- College of Architecture and Art, Hefei University of Technology, Hefei, China
The adsorption of particulate matter (PM) by the leaves of urban plants can effectively reduce the particulate matter concentration in the atmosphere. The use of climbing plants to abate particulate matter is an important means of urban greening in China, which is rich in climbing plant species. In this study, three evergreen climbing plants were selected to assess their ability to adsorb particulate matter and determine their physiological responses to particulate pollution. PM deposition was determined gravimetrically. There were four key results. 1) The adsorption capacity of the three evergreen climbers followed the order of Hedera nepalensis var > Ficus pumila Linn > Euonymus fortunei, and was significantly higher in an overpass environment than in a campus environment for all three species. 2) The seasonal characteristics of particulate adsorption by the three types of climbers in both the overpass and campus environments followed the order of winter > autumn > spring > summer. 3) The net photosynthetic rate of the three climbing plants was significantly affected by particulate matter in a size dependent manner, with fine particulate matter on the plant leaf surface and coarse particulate matter in the leaf waxy layer being important factors affecting plant photosynthesis. 4) Particulate pollution led to changes in the activities of plant protective enzymes in plants, with increases in the superoxide dismutase (SOD) and peroxidase (POD) activity, and the malondialdehyde (MDA) and soluble protein contents. There were different trends in the soluble sugar content among the different plant species. In conclusion, F. pumila had a strong resistance to particulate pollutants, while H. nepalensis was considered suitable for planting in polluted areas where it could improve ambient air quality by adsorbing large amounts of particulate matter.
1 Introduction
With the ongoing urbanization and industrial development in China, particulate matter (PM) has become the main atmospheric pollutant in most cities and is used as an important indicator in the evaluation of urban air quality (Zou et al., 2015; Segalin et al., 2016; Li et al., 2019; Nisha et al., 2021). The sources of PM are complex and difficult to manage, with motor vehicle emissions, fossil fuel combustion, and industrial dust being the main sources. Particulate matter is a mixture of harmful substances and other suspended matter in the atmosphere, and is of particular concern due to its extreme toxicity to humans, resulting in more serious health effects than any other air pollutant (WHO, 2019). Various epidemiological studies have shown an association between PM and adverse health effects, including exacerbation of chronic respiratory and cardiovascular disease, impaired lung function, and premature death (Shaughnessy et al., 2015). The severity of the human health effects due to PM exposure varies according to the particle size, with PM2.5 being the most toxic size fraction (Gu et al., 2012; Du et al., 2016; Xiong et al., 2021).
In addition to reducing pollutant emissions, planting vegetation is an effective means of mitigating PM pollution and improving the atmospheric environment of urban areas. Green plants have the potential to clean polluted air by capturing PM on the leaf surface (Chen et al., 2017; Panda et al., 2018; Choi et al., 2021; Zhang et al., 2021), and if PM contains lipophilic organic pollutants, additional sequestration can occur when the particles penetrate the leaf wax (Dzierzanowski et al., 2011). The ability of plants to retain PM is not only related to the planted area, but also has a strong correlation with the plant leaf surface morphology. It has been reported that plant leaves with fuzzy, furrowed surfaces have a stronger dust retention capacity than leaves with smooth, thin, waxy layers (De Nicola et al., 2008; Sæbø et al., 2012; Jaconis et al., 2017). The overall plant retention of PM is a combination of retention on the leaf surface and in the waxy layer, and it has also been shown that the thickness of the waxy layer is a key factor affecting the plant retention of PM (Sun et al., 2018; Choi et al., 2021). Atmospheric pollutants, including PM, can cause changes in plant physiological parameters such as pigment deterioration, changes in antioxidant enzyme activity, production of antioxidant metabolites, and membrane damage (Chaudhary and Rathore., 2018; Singh et al., 2018). It has been reported that PM may block plant stomata leading to a decrease in the net photosynthetic rate and may also cause additional radiation absorption by leaves leading to an increase in leaf surface temperature (Popek et al., 2018; Li M et al., 2021), which can alter the enzyme activity in plants. In addition, PM may also lead to an imbalance in osmotic pressure and an increase in free radicals in plants (Singh et al., 2020). It has been found that the soluble protein content decreases when French sycamore and oleander are exposed to industrial and urban pollution, while peroxidase activity increases (Doğanlar and Atmaca, 2011). Singh observed a significant increase in oxidative stress in mustard under exposure to ozone (O3), leading to a significant decrease in the photosynthetic pigment and soluble protein content in leaves, while strengthening antioxidant defense systems to resist its effects (Singh et al., 2011; Singh et al., 2020). In summary, PM can enter plants through stomata and affect them by inducing the formation of reactive oxygen species and redox-sensitive signaling pathways that exert toxicity through the synergistic effects of oxidative stress and inflammation (Michael et al., 2013). Therefore, plant response to PM pollution is also one of the most important indicators considered in the selection of tree species used for urban greening.
China has a rich variety of climbing plants, most of which have high ornamental value. Climbing plants do not occupy space at the street level, but use existing building walls, or vertical greening methods on highways and slopes. Vertical greening using climbing plants in urban areas not only expands urban garden space and increases the artistic beauty of urban architecture, but also increases urban green coverage. Climbing plants can also serve as an ideal natural filter to mitigate air pollution (Naomi et al., 2020). However, there have been few studies of the adsorption effects of climbing plants on atmospheric PM. This study analyzed and compared the adsorption of PM by three evergreen climbing plants at different levels of PM pollution and assessed their physiological responses to PM pollution. The results can be used in the selection of climbing species with high PM adsorption and resistance, and will provide a reference for the construction of urban forests.
2 Materials and methods
2.1 Study area and sampling collection
To ensure that the test results were representative of the greening characteristics of the main climbing plants in Nanjing, we selected Himalayan ivy [Hedera nepalensis var. sinensis (Tobl.) Rehd], climbing fig (Ficus pumila Linn), and Fu Fang vine [Euonymus fortunei (Turcz) Hand Mazz] as the test subjects for preliminary research. The climbing plants were growing on bridge pillars in an overpass and on a wall in a university campus. Sampling sites were located at Nanjing Forestry University campus and Yingtian overpass in Nanjing, China. Yingtian overpass is an important section of the Nanjing Inner Ring Expressway. Traffic is heavy, and air pollution caused by vehicle exhaust is a serious problem around the sampling site. Samples were collected in May, August, and November 2021 and January 2022. To evaluate the dust retention ability of different species of climbing plants for different sizes of particulate matter, we selected 28 consecutive days without rainfall for sampling. This ensured that the dust retention of each type of climbing plant reached a saturation value for particulate matter (Liu et al., 2012).
In this study, mature individuals of three common climbing tree species were studied. To ensure that a representative sample was investigated, a range of tree forms, landscape applications, growth conditions, and leaf characteristics were included. Plants that were in good condition with luxuriant foliage, no pests, and aged between 25 and 30 years were selected. For each species of tree, three climbing trees were selected, and leaves collected randomly in four directions (east, south, west, and north) of column, and in four directions (up, down, left, and right) at 1.0–2.5 m above ground, depending on the plant structure with a pruner. Five sample trees were collected for each climbing tree species, as well as 10 pieces in each direction per plant (200 pieces in total for five plants) for H. nepalensis. E. fortune and F. pumila, from which 15 leaf samples in each direction were collected, for a total of 60 pieces (300 pieces in total for five plants) as their leaves were small. Samples for the determination of particulate retention were placed in self-sealing bags and brought back to the laboratory. The surface of each leaf was wiped clean and immediately soaked with liquid nitrogen. All samples were placed back into the self-sealing bags and stored in an ultra-low-temperature refrigerator prior to the enzyme assay.
2.2 Extraction and determination of PM on the leaf surface and from the waxy layer
Dust retention was determined using Przbysz’s method (Przbysz et al., 2014). Filter papers with pore sizes of 10, 2.5, and 0.2 μm were placed inside half-open weighing bottles in an oven at 60°C for 60 min (Dzierżanowski et al., 2011; Chiam et al., 2019; Kończak et al., 2021; Li X. L et al., 2021). The bottles were then covered for 60 min, removed, and placed in a drying dish until a constant weight was obtained. The dried filters were placed on an analytical balance (readability = 0.0001 g) (ME204E, Mettler Toledo, Columbus, OH, United States) and weighed to obtain both the initial weight of each filter paper (g) and the total initial weight of the weighing bottle and filter paper (g).
Particulate matter on the surface of the leaves that could be washed off by water was referred to as “particulate matter on the surface (sPM).” Leaves were placed into beakers with deionized water and stirred for 1 min with a glass rod. The beakers were placed in an ultrasonic cleaner (JP-020, Skymen, Guangdong, China) and shaken for 1 min to remove the PM adsorbed on the leaf surface. The resulting solution was passed through a standard splitting sieve (160 mesh, pore size of about 100 μm), followed by filtering in turn through filter papers with pore sizes of 10 and 2.5 μm to obtain three particle size ranges: large particles of 10–100 μm, coarse particles of 2.5–10 μm, and fine particles of 0.2–2.5 μm. The filter paper was placed into an oven to dry, removed, and left to stand in a drying dish. After reaching a constant weight, the weight of each filter paper after filtration (g) and the total weight of the weighing bottle and filter paper after filtration (g) were determined using an analytical balance. The amount of “surface PM” attached to each sample at each particle size was calculated using the weight difference method.
The mass of PM contained in wax layer (wPM) was detected the rinse and weight method (Dzierżanowski et al., 2011; Chiam et al., 2019). Clean leaf samples were rinsed with deionized water and then rinsed again for 40 s in 150 cm3 of chloroform to dissolve the epicuticular waxes and wash the micro-particles fixed in the wax to obtain the wPM fraction. The liquid obtained from the rinsing was filtered continuously through a filter according to the procedure used to filter aqueous fractions containing surface PM, with the difference that the chloroform remaining after filtration was poured into an empty pre-weighted clean flask. After the chloroform evaporation (more than 10 or even 48 h), the flasks were weighed on a laboratory microbalance. Then, the difference between the masses of the empty flask and flask containing wax was determined. Filtration was performed following the same procedure used for sPM washing. After drying, the filters were also weighed and the PM was determined based on the difference in weight.
2.3 Leaf area determination
After drying, the leaves were scanned using an electronic scanner (V370, Epson, Nagaon, Japan) and then imported into ImageJ 1.48 software to determine the leaf area, with the leaf area of each sample obtained as S (cm2).
2.4 Measurement of the photosynthetic parameters of plant leaves
Four leaves from each climbing plant were selected from the upper, middle, and lower well-developed mature parts of the climbing plant. The analytical instrument used for the measurements was a Li-6400 portable photosynthesis meter (Li-COR, Lincoln, NE, United States). Gas exchange parameters, including the maximum net photosynthetic rate (Pn), stomatal conductance (Gs), intercellular carbon dioxide (CO2) concentration (Ci), and transpiration rate (Tr), were measured in May, August, and November 2021 and January 2022 for the tagged leaves. Mature leaves with healthy growth and a similar growth status were selected for measurements from 09:00 to 11:00 after eight consecutive days without precipitation.
2.5 Determination of the biochemical indexes of plant leaves
About 0.5 g of intact and similarly colored leaf samples were randomly selected from each package of leaves, cut into small pieces, and placed in 5 ml centrifuge tubes with 2 ml of phosphate buffer. The samples were ground with an automatic sample rapid grinder (JXFSTPRP-24, Jingxin Technology, Shandong, China) and then the centrifuge tube was rinsed with extraction medium. The final volume was fixed to 5 ml with the extraction medium, and then the supernatant was extracted with a ST8R centrifuge (Thermo Fisher Scientific, Waltham, MA, United States) at 4,500 rpm for 15 min. The supernatant was then extracted with a 10 ml pipette for the determination of superoxide dismutase (SOD) and peroxidase (POD) activity. Enzyme extract (2.5 ml) was added to 2.5 ml of distilled water for the determination of malondialdehyde (MDA) and soluble protein content.
2.6 Measurement of the photosynthetic parameters of plant leaves
About 0.5 g of intact and similarly colored leaf samples were randomly selected from each package of leaves, cut into small pieces, and placed in 5 ml centrifuge tubes with 2 ml of phosphate buffer. The samples were ground with an automatic sample rapid grinder (JXFSTPRP-24, Jingxin Technology, Shandong, China) and then the centrifuge tube was rinsed with extraction medium. The final volume was fixed to 5 ml with the extraction medium, and then the supernatant was extracted with a ST8R centrifuge (Thermo Fisher Scientific, Waltham, MA, United States) at 4,500 rpm for 15 min. The supernatant was then extracted with a 10 ml pipette for the determination of superoxide dismutase (SOD) and peroxidase (POD) activity. Enzyme extract (2.5 ml) was added to 2.5 ml of distilled water for the determination of malondialdehyde (MDA) and soluble protein content.
2.7 Data processing
The experimental data were subjected to both a one-way and two-way analysis of variance (ANOVA) using SPSS22.0 software, with multiple testing applied to detect whether statistical differences existed between the treatments. Independent sample t-tests were used to rapidly analyze the differences between the physiological and biochemical indices of plants located at the campus and the overpass. Pearson’s correlation coefficient was used to analyze the relationship between plant dust retention capacity and photosynthetic gas parameters. Plots were constructed using Origin 2016 software.
3 Results
3.1 The mass of PM retained on leaf surface in different seasons
There were significant differences in the mass of the different sizes of PM among the different species in spring (Figure 1). Both campus and overpass H. nepalensis had a significantly higher adsorption capacity for total suspended particulate (TSP) and the different particle size classes of PM than the other two climbers, with overpass H. nepalensis adsorbing sPM (18.97 ± 2.03 μg cm−2) (Figure 1A), sPM10–100 (12.51 ± 1.56 μg cm−2) (Figure 1B), sPM2.5–10 (5.13 ± 0.38 μg cm−2) (Figure 1C), and sPM0.2–2.5 (1.33 ± 0.15 μg cm−2) (Figure 1D). In addition, the adsorption of PM2.5–10 was significantly higher (p < 0.05) in F. pumila at the overpass than in E. fortunei, and the adsorption of PM0.2–2.5 was significantly higher (p < 0.05) in E. fortunei than in F. pumila.
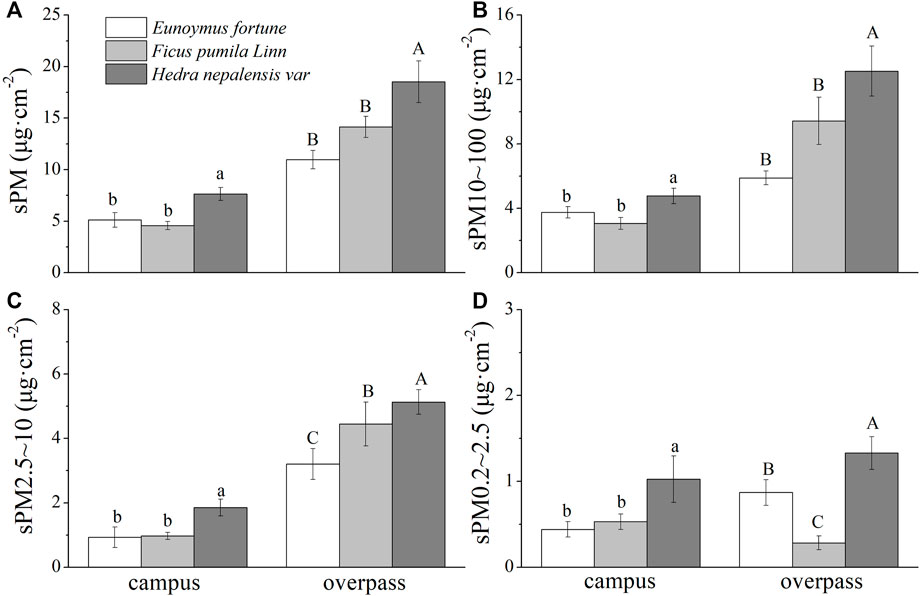
FIGURE 1. The mass of retained particulate matter on the leaf surface in spring (mean ± SE). (A) The total mass of particulate matter. (B) The mass of PM10–100. (C) The mass of PM2.5–10. (D) The mass of PM0.2–2.5.*Bars with different letters indicate signigicant differenc (p < 0.05) between the sites at a specific season. The same below.
The maximum retention of the different particle sizes by H. nepalensis in summer at the overpass was sPM (15.94 ± 1.67 μg cm−2) (Figure 2A), sPM10–100 (11.99 ± 0.75 μg cm−2) (Figure 2B), sPM2.5–10 (2.60 ± 0.13 μg cm−2) (Figure 2C), and sPM0.2–2.5 (1.35 ± 0.11 μg cm−2) (Figure 2D). Campus H. nepalensis adsorbed significantly more sPM and sPM10–100 than the other two climbers, and campus H. nepalensis and E. fortunei adsorbed significantly more sPM0.2–2.5 than F. pumila (p < 0.05) There was no significant difference in the adsorption of sPM2.5–10 among the three plants. The adsorption of sPM and the different particle size classes by H. nepalensis at the overpass was significantly higher than that by E. fortunei (p < 0.05). There was no significant difference between the adsorption of sPM, sPM10–100 and sPM2.5–10 by H. nepalensis and F. pumila, but the adsorption of sPM0.2–2.5 by the three climbing plants was significantly different (p < 0.05).
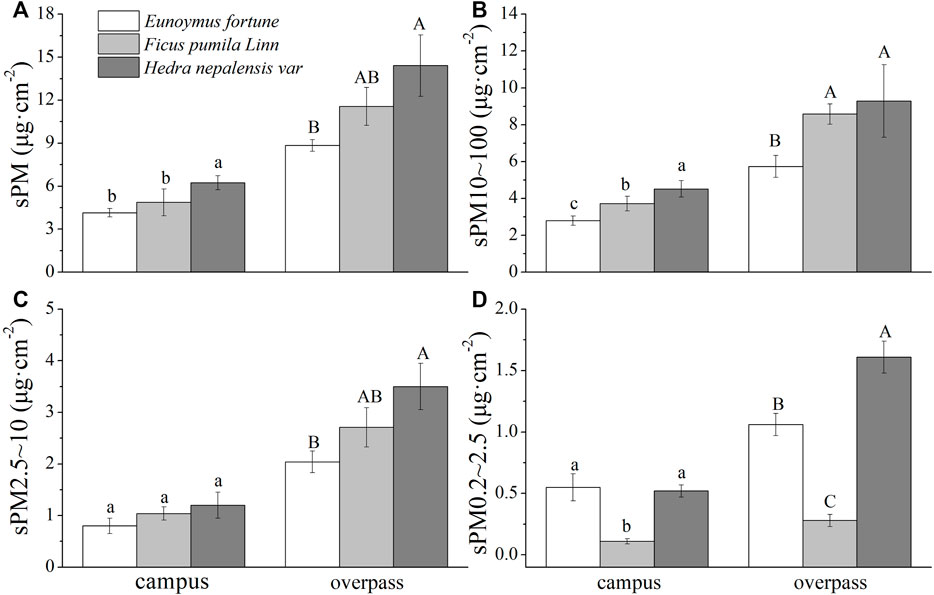
FIGURE 2. The mass of retained particulate matter on the leaf surface in summer (mean ± SE). (A) The total mass of particulate matter. (B) The mass of PM10–100. (C) The mass of PM2.5–10. (D) The mass of PM0.2–2.5.
In autumn, H. nepalensis at the overpass had the highest adsorption among the particles of different particle sizes, sPM (14.41 ± 2.14 μg cm−2) (Figure 3A), s PM10–100 (9.29 ± 1.97 μg cm−2) (Figure 3B), sPM2.5–10 (3.50 ± 0.45 μg cm−2) (Figure 3C), and sPM0.2–2.5 (1.61 ± 0.13 μg cm−2) (Figure 3D). A one-way ANOVA showed that there was no significant difference in sPM adsorption by the three climbers at the campus. The adsorption of sPM10–100 and sPM2.5–10 by H. nepalensis was significantly higher than that of F. pumila and E. fortunei (p < 0.05), and the adsorption of sPM0.2–2.5 by H. nepalensis and F. pumila was significantly higher than that of E. fortunei (p < 0.05). The adsorption of sPM and sPM2.5–10 by H. nepalensis and F. pumila at the overpass was significantly higher than that of E. fortunei (p < 0.05). The adsorption of sPM10–100 by all three plants at the overpass was significantly different (p < 0.05), and the adsorption of sPM0.2–2.5 by F. pumila was significantly higher than that of E. fortunei (p < 0.05).
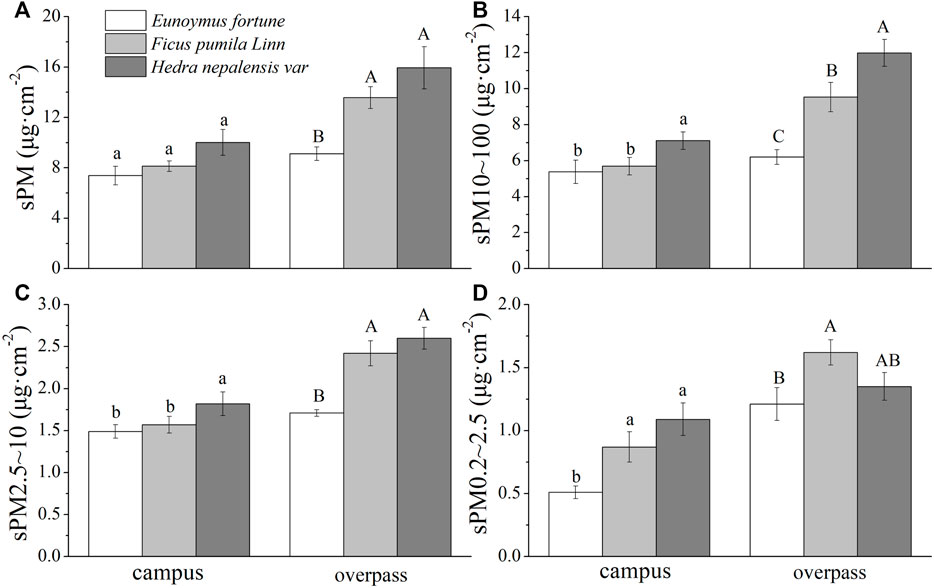
FIGURE 3. The mass of retained particulate matter on the leaf surface in autumn (mean ± SE). (A) The total mass of particulate matter. (B) The mass of PM10–100. (C) The mass of PM2.5–10. (D) The mass of PM0.2–2.5.
The maximum adsorption of the different particle sizes in winter at the overpass for H. nepalensis was: sPM (21.35 ± 2.01 μg cm−2) (Figure 4A), sPM10–100 (14.99 ± 1.36 μg cm−2) (Figure 4B), sPM2.5–10 (3.49 ± 0.31 μg cm−2) (Figure 4C), and sPM0.2–2.5 (2.87 ± 0.15 μg cm−2) (Figure 4D). The adsorption patterns of the three climbers for sPM and sPM10–100 were consistent across sampling sites, with all having a significantly higher adsorption of sPM and sPM10–100 by H. nepalensis than by F. pumila and E. fortunei (p < 0.05). The adsorption of sPM2.5–10 and sPM0.2–2.5 at the campus by H. nepalensis and F. pumila was significantly higher than that of E. fortunei (p < 0.05). The adsorption of sPM2.5–10 by the three climbers at the overpass was not significantly different, and the adsorption of sPM0.2–2.5 by H. nepalensis was significantly higher than that of the other two climbers (p < 0.05).
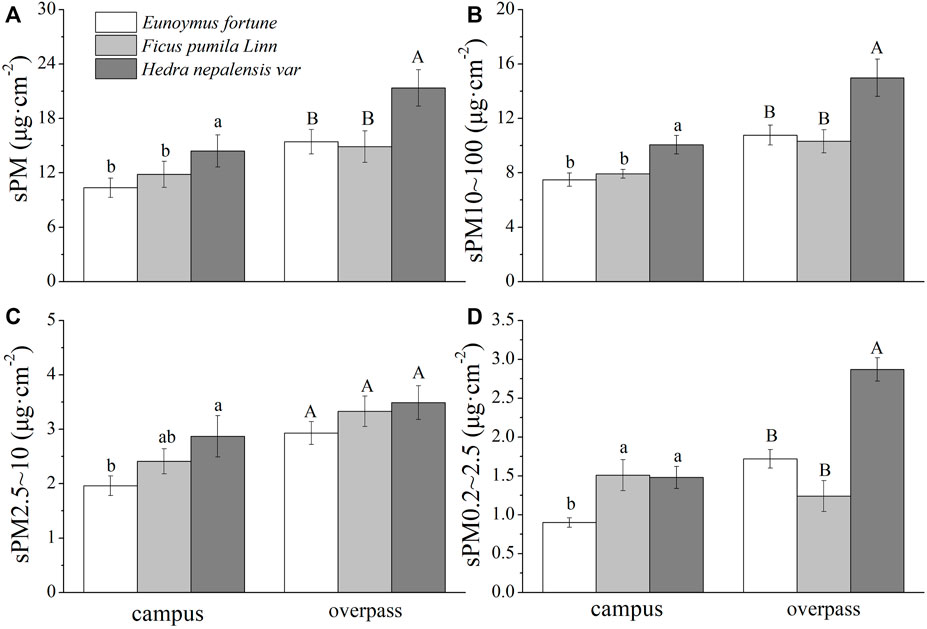
FIGURE 4. The mass of retained particulate matter on the leaf surface in winter (mean ± SE). (A) The total mass of particulate matter. (B) The mass of PM10–100. (C) The mass of PM2.5–10. (D) The mass of PM0.2–2.5.
3.2 The mass of PM retained on the leaf wax layer in different seasons
The adsorption of PM, PM10–100, and PM2.5–10 was highest in the leaf wax layer of H. nepalensis in the spring at the overpass, with values of 13.04 ± 2.28 μg cm−2 (Figure 5A), 7.05 ± 0.69 μg cm−2 (Figure 5B), and 5.37 ± 0.35 μg cm−2 (Figure 5C), respectively, and the adsorption of PM0.2–2.5 was highest in the leaf wax layer of E. fortunei (1.68 ± 0.3 μg cm−2) (Figure 5D). The adsorption of PM and PM2.5–10 by the waxy layer of H. nepalensis leaves in both the campus and overpass was significantly higher than that of the other two climbers (p < 0.05), and the adsorption of PM10–100 by the waxy layer of H. nepalensis and E. fortunei leaves was significantly higher than that of F. pumila (p < 0.05). The adsorption of PM0.2–2.5 by the waxy layer of H. nepalensis leaves at the campus was significantly higher than that of the other two climbers (p < 0.05). The adsorption of PM0.2–2.5 by the waxy layer of the leaves of E. fortunei at the overpass was significantly higher than that of the other two climbers (p < 0.05).
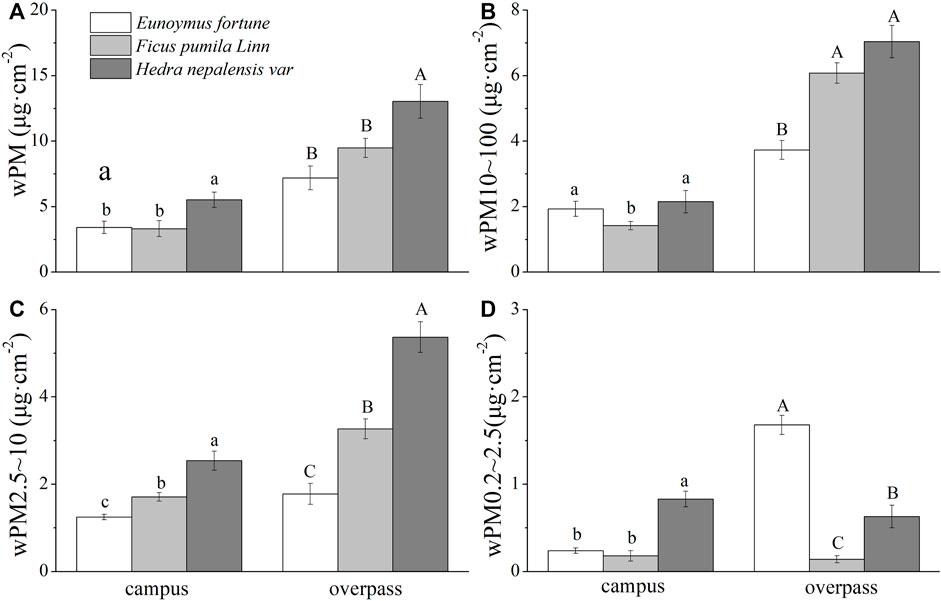
FIGURE 5. The mass of retained particulate matter on the leaf wax layer in spring (mean ± SE). (A) The total mass of particulate matter. (B) The mass of PM10–100. (C) The mass of PM2.5–10. (D) The mass of PM0.2–2.5.*Bars with different letters indicate signigicant differenc (p < 0.05) between the sites at a specific season. The same below.
The adsorption of PM by the waxy layer of H. nepalensis leaves at both the campus and the overpass reached a maximum in summer. The adsorption of PM of different particle sizes by the H. nepalensis leaves at the overpass was: wPM (10.83 ± 0.52 μg cm−2) (Figure 6A), wPM10-100 (7.93 ± 0.36 μg cm−2) (Figure 6B), and wPM0.2–2.5 (1.03 ± 0.15 μg cm−2) (Figure 6D). Overall, the adsorption of PM, PM10–100 (Figure 5C), and PM0.2–2.5 by the waxy layer of campus H. nepalensis leaves was significantly higher than that of the other two climbers (p < 0.05), and the adsorption of PM, PM2.5–10, and PM0.2–2.5 by the waxy layer of H. nepalensis leaves at the overpass was significantly higher than that of the other two climbers (p < 0.05).
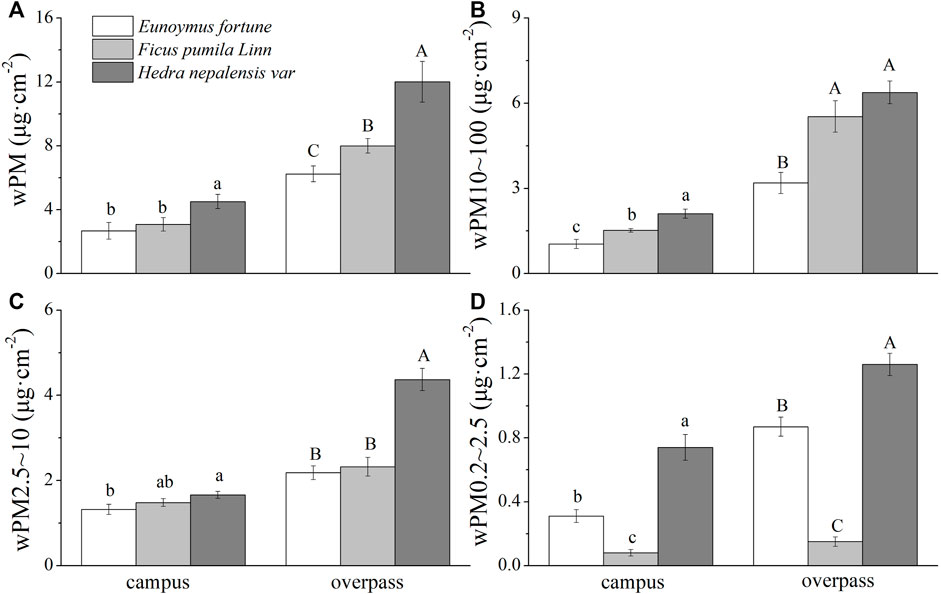
FIGURE 6. The mass of retained particulate matter on the leaf wax layer in summer (mean ± SE). (A) The total mass of particulate matter. (B) The mass of PM10–100. (C) The mass of PM2.5–10. (D) The mass of PM0.2–2.5.
The adsorption of PM by the waxy layer of H. nepalensis leaves at the overpass reached a maximum in autumn for PM (10.83 ± 0.52 μg cm−2) (Figure 7A), PM10–100 (7.93 ± 0.36 μg cm−2) (Figure 7B), PM2.5–10 (1.87 ± 0.21 μg cm−2) (Figure 7C), and PM0.2–2.5 (1.03 ± 0.15 μg cm−2) (Figure 7D). The adsorption pattern of the leaf wax layer of the three climbing plants at the campus was consistent for PM, PM10–100, and PM0.2–2.5, all of which were significantly higher for H. nepalensis than for the other two plants (p < 0.05). The adsorption pattern of the three plants at the overpass was consistent for PM and PM10–100, i.e., H. nepalensis was significantly higher than the other two plants (p < 0.05). The adsorption pattern of the three climbing plants was also consistent for PM2.5–10, while there was no significant difference in the adsorption of PM0.2–2.5 among the three climbers (p < 0.05).
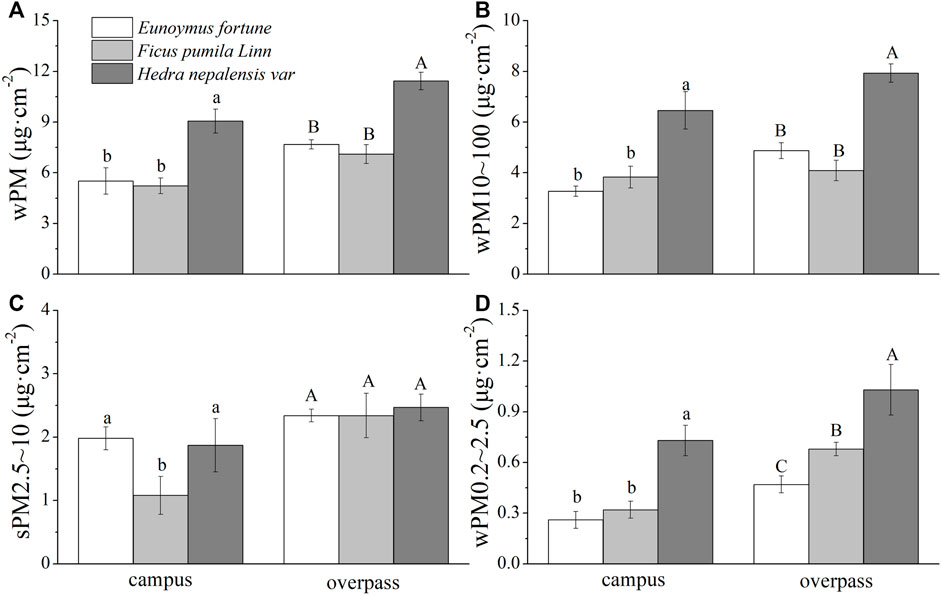
FIGURE 7. The mass of retained particulate matter on the leaf wax layer in autumn (mean ± SE). (A) The total mass of particulate matter. (B) The mass of PM10–100. (C) The mass of PM2.5–10. (D) The mass of PM0.2–2.5.
The adsorption of different particle sizes of PM by the leaf wax layer of all three plants in the winter at the campus was significantly higher for H. nepalensis than for the other two plants (p < 0.05). The adsorption of different particle sizes of PM by the leaf wax layer of H. nepalensis in the overpass was: wPM (10.83 ± 0.52 μg cm−2) (Figure 8A), wPM10–100 (7.93 ± 0.36 μg cm−2) (Figure 8B), and wPM2.5–10 (7.93 ± 0.36 μg cm−2) (Figure 8C). The adsorption patterns of PM, PM10–100, and PM2.5–10 were consistent among the three plants at the overpass, i.e., H. nepalensis was significantly higher than the other two plants (p < 0.05), and there were significant differences (p < 0.05) in the adsorption of PM0.2–2.5 for all three climbers at the overpass (Figure 8D).
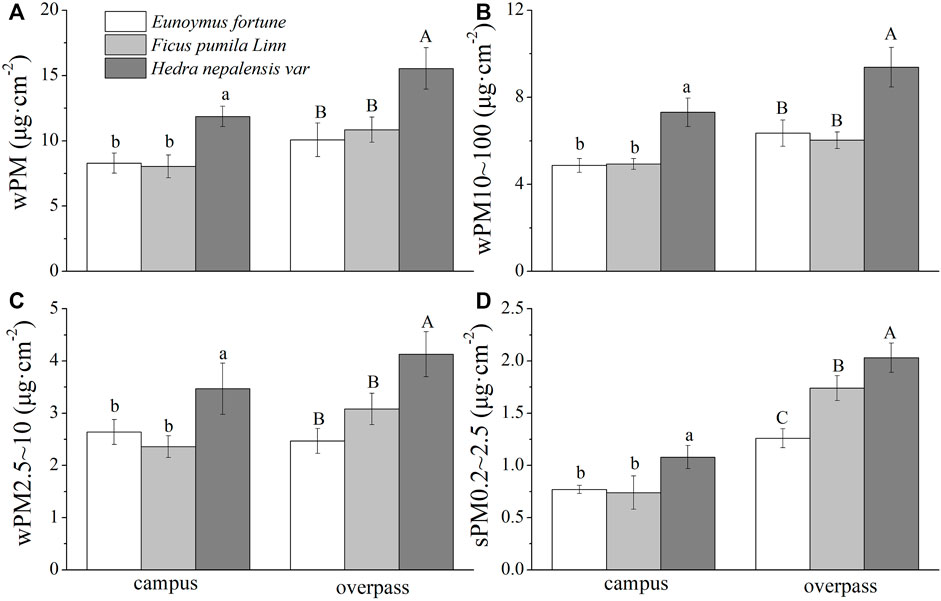
FIGURE 8. The mass of retained particulate matter on the leaf wax layer in winter (mean ± SE). (A) The total mass of particulate matter. (B) The mass of PM10–100. (C) The mass of PM2.5–10. (D) The mass of PM0.2–2.5.
3.3 The photosynthetic gas exchange paremeters response of climbing plants to different levels of PM pollution
The Pn, Ci, and Tr of F. pumila leaves on campus were significantly higher than those of the other two climbers in spring. The Gs of H. nepalensis leaves reached a maximum on campus, which was significantly higher than that of E. fortunei. On the overpass, the Pn of F. pumila leaves was significantly higher than that of E. fortune. The Gs and Tr of F. pumila and H. nepalensis leaves were significantly higher than those of E. fortunei. The Ci of H. nepalensis leaves was significantly higher than that of E. fortune (Table.1).
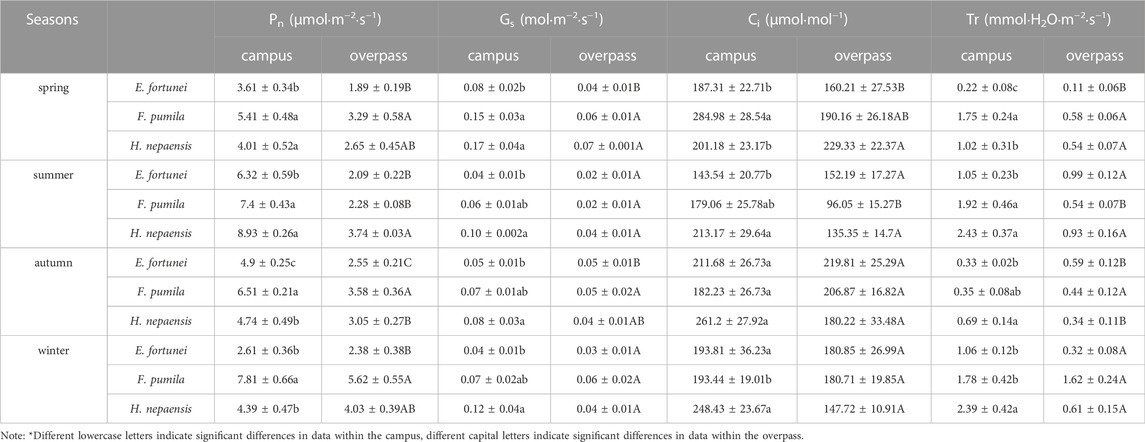
TABLE 1. The photosynthetic gas exchange parameters response of climbing plants to different levels of PM pollution.
As can be seen from Table 1, the Pn, Ci, and TR of E. fortunei leaves at the campus were significantly higher than for the other two climbers in summer. Additionally, the Pn, TR, G, and Ci of E. fortunei leaves all reached a maximum in the summer. The Pn, Gs, and TR of the H. nepalensis and F. pumila leaves at the interchange were significantly higher than those of E. fortunei.
The Pn, Gs, Ci, and TR of H. nepalensis and F. pumila leaves were significantly higher than those of E. fortunei in autumn. The Pn, and Gs of H. nepalensis leaves reached a maximum at the overpass, where the Pn, of H. nepalensis leaves was significantly higher than those of the other two plants. The Ci and TR of H. nepalensis and E. fortunei leaves were significantly higher than those of F. pumila at the overpass, and the Gs of the three plants was not significantly different.
The Pn of F. pumila leaves was significantly higher than that of E. fortunei and H. nepalensis leaves in winter at the campus. The Ci of both E. fortunei and H. nepalensis leaves was significantly higher than that of F. pumila. The Gs and transpiration rate of H. nepalensis leaves reached a maximum in winter, while the Gs of H. nepalensis and F. pumila leaves was significantly higher than that of E. fortunei, and the TR of H. nepalensis leaves was significantly different. The TR of H. nepalensis leaves was significantly higher than that of the other two plants.
3.4 The physiological and biochemical responses of climbing plants to different levels of PM pollution
3.4.1 Seasonal changes of total SOD activity of plants under different levels of PM pollution
As can be seen from Figure 9, the SOD activity of H. nepalensis leaves was significantly higher than that of the other two plants in summer (Figure 9B) and autumn (Figure 9C). The SOD activity of F. pumila leaves was significantly higher than that of the other two plants in winter at the campus (Figure 9D). At the overpass, the SOD activity of H. nepalensis leaves in spring (Figure 9A)and autumn was significantly higher than that of the other two plants, and the SOD activity of H. nepalensis and F. pumila leaves in summer was significantly higher than that of E. fortunei leaves (p < 0.05) (Figure 9B). The SOD activity of F. pumila leaves in winter at the overpass reached a maximum and was significantly higher than that of the other two plants.
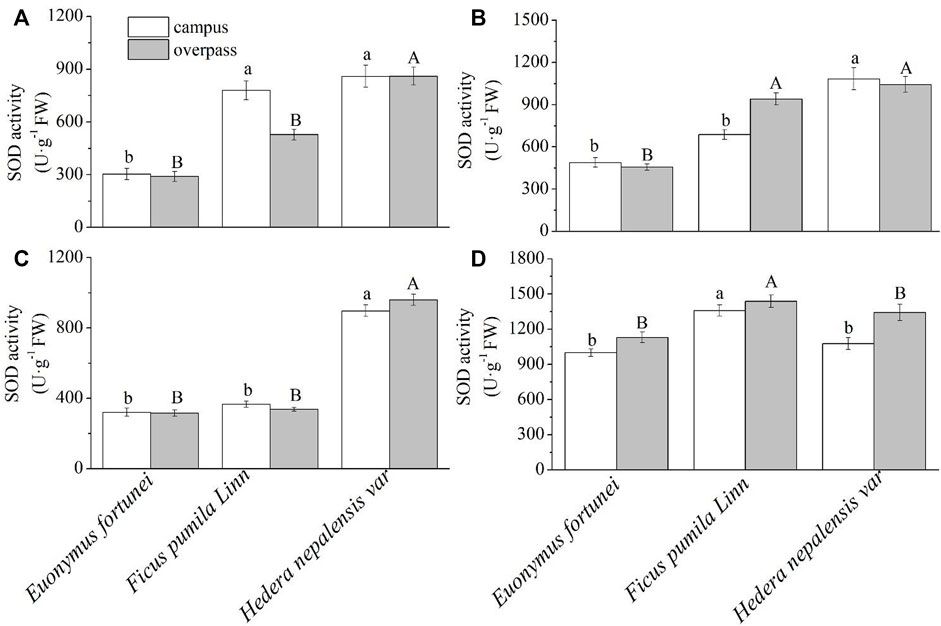
FIGURE 9. Effects of different levels of particulate pollution on SOD activities in plant leaves (mean ± SE). (A) spring, (B) summer, (C) autumn, (D) winter.
3.4.2 Seasonal changes of plant POD activity under different levels of PM pollution
As can be seen from Figure 10, the POD activity of E. fortunei leaves at the campus reached a maximum in spring (Figure 10A), summer (Figure 10B), and winter (Figure 10D). The POD activity of E. fortunei leaves at the campus was significantly higher than that of the other two plants in spring and winter. The POD activity of E. fortune and F. pumila leaves was significantly higher than that of H. nepalensis leaves in summer, and there was no significant difference in the POD activity of the leaves of the three plants in autumn (Figure 10C). The leaf POD activity of E. fortunei was significantly higher than that of the other two plants, and the leaf POD activity of all three plants at the overpass was higher than that at the campus in all four seasons.
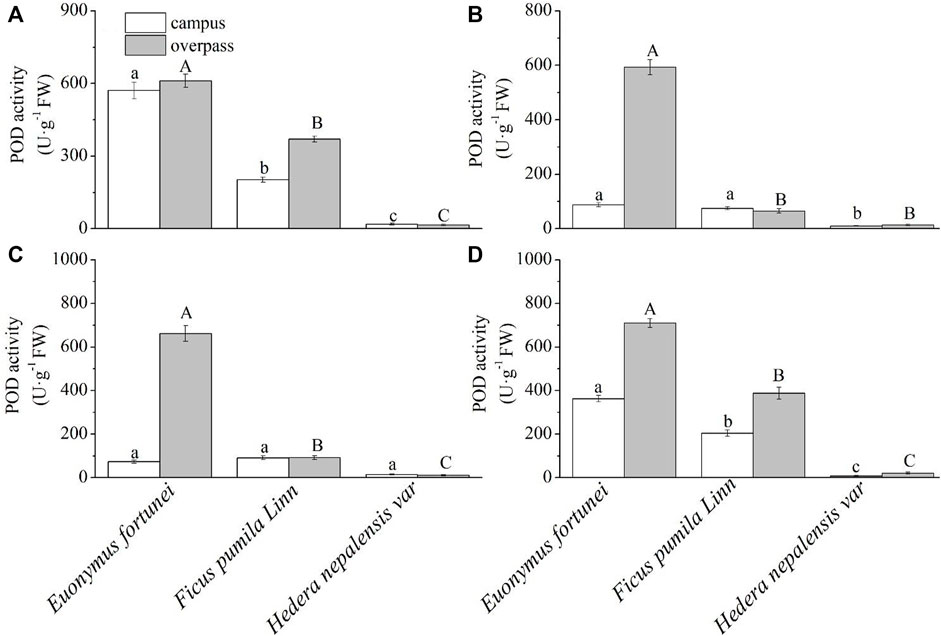
FIGURE 10. Effects of different levels of particulate pollution on POD activities in plant leaves (mean ± SE). (A) spring, (B) summer, (C) autumn, (D) winter.
3.4.3 Seasonal changes of the MDA content of plants under different levels of PM pollution
As can be seen from the Figure 11, the MDA content of F. pumila leaves reached a maximum at the campus, where the MDA content of F. pumila and H. nepalensis leaves was significantly higher than that of E. fortune leaves in spring (Figure 11A) and summer (Figure 11B). The MDA content of F. pumila leaves was significantly higher than that of E. fortunei and H. nepalensis leaves in autumn (Figure 11C) and winter (Figure 11D). At the overpass, the leaf MDA content of H. nepalensis and F. pumila was significantly higher than that of E. fortunei in spring; the leaf MDA content of E. fortunei and F. pumila was significantly higher than that of H. nepalensis in summer; the leaf MDA content of E. fortunei was significantly higher than that of the other two plants in autumn; and the leaf MDA content of F. pumila was significantly higher than that of E. fortunei and H. nepalensis in winter.
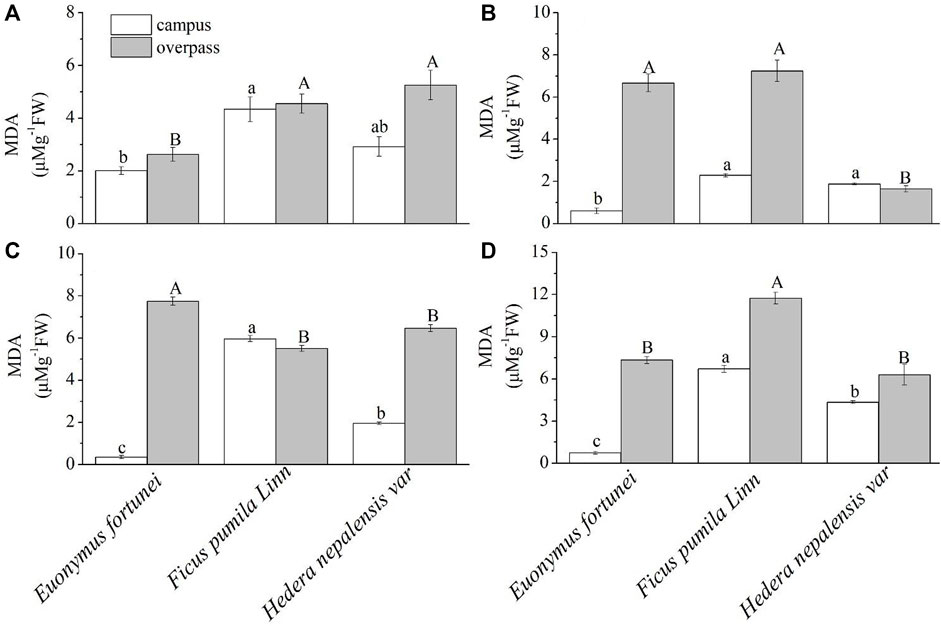
FIGURE 11. Effects of different levels of particulate pollution on MDA content in plant leaves (mean ± SE). (A) spring, (B) summer, (C) autumn, (D) winter.
3.4.4 Seasonal changes of the soluble protein content under different levels of PM pollution
As can be seen from the Table 2, the soluble protein content of H. nepalensis and F. pumila leaves was significantly higher than that of E. fortunei leaves in spring and summer at the campus, and there was no significant difference in the soluble protein content of the leaves of all three plants in autumn. The soluble protein content of F. pumila leaves was significantly higher than that of H. nepalensis and E. fortunei leaves in winter. At the overpass, the soluble protein content of H. nepalensis leaves was largest in spring and autumn, with the soluble protein content of H. nepalensis leaves in spring being significantly higher than that of the other two plants. In summer, the plant soluble protein of F. pumila leaves was significantly higher than that of E. fortune leaves. In autumn, the soluble protein of H. nepalensis leaves was significantly higher than that of E. fortune leaves. In winter, the plant soluble protein of F. pumila leaves was significantly higher than that of the other climbers.

TABLE 2. Effects of different levels of particulate pollution on soluble protein content in plant leaves (g·100 g−1 FW).
3.4.5 Seasonal changes of plant soluble sugar content under different levels of PM pollution
As can be seen from the Table 3, the soluble sugar content of E. fortunei leaves at the campus reached a maximum in spring, summer, and autumn, and the soluble sugar content of E. fortunei leaves and H. nepalensis leaves was significantly higher than that of F. pumila leaves in spring. The soluble sugar content of E. fortunei leaves was significantly higher than that of the other two plants in summer and autumn. The soluble sugar content of H. nepalensis and E. fortunei leaves at the campus was significantly higher than that of F. pumila leaves in winter. At the overpass, the soluble sugar content of E. fortunei leaves was significantly higher than that of F. pumila leaves in spring. At the overpass, the soluble content of H. nepalensis leaves was significantly higher than the other two plants in spring, the soluble sugar content of E. fortunei and F. pumila leaves was significantly higher than that of H. nepalensis leaves in summer, the soluble sugar content of E. fortunei leaves was significantly higher than that of the other two plants in autumn (p < 0.05), and the soluble sugar content of H. nepalensis leaves was significantly higher than that of the other two plants in winter.

TABLE 3. Effects of different levels of particulate pollution on total soluble sugar content in plant leaves (g·100 g−1FW).
3.5 Two-factor ANOVA
As shown in Table 4, the interaction of tree species and sampling site had highly significant effects (p < 0.001) on the sPM, sPM10–100, sPM2.5–10, wPM, wPM10–100, POD activity, MDA content, Pn, and Gs of climbing plants. The effect of tree species on the wPM2.5–10 and wPM0.2–2.5 of all climbing plants was highly significant (p < 0.01). Sampling site had a highly significant effect on the sPM0.2–2.5 and Tr of climbing plants (p < 0.01). Tree species had a highly significant effect on the soluble protein content, Ci, and Tr of climbing plants (p < 0.001). The interaction of tree species and sampling site had highly significant effects on the POD activity (p < 0.001), soluble sugar content (p < 0.01), and sPM10–100, wPM2.5–10, wPM0.2–2.5, MDA, Gs, and Tr (p < 0.05).
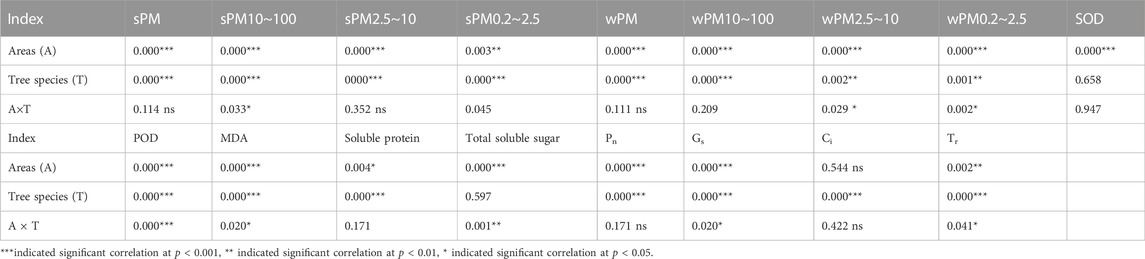
TABLE 4. Effect of tree species and sampling sites on the adsorption particulate matter, photosynthesis and biochemistry by leaves of climbing plants.
3.6 Relationship between particulate matter, photosynthesis and biochemistry by leaves of climbing plants
AS shown in Figure 12, the SOD activity was positively correlated with MDA content, cell concentration (p < 0.01), Gs, sPM, sPM10–100, wPM, wPM10–100 (p < 0.05), and negatively correlated with the POD activity and soluble sugar content (p < 0.05). The POD activity was positively correlated with the MDA content (p < 0.05), and negatively correlated with the soluble protein content, Pn, Gs (p < 0.01), and TR and wPM2.5–10 (p < 0.05). The MDA content was positively correlated with the soluble protein content, sPM, and sPM0.2–2.5 (p < 0.01), and sPM10–100 and sPM2.5–10 (p < 0.05), and negatively correlated with the total soluble sugar content (p < 0.01), and Gs and Ci (p < 0.05). The soluble protein content was positively correlated with the TR (p < 0.01) and Pn (p < 0.05), and negatively correlated with the Ci (p < 0.01). The soluble sugar content was negatively correlated with the Pn (p < 0.01). The Pn was positively correlated with Gs and the Tr (p < 0.01), and negatively correlated with sPM, sPM10–100, sPM2.5–10, wPM, and wPM10–100 (p < 0.01), and sPM0.2–2.5 (p < 0.05). Gs was positively correlated with Ci and the Tr (p < 0.01), and negatively correlated with sPM, sPM10–100, and wPM10–100 (p < 0.05). The sPM was positively correlated with sPM10–100, sPM2.5–10, sPM0.2–2.5, wPM, wPM10–100, wPM2.5–10, and wPM0.2–2.5 (p < 0.01). The sPM10–100 was positively correlated with sPM2.5–10, 1 sPM0.2–2.5, wPM, wPM10–100, wPM2.5–10, and wPM0.2–2.5 (p < 0.01). The sPM2.5–10 was positively correlated with sPM0.2–2.5, wPM, wPM10–100, wPM2.5–10, and wPM0.2–2.5 (p < 0.01). The sPM0.2–2.5 was positively correlated with wPM, wPM10–100, wPM2.5–10, and wPM0.2–2.5 (p < 0.01). The wPM was positively correlated with wPM10–100, wPM2.5–10, and wPM0.2–2.5 (p < 0.01). The wPM10–100 was positively correlated with wPM2.5–10 and wPM0.2–2.5 (p < 0.01). The wPM2.5–10 value was positively correlated with wPM0.2–2.5 (p < 0.01).
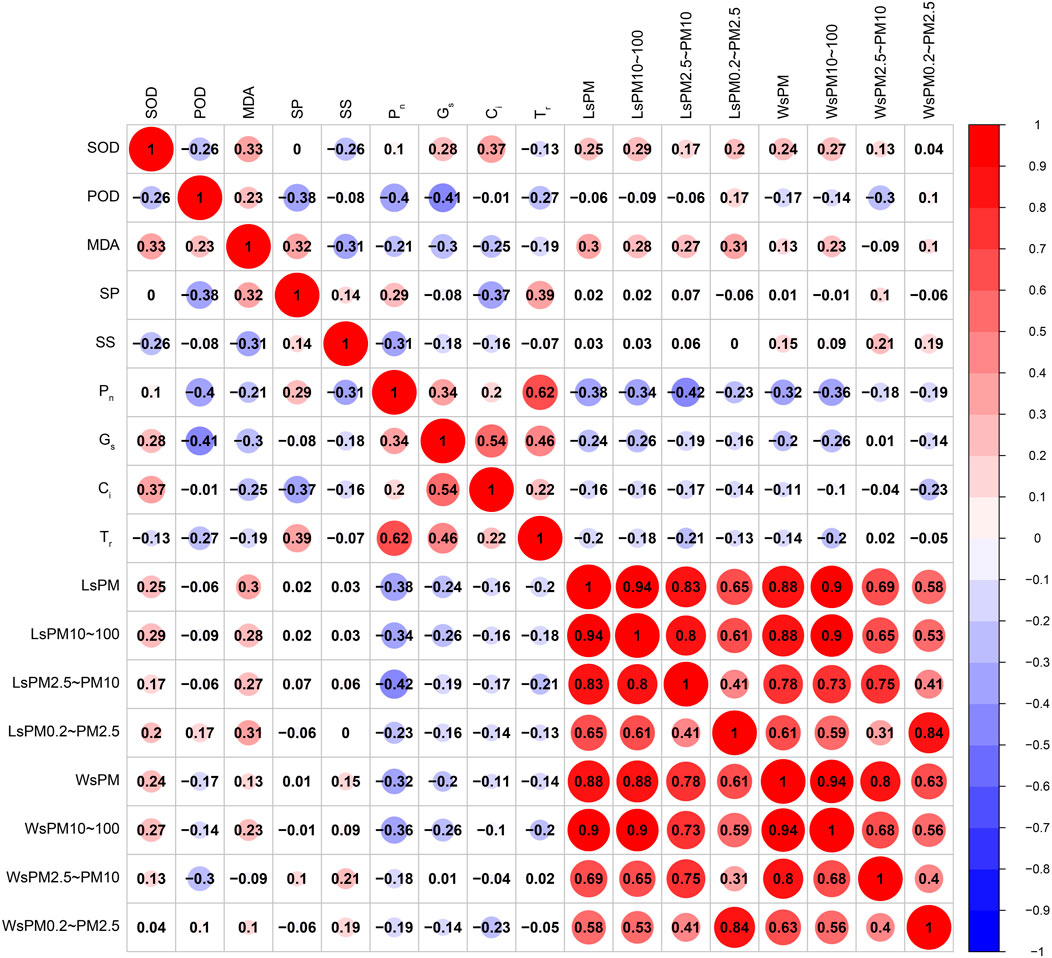
FIGURE 12. Correlations between accumulated particulate matter, photosynthesis and antioxidant enzyme activities by leaves of climbing plants.
4 Discussion
4.1 Particle adsorption by the leaves of different climbing plants
The adsorption of PM by the three evergreen climbers followed the order of H. nepalensis > F. pumila > E. fortunei, indicating that H. nepalensis has a strong adsorption capacity for PM, which is related to its larger individual leaf area, sparsity of scales on the lower surface, and obvious lateral and reticulate veins on the leaf surface. E. fortunei has thin leathery leaves with fine lateral veins and inconspicuous veinlets, and displayed the fewest seasonal differences among the three climbers, indicating a consistent ability to adsorb PM. F. pumila leaves have a glabrous surface with dorsal short hairs and reticulate veins that protrude in the form of a honeycomb, which is conducive to the adsorption of PM. The roughness of the leaf surfaces of the different plants varied, and these differences had a great influence on the adsorption of PM (Wang et al., 2006; Li M et al., 2021). The ability of plants to adsorb PM is closely related to the micromorphology of the leaf surface, and dense, narrow, and deep grooves on the leaf surface could favor the adsorption of PM by plants (Li et al., 2019; Zha et al., 2019). In this study, the adsorption of PM by all three climbing plants was higher at the overpass than at the campus, which was because the overpass was located in the middle of a road segregation zone that was affected by vehicle exhaust. Atmospheric PM pollution was more serious than in other areas, which was consistent with the findings of Beckett et al., 2000 and Freer-Smith et al., 2005. The amount of PM adsorbed by leaf surfaces and wax for all three climbing plants followed the order of winter > spring > summer > autumn, which may be due to the higher level of atmospheric PM pollution in winter than in other seasons, resulting in plants adsorbing more PM. The occurrence of the maximum dust retention in winter was also reported by other researchers (Prajapati and Tripathi, 2008; Zha et al., 2019).
4.2 The effects of PM pollution on the photosynthetic gas exchange parameters of plants
It was found that PM deposited on the leaf surface increased the leaf temperature and interfered with photosynthetic CO2 exchange and transpiration rates by shading and impeding diffusion (Gimeno and Deltoro, 2000). Zia-Khan et al. (2015) found that there may be stomatal occlusion on the leaves of plants growing near coal fields, which may result in a decrease in Gs. In this study, it was found that the Pn of the three climbing plants was more strongly affected under the high PM pollution conditions at the overpass (Table 1). Compared to the overpass, at the campus, the Pn and Gs of the three climbing plants decreased and the Ci concentration increased, indicating that high levels of PM pollution inhibited photosynthesis through its effect on Gs (Guidi et al., 2011; Gajić et al., 2013). The toxic elements from particulate matter can inhibit potosynthetic gas exchange parameters of climbing plants, which consistent with Guidi et al. (2011). The Pearson correlation coefficients between the plant adsorption of different particle sizes and photosynthetic parameters showed that different particle sizes were highly significantly negatively correlated with the net Pn and Gs of plant leaves, i.e., (Table 1). A similar finding was also reported that photosynthesis nad stomatal conductance declined over time at elevated PM2.5, with large variations with PM2.5 concentrations (Yu et al., 2018). The transpiration of stomata was found to make the leaf surface wetter and enhance the deposition of PM. Among the plants investigated in this study H. nepalensis was found to have the highest transpiration rate, which is one of the reasons why it had the greatest ability to adsorb PM (Hinds, 1999). Tree species that display resistance to air pollution are reported to have the highest respiration rates (Lorencplucinska, 1980), and an analysis of the three plants in this study showed that F. pumila had the highest Pn., indicating a strong resistance to particulate pollution.
4.3 The effects of PM pollution on plant biochemical indicators
The degree of oxidative stress in plants depends on the ability of the plant antioxidant capacity system to inhibit superoxide, hydroxyl, and peroxyl radicals at the cellular level (Mroczek-Zdyrska and Wojcik, 2012; Bisoi et al., 2017). Antioxidant enzymes, such as SOD and POD, are physiological and biochemical indicators that are critical for plant stress resistance and the mitigation of oxidative stress (Radwan and Eldeen, 2012).
The three plant species in this study showed a general trend of higher SOD (Figure 10) and POD levels (Figure 11) in all seasons at the overpass than at the campus, indicating an increase in oxidative stress generated by PM pollution on plants. The increase in SOD and POD enzyme activity indicated an increase in free radicals in plant cells caused by PM pollution, with both SOD and POD scavenging these free radicals to reduce the pollution damage to plants (Verma and Dubey 2003; Xu et al., 2010; Sharma et al., 2012; Panda et al., 2018). In addition, in all three plants there was a general trend for a higher MDA content in all seasons at the overpass than at the campus (Figure 12), which was due to the higher level of cell membrane damage in the overpass plants following their exposure to higher pollution levels than the plants at the campus. The higher leaf SOD activity of the three plant species in the winter at both the overpass and campus in this study may be related to high dust levels, high reactive oxygen species production rates, high atmospheric particulate matter pollutant concentrations, and low available light levels during the winter (Singh et al., 2020).
The soluble protein content of the three climbing plants displayed different trends in each season (Table. 2), and the soluble protein content of F. pumila leaves in the summer and winter overpass environments was higher than that of the campus, which may be due to the more extreme weather conditions in summer and winter, when plants actively accumulate soluble proteins to maintain their osmoregulation and sustain higher levels of growth. Kumar and Dubey et al. found that pollutants from automobile exhaust emissions may have an inhibitory effect on protein synthesis (Kumar and Dubey, 1998). The decrease in the foliar protein content in this study may have been due to the breakdown of existing proteins or a decrease in protein synthesis from scratch (Iqba et al., 2000; Singh et al., 1998).
In the three climbing plants investigated in this study there was a general trend for lower soluble sugars in all seasons in the overpass than in the campus, indicating that the three climbing plants were more affected by stress at the overpass (Table. 3). The decrease in the soluble sugar content of damaged leaves may correspond to the photosynthetic inhibition or stimulation of respiration rate (Tzvetkova and Kolarov, 1996). Similarly, Bucker and Ballach et al. found that the level of soluble carbohydrates decreased due to the fumigation of a mixture of O3, SO2, and nitrogen dioxide (NO2) in the leaves, and that the decrease in the soluble sugar content may be the result of increased metabolic depletion under stress (Bücke and Ballach, 2010). Thus, we believe that more particulate matter accumulated in the leaves leads to shade-induced decrease in photosynthetic efficiency, could be responsible for decline in sugar content in the leaves. A similar finding was also reported that heavy metals containing particulate matter can decrease the sugar levels in pistia stratiotes, spirodela polyrrhiza, Eichhornia crassipes, Lagerstomia speciose and Acacia moniliformis (Mishra and Tripathi, 2008; Gupta et al., 2011).
5 Conclusion
In summary, the ability of the three climbing plants to adsorb PM followed the order of H. nepalensis > F. pumila > E. fortunei and the differences in the adsorption characteristics of the different plant species were mainly caused by the different morphological and structural characteristics of the leaf surface. The greater the roughness of the leaf surface and the higher the density of pileus on the leaf, the greater the ability of plant leaves to adsorb PM. There were seasonal differences in PM adsorption by climbing plants, with a trend of winter > autumn > spring > summer. Particulate pollution is an important factor affecting plant photosynthesis, and the effect of PM on the net Pn varies according to particle size. A comprehensive comparative analysis of the three plants showed that F. pumila was the most resistant to PM pollution under the different levels of PM pollution. The three climbing plants actively accumulated biochemical substances in their bodies to regulate osmotic pressure and maintain normal growth when they were exposed to high levels of PM, among which the POD activity and MDA content were the most sensitive indicators of PM stress. Therefore, the identification and assess of the photosynthetic efficacy of these plant species, and their physiological and biochemical responses, may be significant for the selection of climbing tree species for pollution control.
Data availability statement
The original contributions presented in the study are included in the article/Supplymentary Meterial, further inquiries can be directed to the corresponding author.
Author contributions
XL: Conceptualization, writing, and methodology. LC: Data curation. ZL: visualization. JL: writing and investigation.
Funding
This work is supported by the Fundamental Research Funds for the Central Universities” (Grant No. JS2021ZSPY0023) and the Teaching Research Project for Young Teachers of Hefei University of Technology (JYQN2107).
Conflict of interest
The authors declare that the research was conducted in the absence of any commercial or financial relationships that could be construed as a potential conflict of interest.
Publisher’s note
All claims expressed in this article are solely those of the authors and do not necessarily represent those of their affiliated organizations, or those of the publisher, the editors and the reviewers. Any product that may be evaluated in this article, or claim that may be made by its manufacturer, is not guaranteed or endorsed by the publisher.
Supplementary material
The Supplementary Material for this article can be found online at: https://www.frontiersin.org/articles/10.3389/fenvs.2022.1084902/full#supplementary-material
References
Beckett, K. P., Freer-Smith, P., and Taylor, G. (2000). Effective tree species for local air-quality management. J. Arboric. 26 (1), 12–19. doi:10.48044/jauf.2000.002
Bisoi, S. S., Mishra, S. S., Barik, J., and Panda, D. (2017). Effects of different treatmentsof fly ash and mining soil on growth and antioxidant protection of Indian wild rice. Int. J. Phytorem. 19 (5), 446–452. doi:10.1080/15226514.2016.1244164
Bücke, J., and Ballach, H, J. (2010). Alterations in carbohydrate levels in leaves of populus du-e to ambient air pollution. Physiol. Plant 86 (4), 512–517. doi:10.1111/j.1399-3054.1992.tb02163.x
Chaudhary, I. J., and Rathore, D. (2018). Suspended particulate matter deposition and its impact on urban trees. Atmos. Pollut. Res. 9 (6), 1072–1082. doi:10.1016/j.apr.2018.04.006
Chen, L., Liu, C., Zhang, L., Zou, R., and Zhang, Z. (2017). Variation in tree species ability to capture and retain airborne fine particulate matter (PM2.5). Sci. Rep. 7, 1–11. doi:10.1038/s41598-017-03360-1
Chiam, Z., Song, X. P., Lai, H. R., and Tan, H. T. W. (2019). Particulate matter mitigation via plants: understanding complex relationships with leaf traits. Sci. Total Environ. 688, 398–408. doi:10.1016/j.scitotenv.2019.06.263
Choi, Y. K., Song, H. J., Jo, J. W., Bang, S. W., Park, B. H., Kim, H. H., et al. (2021). Morphological and chemical evaluations of leaf surface on particulate matter 2.5 (PM2.5) removal in a botanical plant-based biofilter system. Plants (Basel) 10 (12), 2761. doi:10.3390/plants10122761
De Nicola, F., Maisto, G., Prati, M. V., and Alfani, A. (2008). Leaf accumulation of trace elements and polycyclic aromatic hydrocarbons (PAHs) in Quercus ilex L. Environ. Poll. 153, 376–383. doi:10.1016/j.envpol.2007.08.008
Doğanlar, Z. B., and Atmaca, M. (2011). Influence of airborne pollution on Cd, Zn, Pb, Cu, and Alaccumulation and physiological parameters of plant leaves in Antakya (Turkey). Water Air Soil Pollut. 214 (1), 509–523. doi:10.1007/s11270-010-0442-9
Du, Y., Xu, X., Chu, M., Guo, Y., and Wang, J. (2016). Air particulate matter and cardiovascular disease: the epidemiological, biomedical and clinical evidence. J. Thorac. Dis. 8, E8–E19. doi:10.3978/j.issn.2072-1439.2015.11.37
Dzierżanowski, K., Popek, R., Gawronska, H., Saebø, A., and Gawronska, S. W. (2011). Deposition of particulate matter of different size fractions on leaf surfaces and in waxes of urban forest species. Int J Phytoremediat 13, 1037–1046. doi:10.1080/15226514.2011.552929
Freer-Smith, P. H., Beckrtt, K. P., and Taylor, G. (2005). Deposition velocities to Sorbus aria, Acer campestre, Populus deltoides X trichocarpa ‘Beaupré’, Pinus nigra and X Cupressocyparis leylandii for coarse, fine and ultra-fine particles in the urban environment. Environ. Pollut. 133 (1), 157–167. doi:10.1016/j.envpol.2004.03.031
Gajic, G., Pavlovic, P., Kostic, O., Jaric, S., ĐurĐevic, L., Pavlovic, D., et al. (2013). Ecophysiological and biochemical traits of three herbaceous plants growing on the disposed coal combustion fly ash of different weathering stage. Arch. Biol. Sci. 65 (4), 1651–1667. doi:10.2298/abs1304651g
Gimeno, C., and Deltoro, V. I. (2000). Sulphur dioxide effects on cell structure and photosynthetic performance in the liverwort <i>Frullania dilatata</i>. Can. J. Bot. 78 (1), 98–104. doi:10.1139/cjb-78-1-98
Gu, J., Pitz, M., Breitner, S., Birmili, W., Klot, S., Schneider, A., et al. (2012). Selection of key ambient particulate variables for epidemiological studies-applying cluster and heatmap analyses as tools for data reduction. Sci. Total Environ. 435, 541–550. doi:10.1016/j.scitotenv.2012.07.040
Guidi, L., Degl’Innocenti, E., Carmassi, G., Massa, D., and Pardossi, A. (2011). Effects of boron on leaf chlorophyll fluorescence of greenhouse tomato grown with saline water. Environ. Exp. Bot. 73, 57–63. doi:10.1016/j.envexpbot.2010.09.017
Gupta, S., Nayek, S., and Bhattacharya, P. (2011). Effect of air-borne heavy metals on the biochemical signature of tree species in an industrial region, with an emphasis on anticipated performance index. Chem. Ecol. 27, 381–392. doi:10.1080/02757540.2011.561791
Hinds, W, C. (1999). Aerosol Technology:Properties, behavior, and measurement of airborne -particles. J. Aerosol Sci. 31 (9), 1121–1122. doi:10.1016/S0021-8502(99)00571-6
Iqba, M., Srivastava, P., and Siddiqi, T. (2000). Anthropogenic stresses in the environment and their consequences. Environ. Hazards, 1–40.
Jaconis, S. Y., Culley, T. M., and Meier, A. M. (2017). Does particulate matter along roadsides interfere with plant reproduction? A comparison of effects of different road types on cichorium intybus pollen deposition and germination. Environ. Pollut. 222, 261–266. doi:10.1016/j.envpol.2016.12.047
Kończak, B., Cempa, M., Pierzchala, Ł., and Deska, M. (2021). Assessment of the ability of roadside vegetation to remove particulate matter from the urban air. Environ. Pollut. 268, 115465. doi:10.1016/j.envpol.2020.115465
Kumar, G., and Dubey, P. (1998). Differential response and detoxifying mechanisms of Cassia si-amea Lam and Dalbergia sissoo Roxb of different ages to SO2 treatment. J. Environ. Biol. 19 (3), 243–249.
Li, Y. M., Wang, S. J., and Chen, Q. B. (2019). Potential of thirteen urban greening plants to capture particulate matter on leaf surfaces across three levels of ambient atmospheric pollution. Int. J. Environ. Res. Public Health. 16, 402. doi:10.3390/ijerph16030402
Li, M., Huang, D. M., Zhou, Y. H., Zhang, J., Lin, X. T., and Chen, J. (2021). The legacy effects of PM2.5 depositon on Nerium Oleander L. Chemosphere 281, 130682. doi:10.1016/j.chemosphere.2021.130682
Li, X. L., Zhang, T. R., Sun, F. B., Song, X. M., Zhang, Y. K., Huang, F., et al. (2021). The relation-ship between particulate matter retention capacity and leaf surface micromorphology of ten tree species in Hangzhou, China. Sci. Total Environ. 771, 144–812. doi:10.1016/J.SCITOTENV.2020.144812
Liu, L., Guan, D. S., and Peart, M. R. (2012). The morphological structure of leaves and the dust retaining capability of afforested plants in urban Guangzhou, South China. Environ. Sci. Pollut. R. 19 (8), 3440–3449. doi:10.1007/s11356-012-0876-2
Lorencplucinska, G. (1980). Influence of hydrogen fluoride on the rate of CO2 exchange in Scots pines of different susceptibility to this gas. Arbor: Kórnickie, 269–276.
Michael, S., Montag, M., and Dott, W. (2013). Pro-inflammatory effects and oxidative stress in lung macrophages and epithelial cells induced by ambient particulate matter. Environ. Poll. 183, 19–29. doi:10.1016/j.envpol.2013.01.026
Mishra, V. K., and Tripathi, B. D. (2008). Concurrent removal and accumulation of heavy metals by the three aquatic macrophytes. Bioresour. Technol. 99, 7091–7097. doi:10.1016/j.biortech.2008.01.002
Mroczek-Zdyrska, M., and Wójcik, M. (2012). The influence of selenium on root growth and oxida-tive stress induced by lead in vicia faba l.minor plants. Biol. Trace Elem. Res. 147 (1), 320–328. doi:10.1007/s12011-011-9292-6
Naomi, J., Krix, D., Peter, J., and Torpy, F. R. (2020). Airborne particulate matter accumulation on common green wall plants. Int. J. Phytoremediation 22 (6), 594–606. doi:10.1080/15226514.2019.1696744
Nisha, S., Joosung, O., Sangwon, P., and Hyunook, K. (2021). Impact of particulate matter on primary leaves of Vigna radiata (L.) R. Wilczek. Ecotoxicol. Environ. Saf. 212, 111965. doi:10.1016/j.ecoenv.2021.111965
Panda, D., Panda, D., Padhan, B., and Biswas, M. (2018). Growth and physiological response of lemongrass (Cymbopogon citratus (D.C.) Stapf.) under different levels of fly ash-amended soil. Int. J. Phytoremediat 20 (6), 538–544. doi:10.1080/15226514.2017.1393394
Popek, R., Przybysz, A., Gawrońska, H., Klamkowski, K., and Gawroński, S. W. (2018). Impact of particulate matter accumulation on the photosynthetic apparatus of roadside woody plants growing in the urban conditions. Ecotoxicol. Environ. Saf. 163, 56–62. doi:10.1016/j.ecoenv.2018.07.051
Prajapati, S, K., and Tripathi, B, D. (2008). Seasonal variation of leaf dust accumulation and pigment content in plant species exposed to urban particulates pollution. J. Environ. Qual. 37 (3), 865–870. doi:10.2134/jeq2006.0511
Przybysz, A., Sæbø, A., Hanslin, H. M., and Gawroński, S. W. (2014). Accumulation of particulate matter and trace elements on vegetation as affected by pollution level, rainfall and the passage of time. Sci. Total Environ. 481 (481), 360–369. doi:10.1016/j.scitotenv.2014.02.072
Radwan, M., and Eldeen, D. (2012). Salicylic acid induced alleviation of oxidative stress caused by clethodim in maize (Zea mays L.) leaves. Pestic. Biochem. Phys. 102 (2), 182–188. doi:10.1016/j.pestbp.2012.01.002
Sæbø, A., Popek, R., Nawrot, B., Hanslin, H. M., Gawronska, H., and Gawronski, S. W. (2012). Plant species differences in particulate matter accumulation on leaf surfaces. Sci. Total Environ. 427, 347–354. doi:10.1016/j.scitotenv.2012.03.084
Segalin, B., Kumar, P., Micadei, K., Fornaro, A., and Gonçalves, F. L. T. (2016). Size-segregated particulate matter inside residences of elderly in the metropolitan area of são paulo, brazil. Atmos. Environ. 148, 139–151. doi:10.1016/j.atmosenv.2016.10.004
Sharma, P., Jha, A. B., Dubey, R. S., and Pessarakli, M. (2012). Reactive oxygenspecies, oxidative damage, and antioxidative defense mechanismin plants under stressful conditions. Plant Biotechnol. 32, 243–247. doi:10.1155/2012/217037
Shaughnessy, W. J., Venigalla, M. M., and Trump, D. (2015). Health effects of ambient levels of respirable particulate matter (PM) on healthy, young-adult population. Atmos. Environ. 123, 102–111. doi:10.1016/j.atmosenv.2015.10.039
Singh, P., Agrawal, M., and Agrawal, S. B. (2011). Differences in ozone sensitivity at differ-ent NPK levels of three tropical varieties of mustard (Brassica campestris L.): phot-osynthetic pigments, metabolites, and antioxidants. Water Air Soil Pollut. 214 (1), 435–450. doi:10.1007/s11270-010-0434-9
Singh, S., Tiwari, S., Hopke, P. K., Zhou, C., Turner, J. R., Panicker, A. S., et al. (2018). Ambient black carbon particulate matter in the coal region of Dhanbad, India. Sci. Total Environ. 615, 955–963. doi:10.1016/j.scitotenv.2017.09.307
Singh, S., Pandey, B., Roy, L. B., Shekhar, S., and Singh, R. K. (2020). Tree responses to foliar dust deposition and gradient of air pollution around op-encast coal mines of jharia coalfield, India: gas exchange, antioxidative potential an-d tolerance level. Environ. Sci. Pollut. Res. 28 (7), 8637–8651. doi:10.1007/S11356-020-11088-1
Sun, X., Li, H., Guo, X., Sun, Y., and Li, S. (2018). Capacity of six shrub species to retain atmospheric particulates with different diameters. Environ. Sci. Pollut. Res. Int. 25 (3), 2643–2650. doi:10.1007/s11356-017-0549-2
Tzvetkova, N., and Kolarov, D. (1996). Effect of air pollution on carbohydrate and nutrients co-ncentrations in some deciduous tree species. J. Plant Physiol. 22 (1), 53–63.
Verma, S., and Dubey, R. S. (2003). Lead toxicity induces lipid peroxidation and alters the activities of antioxidant enzymes in growing rice plants. Plant Sci. 164, 645–655. doi:10.1016/s0168-9452(03)00022-0
Wang, L., Liu, L. Y., Gao, S. Y., Hasi, E., and Wang, Z. (2006). Physicochemical characteristics of ambient particles settling upon leaf surfaces of urban plants in Beijing. J. Environ. Sci. (China) 18 (5), 921–926. doi:10.1016/s1001-0742(06)60015-6
WHO World Health Organization (2019). Ambient (outdoor) air pollution. Geneva: World Health Organization.
Xiong, R., Jiang, W., Li, N., Liu, B., He, R., Wang, B., et al. (2021). PM2.5-induced lung injury is attenuated in macrophage-specific NLRP3 deficient mice. Ecotoxicol. Environ. Saf. 221, 112433. doi:10.1016/j.ecoenv.2021.112433
Xu, Q. S., Hu, J. Z., Xie, K. B., Yang, H. Y., Du, K. H., and Shi, G. X. (2010). Accumulation and acute toxicity of silver in Potamogeton crispus (L.). J. Hazard Mater 173, 186–193. doi:10.1016/j.jhazmat.2009.08.067
Yu, W. Q., Wang, Y. J., Wang, Y. Q., Li, B., Liu, Y. J., and Liu, X. (2018). Application of a coupled model of photosynthesis and stomatal conductance for estimating plant physiological response to pollution by fine particulate matter (PM2.5). Environ. Sci. Pollut. Res. 25, 19826–19835. doi:10.1007/s11356-018-2128-6
Zha, Y., Shi, Y., Tang, J., Liu, X., Feng, C., and Zhang, Y. (2019). Spatial-temporal variability and dust-capture capability of 8 plants in urban China. Pol. J. Environ. Stud. 28, 453–462. doi:10.15244/pjoes/81679
Zhang, X., Lyu, J., Zeng, Y., Sun, N., Liu, C., and Yin, S. (2021). Individual effects of trichomes and leaf morphology on PM2.5 dry deposition velocity: a variable-control approach using species from the same family or genus. Environ. Pollut. 272, 116385. doi:10.1016/j.envpol.2020.116385
Zia-Khan, S., Spreer, W., Pengnian, Y., Zhao, X, N., Othmanli, H., He, X., et al. (2015). Effect of dust deposition on stomatal conductance and leaf temperature of cotton in Northwest China. Water-Sui 7, 116–131. doi:10.3390/w7010116
Keywords: climbing plant, particulate matter, physiological, leaf waxy, biochemistry
Citation: Lyu X, Chang L, Lu Z and Li J (2023) The ability of three climbing plant species to capture particulate matter and their physiological responses at different environmental sampling sites. Front. Environ. Sci. 10:1084902. doi: 10.3389/fenvs.2022.1084902
Received: 31 October 2022; Accepted: 09 December 2022;
Published: 06 January 2023.
Edited by:
Tiana Carla Lopes Moreira, University of São Paulo, BrazilReviewed by:
Worradorn Phairuang, Kanazawa University, JapanSanja Potgieter, Manchester Metropolitan University, United Kingdom
Copyright © 2023 Lyu, Chang, Lu and Li. This is an open-access article distributed under the terms of the Creative Commons Attribution License (CC BY). The use, distribution or reproduction in other forums is permitted, provided the original author(s) and the copyright owner(s) are credited and that the original publication in this journal is cited, in accordance with accepted academic practice. No use, distribution or reproduction is permitted which does not comply with these terms.
*Correspondence: Junfeng Li, anVuZTRuaUAxMjYuY29t