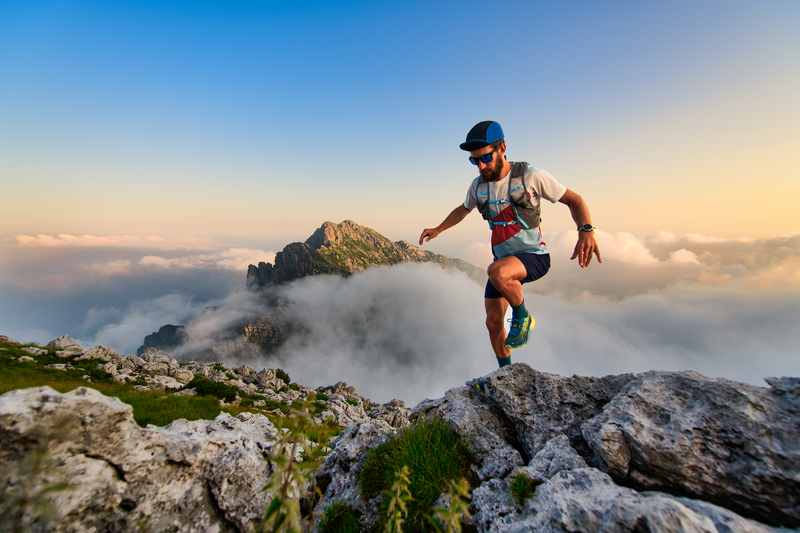
95% of researchers rate our articles as excellent or good
Learn more about the work of our research integrity team to safeguard the quality of each article we publish.
Find out more
REVIEW article
Front. Environ. Sci. , 09 January 2023
Sec. Toxicology, Pollution and the Environment
Volume 10 - 2022 | https://doi.org/10.3389/fenvs.2022.1093920
Pharmaceuticals have been identified as a significant threat to the environment. Their constant flow into aquatic ecosystems means that organisms are chronically exposed. To date, there has been a large number of scientific papers assessing the impact of pharmaceuticals on individual organisms from different taxonomic groups. However, the effects of drugs on the environment can be much broader than what can be determined in toxicity tests on individual organisms. These compounds can disrupt entire communities. In this context, special attention should be paid to microbial communities, which regulate many essential processes underpinning aquatic food webs and ecosystem services. This paper reviews current developments related to the effects of pharmaceuticals on microorganisms with a particular focus on whole-community investigations, in both fresh and salt water. We also summarize the opportunities associated with both in situ and laboratory studies, and highlight important knowledge gaps.
Pharmaceuticals are biologically active compounds widely and increasingly used in human and veterinary medicine to treat different health problems (Fent et al., 2006; Bernhardt et al., 2017). The high consumption of various groups of pharmaceuticals leads to their continuous release into the environment, making them pollutants of emerging concern (Bernhardt et al., 2017; Madikizela and Chimuka, 2017; Ali et al., 2018; Tang et al., 2021). According to Bernhardt et al. (2017), the increase in production and consumption of synthetic chemicals, including pharmaceuticals, far exceeds the rate of change of other global change factors, such as biodiversity loss, elevated atmospheric CO2, nutrient pollution and land use change. Pharmaceuticals enter the environment mainly from wastewater treatment plants (WWTPs), but also from agriculture, landfills, hospital and household waste (Nikolaou et al., 2007). Many papers have confirmed the presence of pharmaceuticals in environmental samples including surface water, sediment and groundwater (Sibeko et al., 2019; Agunbiade and Moodley, 2016; Maranho et al., 2015; Wu et al., 2010; Borecka et al., 2015; Vidal-Dorsch et al., 2012). Several works have also revealed the presence of pharmaceuticals in both freshwater and saltwater aquatic organisms. Pharmaceuticals found include psychoactive, synthetic hormones and non-steroidal anti-inflammatory drugs (NSAIDs). These were detected in crustaceans, cephalopods, fish and bivalves collected in Italy, Sweden, Portugal, Spain and Saudi Arabia (Zhang et al., 2011; Alvarez- Muñoz et al., 2015; Mezzelani et al., 2016; UNESCO and HELCOM, 2017; Ali et al., 2018; Martínez-Morcillo et al., 2020). In addition, many papers have highlighted the adverse effects of pharmaceuticals on aquatic organisms from cellular to tissue level. Commonly observed effects in various taxa exposed to pharmaceuticals include metabolic and gene expression disorders, endocrine and reproductive abnormalities, tissue lesions, cyto- and genotoxicity (Świacka et al., 2022; Parolini et al., 2009; Schwarz et al., 2017; Han et al., 2010; Islas-Flores et al., 2017; Richmond et al., 2017; Heckmann et al., 2006; Nunes et al., 2020). In addition, pharmaceuticals may also induce behavioural changes (Galus et al., 2014; Bertram et al., 2022). Vera-Chang et al. (2018) observed reductions in exploratory behaviour in zebrafish exposed to fluoxetine. In a study by Hellström et al. (2016), oxazepam (anxiolytic drug) promoted migratory behaviour in Atlantic salmon both in the laboratory and in a natural river tributary. On the other hand, carbamazepine (anticonvulsant) and gemfibrozil (blood lipid-regulating pharmaceutical) reduced the courtship behaviour of male zebrafish (Galus et al., 2014).
Microbes are one of the most important biological groups on the planet in terms of functional diversity, as confirmed by metagenomic and ecological studies (Chróst, 1990; Bertilsson and Jones, 2003; Glöckner et al., 2012; Amalfitano et al., 2015). Aquatic microbial communities are co-occurring (present in a specific habitat in time and space) and potentially interacting autotrophic and heterotrophic microorganisms including a wide variety of bacteria, unicellular algae, protists and fungi (Sabater et al., 2002; Lyautey et al., 2005; Proia et al., 2012; Battin et al., 2016; Callieri et al., 2018). Microbes often form communities such as biofilms in flowing systems (Proia et al., 2012). Interestingly, in the natural environment, at least 95% of microorganisms are found in biofilm form (Nikolaev et al., 2007). Biofilms are spatially and metabolically structured microbial communities which are deposited in an extracellular polymer matrix (Nikolaev et al., 2007).
The interactions within microbial communities are remarkably close and complex. For example, by-products such as carbohydrates synthesized by algae are used by bacteria and fungi, while nutrients recycled by bacterial and fungal processing of organic detritus are essential for algal growth (Proia et al., 2012). Furthermore, these food web interactions promote the succession of aquatic microorganisms (Battin et al., 2016; Callieri et al., 2018).
Microbial communities are a key source of energy in aquatic food webs and contribute to global cycles of both energy and matter. They decompose organic matter producing inorganic compounds that can then be used by producers (Sabater et al., 2002; Glöckner et al., 2012; Proia et al., 2012; Battin et al., 2016; Hons, 2018; Richmond et al., 2019). Therefore, without microorganisms, the nutrient cycle and consequently the production of energy would be impossible. In addition, microbial communities are an important indicator for assessing ecosystem health. The community structure is strongly related to environmental conditions, directly reflecting negative effects caused by pollution (Brümmer et al., 2000; Lyautey et al., 2005). Another significant contribution of microbial communities is to the self-purification processes of aquatic ecosystems, by removing both inorganic and organic compounds, including some pharmaceuticals (Sabater et al., 2002; Battin et al., 2016).
Despite microbial communities playing such a significant role in aquatic environments, research on the effects of pharmaceuticals is just emerging, while research on other groups of organisms, such as bivalves and fish, is more advanced (Han et al., 2010; Costa et al., 2019; Derakhsh et al., 2020). Disruption of the biofilm community may provoke functional changes by impairing crucial ecosystem processes including nutrient cycling and primary production. This is related to the fact that the diverse functions of microbial communities cannot be replaced by any other organisms (Battin et al., 2016). Therefore, the influence of pharmaceuticals on aquatic microbial communities may have profound and long-term consequences, leading for example to significant energy and oxygen reduction in freshwater ecosystems and causing overall ecosystem health disruption. Furthermore, disruption of the aquatic ecosystem may also have significant impacts on terrestrial animals and humans using its resources (Karr and Chu, 2000). For example, many aquatic organisms serve as an important food source for terrestrial organisms, so pharmaceuticals affecting aquatic trophic networks also disrupt terrestrial ones (Richmond et al., 2018). Moreover, even if the trophic networks are not disrupted, due to the biomagnification process, pharmaceuticals can accumulate in terrestrial organisms feeding in water (Sullivan et al., 2012).
Therefore, the aim of this review is to summarize the current knowledge on the effects of various groups of pharmaceuticals commonly detected in aquatic environments, individually or in mixtures, on microbial communities naturally occurring in both saltwater and freshwater habitats. Special attention is given to various sublethal effects, particularly those related to the functioning of the microbial community as a whole, including changes in biomass, species composition, oxygen consumption, and photosynthesis. In addition, the pharmaceutical concentration, exposure time and the methods used have been discussed to draw overall conclusions regarding the effects of pharmaceuticals on microbial communities.
For the purposes of this review, it was decided to describe the effects of pharmaceuticals on aquatic microbes at community level. Studies describing toxicity using single organisms grown in monocultures were not considered, nor were microbes associated with sludge in wastewater treatment plants. In this review we focus on the individual pharmaceuticals and as mixtures but exclude personal care products. This review is divided into two sections: saltwater environments (open oceans, bays, and estuaries) and freshwater environments (lakes, rivers, and streams).
To acquire the literature for this review, a search for publications was conducted between May 2022 and July 2022, using publicly available databases (e.g., Google Scholar, Science Direct and Scopus). Various combinations of keywords were used to search these databases, including “pharmaceuticals” and “microbial community” or “biofilm” but also the names of individual groups of compounds (e.g., antibiotics, antihistamines, NSAIDs, psychoactive) or the names of specific compounds. This resulted in formulation of various two-level keywords. The selection of articles for inclusion in this work was done by evaluating their relevance to the main topic, with a particular emphasis on the effects of pharmaceuticals on microbes at community level. In total, 50 articles were selected for this review. The data were complemented by 51 articles containing general information regarding pharmaceuticals and functioning of microbial communities.
Assessing the effects of contaminants on the functioning of whole communities of organisms is a complex task, and to date there is no standardized approach to this issue. The most commonly proposed methods for assessing the effects of pharmaceutical contamination on microorganisms are shown in Figure 1 and include both in situ analyses and those conducted under controlled laboratory conditions.
Among the in situ analyses, studies can be performed by investigating naturally occurring microorganisms in areas where pharmaceutical-rich wastewater enters the environment (Aristi et al., 2015; Chonova et al., 2018). Comparative analysis of organisms from an uncontaminated site in the same body of water, such as a river above the effluent outlet, can then be used as a control. This approach allows for a realistic assessment of the impact of pollutants on the environment but it has the significant disadvantage that it is impossible to control external environmental conditions and to clearly assess which factor(s) actually caused the observed effects. In addition to pharmaceuticals, effluent streams from wastewater treatment plants are complex mixtures that contain many other, potentially toxic compounds that may affect microorganisms. Assessing the impact of pharmaceuticals on naturally occurring microbial communities is possible through the use of pharmaceutical-diffusing substrate (PhaDS). This method, described by Costello et al. (2016), involves placing containers filled with a substrate that gradually release added pharmaceuticals affecting microorganisms colonizing it (Figure 1). The release is via diffusion from the relatively high concentration in the device, through the biofilm growing on the cap and into the overlying water. This allows for semi-controlled analysis of the effects of selected pharmaceuticals on organisms under environmental conditions. Although this approach allows for the selection of the specific compounds with which organisms are exposed, it does not exclude the simultaneous influence of an additional suite of pharmaceuticals (and other stressors) already present in the environment. Additionally, although the substrate is filled with pharmaceuticals of known concentrations, their diffusion into the environment and the final concentration to which the organisms inhabiting it are exposed is difficult to specifically determine because diffusion is influenced by variations in environmental factors, in particular the water velocity at the substrate-water interface which in turn determines the diffuse boundary-layer thickness (Shaw et al., 2015).
Due to the high unpredictability and variability in factors potentially influencing analyses performed directly in the environment (e.g., the effects of cloud cover on photosynthesis), a more commonly used alternative is to work under controlled laboratory conditions, made possible by the use of mesocosms (Corcoll et al., 2015; Richmond et al., 2019; Robson et al., 2020). This approach allows exposure of test organisms to pharmaceuticals at known concentrations, while controlling variables such as water temperature, incident light and stream flow but maintaining a relatively high resemblance to the real environment. Advanced mesocosms can reflect many features of natural water bodies, such as variety of different substrate types, water flow, fluctuating light conditions and constant, regular inflow of pollutants (Robson et al., 2020). An even more simplified option, allowing greatest control over the testing environment is through the use of microcosms e.g., using plates or flasks (Cui et al., 2021). However, by reducing the complexity of conditions and the scale of interactions occurring under experimental conditions, the results obtained may be far from actual environmental outcomes.
Aristi et al. (2015) analysed the effects of effluent from a wastewater treatment plant on river biofilms and ecosystem metabolism, by comparing a segment of the river above the WWTP effluent with three stations influenced by the pollutants. Concentrations of common pharmaceuticals (diclofenac, ibuprofen, carbamazepine, venlafaxine) ranging from a few to tens of ng/L for each compound were measured in all segments of the river. The analyses showed that effluent acted as a subsidy on biofilm biomass and ecosystem respiration (Figure 2), probably as a consequence of enhanced availability of organic carbon. This effect showed a high correlation with both changes in nutrient (nitrate and phosphate) and pharmaceutical concentrations. However, the study also observed some indications of stress effects, which were reflected in non-photochemical quenching disturbances in the biofilms. Chonova et al. (2018) also conducted an in-depth analysis of the effects of pharmaceuticals emitted from the WWTP on river biofilm by comparing communities of biofilm-forming bacteria above and below the effluent inflow from a WWTP. The 3-years study analysed environmental samples during summer and winter and determined concentrations of 10 commonly used pharmaceuticals. It was observed that bacterial community structures, characterized by denaturing gradient gel electrophoresis (DGGE), were strongly related to concentrations of sulfamethoxazole for the station located close downstream to the effluent (Table 1). On the other hand, DGGE profiles from the station located far downstream from the effluent were related to concentrations of ibuprofen and atenolol. Aubertheau et al. (2017) also examined the effect of WWTP effluent on river biofilm. Their study analysed 12 pharmaceuticals from different groups at sites located downstream from 12 WWTPs, showing the presence of 11 of them in the biofilm, with the highest occurrence frequency for diclofenac, carbamazepine, sulfamethoxazole and propranolol (Table 1). The results confirm that biofilm-forming microorganisms can sorb and accumulate numerous pharmaceuticals. In addition, this work shows that WWTP effluent can be a factor modifying biofilm species composition, in particular reducing the abundance of the cyanobacteria present (Figure 2). Importantly, the abundance of resistance genes was observed to increase. Antimicrobial Resistance (AMR) is a phenomenon that involves the activation or development of mechanisms enabling microorganisms to survive in the presence of chemicals intended to kill them (Christaki et al., 2020). The release of residual antimicrobial compounds from WWTPs into the environment is one important cause of this phenomenon. Observations in recent years unambiguously confirm that AMR should be counted among the main threats to the modern world, and this phenomenon, although already causing a significant problem, is likely to intensify (O’Neil, 2014; Matviichuk et al., 2022).
FIGURE 2. Examples of processes and features of the microbial community that are widely affected by pharmaceuticals.
Rosi-Marshall et al. (2013) used PhaDS filled with selected compounds at concentrations of 12–15 mM each, to investigate the effects of several pharmaceuticals (caffeine, ciprofloxacin, cimetidine, diphenhydramine, metformin, and ranitidine) individually and in mixtures on river biofilm. Of the three antihistamine compounds tested, only diphenhydramine had a statistically significant effect on gross primary production and respiration, causing rates of both processes to decrease significantly (Table 1). Interestingly, the effects of this compound were only slightly smaller than those of the antibiotic, ciprofloxacin. Effects on respiration were observed on both the inorganic substrate at the top of the PhaDS device, which promotes the growth of photosynthetic organisms, and the organic substrate, which promotes the growth of heterotrophic bacteria. Analysis of biofilm composition indicated that the observed diphenhydramine effect on respiration may be due to changes in species composition. Similar observations were noted in the work of Rosi et al. (2018), where they compared the effects on biofilm in streams with different degrees of urbanization, observing that the effects of ciprofloxacin and diphenhydramine on respiration occurred in the least urbanized sites, while they were not observed in the more polluted ones, suggesting the development of tolerance to these pharmaceuticals when there is constant exposure. Interestingly, in the case of ciprofloxacin, it was observed that in the urban streams where this compound had no significant effect on respiration, it caused changes in the taxonomic composition of the biofilm. Furthermore, the effect on composition was greatest at the site where respiration was least affected. Shaw et al. (2015) studied the same pharmaceuticals except that the diffusing substrates were placed in lentic systems. In this work, a strong effect of diphenhydramine on biofilm-forming organisms was observed, where the decrease in rates of gross primary production and respiration was much greater than for other tested compounds, including ciprofloxacin. Ogata et al. (2020) observed that a mixture of diphenhydramine and caffeine affected the taxonomic composition of stream biofilms (Table 1), although this was primarily through promoting unique taxa associated with contaminant tolerance and/or degradation, as caffeine and diphenhydramine, may have served as a carbon and/or energy source for taxa capable of degrading one or both compounds.
Several studies have evaluated the effects of pharmaceutical mixtures on biofilm-forming microorganisms under controlled laboratory conditions. Proia et al. (2013) conducted translocation experiments in which they exposed the biofilm for 16 days to water taken from a clean reference river, a moderately polluted river and a heavily polluted river in which pharmaceuticals (57 compounds) and pesticides (16 compounds) were detected. After biofilm translocation from clean to polluted water, it was observed that autotrophic biomass and peptidase increased while phosphatase and photosynthetic efficiency decreased, and these effects were associated with analgesics and anti-inflammatories. In contrast, Corcoll et al. (2015) used artificial streams to evaluate the effects of a mixture of nine pharmaceuticals at 5 μg/L on river biofilm using fully controlled conditions. During 11 days of experiment, pharmaceuticals caused a decrease in biomass and number of algal species, as well as changes in bacterial structure, as demonstrated by a reduction of the operational taxonomic unit (OTU) richness (Table 1). On the other hand, a stimulatory effect of the tested pharmaceuticals was also observed, which caused an increase in primary production and respiration, which could be related to the promoted growth of green algae. Using artificial stream mesocosms, Robson et al. (2020) analysed the effects of a mixture of three pharmaceuticals—fluoxetine, diphenhydramine and ciprofloxacin—on established and successional river biofilm for 20 days. Although the effect on established biofilm was small, a substantial reduction in both gross primary production and community respiration was observed in successional biofilm which was attributed to the lack of a protective EPS (extracellular polysaccharide) layer (Table 1). Under shaded conditions, these same compounds also interfered with denitrification. The major effect of these pharmaceuticals on the established biofilm was on changing in diatom community structure.
Several papers have highlighted the potential effects of SSRIs on freshwater microorganisms (Richmond et al., 2016; Richmond et al., 2019; Yang et al., 2019; Robson et al., 2020; Cui et al., 2021). Richmond et al. (2016) analysed the effects of fluoxetine and citalopram (20 μg/L of each; individually and in a mixture) using artificial stream mesocosms. Both compounds suppressed gross primary production and community respiration but had no effect on algal biomass and whole-stream metabolism (Table 1). Synergistic effects were not observed with the mixture, as results were similar to those in individual exposures. In a subsequent paper by Richmond et al. (2019), the same artificial stream mesocosms were exposed to fluoxetine, but the experimental duration was extended to 21 days using an environmentally relevant concentration of 20 ng/L in addition to the 20 μg/L from the earlier study. In contrast to the earlier work where already developed communities were exposed, the effect of fluoxetine on the process of biofilm colonization of stones was observed. It found that a trace concentration of 20 ng/L caused disruption of algal colonization and affected primary productivity on day 13 (Table 1). However, after 21 days, all parameters tested including chl-a, NEP and GPP were consistent with the control. These findings indicate that the colonization rate may be an important parameter when considering effects of pharmaceuticals on biofilm forming organisms. Robson et al. (2020) confirmed these observations, demonstrating that 20 ng/L fluoxetine causes a reduction in rates of gross primary production and community respiration in successional biofilm, while having no significant effect on established biofilm (Table 1).
Yang et al. (2019) evaluated the effect of another SSRI, sertraline, on lake microorganisms using microcosms. A 15-days exposure to 50 μg/L resulted in reduced chlorophyll-a content in the microcosms, suggesting this compound affected photosynthetic processes. Additionally, it was shown that sertraline can stimulate the growth of some organisms (bacteria) while reducing the diversity of others (cyanobacteria) (Table 1). Cui et al. (2021) conducted an extension of this study, by meta-transcriptomic profiling of functional variation of a microbial community affected by sertraline (50 μg/L). The analysis showed that sertraline disrupts the function of both eukaryotic and prokaryotic organisms, but the effects are more intense in bacteria (Table 1). Molecular studies have shown that sertraline causes inhibition of pathways related to lipid metabolism, energy metabolism, membrane transport function, and genetic information processing in the aquatic microbial community (Cui et al., 2021). Although the endpoints analysed in the work of Yang et al. (2019) and Cui et al. (2021) were different from those analysed for fluoxetine (Richmond et al., 2016; 2019; Robson et al., 2020), all of these studies confirm that psychoactive drugs can have significant effects on both the ecological structure and biochemistry of freshwater microbial communities.
Lawrence et al. (2005) analysed the effects of 10 μg/L ibuprofen on riverine biofilm, showing that this compound can significantly reduce cyanobacterial biomass (Figure 2) and affect bacterial community composition. The effects of ibuprofen, individually and in mixture with 17α-ethinylestradiol (103 and 148 mg/L, respectively) on biofilm were also analysed by McClean and Hunter (2020). Their study showed that this compound can decrease biofilm respiration and interfere with net primary production (Table 1). While respiration was also suppressed in the mixture, this was not the case for primary production. Although significant effects were observed in this study, the concentrations used were well above environmentally relevant values, which are usually measured in ng/L (Zuo et al., 2006; Rocha et al., 2013). Lawrence et al. (2007) examined the effect of another NSAID, diclofenac, on the microbial community. The observed effects at concentrations of 10 and 100 μg/L were inconclusive, as this compound had a stimulatory effect by increasing bacterial biomass but also had a negative effect on biodiversity. The observed changes were also different depending on season.
Kergoat et al. (2021) exposed freshwater biofilms for 4 weeks to two sulphonamides, sulfamethazine and sulfamethoxazole, at concentrations of 500 and 5,000 ng/L. Exposure to these antibiotics caused changes in structure, diversity, viability, and integrity of diatom cells. Additionally, a twofold increase in mortality of diatoms was observed in the sulfonamide-exposed group compared to the untreated biofilm. Sulfamethazine caused a reduction in species diversity as well as teratologies (deformities) in diatoms (Table 1). The observed changes indicate that sulfonamides pose a serious threat to the microbial community. Using mesocosms, Corno et al. (2014) examined the effects of a mixture of three commonly used antibiotics in Europe (tetracycline, imipenem, levofloxacin at 12.5 μg/L and 125 μg/L) on aquatic bacterial communities from European lakes. The results showed that the antibiotics caused a reduction in the number of bacteria (by about 75%), but this was not dependent on the drug concentration. In addition, the antibiotic mixture also had a large effect on the phenotypic distribution. In the presence of antibiotics (regardless of concentration), the bacteria formed large aggregates consisting of several different strains and small clusters composed of a single strain. It was proposed that the formation of aggregates is a resistance strategy developed by bacteria to create antibiotic-free space inside the clusters. Quinlan et al. (2011) observed that tetracycline (.1–100 μg/L) reduced microbiome numbers over a 28-days exposure period. Brosche and Backhaus (2010) exposed a community of limnic microorganisms to five antibiotics that inhibit protein synthesis: Streptomycin, chloramphenicol, fusidic acid, rifampicin, and chlortetracycline (in mixture and individually). The single-substance toxicity tests showed that each of the tested antibiotics inhibited bacterial protein biosynthesis, but with different potency: EC50 values ranged from 66 μg/L (chlortetracycline) to 46 mg/L (streptomycin) (Table 1). The adverse effects of levofloxacin and another of the tetracycline group antibiotics, oxytetracycline, on aquatic microorganisms were also demonstrated by Zhou et al. (2020). After 2 weeks of incubation, the microbial community was exposed to levofloxacin and oxytetracycline (individually) at a concentration of 5 μg/L. After 14 days exposure, the composition of the prokaryotic microbiota significantly changed at the genus level. These changes were dependent on the type of antibiotic, suggesting that the sensitivity of the bacterial community in this environment to a given contaminant varies. In the case of eukaryotes, 14-days exposure to levofloxacin and oxytetracycline (5 μg/L) did not significantly affect their diversity. The observed changes in the composition of the prokaryotic biofilm demonstrates that even low doses of these antibiotics can disrupt development, and generate phenotypic and genotypic variation, which in turn may accelerate the spread of resistance genes. Wang et al. (2022) showed in a 12-days mesocosm experiment that the lowest dose of oxytetracycline hydrochloride (5 mg/L) stimulated photosynthesis and biomass growth, while these parameters were inhibited at higher concentrations (25 and 75 mg/L). However, it should be noted that these concentrations are much higher than environmentally relevant. Effects on photosynthesis were also observed by Deng et al. (2022) when riverine biofilm was exposed to ofloxacin at concentrations of .01, .1, 1, 2, and 5 mg/L. After a 4-days exposure, concentrations of Chlorophyll-a and Chlorophyll-b decreased by 31.4% and 36.8%, respectively, compared to the control group, indicating ofloxacin at these “high” concentrations may result in reduced photosynthetic capacity.
Several studies have examined the effect of selected antibiotics on nutrient cycling. Gray and Bernhardt. (2022) treated microbial communities from stream sediments with three antibiotics (sulfamethoxazole, danofloxacin, and erythromycin as mixtures and individually at 10 μg/L) to measure rates of bacterial respiration and nitrogen transformation. Contrary to expectations, there was no effect of individual, and mixtures of, antibiotics on NO3− uptake or production of N2O, N2, CH4, and CO2. However, the mixture induced a marked increase in NH4+ concentration. Rico et al. (2014) observed a potential effect on nitrification of the antibiotic enrofloxacin, added to microcosms for 7 days at nominal concentrations of 1, 10, 100, and 1,000 μg/L. The highest concentration of enrofloxacin significantly reduced the number of ammonia-oxidizing bacteria in the sediment, which translated into a higher ammonia concentration in the water and a lower nitrification rate (Table 1). Hou et al. (2015) showed that sulfamethazine causes a large decrease (approximately 20%–30%) in denitrification rate by inhibiting the growth of denitrifying bacteria (Table 1). This decrease was observed at drug concentrations up to 5 μg/L, above which denitrification rates remained constant. Inhibition of denitrification may lead to excessive accumulation of nitrogen (as ammonia) in the ecosystem and thus cause eutrophication. The denitrification process occurs through a series of related reduction steps mediated by various enzymes and genes. Sulfamethazine was found to increase N2O production due to inhibition of its reduction to free nitrogen. Sulfonamide-mediated inhibition of denitrification was also demonstrated by Xu et al. (2020), who studied the effects of sulfamethoxazole on denitrification and N2O release in river sediments. Collected sediments were incubated for about 1 month in the lab to remove sulfamethoxazole residues. Depending on the parameter under investigation, the antibiotic was introduced into the slurry at concentrations ranging from 1 to 100 μg/L. A clear decrease in the denitrification rate (by as much as 96%) with increasing sulfamethoxazole concentration was observed (Table 1). Sulfamethoxazole also decreased the rates of anaerobic ammonium oxidation (“anammox”); the rate of anammox decreased with increasing concentrations of sulfamethoxazole. Furthermore, 2-ethylhexyl-4-methoxycinnamate (UVB blocker) enhanced the observed inhibitory effects of sulfamethoxazole. Sulfamethoxazole also decreased the expression levels of important denitrification genes (nirS and nosZ) (Table 1).
Selected antibiotics have shown marked effects on enzymatic activity and biofilm metabolism. In these studies, enzyme activity was most commonly measured during or after biofilm exposure to pharmaceuticals. Paumelle et al. (2021) found that 14 days exposure of microcosms (containing colonized alder leaves immersed in water) to sulfamethoxazole and sulfamethazine at 5 μg/L, significantly reduced leaf-associated microbial community biomass (especially between days 3 and 7), accompanied by an increase in β-glucosidase activity, which has been attributed to the bacteria’s stress response to antibiotics (Table 1). In contrast, no effect of the drugs was observed on the four other enzyme activities measured: Cellobiohydrolase, phenol oxidase, alkaline phosphatase and leucine aminopeptidase. A significant increase in the activity of β-glucosidase with a 14 days exposure to sulfamethoxazole (500 and 5,000 ng/L) was confirmed by Pesce et al. (2021). On the other hand, sulfamethazine caused an approximate 20% decrease in β-glucosidase activity, which, according to the authors, may reflect the greater toxicity of sulfamethazine compared to sulfamethoxazole or the greater sensitivity of microorganisms to this antibiotic. These differences indicate that caution should be exercised when formulating conclusions about the properties of a given group of antibiotics on the basis of studies performed for a single compound. Effects of antibiotics on enzymatic activity were also noted by Wang et al. (2019) following a 192 h microcosm exposure to florfenicol and ofloxacin introduced separately at concentrations of 10, 100, and 1,000 μg/L. Both antibiotics caused an up to 6.7 times increase in the activity of antioxidant enzymes (superoxide dismutase and catalase) compared to baseline values for the untreated control groups (Table 1). The activity of these enzymes increased with increasing antibiotic concentration. Moreover, it was shown that in biofilms devoid of extracellular polymeric substances (EPS), antibiotic distribution coefficients (expressed by ratio of sorption amount to concentration of antibiotics at equilibrium solution) were 3.2 and 6.5 times higher (for florfenicol and ofloxacin, respectively) compared to the control group. This may indicate that EPS can act as a protective barrier for biofilm and increase its resistance to antibiotics, as noted above (Robson et al., 2020). Pu et al. (2021) studied the effects of erythromycin (6 μg/L) on freshwater biofilms. The biofilm used in this study was cultured in reactors, in aquarium water, allowing the lab to replicate a freshwater environment. The metabolic data indicated that despite the presence of the drug, cell-to-cell communication pathways, amino acid metabolism and peptidoglycan biosynthesis remained unchanged. However, changes in lipid and linoleic acid metabolism were observed (Table 1).
Several studies have used exposure-induced changes in transcription and gene expression to evaluate the effects of antibiotics on biofilm. Yergeau et al. (2010) used meta-transcriptomic analysis to investigate the response of river biofilms to four antibiotics: Erythromycin, gemfibrozil, sulfamethazine, and sulfamethoxazole. Biofilm reactors were inoculated with water from two rivers of different trophic status and exposed to different doses of drugs (.5 or 1 μg/L). It was observed that drug exposure can induce both positive and negative effects on gene transcription in the biofilm. Erythromycin had a positive effect on guanosine tetraphosphate production, the level of which is associated with many cellular functions. Both sulfonamides induced changes in DNA and RNA polymerase, which are responsible for replication and transcription. Moreover, sulfamethazine also affected changes in the expression of genes related to the envelope and outer membrane (Table 1). The effect of gemfibrozil on genes related to lipid metabolism was also observed. In their subsequent study, Yergeau et al. (2012) used the same group of drugs, testing whether, in addition to changes in gene expression, these drugs would also induce changes in the community composition. Based on the 16 S rRNA gene amplicons, they found that exposure to these antibiotics caused only minor changes in the composition of the bacterial community. However, it was observed that these drugs can induce numerous functional changes in the communities. Their presence had a large effect on the expression of genes responsible for nitrogen, phosphorus and carbon cycles (Table 1). Consequently, this may have led to a shift in the rate of phosphorus and carbon cycling, but this was not investigated in this work. These results again confirm that antibiotics at environmentally relevant concentrations can have a major impact on biofilm function and lead to disruption of important ecosystem processes.
One of the few papers directly examining the effects of pharmaceutical contamination on microbes in the marine environment is from Peele et al. (1981). This study investigated whether a pharmaceutical waste dump 64 km north of Arecibo (Puerto Rico Trench, Atlantic Ocean) affected microorganism composition in surface waters. Surface water was collected from the dump site and from several stations located nearby (close to the Puerto Rico shore) and transported to the laboratory. Microbial colonisation experiments showed that the pharmaceutical dumping site significantly affected taxonomic composition of microorganisms from surface waters. Vibrio and Aeromonas spp. were the dominant marine microorganisms culturable on agar from stations closest to the Puerto Rico shore and near the pharmaceutical dump site. However, the abundance of Vibrio and Aeromonas spp. decreased on the pharmaceutical dump sites, with an increase in the abundance and dominance of Gram-positive organisms (staphylococci, micrococci, and bacilli), which were proposed to have been introduced with the pharmaceutical waste (Table 2). In addition, an increase in specific bacterial activity rates, the ratio of 14C-labeled amino acid uptake to the total number of bacteria, was observed near the dump site. According to Peele et al. (1981) these changes in specific activity may be related to the shift in taxonomic composition of the bacterial community.
Porsbring et al. (2009) studied the effect of clotrimazole, an anti-fungal pharmaceutical commonly used in human and veterinary medicine, on marine periphyton. Periphyton were grown on submerged glass discs in Kalvhagefjorden Bay (Sweden) for a period of 7–9 days. After colonization and retrieval, discs were treated with clotrimazole at concentrations of 3.45 ng/L to 345 μg/L for 4 days. Seawater from a reference site with temperature and salinity matching the study site was used as the medium.
Toxic effects of clotrimazole included inhibition of 14α-demethylase-dependent sterol synthesis, total sterol content reduction, community chlorophyll a content reduction along with changes to the cycling of the photoprotective xanthophyll pigments and overall pigment profile, as well as a reduction in community growth pigment profile. The community pigment profile describes the relative amounts of pigments in individual community members, as well as changes in relative abundance between species that have different pigment patterns. Disruption of the pigment profile can lead to changes (often decreases) in photosynthetic capability.
Interestingly, some of the observed effects, such as accumulation of C14 α-methylated sterol precursors and pigment profile reduction were dose-dependent (Table 2). Backhaus et al. (2011) tested the influence of pharmaceuticals (fluoxetine-psychoactive, propranolol- β-blocker and clotrimazole) and personal care products (triclosan, zinc-pyrithione) as a mixture and as single substances on communities of marine microorganisms. Similar to Porsbring et al. (2009), microorganisms were grown on glass discs submerged for 7 days in Kalvhagefjorden Bay and then transported to the laboratory. Periphyton was exposed to chemicals at concentrations ranging from .6 ng/L to 34.5 mg/L for 96 h. Each of the tested compounds, individually and as a mixture, inhibited periphyton growth as determined by the total pigment content. Interestingly, in the lower concentration range (69 μg/L and below) a hormesis effect was observed. This phenomenon shows that an organism’s response to low-level doses of an agent, such as a xenobiotic chemical, is notably different to the response at high doses. This may result in low doses of an agent may have a stimulating effect (Agathokleous, 2018), as demonstrated in Backhaus et al. (2011) as an increase in pigment content in the group exposed to low concentrations of the drug compared to controls. The median effective concentration (EC50) was determined for final biomass formation (by measuring pigment content) and ranged for a single compound from 2.3 μg/L (Zn-pyriton) to 347 μg/L (triclosan), while the EC50 for the mixture fell between these values. This indicates no strong synergism or antagonism of the mixture components.
Johansson et al. (2014) also investigated the effect of pharmaceuticals (ciprofloxacin and sulfamethoxazole) on marine periphyton sampled along the Swedish west coast (Kalvhagefjorden Bay). Marine microorganisms were cultured and tested as described above (Porsbring et al., 2009). Microorganisms grown on these glass discs were treated with antibiotics in the concentration range 1.27 μg/L to 3 mg/L for 4 days. Carbon source utilization was used to evaluate the toxic effects of the pharmaceuticals. Briefly, optical density, followed by an estimation of the total metabolism of each individual carbon source by fitting a Weibull model in the control and treatment groups, were used to measure carbon source utilization (see Johansson et al., 2014). Sulfamethoxazole caused a decrease in carbon source utilization, while ciprofloxacin exposure led to a 20%–50% change in carbon utilization patterns, indicating that the two pharmaceuticals are having different effects on the microorganisms (largely bacteria), responsible for carbon utilization (Table 2). Interestingly, 1.3 μg/L sulfamethoxazole stimulated biofilm total pigment content (hormesis effect). Neither sulfamethoxazole nor ciprofloxacin caused an inhibitory effect on the amount and or affected the composition of pigments at any concentration tested. Beretta et al. (2011) studied the effects of two pharmaceuticals, atenolol (antihypertensive drug) and erythromycin (antibiotic) on microorganisms in marine sediments. For the toxicity evaluation, sediment samples were collected from the near-shore of All Saints Bay, Brazil, and suspended in marine water. Next, the sediment suspensions containing microorganisms were treated with three pharmaceutical concentrations (.002, .02 and .2 g/L) and added to Plate Count Agar. These selected concentrations were much higher than the concentrations of these compounds measured in the environment. Colony forming units (CFU) were used to evaluate pharmaceutical effects; a 20% reduction in CFU was considered toxic. Results showed that erythromycin at the highest concentration (.2 g/L) significantly inhibited microorganism growth, while atenolol did not induce toxic effects (Table 2).
Chen et al. (2022) investigated the influence of the antibiotic norfloxacin, individually and in combination with copper, on sediment microbial communities. Sediment collected from Shantou Bay estuary (China) was incubated in the dark over 7 days before treatment with norfloxacin alone (1, 10, and 20 μg/g dry weight) and in combination with copper (40 μg/g dw) for 28 days. During the exposure, the bacterial population decreased significantly in tanks with norfloxacin, copper and their mixture except on day 28, when the population recovered. Nevertheless, on this last day of exposure, all treated groups (copper, norfloxacin and their combination) had lower Shannon or Simpson indices compared to the control, suggesting an effect of both compounds and their combination on microbial diversity (Table 2). In addition, the negative effects of norfloxacin at concentrations of 10 μg/g and 20 μg/g on days 7 and 28, respectively, were intensified by copper.
A few papers have studied the toxic effects of pharmaceuticals on salt marsh microbes. Cordova-Kreylos and Scow (2007) collected sediment from three Californian salt marshes (San Francisco Bay) and used microcosms to expose this sediment to ciprofloxacin at concentrations ranging from .02 to 200 mg/L for 30 days. These concentrations were well above environmental levels to allow for high sorption of the pharmaceutical to the sediment. Phospholipid fatty acid (PLFA) analysis was used to assess microbial biomass and community composition. Interestingly, higher concentrations of the antibiotic (2–200 mg/L) caused a significant increase in both biomass and PLFA richness of sulfate-reducing bacteria and Gram-negative bacteria (Table 2). The proposed reason for the observed stimulatory effect was ciprofloxacin acting as a carbon source for these bacteria. The lack of an inhibitory effect was explained by the high sorption of the antibiotic and thus reduced bioavailability. According to Cordova-Kreylos and Scow (2007), the selective effects of an antibiotic on the microorganisms may promote resistance of the bacterial community to the compound. It was not stated whether the sampling sites, which varied in exposure to toxic materials, had prior exposure to ciprofloxacin. Näslund et al. (2008) also investigated the influence of ciprofloxacin on sediment microorganisms using microcosms with 7 weeks exposure to antibiotic concentrations of 20, 200, 1,000, and 2,000 μg/L. Unlike Cordova-Kreylos and Scow (2007), results of this study showed a negative effect of ciprofloxacin on both microbial diversity and the effectiveness of that community to mineralize the toxic polycyclic organic hydrocarbon, pyrene (Table 2).
Fernandes et al. (2015) tested the effect of tetracycline and the veterinary antibiotic enrofloxacin on microorganisms associated with the salt marsh plant, Phragmites australis. Sediment in contact with roots (rhizosediment) of this plant, along with estuarine site water, was collected from the Lima River estuary (Portugal). Two experimental scenarios were prepared: planted-collected rhizosediment with P. australis and unplanted sediment without P. australis. Sediment slurries were exposed to 100 μg/L of either drug for 7 days. Total cell counts and microbial community structure were used to assess impact of the antibiotics. No significant effect of the antibiotics on microbial abundance was observed. Enrofloxacin affected community richness, but only in the unplanted system, suggesting a protective role of P. australis (Table 2). It was suggested that carbon exudates from plant roots helped shape the microbial community structure and reduced potential sensitivity of that community to the antibiotics by providing a labile carbon source. In addition, some studies have reported pharmaceutical uptake by plants which also may contribute to reduce toxicity to the microbial communities (Kümmerer, 2009; Carvalho et al., 2014).
Antibiotics pose a great threat to both saltwater and freshwater microorganisms, with concentrations as low as the ng/L range causing a variety of ecologically important disorders. In microorganisms exposed to antibiotics for only several hours, toxic effects such as decreased denitrification rate and inhibition of microorganism growth have been observed. Moreover, a major threat posed by exposure of microorganisms to antibiotics is the development of bacterial resistance to these compounds. The observed resistance was most often due to an increase in the abundance of resistance genes and/or the formation of a protective barrier by bacteria exposed to the tested antibiotic. When looking at the number of published studies, the effects of antibiotics are arguably the best understood. Another group of drugs that result in relatively high toxicity to both fresh and saltwater microorganisms are SSRIs. Both, antibiotics and SSRIs at low µg/L (i.e., environmentally relevant) concentrations caused decreases in microbial abundance and richness, pigment profile alteration and growth inhibition.
Some studies have demonstrated toxic effects of mixtures of pharmaceuticals from different therapeutic classes, such as NSAIDs, synthetic hormones, lipid-lowering agents and antibiotics on fresh and saltwater microorganisms although the observed effects were often similar to those of single compounds. However, it is important to keep in mind that the effects of a mixture cannot be easily attributed to individual compounds or classes of compounds, so it is difficult to determine which compound(s) in the mixture had the greatest effect as well as assessing synergistic and antagonistic effects on all but simple mixtures of only two or perhaps three pharmaceuticals. The major advantage of such studies is that they better represent environmental conditions where a large number and variety of pharmaceuticals are present. Therefore, both the toxicity of the mixture and of the single compounds need to be considered in future studies. Furthermore, in the case of mixtures, hormesis was additionally observed at lower concentrations. The hormetic response was often revealed by an increase in pigment content. In such pharmaceutical toxicity studies, the hormetic response to low doses is still poorly understood due to the number and complexity of cellular processes. Development of understanding the physiological mechanism(s) resulting in hermetic effects is considered an important goal in future studies as hormesis often coincides with experiments performed at environmentally relevant concentrations.
This review shows the trend of moving from traditional toxicity studies that use high pharmaceutical concentrations and focus on acute toxicity endpoints, to using environmentally relevant concentrations and studying more complex endpoints like effects on respiratory processes, primary production, nutrient cycling and gene expression. These processes provide key ecosystem services, and the data discussed in this review clearly indicate that pharmaceutical contaminants can pose an environmental threat by disrupting these processes. Studying the effects of pharmaceuticals at concentrations found in the waterways of interest is extremely important if the goal is to realistically assess the potential effects of these chemicals and future research should continue to move in this direction.
Of potential concern, only a few papers have focused on the effects of pharmaceuticals on microbial communities in saltwater ecosystems, with the majority of the still relatively small number of papers on this topic focused on freshwater habitats, mainly rivers and streams. Pharmaceutical contamination affects not only freshwater, but also saltwater, as evidenced by a number of papers in which pharmaceutical compounds have been detected in both saltwater and freshwater organisms.
Finally, this review has also highlighted that inferring effects of one pharmaceutical on the microbial community and its functioning based on similarity to another that has been tested can potentially be misleading, as several studies have demonstrated that even relatively similar compounds in the same pharmaceutical class can have quite different effects.
All authors listed have made a substantial, direct, and intellectual contribution to the work and approved it for publication.
The authors declare that the research was conducted in the absence of any commercial or financial relationships that could be construed as a potential conflict of interest.
All claims expressed in this article are solely those of the authors and do not necessarily represent those of their affiliated organizations, or those of the publisher, the editors and the reviewers. Any product that may be evaluated in this article, or claim that may be made by its manufacturer, is not guaranteed or endorsed by the publisher.
Agathokleous, E. (2018). Environmental hormesis, a fundamental non-monotonic biological phenomenon with implications in ecotoxicology and environmental safety. Ecotoxicol. Environ. Saf. 148, 1042–1053. doi:10.1016/j.ecoenv.2017.12.003
Agunbiade, F. O., and Moodley, B. (2016). Occurrence and distribution pattern of acidic pharmaceuticals in surface water, wastewater, and sediment of the Msunduzi River, Kwazulu-Natal, South Africa. Environ. Toxicol. Chem. 35 (1), 36–46. doi:10.1002/etc.3144
Ali, A. M., Rønning, H. T., Sydnes, L. K., Alarif, W. M., Kallenborn, R., and Al-Lihaibi, S. S. (2018). Detection of PPCPs in marine organisms from contaminated coastal waters of the Saudi Red Sea. Sci. Total Environ. 621, 654–662. doi:10.1016/j.scitotenv.2017.11.298
Álvarez-Muñoz, D., Rodríguez-Mozaz, S., Maulvault, A. L., Tediosi, A., Fernández-Tejedor, M., Van den Heuvel, F., et al. (2015). Occurrence of pharmaceuticals and endocrine disrupting compounds in macroalgaes, bivalves, and fish from coastal areas in Europe. Environ. Res. 143, 56–64. doi:10.1016/j.envres.2015.09.018
Amalfitano, S., Coci, M., Corno, G., and Luna, G. M. (2015). A microbial perspective on biological invasions in aquatic ecosystems. Hydrobiologia 746 (1), 13–22. doi:10.1007/s10750-014-2002-6
Aristi, I., von Schiller, D., Arroita, M., Barceló, D., Ponsatí, L., García-Galán, M. J., et al. (2015). Mixed effects of effluents from a wastewater treatment plant on river ecosystem metabolism: Subsidy or stress? Freshwwater Biol. 60, 1398–1410. doi:10.1111/fwb.12576
Aubertheau, E., Stalder, T., Mondamert, L., Ploy, M. C., Dagot, C., and Labanowski, J. (2017). Impact of wastewater treatment plant discharge on the contamination of river biofilms by pharmaceuticals and antibiotic resistance. Sci. Total Environ. 579, 1387–1398. doi:10.1016/j.scitotenv.2016.11.136
Backhaus, T., Porsbring, T., Arrhenius, Å., Brosche, S., Johansson, P., and Blanck, H. (2011). Single-substance and mixture toxicity of five pharmaceuticals and personal care products to marine periphyton communities. Environ. Toxicol. Chem. 30 (9), 2030–2040. doi:10.1002/etc.586
Battin, T. J., Besemer, K., Bengtsson, M. M., Romani, A. M., and Packmann, A. I. (2016). The ecology and biogeochemistry of stream biofilms. Nat. Rev. Microbiol. 14 (4), 251–263. doi:10.1038/nrmicro.2016.15
Beretta, M., Perelo, L. W., and de Oliveira, I. B. (2011). Quantification and toxicity testing of pharmaceuticals in tropical marine sediments, All Saints Bay, Bahia, Brazil. Microorg. Industry Environ. Sci. Industrial Res. Consumer Prod. 2010, 187–191. doi:10.1142/9789814322119_0042
Bernhardt, E. S., Rosi, E. J., and Gessner, M. O. (2017). Synthetic chemicals as agents of global change. Front. Ecol. Environ. 15 (2), 84–90. doi:10.1002/fee.1450
Bertilsson, S., and Jones, J. B. (2003). Supply of dissolved organic matter to aquatic ecosystems: Autochthonous sources. Aquat. Ecosyst. 2003, 3–24. doi:10.1016/B978-012256371-3/50002-0
Bertram, M. G., Martin, J. M., McCallum, E. S., Alton, L. A., Brand, J. A., Brooks, B. W., et al. (2022). Frontiers in quantifying wildlife behavioural responses to chemical pollution. Biol. Rev. 97, 1346. doi:10.1111/brv.12844
Borecka, M., Siedlewicz, G., Haliński, Ł. P., Sikora, K., Pazdro, K., Stepnowski, P., et al. (2015). Contamination of the southern baltic sea waters by the residues of selected pharmaceuticals: Method development and field studies. Mar. Pollut. Bull. 94 (1-2), 62–71. doi:10.1016/j.marpolbul.2015.03.008
Brosche, S., and Backhaus, T. (2010). Toxicity of five protein synthesis inhibiting antibiotics and their mixture to limnic bacterial communities. Aquat. Toxicol. 99 (4), 457–465. doi:10.1016/j.aquatox.2010.06.008
Brümmer, I. H. M., Fehr, W., and Wagner-Döbler, I. (2000). Biofilm community structure in polluted rivers: Abundance of dominant phylogenetic groups over a complete annual cycle. Appl. Environ. Microbiol. 66 (7), 3078–3082. doi:10.1128/aem.66.7.3078-3082.2000
Callieri, C., Eckert, E. M., Cesare, A. D., and Bertoni, F. (2018). Microbial communities. Encycl. Ecol. 1, 126–134. doi:10.1016/b978-0-12-409548-9.11222-9
Carvalho, P. N., Basto, M., Almeida, C. M. R., and Brix, H. (2014). A review of plant-pharmaceutical interactions: From uptake and effects in crop plants to phytoremediation in constructed wetlands. Environ. Sci. Pollut. Res. 21 (20), 11729–11763. doi:10.1007/s11356-014-2550-3
Chen, X., Chen, J., Yu, X., Sanganyado, E., Wang, L., Li, P., et al. (2022). Effects of norfloxacin, copper, and their interactions on microbial communities in estuarine sediment. Environ. Res. 212, 113506. doi:10.1016/j.envres.2022.113506
Chonova, T., Labanowski, J., Cournoyer, B., Chardon, C., Keck, F., Laurent, É., et al. (2018). River biofilm community changes related to pharmaceutical loads emitted by a wastewater treatment plant. Environ. Sci. Pollut. Res. 25, 9254–9264. doi:10.1007/s11356-017-0024-0
Christaki, E., Marcou, M., and Tofarides, A. (2020). Antimicrobial resistance in bacteria: Mechanisms, evolution, and persistence. J. Molecul. Evol. 88 (1), 26–40.
Chróst, R. J. (1990). Microbial ectoenzymes in aquatic environments. In aquatic microbial ecology. New York, NY: Springer, 47–78.
Corcoll, N., Casellas, M., Huerta, B., Guasch, H., Acuña, V., Rodríguez-Mozaz, S., et al. (2015). Effects of flow intermittency and pharmaceutical exposure on the structure and metabolism of stream biofilms. Sci. Total Environ. 503-504, 159–170. doi:10.1016/j.scitotenv.2014.06.093
Cordova-Kreylos, A. L., and Scow, K. M. (2007). Effects of ciprofloxacin on salt marsh sediment microbial communities. ISME J. 1 (7), 585–595. doi:10.1038/ismej.2007.71
Corno, G., Coci, M., Giardina, M., Plechuk, S., Campanile, F., and Stefani, S. (2014). Antibiotics promote aggregation within aquatic bacterial communities. Front. Microbiol. 5, 297. doi:10.3389/fmicb.2014.00297
Costa, S., Coppola, F., Pretti, C., Intorre, L., Meucci, V., Soares, A. M. V. M., et al. (2019). The influence of climate change related factors on the response of two clam species to diclofenac. Ecotoxicol. Environ. Saf. 189, 109899. doi:10.1016/j.ecoenv.2019.109899
Costello, D. M., Rosi-Marshall, E. J., Shaw, L. E., Grace, M. R., and Kelly, J. J. (2016). A novel method to assess effects of chemical stressors on natural biofilm structure and function. Freshw. Biol. 61, 2129–2140. doi:10.1111/fwb.12641
Cui, H., Zhou, W., Deng, Y., Zheng, B., Zhang, Q., Zhang, Z., et al. (2021). Meta-transcriptomic profiling of functional variation of freshwater microbial communities induced by an antidepressant sertraline hydrochloride. Sci. Total Environ. 786, 147434. doi:10.1016/j.scitotenv.2021.147434
Deng, Y., Debognies, A., Zhang, Q., Zhang, Z., Zhou, Z., Zhang, J., et al. (2022). Effects of ofloxacin on the structure and function of freshwater microbial communities. Aquat. Toxicol. 244, 106084. doi:10.1016/j.aquatox.2022.106084
Derakhsh, M. P., Mashinchian Moradi, A., Sharifpour, I., and Jamili, S. (2020). Toxic effects of diclofenac on gills, liver and kidney of Cyprinus carpio (Linnaeus, 1758). Iran. J. Fish. Sci. 19 (2), 735–747. doi:10.22092/ijfs.2018.119517
Fent, K., Weston, A. A., and Caminada, D. (2006). Ecotoxicology of human pharmaceuticals. Aquat. Toxicol. 76 (2), 122–159. doi:10.1016/j.aquatox.2005.09.009
Fernandes, J. P., Almeida, C. M. R., Pereira, A. C., Ribeiro, I. L., Reis, I., Carvalho, P., et al. (2015). Microbial community dynamics associated with veterinary antibiotics removal in constructed wetlands microcosms. Bioresour. Technol. 182, 26–33. doi:10.1016/j.biortech.2015.01.096
Galus, M., Rangarajan, S., Lai, A., Shaya, L., Balshine, S., and Wilson, J. Y. (2014). Effects of chronic, parental pharmaceutical exposure on zebrafish (Danio rerio) offspring. Aquat. Toxicol. 151, 124–134. doi:10.1016/j.aquatox.2014.01.016
Glöckner, F. O., Gasol, J. M., McDonough, N., and Calewaert, J. B. (2012). Marine microbial diversity and its role in ecosystem functioning and environmental change. belgium: European Science Foundation.
Gray, A. D., and Bernhardt, E. (2022). Are nitrogen and carbon cycle processes impacted by common stream antibiotics? A comparative assessment of single vs. mixture exposures. PloS One 17 (1), e0261714. doi:10.1371/journal.pone.0261714
Han, S., Choi, K., Kim, J., Ji, K., Kim, S., Ahn, B., et al. (2010). Endocrine disruption and consequences of chronic exposure to ibuprofen in Japanese medaka (Oryzias latipes) and freshwater cladocerans Daphnia magna and Moina macrocopa. Aquat. Toxicol. 98 (3), 256–264. doi:10.1016/j.aquatox.2010.02.013
Heckmann, L. H., Connon, R., Hutchinson, T. H., Maund, S. J., Sibly, R. M., and Callaghan, A. (2006). Expression of target and reference genes in Daphnia magna exposed to ibuprofen. BMC Genomics 7 (1), 175–178. doi:10.1186/1471-2164-7-175
Hellström, G., Klaminder, J., Finn, F., Persson, L., Alanärä, A., Jonsson, M., et al. (2016). GABAergic anxiolytic drug in water increases migration behaviour in salmon. Nat. Commun. 7 (1), 13460–13467. doi:10.1038/ncomms13460
Hiba, Z., Mondamert, L., Remaury, Q. B., Cleon, A., Leitner, N. K. V., and Labanowski, J. (2021). Occurrence of carbamazepine, diclofenac, and their related metabolites and transformation products in a French aquatic environment and preliminary risk assessment. Water Res. 196, 117052. doi:10.1016/j.watres.2021.117052
Hons, B. S. (2018). The impacts of pharmaceuticals on biogeochemical processes and basal resources in freshwater ecosystems. Doctoral dissertation. Australia: Monash University.
Hou, L., Yin, G., Liu, M., Zhou, J., Zheng, Y., Gao, J., et al. (2015). Effects of sulfamethazine on denitrification and the associated N2O release in estuarine and coastal sediments. Environ. Sci. Technol. 49 (1), 326–333. doi:10.1021/es504433r
Islas-Flores, H., Manuel Gómez-Oliván, L., Galar-Martínez, M., Michelle Sánchez-Ocampo, E., SanJuan-Reyes, N., Ortíz-Reynoso, M., et al. (2017). Cyto-genotoxicity and oxidative stress in common carp (Cyprinus carpio) exposed to a mixture of ibuprofen and diclofenac. Environ. Toxicol. 32 (5), 1637–1650. doi:10.1002/tox.22392
Johansson, C. H., Janmar, L., and Backhaus, T. (2014). Toxicity of ciprofloxacin and sulfamethoxazole to marine periphytic algae and bacteria. Aquat. Toxicol. 156, 248–258. doi:10.1016/j.aquatox.2014.08.015
Karr, J. R., and Chu, E. W. (2000). Introduction: Sustaining living rivers. In assessing the ecological integrity of running waters. Dordrecht: Springer.
Kergoat, L., Besse-Hoggan, P., Leremboure, M., Beguet, J., Devers, M., Martin-Laurent, F., et al. (2021). Environmental concentrations of sulfonamides can alter bacterial structure and induce diatom deformities in freshwater biofilm communities. Front. Microbiol. 12, 643719. doi:10.3389/fmicb.2021.643719
Kümmerer, K. (2009). The presence of pharmaceuticals in the environment due to human use-present knowledge and future challenges. J. Environ. Manag. 90 (8), 2354–2366. doi:10.1016/j.jenvman.2009.01.023
Lawrence, J. R., Swerhone, G. D. W., Topp, E., Korber, D. R., Neu, T. R., and Wassenaar, L. I. (2007). Structural and functional responses of river biofilm communities to the nonsteroidal anti-inflammatory diclofenac. Environ. Toxicol. Chem. 26, 573–582. doi:10.1897/06-340R.1
Lawrence, J. R., Swerhone, G. D. W., Wassenaar, L. I., and Neu, T. R. (2005). Effects of selected pharmaceuticals on riverine biofilm communities. Can. J. Microbiol. 51, 655–669. doi:10.1139/w05-047
Lu, T., Zhu, Y., Ke, M., Peijnenburg, W. J. G. M., Zhang, M., Wang, T., et al. (2019). Evaluation of the taxonomic and functional variation of freshwater plankton communities induced by trace amounts of the antibiotic ciprofloxacin. Environment Inter. 126, 268–278.
Lyautey, E., Jackson, C. R., Cayrou, J., Rols, J. L., and Garabétian, F. (2005). Bacterial community succession in natural river biofilm assemblages. Microb. Ecol. 50 (4), 589–601. doi:10.1007/s00248-005-5032-9
Madikizela, L. M., and Chimuka, L. (2017). Occurrence of naproxen, ibuprofen, and diclofenac residues in wastewater and river water of KwaZulu-Natal Province in South Africa. Environ. Monit. Assess. 189 (7), 348–412. doi:10.1007/s10661-017-6069-1
Maranho, L. A., Garrido-Pérez, M. C., Baena-Nogueras, R. M., Lara-Martín, P. A., Antón-Martín, R., DelValls, T. A., et al. (2015). Are WWTPs effluents responsible for acute toxicity? Seasonal variations of sediment quality at the bay of cádiz (SW, Spain). Ecotoxicology 24 (2), 368–380. doi:10.1007/s10646-014-1385-5
Martinez-Morcillo, S., Rodríguez-Gil, J. L., Fernández-Rubio, J., Rodriguez-Mozaz, S., Míguez-Santiyán, M. P., Valdes, M. E., et al. (2020). Presence of pharmaceutical compounds, levels of biochemical biomarkers in seafood tissues and risk assessment for human health: Results from a case study in north-western Spain. Int. J. Hyg. Environ. Health 223 (1), 10–21. doi:10.1016/j.ijheh.2019.10.011
Matviichuk, O., Mondamert, L., Geffroy, C., Gaschet, M., Dagot, C., and Labanowski, J. (2022). river biofilms microbiome and resistome responses to wastewater treatment plant effluents containing antibiotics. Front. Microbiol. 13, 795206. doi:10.3389/fmicb.2022.795206
Maul, J. D., Schuler, L. J., Belden, J. B., Whiles, M. R., and Lydy, M. J. (2006). Effects of the antibiotic ciprofloxacin on stream microbial communities and detritivorous macroinvertebrates. Environ. Toxicol. Chem. Int. J. 25 (6), 1598–1606. doi:10.1897/05-441r.1
McClean, P., and Hunter, W. R. (2020). 17α-ethynylestradiol (EE2) limits the impact of ibuprofen upon respiration by streambed biofilms in a sub-urban stream. Environ. Sci. Pollut. Res. 27, 37149–37154. doi:10.1007/s11356-020-10096-5
Mezzelani, M., Gorbi, S., Da Ros, Z., Fattorini, D., d’Errico, G., Milan, M., et al. (2016). Ecotoxicological potential of non-steroidal anti-inflammatory drugs (NSAIDs) in marine organisms: Bioavailability, biomarkers and natural occurrence in Mytilus galloprovincialis. Mar. Environ. Res. 121, 31–39. doi:10.1016/j.marenvres.2016.03.005
Näslund, J., Hedman, J. E., and Agestrand, C. (2008). Effects of the antibiotic ciprofloxacin on the bacterial community structure and degradation of pyrene in marine sediment. Aquat. Toxicol. 90 (3), 223–227. doi:10.1016/j.aquatox.2008.09.002
Nikolaou, A., Meric, S., and Fatta, D. (2007). Occurrence patterns of pharmaceuticals in water and wastewater environments. Anal. Bioanal. Chem. 387 (4), 1225–1234. doi:10.1007/s00216-006-1035-8
Nunes, B., Daniel, D., Canelas, G. G., Barros, J., and Correia, A. T. (2020). Toxic effects of environmentally realistic concentrations of diclofenac in organisms from two distinct trophic levels, Hediste diversicolor and Solea senegalensis. Comp. Biochem. Physiology Part C Toxicol. Pharmacol. 231, 108722. doi:10.1016/j.cbpc.2020.108722
Ogata, E. M., Baker, M. A., Rosi, E. J., Smart, T. B., Long, D., and Aanderud, Z. T. (2020). Nutrients and pharmaceuticals structure bacterial core communities in urban and montane stream biofilms. Front. Microbiol. 11, 526545–526615. doi:10.3389/fmicb.2020.526545
O’Neill, J. (2014). Antimicrobial Resistance: Tackling a crisis for the health and wealth of nations
Parolini, M., Binelli, A., Cogni, D., Riva, C., and Provini, A. (2009). An in vitro biomarker approach for the evaluation of the ecotoxicity of non-steroidal anti-inflammatory drugs (NSAIDs). Toxcilogy Vitro 23, 935–942. doi:10.1016/j.tiv.2009.04.014
Paumelle, M., Donnadieu, F., Joly, M., Besse-Hoggan, P., and Artigas, J. (2021). Effects of sulfonamide antibiotics on aquatic microbial community composition and functions. Environ. Int. 146, 106198. doi:10.1016/j.envint.2020.106198
Peele, E. R., Singleton, F. L., Deming, J. W., Cavari, B., and Colwell, R. R. (1981). Effects of pharmaceutical wastes on microbial populations in surface waters at the Puerto Rico dump site in the Atlantic Ocean. Appl. Environ. Microbiol. 41 (4), 873–879. doi:10.1128/aem.41.4.873-879.1981
Pesce, S., Kergoat, L., Paris, L., Billet, L., Besse-Hoggan, P., and Bonnineau, C. (2021). Contrasting effects of environmental concentrations of sulfonamides on microbial heterotrophic activities in freshwater sediments. Front. Microbiol. 12, 753647. doi:10.3389/fmicb.2021.753647
Porsbring, T., Blanck, H., Tjellström, H., and Backhaus, T. (2009). Toxicity of the pharmaceutical clotrimazole to marine microalgal communities. Aquat. Toxicol. 91 (3), 203–211. doi:10.1016/j.aquatox.2008.11.003
Proia, L., Cassió, F., Pascoal, C., Tlili, A., and Romaní, A. M. (2012). The use of attached microbial communities to assess ecological risks of pollutants in river ecosystems: The role of heterotrophs. Emerg. Prior. Pollut. Rivers 2012, 55–83. doi:10.1007/978-3-642-25722-3_3
Proia, L., Lupini, G., Osorio, V., Pérez, S., Barceló, D., Schwartz, T., et al. (2013). Response of biofilm bacterial communities to antibiotic pollutants in a Mediterranean river. Chemosphere 92 (9), 1126–1135. doi:10.1016/j.chemosphere.2013.01.063
Proia, L., Osorio, V., Soley, S., Köck-Schulmeyer, M., Pérez, S., Barceló, D., et al. (2013). Effects of pesticides and pharmaceuticals on biofilms in a highly impacted river. Environ. Pollut. 178, 220–228. doi:10.1016/j.envpol.2013.02.022
Pu, Y., Pan, J., Yao, Y., Ngan, W. Y., Yang, Y., Li, M., et al. (2021). Ecotoxicological effects of erythromycin on a multispecies biofilm model, revealed by metagenomic and metabolomic approaches. Environ. Pollut. 276, 116737. doi:10.1016/j.envpol.2021.116737
Quinlan, E. L., Nietch, C. T., Blocksom, K., Lazorchak, J. M., Batt, A. L., Griffiths, R., et al. (2011). Temporal dynamics of periphyton exposed to tetracycline in stream mesocosms. Environ. Sci. Technol. 45 (24), 10684–10690. doi:10.1021/es202004k
Richmond, E. K., Grace, M. R., Kelly, J. J., Reisinger, A. J., Rosi, E. J., and Walters, D. M. (2017). Pharmaceuticals and personal care products (PPCPs) are ecological disrupting compounds (EcoDC). Elementa 5, 252. doi:10.1525/elementa.252
Richmond, E. K., Rosi, E. J., Reisinger, A. J., Hanrahan, B. R., Thompson, R. M., and Grace, M. R. (2019). Influences of the antidepressant fluoxetine on stream ecosystem function and aquatic insect emergence at environmentally realistic concentrations. J. Freshw. Ecol. 34 (1), 513–531. doi:10.1080/02705060.2019.1629546
Richmond, E. K., Rosi, E. J., Walters, D. M., Fick, J., Hamilton, S. K., Brodin, T., et al. (2018). A diverse suite of pharmaceuticals contaminates stream and riparian food webs. Nat. Commun. 9 (1), 4491–4499. doi:10.1038/s41467-018-06822-w
Richmond, E. K., Rosi-Marshall, E. J., Lee, S. S., Thompson, R. M., and Grace, M. R. (2016). Antidepressants in stream ecosystems: Influence of selective serotonin reuptake inhibitors (SSRIs) on algal production and insect emergence. Freshw. Sci. 35, 845–855. doi:10.1086/687841
Rico, A., Dimitrov, M. R., Van Wijngaarden, R. P., Satapornvanit, K., Smidt, H., and Van den Brink, P. J. (2014). Effects of the antibiotic enrofloxacin on the ecology of tropical eutrophic freshwater microcosms. Aquat. Toxicol. 147, 92–104. doi:10.1016/j.aquatox.2013.12.008
Robson, S. V., Rosi, E. J., Richmond, E. K., and Grace, M. R. (2020). Environmental concentrations of pharmaceuticals alter metabolism, denitrification, and diatom assemblages in artificial streams. Freshw. Sci. 39, 256–267. doi:10.1086/708893
Rocha, S., Domingues, V. F., Pinho, C., Fernandes, V. C., Delerue-Matos, C., Gameiro, P., et al. (2013). Occurrence of bisphenol A, estrone, 17β-estradiol and 17α-ethinylestradiol in Portuguese rivers. Bull. Environ. Contam. Toxicol. 90, 73–78. doi:10.1007/s00128-012-0887-1
Rosi, E. J., Bechtold, H. A., Snow, D., Rojas, M., Reisinger, A. J., and Kelly, J. J. (2018). Urban stream microbial communities show resistance to pharmaceutical exposure. Ecosphere 9, 2041. doi:10.1002/ecs2.2041
Rosi-Marshall, E. J., Kincaid, D. W., Bechtold, H. A., Royer, T. V., Rojas, M., and Kelly, J. J. (2013). Pharmaceuticals suppress algal growth and microbial respiration and alter bacterial communities in stream biofilms. Ecol. Appl. 23, 583–593. doi:10.1890/12-0491.1
Sabater, S., Guasch, H., Romaní, A., and Muñoz, I. (2002). The effect of biological factors on the efficiency of river biofilms in improving water quality. Hydrobiologia 469 (1), 149–156. doi:10.1023/a:1015549404082
Schwarz, S., Schmieg, H., Scheurer, M., Köhler, H. R., and Triebskorn, R. (2017). Impact of the NSAID diclofenac on survival, development, behaviour and health of embryonic and juvenile stages of Brown trout, Salmo trutta f. fario. Sci. Total Environ. 607, 1026–1036. doi:10.1016/j.scitotenv.2017.07.042
Shaw, L., Phung, C., and Grace, M. (2015). Pharmaceuticals and personal care products alter growth and function in lentic biofilms. Environ. Chem. 12 (3), 301–306. doi:10.1071/en14141
Sullivan, S. M. P., and Rodewald, A. D. (2012). In a state of flux: The energetic pathways that move contaminants from aquatic to terrestrial environments. Environ. Toxicol. Chem. 31 (6), 1175–1183.
Sibeko, A. P., Naicker, D., Mdluli, P. S., and Madikizela, L. M. (2019). Naproxen, ibuprofen, and diclofenac residues in river water, sediments and Eichhornia crassipes of mbokodweni river in south Africa: An initial screening. Environ. Forensics 20, 129. doi:10.1080/15275922.2019.1597780
Świacka, K., Maculewicz, J., Świeżak, J., Caban, M., and Smolarz, K. (2022). A multi-biomarker approach to assess toxicity of diclofenac and 4-OH diclofenac in Mytilus trossulus mussels-First evidence of diclofenac metabolite impact on molluscs. Environ. Pollut. 315, 120384. doi:10.1016/j.envpol.2022.120384
Tang, J., Sun, J., Wang, W., Yang, L., and Xu, Y. (2021). Pharmaceuticals in two watersheds in Eastern China and their ecological risks. Environ. Pollut. 277, 116773. doi:10.1016/j.envpol.2021.116773
UNESCO and HELCOM (2017). Pharmaceuticals in the aquatic environment of the baltic sea region: A status report UNESCO Series on Emerging Pollutants in Water. Available at: https://unesdoc.unesco.org/ark:/48223/pf0000247889.
Vera-Chang, M. N., St-Jacques, A. D., Gagné, R., Martyniuk, C. J., Yauk, C. L., Moon, T. W., et al. (2018). Transgenerational hypocortisolism and behavioral disruption are induced by the antidepressant fluoxetine in male zebrafish Danio rerio. Proc. Natl. Acad. Sci. 115 (52), E12435–E12442. doi:10.1073/pnas.1811695115
Vidal-Dorsch, D. E., Bay, S. M., Maruya, K., Snyder, S. A., Trenholm, R. A., and Vanderford, B. J. (2012). Contaminants of emerging concern in municipal wastewater effluents and marine receiving water. Environ. Toxicol. Chem. 31 (12), 2674–2682. doi:10.1002/etc.2004
Wang, C., Dong, D., Zhang, L., Song, Z., Hua, X., and Guo, Z. (2019). Response of freshwater biofilms to antibiotic florfenicol and ofloxacin stress: Role of extracellular polymeric substances. Int. J. Environ. Res. Public Health 16 (5), 715. doi:10.3390/ijerph16050715
Wang, Z., Yin, S., Chou, Q., Zhou, D., Jeppesen, E., Wang, L., et al. (2022). Community-level and function response of photoautotrophic periphyton exposed to oxytetracycline hydrochloride. Environ. Pollut. 294, 118593. doi:10.1016/j.envpol.2021.118593
Wu, C., Spongberg, A. L., Witter, J. D., Fang, M., and Czajkowski, K. P. (2010). Uptake of pharmaceutical and personal care products by soybean plants from soils applied with biosolids and irrigated with contaminated water. Environ. Sci. Technol. 44 (16), 6157–6161. doi:10.1021/es1011115
Xu, H., Lu, G., and Xue, C. (2020). Effects of sulfamethoxazole and 2-ethylhexyl-4-methoxycinnamate on the dissimilatory nitrate reduction processes and N2O release in sediments in the Yarlung Zangbo river. Int. J. Environ. Res. Public Health 17 (6), 1822.
Yang, Z., Lu, T., Zhu, Y., Zhang, Q., Zhou, Z., Pan, X., et al. (2019). Aquatic ecotoxicity of an antidepressant, sertraline hydrochloride, on microbial communities. Sci. Total Environ. 654, 129–134. doi:10.1016/j.scitotenv.2018.11.164
Yergeau, E., Lawrence, J. R., Waiser, M. J., Korber, D. R., and Greer, C. W. (2010). Metatranscriptomic analysis of the response of river biofilms to pharmaceutical products, using anonymous DNA microarrays. Appl. Environ. Microbiol. 76 (16), 5432–5439. doi:10.1128/aem.00873-10
Yergeau, E., Sanschagrin, S., Waiser, M. J., Lawrence, J. R., and Greer, C. W. (2012). Sub-inhibitory concentrations of different pharmaceutical products affect the meta-transcriptome of river biofilm communities cultivated in rotating annular reactors. Environ. Microbiol. Rep. 4 (3), 350–359. doi:10.1111/j.1758-2229.2012.00341.x
Zhang, X., Gao, Y., Li, Q., Li, G., Guo, Q., and Yan, C. (2011). Estrogenic compounds and estrogenicity in surface water, sediments, and organisms from Yundang Lagoon in Xiamen, China. Archives Environ. Contam. Toxicol. 61 (1), 93–100. doi:10.1007/s00244-010-9588-0
Zhou, Z., Zhang, Z., Feng, L., Zhang, J., Li, Y., Lu, T., et al. (2020). Adverse effects of levofloxacin and oxytetracycline on aquatic microbial communities. Sci. Total Environ. 734, 139499. doi:10.1016/j.scitotenv.2020.139499
Keywords: drugs, toxicity, emerging contaminants, microorganisms, microbes, biofilm, environmental processes, antibiotics
Citation: Świacka K, Maculewicz J, Kowalska D and Grace MR (2023) Do pharmaceuticals affect microbial communities in aquatic environments? A review. Front. Environ. Sci. 10:1093920. doi: 10.3389/fenvs.2022.1093920
Received: 09 November 2022; Accepted: 16 December 2022;
Published: 09 January 2023.
Edited by:
Xiaohui Liu, Chinese Research Academy of Environmental Sciences, ChinaReviewed by:
Zhi Wang, Innovation Academy for Precision Measurement Science and Technology (CAS), ChinaCopyright © 2023 Świacka, Maculewicz, Kowalska and Grace. This is an open-access article distributed under the terms of the Creative Commons Attribution License (CC BY). The use, distribution or reproduction in other forums is permitted, provided the original author(s) and the copyright owner(s) are credited and that the original publication in this journal is cited, in accordance with accepted academic practice. No use, distribution or reproduction is permitted which does not comply with these terms.
*Correspondence: Klaudia Świacka, a2xhdWRpYS5zd2lhY2thQHBoZHN0dWQudWcuZWR1LnBs
Disclaimer: All claims expressed in this article are solely those of the authors and do not necessarily represent those of their affiliated organizations, or those of the publisher, the editors and the reviewers. Any product that may be evaluated in this article or claim that may be made by its manufacturer is not guaranteed or endorsed by the publisher.
Research integrity at Frontiers
Learn more about the work of our research integrity team to safeguard the quality of each article we publish.