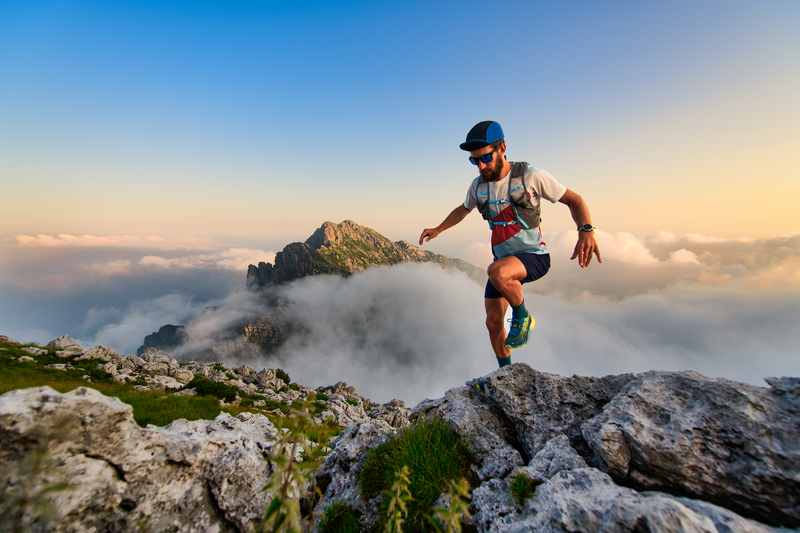
94% of researchers rate our articles as excellent or good
Learn more about the work of our research integrity team to safeguard the quality of each article we publish.
Find out more
BRIEF RESEARCH REPORT article
Front. Environ. Sci. , 05 January 2023
Sec. Toxicology, Pollution and the Environment
Volume 10 - 2022 | https://doi.org/10.3389/fenvs.2022.1107005
In a greenhouse experiment, silver nanoparticles (Ag-NPs) were applied on European beech (Fagus sylvatica L.) leaves using the droplet application method. Scanning electron microscopy (SEM) analyses showed that after 24 h silver nanoparticles were mostly present in aggregates or as single particles on the surface of the leaf, surrounding or covering the stomata. Analyses of cross sections of the leaf revealed that some silver nanoparticles were adhering to the cell walls of the mesophyll and palisade cells, most likely after penetration into the leaf through the stomata as particles and not as Ag ions. Our preliminary results showed evidence of foliar uptake of silver nanoparticles in European beech. This opens new insights on the ability of trees to take up solid nanosized particles, eventually contained in raindrops, through their leaves, and potentially transport them to other parts of the tree. This study would be helpful for investigating the role of trees in atmospheric ultrafine particle mitigation.
Plants exposed to airborne ultrafine particulate matter (PM) can take it up. This can have dangerous but also useful effects. For example, edible plants can be contaminated by the heavy metals contained in the PM so that particles enter the food chain. These ultrafine particles can be directly deposited onto the leaves of plants, through wet or dry deposition (Luo et al., 2019), and eventually penetrate from the leaf surface into the internal tissues mainly through the stomatal pathway (Lv et al., 2019). Atmospheric fallouts of lead nanoparticles (NPs) were found in leaves of lettuce near a lead-recycling plant (Uzu et al., 2010), confirming once more that atmospheric contamination in edible plants is a major issue. Trees have been used for phytoremediation of contaminated soils (Shah and Daverey, 2020), water and atmosphere (Wei et al., 2021). Engineered NPs (with at least one dimension less than 100 nm) can have the same geometrical shape and dynamic effects of ultrafine PM particles, e.g., PM1 or PM2.5 of urban road dust, and they can be absorbed into plants by foliar and root uptake (Ha et al., 2021).
Less explored than the soil-root uptake and transport of NPs, their adhesion on leaves and consequent foliar uptake have been confirmed in experiments on annual plants. Such studies reported that NPs were mainly absorbed through stomatal pathways as determined for fluorescent polystyrene particles (43 nm in diameter) in leek and broad bean (Eichert et al., 2008), of liposome-NPs in cherry-tomato plants (Karny et al., 2018), of gold NPs (Au-NPs) in wheat (Avellan et al., 2019) and perilla (Ha et al., 2021). Root and leaf uptake and transport of NPs have also been demonstrated in trees (e.g., Cocozza et al., 2019; Rossi et al., 2019; Ballikaya et al., 2022a), but what happens to the NPs in trees is still under debate (see Ballikaya et al., 2022b). Using 3D chemical tomography, our group could confirm (Ballikaya et al., 2022a) the leaf uptake of Au-NPs sprayed onto the leaves of European beech by identifying single Au-NPs inside the leaf structure and inside a trichome (leaf hair). Similarly, silver NPs (Ag-NPs) were detected within a leaf of a clementine mandarin tree by microscopic hyperspectral imaging, after branch immersion in an Ag-NP suspension for 24 h (Su et al., 2020). TiO2 particles (400–500 nm) were observed by transmission electron microscopy (TEM) inside Betula pendula leaves exposed to the particles at wind speed for 2 h (Räsänen et al., 2017). However, the exact mechanisms of leaf uptake are not yet understood and evidence of metal NPs inside leaf structures of trees is scarce.
In a pilot greenhouse experiment, we aimed at investigating the foliar uptake of Ag-NPs in European beech (Fagus sylvatica L.), the most common native, broadleaf species of European forests, which was recently proven to efficiently take up metal NPs such as Au-NPs through the leaves and transport them to stem and roots 20 days after the treatment (Ballikaya et al., 2022a). In this study, we explored if the foliar uptake already occurred 24 h after the Ag-NP exposure and if Ag was detectable in its nanoparticulate form inside the leaves of beech using scanning electron microscopy (SEM) and energy dispersive X-ray (EDX) analyses. Ag-NPs were used to simulate deposition of raindrops containing ultrafine particles on tree leaves, similar as previously done with Au-NPs on a mint-like edible plant (Ha et al., 2021). Ag-NPs were chosen because they are easy to track in complex plant matrix since background Ag concentrations are absent or low. Moreover, the foliar uptake of Ag-NPs in trees can have important environmental implications because trees could mitigate the hazardous increase of Ag-NP emissions into the environment (Giese et al., 2018), driven by high demand of Ag-NPs in many fields of application, e.g., as antibacterial, antifungal, anti-cancer agents, textiles, bio-sensing, imaging, water treatment, cosmetics (Zhang et al., 2016). Airborne Ag-NPs are considered of medium-high concern due to their ability to penetrate deep into human lungs (European Commission, 2013) as well as to induce changes in the morphology of plants after their exposure (Yan and Chen, 2019).
Two healthy 3-year old European beech seedlings were kept in a greenhouse under natural conditions. The trees were growing in pots filled with mixed soil substrate (“Containererde,” Ökohum GmbH, Switzerland) from February to July 2020, until fully expanded leaves were formed. The trees were regularly watered, and no fertilizer was added.
Biopure™ 40 nm spherical citrate coated Ag-NPs (1 mg mL−1) were obtained from NanoComposix (San Diego CA, United States.). Ag-NP suspensions (200 mg L−1) were prepared in MilliQ water and dispersed by ultrasonication for 3 min in order to improve the homogeneity and stability of the suspension. We selected the most inert form of Ag-NPs, i.e., citrate coated, following manufacturer’s instructions for the exposure to minimize chemical reactions on leaf surfaces and toxicity to plants.
Droplets of Ag-NP suspensions were then pipetted on the leaves of beech (Figure 1A), while the air humidity was set to 90%–100% to ensure stomatal opening. The soil was covered with Parafilm® to avoid contamination. Twenty droplets (a total volume of approximately 20 µL) were carefully applied on the abaxial (lower) side of each leaf, where the stomata are present (Van Wittenberghe et al., 2012), following the midrib and a lateral vein (Figures 1A, B). Similarly, Ag-NP-free droplets (only ultrapure water without any NPs) were applied on leaves of beech trees as a control. The droplet application method was chosen because the wet deposition increases the residence time, retention amount, and influx of NPs to plants compared with dry deposition, which is mainly driven by gravitational sedimentation and weak electrostatic force near the leaf surface (Ha et al., 2021).
FIGURE 1. (A) Representation of the application method where droplets of Ag-NPs were applied following the mid rib and a lateral vein of the lower leaf side of beech. (B) A flat area containing a drop of Ag-NPs and a cross section were considered for the SEM analyses. The cross section was done by cutting through a drop of Ag-NPs from the upper to the lower side of the leaf to avoid contamination.
Twenty-four hours after the exposure, one randomly selected leaf was removed, dissected and investigated natively using SEM (Zeiss GeminiSEM 450) equipped with an X-ray energy dispersive spectrometer (X-MAX80, AZTec Advanced, Oxford) for elemental analysis, as follows. A flat area (approx. 1 × 1 mm in size) and a cross section of unwashed leaf were neatly cut around and in the middle of the dried droplet, respectively, using a sharp blade. The cut was carefully made from the adaxial (upper) side, free of Ag-NPs, to the abaxial side of the leaf taking special care to avoid smearing the Ag-NPs along the cross section (Figure 1B). We did not wash the leaf because we wanted to easily identify the area where the Ag-NP droplet was applied and to avoid the risk of spreading the Ag-NPs to uncontaminated leaf areas, e.g., adaxial side, that would have caused more methodological complications. The leaf specimens (flat area and cross section) met the main requirements, that are a) the surface to observe was clearly visible, b) the specimens were firmly fixed to the specimen mount using double sided conductive sticky tabs. Thus, the leaf specimens were processed without any further treatment (e.g., without being dehydrated nor stained with gold) in order to avoid contamination or removal of the Ag-NPs from the leaf surface and internal structures. One leaf sample per tree was analyzed at an acceleration voltage of .8 keV and a beam current of 200 pA. Images were acquired with a SE2 detector. EDX spectra were recorded at an acceleration voltage of 10 keV. All microscopic investigations were performed at the Center for Microscopy and Image Analysis, University of Zurich (Switzerland).
Ag-NPs were identified on the lower leaf surface and on a cross section. After 24 h of exposure, SEM images showed the spatial distribution of the Ag-NPs on the leaf surface after the evaporation of the droplet (Figure 2A). High concentration of Ag-NPs in suspension (200 mg L−1) caused the formation of large areas of particle clusters visible on the leaf surface, partially or completely covering the cuticle and the stomata. The used NP concentration in this study was not representative of current or expected concentrations of Ag-NP in nature, but rather to assure higher chances to detect the NPs in the leaf tissues. Nevertheless, future concentrations for silicon dioxide (SiO2), cerium dioxide (CeO2) and Ag engineered NPs were predicted to increase from pg L−1 to ng L−1 until 2050, with relatively low impact on water, air and soil, but unknown for organisms living close to NP point sources (Giese et al., 2018).
FIGURE 2. (A) Presence of Ag-NPs on the abaxial side of a leaf after droplet deposition. (B) Element analysis of the particles using energy-dispersive X-ray spectroscopy confirmed the presence of Ag-NPs (in aggregates and single particles) observed around the magnified stoma in the yellow box. Scale bars: (A) 4 µm and (B) 1 µm.
X-ray elemental analysis confirmed the presence of Ag-NPs close to the stomatal aperture in aggregates mixed with salts from the dried droplet (see analysis spot of spectrum four in Figure 2B) or single particles (see analysis spot of spectrum five in Figure 2B). Other elements, such as O, C, Ca, Na, Mg, Si, S, K, could have originated from the leaf sample or the chemical composition of the Ag-NP suspension (e.g., high signal from Na, C and O likely originated from the sodium citrate solution containing the Ag-NPs).
Tree leaf morphology and, thus, the leaf surface, is characterized by several macro- and micro features that can affect the NP adhesion (i.e., the ability of the NPs to remain on the plant surface after wet deposition) and uptake (Schwab et al., 2016). NP size and surface properties can interact with specific compounds of the foliage surface (e.g., proteins, glucosides, waxes), as well as with leaf hydrophobicity and surface roughness, mostly preventing the NPs to penetrate internal tissues of the leaf (Avellan et al., 2021). Moreover, the physical dynamics (e.g., sedimentation, evaporation, shrinkage of drop interface) of NPs in a non-movable droplet, i.e., sessile drop, can affect the absorption of the NPs, as well described in Ha et al. (2021). These considerations can explain the reason of high aggregation of Ag-NPs on the surface of the treated beech leaf.
Following deposition and adhesion onto the leaf surface, a fraction of spherical particles were found on the cell wall of the lower epidermis in the magnified area of the orange box in Figure 3B and confirmed to be Ag-NPs by X-ray elemental analysis (see analysis spots spectrum five and six in Figure 3A). Thus, Ag penetrated the leaf tissues in its original nanoparticulate form and not as dissolved ions. Possible biotransformation of Ag-NPs into ionic Ag within the leaf, after their penetration, could still be possible, as previously documented in lettuce by Larue et al. (2014) and as observed for other NPs such as copper (Cu)O NPs (Xiong et al., 2017) and SiO2 NPs (El-Shetehy et al., 2021). Given the size of our Ag-NPs, i.e., 40 nm, penetration through the cuticle can be excluded as the size exclusion limit to enter the cuticular pathway is estimated to be smaller than 5 nm (Eichert et al., 2008). We can also convincingly exclude that contamination has occurred during handling the sample and cutting the cross section, because of the accuracy of the sectioning method described above. Thus, the stomatal pathway seems to be the main route for the Ag-NPs internalization, probably caused by diffusion of solutes or suspended particles in water adsorbed to the walls of the stomatal opening (Eichert et al., 2008), which was likely favored by high humidity conditions during the experiment. As reported by Eichert et al. (2008), the stomatal uptake can start immediately and persist as long as the droplets are not completely dried out. We thus believe that some Ag-NPs penetrated the stomata the moment the droplets were applied until they dried out (approx. 20–25 min after the drop application), while the majority of Ag-NPs that were not directly on the stomata or were not moved by internal drop flows adhered to the leaf surface. Since ours was an acute application of a highly concentrated Ag-NP solution, repeated incubations or steps that would maintain the solubility of the droplets for longer time could produce a higher entry of nanoparticles. Ha et al. (2021) have already been showing a dynamic behavior of Au-NPs in a sessile drop, moving toward the contact lines of the drop on the leaf surface by evaporation-driven internal flow. Thus, in our case, stomata positioned near the contact lines were more likely infiltrated by Ag-NPs in suspension too. However, stomatal uptake of NPs is limited by the stomatal aperture size (generally higher in coniferous than in broadleaved species), stomatal number density (in beech approx. 420 mm−2; Van Wittenberghe et al., 2012) and stomatal opening cycle. Differences in coating materials can also affect the uptake and transport of Ag-NPs. Su et al. (2020) mentioned that gum Arabic coated Ag-NPs, compared to citrate coated, were highly effective in inhibiting the aggregation of NPs in synthetic sap and enhancing the mobility of NPs in trees. On the contrary, it was shown that Au-NP transport from leaves to roots was not affected by different surface coatings (Avellan et al., 2019; Ballikaya et al., 2022a), but adhesion on the leaf surface was increased for citrate Au-NPs compared to polyvinylpyrrolidone (PVP) Au-NPs, with some uptake potentially preferentially occurring through the stomata (Avellan et al., 2019). However, after entering the plant leaf, PVP Au-NPs remained trapped in the leaf mesophyll, but the citrate Au-NPs were not, likely due to differences in coating affinity with cell structures and macromolecules. In our study, we were not able to test and compare the influence of different coatings on the NP uptake and transport, but we do not exclude possible effects of the citrate coating as described in previous studies, i.e., less uptake, more aggregation, higher transport than seen with other surface coatings.
FIGURE 3. (A) Element analysis of the particles using X-ray energy-dispersive spectrometer confirmed the presence of the Ag-NPs on internal structures of the leaf; the stripes in the image are charging artefacts of this location. (B) Magnified cross section of leaf showing different anatomical structures: l: lower epidermis; m: spongy mesophyll; v: vein (xylem and phloem); p: palisade mesophyll; u: upper epidermis. (C) Yellow arrows indicate aggregates of Ag-NPs (details in magnified white box) adhering on the cell wall of the spongy mesophyll cells under the lower epidermis (magnified area in yellow box). (D) Red arrows indicate single and aggregates of Ag-NPs (details in magnified white box) adhering on the cell wall of the palisade parenchyma under the upper epidermis (magnified area in red box). Scale bars: (A, B) 10 μm; (C) 500 nm; detail in (C) 200 nm; (D) 500 nm; detail in (D) 200 nm.
Observing the leaf cross section (Figure 3B), aggregates of Ag-NPs were found adhering onto cell walls of the spongy mesophyll close to the lower side of the leaf surface where stomata were located just below the drop deposition (Figure 3C). Similar findings were reported by Räsänen et al. (2017). The authors observed presence of TiO2 particles aggregated in the intercellular space or on the cell walls of spongy mesophyll cells in leaves of B. pendula but not in Betula pubescens, likely due to species-related characteristics. We also found Ag-NPs, as single particles or as aggregates, on the palisade mesophyll cells closer to the upper epidermis (Figure 3D). As previously speculated by Su et al. (2020), an apoplastic movement of the NPs could have occurred via the mesophyll cells along the leaf cross section due to diffusion of assimilated sugars from photosynthesis in mesophyll cells, and then eventually transported into the phloem, or due to coating affinity with cell structures which could have moved the NPs at a high rate at the early time of exposure, as demonstrated in Avellan et al. (2019). The transport mechanisms at cellular level are still not well understood and many pathways of NP translocation are possible, such as through passive diffusion (polar paths) or facilitated transport via receptor binding and endocytosis (Schwab et al., 2016). Penetration of various fluorescent NPs (<6 nm in size) into the cell wall and movement into the phloem was observed in citrus leaves, although only after assisted penetration through superficial perforations in the cuticle created by laser light beam (Etxeberria et al., 2016).
Because future concentrations of Ag-NPs are predicted to increase in all environmental compartments, with special concerns for water and atmosphere (Giese et al., 2018) that would directly affect human health as well as vegetation, more research should be done on the interaction of Ag-NPs with trees, which can act as bio-accumulators of NPs and improve air quality (Ballikaya et al., 2022b). Particular attention should be given to the foliar uptake because leaves are the direct organs that interact with atmospheric emissions of NPs. Future research should invest in exploring the phytotoxicity of Ag-NPs in plants too, because effects of Ag-NPs in high concentrations were observed to cause oxidative stress and reduction of biomass in annual plants (e.g., Cvjetko et al., 2017; Yan and Chen, 2019), as well as in trees (Sweet and Singleton, 2015).
Our findings are a good indication that Ag-NPs can enter into the leaves of European beech. This is the first preliminary study that identified, imaged by SEM, the presence of metal NPs inside of a beech leaf, 24 h after the NP exposure Moreover, our results showed that the detected Ag was in its original nanoparticulate form, which excludes dissolution into Ag ions at the moment of foliar uptake. Thus, our preliminary results provide valuable insights on the ability of trees to take up solid nanosized particles through their leaves and potentially transport them to other parts of the tree.
The raw data supporting the conclusion of this article will be made available by the authors, without undue reservation.
PB, IB, and PC contributed to conception and design of the study. PB performed the experiment. PB, JM, and AK performed the analyses and interpreted the data. PB prepared the figures and wrote the first draft of the manuscript. All authors contributed to manuscript revision, read, and approved the submitted version.
The study and the analyses were supported by the Swiss National Science Foundation (SNSF, 200021_182042). Open access funding provided by WSL—Swiss Federal Institute For Forest, Snow And Landscape Research
The authors would like to thank the staff and facility of the Center for Microscopy and Image Analysis of the University of Zurich where the microscopic analysis was performed.
The authors declare that the research was conducted in the absence of any commercial or financial relationships that could be construed as a potential conflict of interest.
All claims expressed in this article are solely those of the authors and do not necessarily represent those of their affiliated organizations, or those of the publisher, the editors and the reviewers. Any product that may be evaluated in this article, or claim that may be made by its manufacturer, is not guaranteed or endorsed by the publisher.
Avellan, A., Yun, J., Morais, B. P., Clement, E. T., Rodrigues, S. M., and Lowry, G. V. (2021). Critical review: Role of inorganic nanoparticle properties on their foliar uptake and in planta translocation. Environ. Sci. Technol. 55 (20), 13417–13431. doi:10.1021/acs.est.1c00178
Avellan, A., Yun, J., Zhang, Y., Spielman-Sun, E., Unrine, J. M., Thieme, J., et al. (2019). Nanoparticle size and coating chemistry control foliar uptake pathways, translocation, and leaf-to-rhizosphere transport in wheat. ACS Nano 13 (5), 5291–5305. doi:10.1021/acsnano.8b09781
Ballikaya, P., Brunner, I., Cocozza, C., Grolimund, D., Kaegi, R., Murazzi, M. E., et al. (2022a). First evidence of nanoparticle uptake through leaves and roots in beech (Fagus sylvatica L.) and pine (Pinus sylvestris L.). Tree Physiol., tpac117. tpac117. doi:10.1093/treephys/tpac117
Ballikaya, P., Marshall, J., and Cherubini, P. (2022b). Can tree-ring chemistry be used to monitor atmospheric nanoparticle contamination over time? Atmos. Environ. 268, 118781. doi:10.1016/j.atmosenv.2021.118781
Cocozza, C., Perone, A., Giordano, C., Salvatici, M. C., Pignattelli, S., Raio, A., et al. (2019). Silver nanoparticles enter the tree stem faster through leaves than through roots. Tree Physiol. 39 (7), 1251–1261. doi:10.1093/treephys/tpz046
Cvjetko, P., Milošić, A., Domijan, A. M., Vrček, I. V., Tolić, S., Štefanić, P. P., et al. (2017). Toxicity of silver ions and differently coated silver nanoparticles in Allium cepa roots. Ecotoxicol. Environ. Saf. 137, 18–28. doi:10.1016/j.ecoenv.2016.11.009
Eichert, T., Kurtz, A., Steiner, U., and Goldbach, H. E. (2008). Size exclusion limits and lateral heterogeneity of the stomatal foliar uptake pathway for aqueous solutes and water-suspended nanoparticles. Physiol. Plant. 134 (1), 151–160. doi:10.1111/j.1399-3054.2008.01135.x
El-Shetehy, M., Moradi, A., Maceroni, M., Reinhardt, D., Petri-Fink, A., Rothen-Rutishauser, B., et al. (2021). Silica nanoparticles enhance disease resistance in Arabidopsis plants. Nat. Nanotechnol. 16 (3), 344–353. doi:10.1038/s41565-020-00812-0
Etxeberria, E., Gonzalez, P., Bhattacharya, P., Sharma, P., and Ke, P. C. (2016). Determining the size exclusion for nanoparticles in citrus leaves. HortScience 51 (6), 732–737. doi:10.21273/HORTSCI.51.6.732
European Commission (2013). “Guidance for employers and health and safety practitioners - guidance on the protection of the health and safety of workers from the potential risks related to nanomaterials at work,” in Employment, social affairs & inclusion, 1–63. page 23.
Giese, B., Klaessig, F., Park, B., Kaegi, R., Steinfeldt, M., Wigger, H., et al. (2018). Risks, release and concentrations of engineered nanomaterial in the environment. Sci. Rep. 8 (1), 1565–1618. doi:10.1038/s41598-018-19275-4
Ha, N., Seo, E., Kim, S., and Lee, S. J. (2021). Adsorption of nanoparticles suspended in a drop on a leaf surface of Perilla frutescens and their infiltration through stomatal pathway. Sci. Rep. 11 (1), 7589–7613. doi:10.1038/s41598-021-91073-x
Karny, A., Zinger, A., Kajal, A., Shainsky-Roitman, J., and Schroeder, A. (2018). Therapeutic nanoparticles penetrate leaves and deliver nutrients to agricultural crops. Sci. Rep. 8 (1), 1–10. doi:10.1038/s41598-018-25197-y
Larue, C., Castillo-Michel, H., Sobanska, S., Cécillon, L., Bureau, S., Barthès, V., et al. (2014). Foliar exposure of the crop Lactuca sativa to silver nanoparticles: Evidence for internalization and changes in Ag speciation. J. Hazard. Mater. 264, 98–106. doi:10.1016/j.jhazmat.2013.10.053
Luo, X., Bing, H., Luo, Z., Wang, Y., and Jin, L. (2019). Impacts of atmospheric particulate matter pollution on environmental biogeochemistry of trace metals in soil-plant system: A review. Environ. Pollut. 255, 113138. doi:10.1016/j.envpol.2019.113138
Lv, J., Christie, P., and Zhang, S. (2019). Uptake, translocation, and transformation of metal-based nanoparticles in plants: Recent advances and methodological challenges. Environ. Sci. Nano 6 (1), 41–59. doi:10.1039/C8EN00645H
Räsänen, J. V., Leskinen, J. T., Holopainen, T., Joutsensaari, J., Pasanen, P., and Kivimäenpää, M. (2017). Titanium dioxide (TiO2) fine particle capture and BVOC emissions of Betula pendula and Betula pubescens at different wind speeds. Atmos. Environ. 152, 345–353. doi:10.1016/j.atmosenv.2017.01.003
Rossi, L., Fedenia, L. N., Sharifan, H., Ma, X., and Lombardini, L. (2019). Effects of foliar application of zinc sulfate and zinc nanoparticles in coffee (Coffea arabica L.) plants. Plant Physiology Biochem. 135, 160–166. doi:10.1016/j.plaphy.2018.12.005
Schwab, F., Zhai, G., Kern, M., Turner, A., Schnoor, J. L., and Wiesner, M. R. (2016). Barriers, pathways and processes for uptake, translocation and accumulation of nanomaterials in plants–Critical review. Nanotoxicology 10 (3), 257–278. doi:10.3109/17435390.2015.1048326
Shah, V., and Daverey, A. (2020). Phytoremediation: A multidisciplinary approach to clean up heavy metal contaminated soil. Environ. Technol. Innovation 18, 100774. doi:10.1016/j.eti.2020.100774
Su, Y., Ashworth, V. E., Geitner, N. K., Wiesner, M. R., Ginnan, N., Rolshausen, P., et al. (2020). Delivery, fate, and mobility of silver nanoparticles in citrus trees. ACS Nano 14 (3), 2966–2981. doi:10.1021/acsnano.9b07733
Sweet, M. J., and Singleton, I. (2015). Soil contamination with silver nanoparticles reduces Bishop pine growth and ectomycorrhizal diversity on pine roots. J. Nanoparticle Res. 17 (11), 448. doi:10.1007/s11051-015-3246-4
Uzu, G., Sobanska, S., Sarret, G., Munoz, M., and Dumat, C. (2010). Foliar lead uptake by lettuce exposed to atmospheric fallouts. Environ. Sci. Technol. 44 (3), 1036–1042. doi:10.1021/es902190u
Van Wittenberghe, S., Adriaenssens, S., Staelens, J., Verheyen, K., and Samson, R. (2012). Variability of stomatal conductance, leaf anatomy, and seasonal leaf wettability of young and adult European beech leaves along a vertical canopy gradient. Trees 26 (5), 1427–1438. doi:10.1007/s00468-012-0714-7
Wei, Z., Van Le, Q., Peng, W., Yang, Y., Yang, H., Gu, H., et al. (2021). A review on phytoremediation of contaminants in air, water and soil. J. Hazard. Mater. 403, 123658. doi:10.1016/j.jhazmat.2020.123658
Xiong, T., Dumat, C., Dappe, V., Vezin, H., Schreck, E., Shahid, M., et al. (2017). Copper oxide nanoparticle foliar uptake, phytotoxicity, and consequences for sustainable urban agriculture. Environ. Sci. Technol. 51 (9), 5242–5251. doi:10.1021/acs.est.6b05546
Yan, A., and Chen, Z. (2019). Impacts of silver nanoparticles on plants: A focus on the phytotoxicity and underlying mechanism. Int. J. Mol. Sci. 20 (5), 1003. doi:10.3390/ijms20051003
Keywords: airborne ultrafine particles, trees, European beech, drop application, leaf uptake, stomatal pathway
Citation: Ballikaya P, Mateos JM, Brunner I, Kaech A and Cherubini P (2023) Detection of silver nanoparticles inside leaf of European beech (Fagus sylvatica L.). Front. Environ. Sci. 10:1107005. doi: 10.3389/fenvs.2022.1107005
Received: 24 November 2022; Accepted: 21 December 2022;
Published: 05 January 2023.
Edited by:
Xue Bai, Hohai University, ChinaReviewed by:
Abhay Kumar, University of Tuscia, ItalyCopyright © 2023 Ballikaya, Mateos, Brunner, Kaech and Cherubini. This is an open-access article distributed under the terms of the Creative Commons Attribution License (CC BY). The use, distribution or reproduction in other forums is permitted, provided the original author(s) and the copyright owner(s) are credited and that the original publication in this journal is cited, in accordance with accepted academic practice. No use, distribution or reproduction is permitted which does not comply with these terms.
*Correspondence: Paula Ballikaya, cGF1bGEuYmFsbGlrYXlhQHdzbC5jaA==
Disclaimer: All claims expressed in this article are solely those of the authors and do not necessarily represent those of their affiliated organizations, or those of the publisher, the editors and the reviewers. Any product that may be evaluated in this article or claim that may be made by its manufacturer is not guaranteed or endorsed by the publisher.
Research integrity at Frontiers
Learn more about the work of our research integrity team to safeguard the quality of each article we publish.