014). For example, following the 340 m3 s−1 high flow event in 2011, monitoring during and after the event showed a net transport out of the study reach and reach-scale erosion in the downstream reaches indicating gravel loads about 4.5 times larger than the quantity of gravel injected upstream (Gaeuman, 2014). Gravel budget calculations from 2003 to 2015 showed that gravel storage continued to increase over time in all but the most downstream monitoring reach (Figure 7B); however, year to year variability in gravel transport relative to high flow magnitudes illustrate the vulnerability of features created by deposition of gravel injected during moderate flow events to erosion by larger flow events (Gaeuman and Stewart 2017).
Monitoring results from continued gravel augmentations in 2016–2019 supported previous findings that moderate flow events (e.g., 269 m3 s−1 in 2016, 255 m3 s−1 in 2019) deposited injected gravel in dynamically formed channel features close to injection sites, while higher flow events (e.g., 340 m3 s−1 in 2017; 309 m3 s−1 in 2019) eroded these features and transported sediment further downstream (Gaeuman, 2020). As a result, restoration goals of supporting dynamic geomorphic processes that create diverse habitats throughout the study reach have only been partially met. Specifically, geomorphic responses have been limited in some areas, such as immediately below the dam, due to the presence of confined banks and bedrock boundaries that make the river less alluvial and responsive than originally hypothesized (Buffington et al., 2014). The straight confined nature of the channel and lack of velocity reversals or fluctuations in channel width results in high shear stresses at moderate and high flows that limit self-sustaining features (Brown and Pasternack, 2008). Thus, recommendations within the adaptive management framework of the TRRP include implementing and testing dynamic rehabilitation designs (such as gravel augmentation and lateral widening) in predominantly alluvial reaches, but employing static designs in constrained and semi-alluvial reaches where habitat enhancement is desired but cannot be achieved due to a lack of dynamic condition (Buffington et al., 2014).
4.4 Ecosystem response to channel restoration
The success of the sediment management program within the TRRP with respect to improving fish rearing and spawning habitat and associated fish response has been mixed according to several recent follow-up studies. The channel rehabilitation and gravel augmentation projects generally increased juvenile rearing habitat availability at baseflow (summer = 12.7 m3 s−1; winter = 8.5 m3 s−1) over time for coho (Oncorhynchus kisutch) and Chinook salmon (O. tshawytscha) (Buffington et al., 2014; Boyce et al., 2020). However, returns (escapement) of Chinook salmon from 2002 to 2017 did not meet restoration program goals, and there was not a significant change in the abundance of Chinook salmon redds over the same period (Gough et al., 2019; Boyce et al., 2020). Given the number of confounding factors and stresses that adult salmon face during their life cycle, data has been insufficient thus far to determine if juvenile rearing habitat is the key limiting factor for adult survival and associated population size in the Trinity River.
Despite a lack of relationship between channel habitat improvements and fish population size, the creation and maintenance of in-channel habitat features such as gravel bars from the environmental flow regime, gravel augmentation, and channel rehabilitation efforts has had notable effects on the aquatic habitat in the Trinity River in general. Ock et al. (2015) found that gravel bars resulting from the gravel augmentations increased channel complexity, promoted hyporheic flows, and increased suspended particulate organic matter retention, ultimately resulting in thermal heterogeneity and food availability along the gravel bar and channel. These conditions can lead to rapid colonization of macroinvertebrate species which benefit other aquatic species in addition to fish (Merz and Ochikubo Chan, 2005). While these ecological benefits can be transitory if the gravel bars and in-channel structures are washed away in subsequent high flow years without ongoing gravel replenishment, a diversity of design approaches to maintaining habitats will promote species resilience to changing environmental conditions (Buffington et al., 2014). For example, varying the locations of gravel augmentations from year to year would promote local geomorphic change and increase gravel dispersion, increasing habitat benefits over longer stretches of the river and reducing the risk of local habitat simplification associated with the oversupply of gravel to a small area (Gaeuman, 2020). Similarly, focusing dynamic restoration efforts in alluvial reaches that respond quickly to changes in flow and sediment regimes could better support the ecological and geomorphic functions that promote diverse habitat conditions over time.
4.5 Functional flows in the Trinity River
The Trinity River case study highlights the importance of understanding not only interactions between the flow and sediment regimes, but also the various river dependent functions that create the abiotic channel forms and biotic community structure (Figure 1). In confined or incised reaches where erosion and deposition processes are limited due to a lack of sediment supply from upstream and lack of access to the floodplain as a local sediment source, the channel form becomes static, homogeneous, and lacking in spatial heterogeneity. The lack of abiotic functionality results in an inability for the channel form and community structure to flux over time or space, decreasing complexity in the river templates, and limiting diverse ecosystem responses (Figure 1). When sediment supplies were augmented in the confined reaches of the Trinity River allowing for erosion and re-deposition of sediment into gravel bars, instream channel heterogeneity increased and aquatic communities responded (Ock et al., 2015). However, without on-going sediment augmentation supporting the sediment regime, which requires continued funding and available gravel, this abiotic functionality will decrease over time and space.
In the semi-confined reaches of the Trinity River where access to side-channels and available floodplain is possible, local sediment sources become available for mobilization and redistribution by varying flows, resulting in increased geomorphic heterogeneity. As discussed in the Yanga Floodplain case study, but on a smaller scale, increased geomorphic heterogeneity within the channel and adjacent overbank areas allows for increased complexity in ecosystem responses when flows can access such areas. Activities such as channel reconfiguration or targeted floodplain restoration that promote self-sustaining abiotic processes in these less confined reaches will increase the overall functionality and resilience within the river system. However, if the environmental flow regime remains focused on the spring flow component (Figure 6) without consideration of larger winter flood flows that access the available floodplain, promote deep scour, and generate riparian succession, greater functionality within the river system will be limited. Within the TRRP, expanding the defined measures of success beyond fish population size to include geomorphic heterogeneity, diversity in community structure and water quality conditions, and the degree to which these templates flux over time and space, will allow for a more comprehensive understanding of whether management actions taken to promote river functionality have increased ecosystem responses and associated species diversity.
5 The importance of biophysical process understanding in the application of functional flows for enhancing resilience in Anthropocene Rivers
The concept of resilience and its application to the management of riverine landscapes been developing over the last decade (Thoms et al., 2018; Pingram et al., 2019). Resilience relates to the ability to persist in the face of gradual and abrupt change and the ability to transform or adapt along new development pathways (Parsons and Thoms, 2018). The classic definition of Holling (1978) defines resilience as the amount of change a system can undergo (its capacity to absorb disturbance) and remain within the same regime—essentially retaining the same function, structure, and feedbacks. The concept of resilience also comprises other components that describe the dynamics of riverine landscapes and their associated ecosystems: multiple “basins of attractions” or system states, regime shifts, thresholds and tipping points, fast and slow variables, and adaptive cycles (Thoms et al., 2018). Much of the empirical basis of resilience is generated from studies of these components.
In dynamic systems, changes in a driver variable (cf. Figure 1) can lead to changes that create feedbacks to the original variable (Scheffer, 2009). Feedbacks can amplify (positive feedbacks) or dampen (negative feedbacks). Slow variables, such as changes initiated by changes in climate, land use, or flow and sediment regimes, influence river system dynamics over decades. Fast variables respond quickly at daily, seasonal, or annual time scales. For example, in the case of the Trinity River, installation of the dams blocked sediment supply to downstream reaches, fundamentally changing the sediment regime over the course of decades and resulting in channel incision and armored substrate to which native fish responded negatively over time. Controlling variables set bounds on the possible configurations of a river system, such that resilient systems are able to absorb disturbances and maintain structure, function, and feedbacks, and therefore are able to remain in the same state (Biggs et al., 2015). As shown in the Yanga Floodplain, surface topography is a controlling variable of floodplain heterogeneity. It influences not only the distribution of inundated floodwaters, and ultimately the diversity of soil moisture conditions across the floodplain, but also vegetation growth and rigor (Thapa et al., 2016). Changes in surface topography have occurred across the Yanga Floodplain through land clearance (Scown et al., 2016b). This change in floodplain heterogeneity may represent a trigger to a different state or basin of attraction that influences the longer-term stability of floodplain ecosystems (Thoms and Parsons, 2016). If a river system becomes unable to absorb or adapt to disturbances, a threshold (or tipping point) may be reached and crossed. When systems are close to a tipping point, disturbances that a river ecosystem was once able to absorb may now push it over a tipping point to an alternative state, with a different structure, function, and feedbacks (Scheffer, 2009). This flip into a new “basin of attraction” or system state is irreversible and associated with a decrease in system productivity. Moreover, it has been hypothesized that once a system flips into a new state or basin of attraction, the potential for further flips increases (Scheffer, 2009). Recent research along the Illinois River (IL, United States) showed that once degraded by pollution from the Chicago metropolis, the river did not recover to its Pre-Settlement state following major watershed wide restoration efforts (DeBoer et al., 2019; DeBoer et al., 2020). Rather, the Illinois River showed “novel” unexpected responses, some of which suggest a reduction in its capacity to absorb future disturbances.
Many argue riverine landscapes have flipped into a new system state during the Anthropocene (e.g., Kelly et al., 2018). Less than 37% of rivers longer than 1,000 km remain free flowing, and only 23 percent flow uninterrupted to oceans because of dams and reservoirs (Grill et al., 2019). Humans have also extensively modified riverine landscapes through land uses and other activities (Vitousek et al., 1997). These pronounced and persistent modifications have resulted in human induced regime shifts. Thus, establishing different strategies for the restoration and management of Anthropocene riverine landscapes needs to occur because those based on our understanding of pristine systems are often not applicable. Understanding the response of Anthropocene riverine landscapes to both future “natural” disturbances and human management actions is challenging, in part because research has been limited to date; however, The application of a functional flows approach provides an excellent example for managing regulated rivers in the Anthropocene.
The integration of a Functional Flows approach and resilience thinking provides a pathway forward for managing regulated rivers in the Anthropocene. First, the conceptual framework for Functional Flows outlined in Figure 1 highlights an interdisciplinary approach that recognizes the structure, function, and interactions in and between the physical, chemical, and biological domains of riverine landscapes in both time and space. Reinstating the functionality of flows over time, i.e., flow variability (Poff et al., 1997; Naiman et al., 2008), is a central tenet and focus of most environmental flow programs. While beneficial, a focus on flow variability alone ignores the spatial component of flow regime changes, their interacting processes, and their ecosystem responses. A Functional Flows approach explicitly incorporates a spatial component, i.e., the flows needed to support functionality in space and the heterogeneity of functions. Combining temporal variability and spatial heterogeneity in the management of riverine landscapes creates a dynamic habitat mosaic across both the riverscape and floodscape (Yarnell et al., 2015). A dynamic habitat mosaic is the product of flow interacting with multiple biophysical templates (Figure 1). The substantial changes in the mosaic of floodplain wet patches occurring over time in the Yanga floodplain (Section 3 above) was the product of flow changes interacting with the heterogeneity of the floodplain surface topography. Further, a Functional Flows approach, like other holistic environmental flow frameworks (e.g., Poff et al., 2010), explicitly incorporates the importance of hydrological, geomorphological, and ecological processes and their interactions. While acknowledging flow as an important driver of river ecosystems and management strategy in regulated rivers, it is not the only driver that influences ecosystem functionality across riverine landscapes (Figure 1). The interplay between flow and sediment regimes are particularly important in confined and semi-confined river systems, such as the Trinity River, where alterations to the relationship between sediment supply and flow transport capacity from dams or other infrastructure development can drastically alter channel conditions beyond what is required by native aquatic species (Section 4 above). Understanding the interrelated functional processes that create a dynamic habitat mosaic in floodplains and support diverse habitat in channels can lead to more efficient flow management with improved ecosystem outcomes, thereby moving beyond just attempting to reinstate a more natural flow regime.
Second, the future resilience of riverine landscapes means maintaining capacity to absorb and adapt to drivers of change or disturbances, while remaining in essentially the same regime that retains the same function, structure, and feedbacks. The focus of flow management in the Anthropocene would be to prevent further flips into other less productive states, regimes, or basins of attractions. Theoretically, such focus would aim to increase diversity and redundancy in the structure, function, and interactions occurring within the riverine landscape, thus enhancing the system’s adaptive capacity or ability to absorb disturbances and retain the same structure, function, and set of interactions. The aim of a Functional Flows approach is to promote dynamic riverine landscapes over space and time, thus enhancing high biodiversity and the processes for self-rehabilitation. Resilience in the face of future disturbances such as climate change can only be achieved when rivers are dynamic, variable, and have the ability to naturally adjust. Maximizing functionality enhances the adaptive capacity of riverine landscapes, thereby promoting resilience, according to our understanding of river processes (cf. Figure 1). Dynamic interactions are linked across flow, sediment, and biogeochemical regimes. If these linkages are supported and maintained, the system has the ability to respond to changing conditions. Our current state of knowledge suggests this can be achieved by looking at river processes and functions holistically, and working to restore the functionality of flow regimes.
6 Conclusion
In highly modified riverine landscapes—Anthropocene systems—environmental flows should consider physical and ecological processes (the basis for functioning river systems) and embrace channel dynamism to better support riverine health and biodiversity. Although other factors such as water quality and non-native species interactions may also limit ecosystem functioning, dynamic flow and sediment interactions are the core physical processes central to the interconnected river web, as demonstrated here in the Yanga floodplain and Trinity River case studies. Environmental flow efforts that do not include consideration of geomorphic processes may be effective, but not achieve the full functionality that riverine landscapes and their associated ecosystems require.
A Functional Flows approach to managing rivers in the Anthropocene challenges current environmental flow paradigms. The approach emphasizes the need to understand how flows and other biophysical processes function over time and space within riverine landscapes. Knowledge of these complex interactions is critical in order to maintain future adaptive capacity, which is a key part of resilience. Adaptive capacity is promoted by inherent redundancies within complex systems; redundancies that are essential for the biodiversity of riverine landscapes and their ability to absorb future disturbances. Identifying and promoting redundancies in river functions, templates, and species responders are key objectives to increasing resilience under a Functional Flows approach (Figure 1), all of which requires a biophysical process understanding of riverine landscapes.
An important goal for water managers and environmental flow programs is to ensure Anthropocene rivers remain resilient and limit transitions to new unintended, less productive, future states. Focusing on the full functionality of flows within riverine landscapes can achieve this. Environmental flow science has largely been the domain of freshwater ecologists who have in the past emphasized the importance of mimicking the natural flow regime (e.g., Poff et al., 1997; Palmer and Ruhi, 2019). This singular focus, while important, is limited and limiting. An understanding of fluvial geomorphology allows for greater emphasis on abiotic processes and sediment dynamics over space and time that directly relate to ecosystem responses. Similarly, incorporating an understanding of biogeochemical processes is important in Anthropocene rivers with degraded water quality. A Functional Flows approach to environmental flow management takes a holistic perspective towards restoring processes and functions that confer heterogeneity and diversity within river systems. Dynamic riverine landscapes promote complexity, and thus resilience, helping to buffer against changing climate conditions and additional anthropogenic perturbations thereby promoting long term sustainable ecosystem services that are valued by society.
Data availability statement
The original contributions presented in the study are included in the article/supplementary material, further inquiries can be directed to the corresponding author.
Author contributions
SY led the preparation of this manuscript. MT completed the Murrumbidgee Floodplain case study. SY completed the Trinity River case study. SY and MT contributed to the development of the manuscript concepts and to writing the manuscript.
Funding
Funds towards open access publication fees were provided by the University of California, Davis library.
Acknowledgments
We thank the chief and associate editors and reviewers for their insightful comments which improved this manuscript. We are grateful for access to data collected in the Yanga floodplain by R Shilpakar and M Scown, as well as the support provided by the Murrumbidgee Catchment Management Agency to MT for the Yanga study.
Conflict of interest
The authors declare that the research was conducted in the absence of any commercial or financial relationships that could be construed as a potential conflict of interest.
Publisher’s note
All claims expressed in this article are solely those of the authors and do not necessarily represent those of their affiliated organizations, or those of the publisher, the editors and the reviewers. Any product that may be evaluated in this article, or claim that may be made by its manufacturer, is not guaranteed or endorsed by the publisher.
References
Abbe, T. B., Brooks, A. P., and Montgomery, D. R. (2003). “Wood in river rehabilitation and management,” in Ecology and management of wood in world rivers. Editors S. V. Gregory, K. L. Boyer, and A. M. Gurnell (Bethesda, Maryland: American Fisheries Society), 367–389.
Amoros, C., and Bornette, G. (2002). Connectivity and biocomplexity in water bodies of riverine floodplains. Freshw. Biol. 47 (4), 761–776. doi:10.1046/j.1365-2427.2002.00905.x
Arthington, A. H., Bhaduri, A., Bunn, S. E., Jackson, S. E., Tharme, R. E., Tickner, D., et al. (2018). The brisbane declaration and global action agenda on environmental flows (2018). Front. Environ. Sci. 6, 45. doi:10.3389/fenvs.2018.00045
Beechie, T. J., Sear, D. A., Olden, J. D., Pess, G. R., Buffington, J. M., Moir, H., et al. (2010). Process-based principles for restoring river ecosystems. Bioscience 60 (3), 209–222. doi:10.1525/bio.2010.60.3.7
Bernhardt, E. S., Blaszczak, J. R., Ficken, C. D., Fork, M. L., Kaiser, K. E., and Seybold, E. C. (2017). Control points in ecosystems: Moving beyond the hot spot hot moment concept. Ecosystems 20, 665–682. doi:10.1007/s10021-016-0103-y
Biggs, R., Schlüter, M., and Schoon, M. L. (2015). “An introduction to the resilience approach and principles to sustain ecosystem services in social–ecological systems,” in Principles for building resilience: Sustaining ecosystem services in social–ecological systems (Cambridge, UK: Cambridge University Press), 1–31.
Bovee, K. D. (1982). A guide to stream habitat analysis using the instream flow incremental methodology. Fort Collins, CO: U.S. Fish and Wildlife Service.
Boyce, J., Goodman, D. H., Som, N. A., Alvarez, J., Hopkins, K., and Martin, A. (2020). Streamflow and juvenile salmonid habitat availability at six rehabilitation sites on the Trinity River, California 2008-2017. Arcata, California: U.S. Fish and Wildlife Service; Arcata Fish and Wildlife Office.
Brown, R. A., and Pasternack, G. B. (2008). Engineered channel controls limiting spawning habitat rehabilitation success on regulated gravel-bed rivers. Geomorphology 97 (3-4), 631–654. doi:10.1016/j.geomorph.2007.09.012
Buffington, J. M., Jordan, C., Merigliano, M., Peterson, J. T., and Stalnaker, C. B. (2014). “Review of the Trinity River restoration program following phase 1,” in With emphasis on the Program's channel rehabitliation strategy Trinity River Restoration Program (Weaverville, CA.
Campbell, C. J., Freestone, F. L., Duncan, R. P., Higgisson, W., and Healy, S. J. (2021). The more the merrier: Using environmental flows to improve floodplain vegetation condition. Mar. Freshw. Res. 72, 1185. doi:10.1071/mf20303
Church, M. (1995). Geomorphic response to river flow regulation - case-studies and time-scales. Regul. Rivers Res. Mgmt. 11 (1), 3–22. doi:10.1002/rrr.3450110103
Collins, A. L., Walling, D. E., and Leeksz, G. J. L. (2005). “Storage of fine-grained sediment and associated contaminants within the channels of lowland permeable catchments in the UK,” in Sediment budgets. Editors D. E. Walling, and A. J. Horowitz (Oxfordshire, UK: Taylor & Francis), 259–268.
[Dataset] NSW Office of Water (2009). Pinneena cm — version 9.3 on DVD released in december 2009. NSW Office of water. Sydney, Australia. Available: http://waterinfo.nsw.gov.au/pinneena/cm.shtml.
DeBoer, J. A., Thoms, M. C., Casper, A. F., and Delong, M. D. (2019). The response of fish diversity in a highly modified large river system to multiple anthropogenic stressors. J. Geophys. Res. Biogeosci. 124 (2), 384–404. doi:10.1029/2018jg004930
DeBoer, J. A., Thoms, M. C., Delong, M. D., Parsons, M. E., and Casper, A. F. (2020). Heterogeneity of ecosystem function in an "Anthropocene" river system. Anthropocene 31, 100252. doi:10.1016/j.ancene.2020.100252
Delong, M. D., and Thoms, M. C. (2016). “An ecosystem framework for river science and management,” in River science: Research and management for the 21st century. Editors D. A. Gilvear, M. W. Greenwood, M. C. Thoms, and P. A. Wood (Chichester: John Wiley & Sons).
Dollar, E. S. J., James, C. S., Rogers, K. H., and Thoms, M. C. (2007). A framework for interdisciplinary understanding of rivers as ecosystems. Geomorphology 89 (1-2), 147–162. doi:10.1016/j.geomorph.2006.07.022
Dyson, M., Bergkamp, G., and Scanlon, J. (2003). Flow: The essentials of environmental flows. Gland, Switzerland and Cambridge, UK: IUCN.
Fremier, A. K., and Strickler, K. M. (2010). Topics in river structure and function. Herndon, VA: American Institute of Biological Sciences.
Frissell, C. A., Liss, W. J., Warren, C. E., and Hurley, M. D. (1986). A hierarchical framework for stream habitat classification: Viewing streams in a watershed context. Environ. Manag. 10 (2), 199–214. doi:10.1007/bf01867358
Gaeuman, D. (2014). High-flow gravel injection for constructing designed in-channel features. River Res. Appl. 30 (6), 685–706. doi:10.1002/rra.2662
Gaeuman, D., and Stewart, R. (2017). Sediment transport in the Trinity River, CA: Data synthesis 2004-2015. Weaverville, CA: Trinity River Restoration Program.
Gaeuman, D. (2020). WY2016-2017 Trinity River gravel augmentation monitoring report. Klamath, CA: Trinity River Restoration Program.
Gilvear, D. J., Greenwood, M. T., Thoms, M. C., and Wood, P. J. (2016). River science: Research and management for the 21st century. Chichester UK: John Wiley & Sons.
Gough, S. A., Som, N. A., Quinn, N. S., Matilton, W. C., Hill, A. M., and Brock, W. (2019). Mainstem Trinity River chinook salmon spawning survey, 2017. Arcata, California: U.S. Fish and Wildlife Service, Arcata Fish and Wildlife Office.
Grams, P. E., Schmidt, J. C., and Topping, D. J. (2007). The rate and pattern of bed incision and bank adjustment on the Colorado River in Glen Canyon downstream from Glen Canyon Dam, 1956-2000. Geol. Soc. Am. Bull. 119 (5-6), 556–575. doi:10.1130/b25969.1
Grill, G., Lehner, B., Thieme, M., Geenen, B., Tickner, D., Antonelli, F., et al. (2019). Mapping the world's free-flowing rivers. Nature 569 (7755), 215–221. doi:10.1038/s41586-019-1111-9
Grimm, N. B., Pickett, S. T. A., Hale, R. L., and Cadenasso, M. L. (2017). Does the ecological concept of disturbance have utility in urban social-ecological-technological systems? Ecosyst. Health Sustain. 3 (1), e01255. doi:10.1002/ehs2.1255
Hall, A. A., Rood, S. B., and Higgins, P. S. (2011). Resizing a river: A downscaled, seasonal flow regime promotes riparian restoration. Restor. Ecol. 19 (3), 351–359. doi:10.1111/j.1526-100X.2009.00581.x
Hardwick, L., and Maquire, J. (2012). “Environmental water needs of the lower murrumbidgee (lowbidgee) floodplain: Discussion paper 1 - approach and ecological considerations,” in NSW department of trade and investment, regional infrastructure and services (Sydney, Australia. www.water.nsw.gov.au.
Hobbs, R. J., Higgs, E., Hall, C. M., Bridgewater, P., Chapin, F. S., Ellis, E. C., et al. (2014). Managing the whole landscape: Historical, hybrid, and novel ecosystems. Front. Ecol. Environ. 12 (10), 557–564. doi:10.1890/130300
Holling, C. S., and Gunderson, L. H. (2002). Panarchy: Understanding transformations in human and natural systems. Washington, DC: Island Press.
Horne, A., Webb, J. A., Stewardson, M., Richter, B., and Acreman, M. (2017). Water for the environment: From policy and science to implementation and management. London UK: Academic Press.
Hulvey, K. B., Standish, R. J., Hallet, L. M., Starzomski, B. M., Murphy, S. D., Nelson, C. R., et al. (2013). “Incorprating novel ecosystems into mangement frameworks,” in Novel Ecosystems: Intervening in the new ecological world order. Editors R. J. Hobbs, E. S. Higgs, and C. M. Hall ((United Kingdom: John Wiley & Sons), 157–171.
Keiser, D. A., and Shapiro, J. S. (2019). Consequences of the clean water act and the demand for water quality. Q. J. Econ. 134 (1), 349–396. doi:10.1093/qje/qjy019
Kelly, J. M., Scarpino, P. V., Berry, H., Syvitski, J., and Meybeck, M. (2018). Rivers of the Anthropocene. Oakland, California: University of California Press.
Kingsford, R., and Thomas, R. F. (2001). “Changing water regimes and wetland habitat on the lower murrumbidgee floodplain of the Murrumbidgee River in arid Australia,” in Report to environment Australia 2001 (Water, Heritage and the Arts): Department of Environment).
Kondolf, G. M., and Minear, J. T. (2004). “Coarse sediment augmentation on the Trinity River below Lewiston Dam: Geomorphic perspectives and review of past projects, final report,” in Trinity River restoration program, weaverville, calif.).
Kondolf, G. M., Piegay, H., and Landon, N. (2002). Channel response to increased and decreased bedload supply from land use change: Contrasts between two catchments. Geomorphology 45 (1-2), 35–51. doi:10.1016/s0169-555x(01)00188-x
Kondolf, G. M. (1997). Profile: Hungry water: Effects of dams and gravel mining on river channels. Environ. Manag. 21, 533–551. doi:10.1007/s002679900048
Lane, E. W. (1955). Design of stable channels. Trans. Am. Soc. Civ. Eng. 120, 1234–1260. doi:10.1061/taceat.0007188
Lehner, B., Liermann, C. R., Revenga, C., Vorosmarty, C., Fekete, B., Crouzet, P., et al. (2011). High-resolution mapping of the world's reservoirs and dams for sustainable river-flow management. Front. Ecol. Environ. 9 (9), 494–502. doi:10.1890/100125
Lisle, T. E., Iseya, F., and Ikeda, H. (1993). Response of a channel with alternate bars to a decrease in supply of mixed-size bed-load - a flume experiment. Water Resour. Res. 29 (11), 3623–3629. doi:10.1029/93wr01673
Maher, P. (1990). Bird survey of the Lachlan/Murrumbidgee confluence wetlands. Australia: Hurseville: NSW National Parks and Wildlife Service.
Meitzen, K. M., Doyle, M. W., Thoms, M. C., and Burns, C. E. (2013). Geomorphology within the interdisciplinary science of environmental flows. Geomorphology 200, 143–154. doi:10.1016/j.geomorph.2013.03.013
Merz, J. E., and Ochikubo Chan, L. K. (2005). Effects of gravel augmentation on macroinvertebrate assemblages in a regulated California river. River Res. Appl. 21 (1), 61–74. doi:10.1002/rra.819
Montgomery, D. R., and Buffington, J. M. (1997). Channel-reach morphology in mountain drainage basins. Geol. Soc. Am. Bull. 109 (5), 596–611. doi:10.1130/0016-7606(1997)109<0596:crmimd>2.3.co;2
Naiman, R. J., Latterell, J. J., Pettit, N. E., and Olden, J. D. (2008). Flow variability and the biophysical vitality of river systems. Comptes Rendus Geosci. 340 (9-10), 629–643. doi:10.1016/j.crte.2008.01.002
Nanson, G. C., and Croke, J. C. (1992). A genetic classification of floodplains. Geomorphology 4 (6), 459–486. doi:10.1016/0169-555x(92)90039-q
Nelson, R. W., Dwyer, J. R., and Greenberg, W. E. (1987). Regulated flushing in a gravel-bed river for channel habitat maintenance: A Trinity River fisheries case study. Environ. Manag. 11 (4), 479–493. doi:10.1007/bf01867656
Ock, G., Gaeuman, D., McSloy, J., and Kondolf, G. M. (2015). Ecological functions of restored gravel bars, the Trinity River, California. Ecol. Eng. 83, 49–60. doi:10.1016/j.ecoleng.2015.06.005
Palmer, M. A., Menninger, H. L., and Bernhardt, E. (2010). river Restoration, habitat heterogeneity and biodiversity: A failure of theory or practice? Freshw. Biol. 55, 205–222. doi:10.1111/j.1365-2427.2009.02372.x
Palmer, M. A., and Ruhi, A. (2019). Linkages between flow regime, biota, and ecosystem processes: Implications for river restoration. Science 365 (6459), eaaw2087. doi:10.1126/science.aaw2087
Parsons, M., Thoms, M. C., Flotemersch, J., and Reid, M. (2016). “Monitoring the resilience of rivers as social-ecological systems: A paradigm shift for river assessment in the twenty-first century,” in River science: Research and management for the 21st century. Editors D. J. Gilvear, M. T. Greenwood, M. C. Thoms, and P. J. Wood (United Kingdom: John Wiley & Sons), 197–220.
Parsons, M., and Thoms, M. C. (2018). From academic to applied: Operationalising resilience in river systems. Geomorphology 305, 242–251. doi:10.1016/j.geomorph.2017.08.040
Petts, G. E., and Gurnell, A. M. (2013). “Hydrogeomorphic effects of reservoirs, dams, and diversions,” in Treatise on geomorphology. Editors J. F. Shroder, L. A. James, C. P. Harden, and J. J. Clague (San Diego: Academic Press, Elsevier Inc.).
Pingram, M., Price, J., and Thoms, M. C. (2019). Integrating multiple aquatic values: Perspectives and a collaborative future for river science. River Res. Appl. 35 (10), 1607–1614. doi:10.1002/rra.3562
Poff, N. L., Allan, J. D., Bain, M. B., Karr, J. R., Prestegaard, K. L., Richter, B. D., et al. (1997). The natural flow regime. Bioscience 47 (11), 769–784. doi:10.2307/1313099
Poff, N. L., and Matthews, J. H. (2013). Environmental flows in the anthropocence: Past progress and future prospects. Curr. Opin. Environ. Sustain. 5 (6), 667–675. doi:10.1016/j.cosust.2013.11.006
Poff, N. L., Richter, B. D., Arthington, A. H., Bunn, S. E., Naiman, R. J., Kendy, E., et al. (2010). The ecological limits of hydrologic alteration (ELOHA): A new framework for developing regional environmental flow standards. Freshw. Biol. 55 (1), 147–170. doi:10.1111/j.1365-2427.2009.02204.x
Reid, A. J., Carlson, A. K., Creed, I. F., Eliason, E. J., Gell, P. A., Johnson, P. T. J., et al. (2019). Emerging threats and persistent conservation challenges for freshwater biodiversity. Biol. Rev. 94 (3), 849–873. doi:10.1111/brv.12480
Scheffer, M. (2009). Critical transitions in nature and society. Princeton: Princeton University Press.
Schumm, S. A. (1985). Patterns of alluvial rivers. Annu. Rev. Earth Planet. Sci. 13 (1), 5–27. doi:10.1146/annurev.ea.13.050185.000253
Scown, M. W., Thoms, M. C., and De Jager, N. R. (2016a). An index of floodplain surface complexity. Hydrol. Earth Syst. Sci. 20 (1), 431–441. doi:10.5194/hess-20-431-2016
Scown, M. W., Thoms, M. C., and De Jager, N. R. (2016b). “Measuring spatial patterns in floodplains: A step towards understanding the complexity of floodplain ecosystems,” in River science: Research and management for the 21st century. Editors D. J. Gilvear, M. T. Greenwood, M. C. Thoms, and P. J. Wood (United Kingdom: John Wiley & Sons), 103–131.
Shilpakar, R. L. (2013). Floodplain vegetation landscapes: An ecotone or patch mosaic. Armidale, Australia: PhD, University of New England.
Shilpakar, R. L., Thoms, M. C., and Reid, M. A. (2021). The resilience of a floodplain vegetation landscape. Landsc. Ecol. 36 (1), 139–157. doi:10.1007/s10980-020-01127-0
Shilpakar, R. L., Thoms, M. C., and Scown, M. (Forthcoming 2022). The spatial and temporal character of an inundated floodplain landscape. Water Resour. Res.
Simon, A., Doyle, M., Kondolf, M., Shields, F. D., Rhoads, B., and McPhillips, M. (2007). Critical evaluation of how the Rosgen classification and associated "natural channel design" methods fail to integrate and quantify fluvial processes and channel response. J. Am. Water Resour. Assoc. 43 (5), 1117–1131. doi:10.1111/j.1752-1688.2007.00091.x
Thapa, R., Thoms, M. C., Parsons, M., and Reid, M. (2016). Adaptive cycles of floodplain vegetation response to flooding and drying. Earth Surf. Dynam. 4 (1), 175–191. doi:10.5194/esurf-4-175-2016
Thapa, R., Thoms, M. C., Reid, M., and Parsons, M. (2020). Do adaptive cycles of floodplain vegetation response to inundation differ among vegetation communities? River Res. Appl. 36 (4), 553–566. doi:10.1002/rra.3538
Thoms, M. C., Beyer, P. J., and Rogers, K. H. (2006). “Variability, complexity and diversity: The geomorphology of river ecosystems in dryland regions,” in Ecology of desert rivers. Editor R. Kingsford (Cambridge UK: Cambridge University Press), 47–75.
Thoms, M. C., Delong, M. D., Flotemersch, J. E., and Collins, S. E. (2017). Physical heterogeneity and aquatic community function in river networks: A case study from the kanawha river basin, USA. Geomorphology 290, 277–287. doi:10.1016/j.geomorph.2017.02.027
Thoms, M. C. (2003). Floodplain-river ecosystems: Lateral connections and the implications of human interference. Geomorphology 56 (3-4), 335–349. doi:10.1016/s0169-555x(03)00160-0
Thoms, M. C., and Parsons, M. (2016). “The physical environment of Australian floodplain ecosystems,” in Australian floodplain vegetation. Editors S. Capon, and M. A. Reid (Canberra, Australia: CSIRO).
Thoms, M. C., Piegay, H., and Parsons, M. (2018). What do you mean, 'resilient geomorphic systems. Geomorphology 305, 8–19. doi:10.1016/j.geomorph.2017.09.003
Thoms, M. C., Rose, T., and Dyer, F. (2020). Riverine landscapes, water resource development and management: A view from downunder. River Res. Appl. 36 (4), 505–511. doi:10.1002/rra.3622
Thoms, M. C., and Sheldon, F. (2000). Lowland rivers: An Australian introduction. Regul. Rivers Res. Mgmt. 16 (5), 375–383. doi:10.1002/1099-1646(200009/10)16:5<375::Aid-rrr591>3.0.Co;2-#
Thoms, M. C. (2006). Variability in riverine ecosystems. River Res. Appl. 22 (2), 115–121. doi:10.1002/rra.900
Thorp, J. H., Thoms, M. C., and Delong, M. D. (2006). The riverine ecosystem synthesis: Biocomplexity in river networks across space and time. River Res. Appl. 22 (2), 123–147. doi:10.1002/rra.901
Tickner, D., Kaushal, N., Speed, R., and Tharme, R. (2020). Editorial: Implementing environmental flows: Lessons for policy and practice. Front. Environ. Sci. 8. doi:10.3389/fenvs.2020.00106
TNC (2018). Environmental flow concepts [online]. The nature conservancy. San Francisco, CA. Available: http://www.conservationgateway.org/ConservationPractices/Freshwater/EnvironmentalFlows/Concepts/Pages/environmental-flows-conce.aspx (Accessed June 25, 2021 2021).
Tockner, K., and Stanford, J. A. (2002). Riverine flood plains: Present state and future trends. Environ. Conserv. 29 (3), 308–330. doi:10.1017/s037689290200022x
Trrp, (2009). Conceptual models and hypotheses for the Trinity River restoration program. Weaverville, CA: U.S. Dept of Interior, Bureau of Reclamation.
Turner, W., Spector, S., Gardiner, N., Fladeland, M., Sterling, E., and Steininger, M. (2003). Remote sensing for biodiversity science and conservation. Trends Ecol. Evol. 18 (6), 306–314. doi:10.1016/s0169-5347(03)00070-3
USFWS and HVT U.S Fish and Wildlife Service and Hoopa Valley Tribes (1999). Trinity River flow evaluation final report". Trinity river restoration program. Weaverville, CA.
van Rees, C. B., Waylen, K. A., Schmidt-Kloiber, A., Thackeray, S. J., Kalinkat, G., Martens, K., et al. (2021). Safeguarding freshwater life beyond 2020: Recommendations for the new global biodiversity framework from the European experience. Conserv. Lett. 14 (1). doi:10.1111/conl.12771
Vitousek, P. M., Mooney, H. A., Lubchenco, J., and Melillo, J. M. (1997). Human domination of Earth's ecosystems. Science 277 (5325), 494–499. doi:10.1126/science.277.5325.494
Vorosmarty, C. J., McIntyre, P. B., Gessner, M. O., Dudgeon, D., Prusevich, A., Green, P., et al. (2010). Global threats to human water security and river biodiversity. Nature 467 (7315), 555–561. doi:10.1038/nature09440
Ward, J. V., and Stanford, J. A. (1995). Ecological connectivity in alluvial river ecosystems and its disruption by flow regulation. Regul. Rivers Res. Mgmt. 11 (1), 105–119. doi:10.1002/rrr.3450110109
Ward, J. V., Tockner, K., and Schiemer, F. (1999). Biodiversity of floodplain river ecosystems: Ecotones and connectivity1. Regul. Rivers Res. Mgmt. 15 (1-3), 125–139. doi:10.1002/(sici)1099-1646(199901/06)15:1/3<125::aid-rrr523>3.0.co;2-e
Wassens, S., Arnaiz, O., Healy, S., Watts, R., and Maguire, J. (2008). Identification of hydrological habitat requirements to maintain viable southern bell frog (Litoria raniformis) populations on the Lowbidgee floodplain - phase 1. NSW): Institute for Land, Water and Society, Charles Strurt University.
Wen, L., Ling, J., Saintilan, N., and Rogers, K. (2009). An investigation of the hydrological requirements of river red gum (Eucalyptus camaldulensis) forest, using classification and regression tree modelling. Ecohydrology 2 (2), 143–155. doi:10.1002/eco.46
Wohl, E., Bledsoe, B. P., Jacobson, R. B., Poff, N. L., Rathburn, S. L., Walters, D. M., et al. (2015). The natural sediment regime in rivers: Broadening the foundation for ecosystem management. BioScience 65, 358–371. doi:10.1093/biosci/biv002
Keywords: functional flows, floodplain function, sediment augmentation, channel restoration, climate resilience
Citation: Yarnell SM and Thoms M (2022) Enhancing the functionality of environmental flows through an understanding of biophysical processes in the riverine landscape. Front. Environ. Sci. 10:787216. doi: 10.3389/fenvs.2022.787216
Received: 30 September 2021; Accepted: 14 September 2022;
Published: 21 October 2022.
Edited by:
Kate Rowntree, Rhodes University, South AfricaReviewed by:
Joris Eekhout, Center for Edaphology and Applied Biology of Segura (CSIC), SpainGisela Mayora, National Institute of Limnology (INALI), Argentina
Copyright © 2022 Yarnell and Thoms. This is an open-access article distributed under the terms of the Creative Commons Attribution License (CC BY). The use, distribution or reproduction in other forums is permitted, provided the original author(s) and the copyright owner(s) are credited and that the original publication in this journal is cited, in accordance with accepted academic practice. No use, distribution or reproduction is permitted which does not comply with these terms.
*Correspondence: Sarah M. Yarnell, smyarnell@ucdavis.edu