- 1Israel Oceanographic and Limnological Research, National Institute of Oceanography, Haifa, Israel
- 2Recanati Institute of Marine Science, Charney School of Marine Sciences, University of Haifa, Haifa, Israel
Marine sediments are ecologically-important environments that act as a long-term depository for different contaminants from natural and anthropogenic sources. We investigated the response of crude-oil and gas-condensate spills on benthic microbial populations in the oligotrophic southeastern Mediterranean Sea using costume-design benthocosms. Additions of crude oil and gas condensate significantly changed the sediment’s chemical properties, with 2-fold elevated levels of total organic carbon (TOC) and up to ∼ 6-fold higher concentration of total polycyclic aromatic hydrocarbons (∑PAHs) relative to unamended sediments. Naphthalene and benzo(a)pyrene were the dominant species comprising the PAHs in both the crude-oil and gas-condensate treatments (29–43% and 26–35%, respectively). Porewater PO43+ drastically declined throughout the experiment, whereas NO2− + NO3− decreased ∼100 days post hydrocarbons addition and then increased in the remaining ∼100 days till the conclusion of the experiment. This temporal variability in NO2− + NO3− hints that hydrocarbon pollution may affect the interplay between benthic denitrification and N2 fixation, thus affecting nutrient limitation for benthic heterotrophic bacteria and phytoplankton. Moreover, our results show that crude oil and gas-condensate usually lead to a decline in benthic autotrophic microbial biomass (50–80%), while heterotrophic bacterial abundances remained unchanged, and bacterial production rapidly increased (maximal 1,600%, crude-oil > gas-cindensate). These effects were prolonged and lasted several months post hydrocarbons addition, highlighting the sediments as a repository for oil contaminants. Amplicon sequencing of the 16S rRNA gene revealed hydrocarbonoclastic bacteria including Methylophaga, Ponticaulis and Alcanivorax genera post crude-oil addition and Actinobacterota 67–14 lineage following gas-condensate amendments. Our results may enable applying a better science-based environmental policy for the benthic marine environment.
Introduction
Coastal marine sediments are considered a hotspot for microbial activity and diversity (Cravo-Laureau and Duran, 2014; Bienhold et al., 2016; Rubin-Blum et al., 2022). Benthic microbes are involved in many biochemical processes and nutrient cycles (Gobet et al., 2012) and play a pivotal role in the ecology of coastal ecosystems (Luna et al., 2002). Marine sediments also act as a depository for contaminants released from natural and anthropogenic sources (Feng et al., 2004), which may affect benthic microbial populations (Cravo-Laureau and Duran, 2014; Frank et al., 2017), as well as other epifaunal and infaunal organisms (Johnston and Roberts, 2009; Rocchetti et al., 2012; Kress et al., 2016).
One of the major threats to the marine and especially benthic environments is crude oil or gas-condensate spills that tend to sink to the bottom and may spread over hundreds of square kilometers (Peterson et al., 2003; Banerjee et al., 2018). Crude oil contains many toxic aliphatic and polycyclic aromatic hydrocarbons (PAHs), polar compounds, heavy metals and volatiles (Laflamme and Hites, 1978; Astrahan et al., 2017). Gas-condensate is a mixture of light hydrocarbons formed by the condensation of hydrocarbon vapors from oil or gas deposits (Gieg et al., 1999; Faramawy et al., 2016) and is considered an acutely toxic petroleum hydrocarbon mixture that behaves differently to the heavier crude-oil in the marine environment (Øksenvåg et al., 2017). Hydrocarbon spills may limit gas exchange across the air-sea interface (Hasse and Liss, 1980), reduce phytoplankton abundance and primary productivity rates (Abbriano et al., 2011; Shai et al., 2021), alter the microbial abundance, diversity and function (Hazen et al., 2010; Brussaard et al., 2016) and kill many zooplankton species (Almeda et al., 2016). These spills often increase total heterotrophic bacterial abundances and activities, while reducing bacterial diversity (Lee and Levy, 1989; Joel et al., 2011).
Whereas the effect of hydrocarbon pollution on pelagic communities has been widely explored, less is known about processes that occur at the seabed following a pollution event. During the Deepwater Horizon spill in the Gulf of Mexico, about 2–15% of the oil was deposited on the seabed by sedimentation of oil-contaminated marine snow (Valentine et al., 2014; Chanton et al., 2015). This sedimentation and flocculent accumulation of marine oil snow, often abbreviated as MOSSFA, deposits oil-derived compounds (Hatcher et al., 2018; Bacosa et al., 2020), alters the biodegradation of oil-derived macromolecules in sediments (Daly et al., 2016; Rahsepar et al., 2022), and harms benthic life (Rohal et al., 2020). Microbes play a crucial role in the formation and degradation of marine oil snow (MOS) (Gregson et al., 2021). The Deepwater Horizon spill impacted pelagic as well as benthic communities, including prokaryotes and eukaryotes (Mason et al., 2014). Whereas in the deep sea, MOS is partially degraded while sinking to the seafloor, in shallow coastal water MOS can reach the benthic environment before significant degradation occurs (Ross et al., 2021). Most importantly, little is known about MOS formation following spills that do not involve crude oil, e.g. gas-condensate leaks.
To date, most studies of MOSSFA and its effects on marine ecology focused on the Gulf of Mexico and Arctic regions (Gregson et al., 2021). Little is known regarding the effects of hydrocarbon pollution on benthic microbial populations in oligotrophic basins, such as the southeastern Mediterranean Sea, an enclosed and oligotrophic basin that may be highly sensitive to environmental perturbations (Shai et al., 2021). The southeastern Mediterranean Sea offshore Israel is highly susceptible to oil-based contaminants that may impact the sediment, especially in shallow littoral-coastal areas, due to the widespread extraction of natural gas deposits and transport of byproducts, such as gas-condensate (Shaffer, 2011). It was heavily polluted by hydrocarbons in the 1970-80s, averaging ∼3.6 Kg of tar per 1 m of the front beach (Golik et al., 1988). Nowadays the coastal seabed along the Israeli coast is considered clean, yet sporadic oil spill events do occur, with the most notorious and recent event dated February-March 2021 (www.sviva.org.il). Moreover, in recent years, a dramatic increase in activities related to seabed hydrocarbon exploitation, offshore platforms and oil transfer, occurs in the region (Shaffer, 2011; Carpenter and Kostianoy, 2018), thus posing additional risk to the sensitive-oligotrophic southeastern Mediterranean Sea. A model-based risk analysis carried out in the European RAOP-MED project suggested the probability of ‘medium\large’ oil spills in the water column in the eastern Mediterranean Sea is high both on spatial and temporal scales. However, this risk analysis does not consider the ramifications of crude-oil and gas-condensate spills in the benthic environment, despite being a long-term depository for pollutants such as PAHs and other non-volatile hydrocarbons (Quero et al., 2015). This study addresses some of these knowledge gaps to support modeling and to help maintain Good Environmental Status (GES) in the southeastern Mediterranean water.
In this study, we examined the effects of crude-oil and gas-condensate contamination in the nearshore water on benthic autotrophic and heterotrophic microbial populations in the shallow coastal sediments of the southeastern Mediterranean Sea. To this end, we used an experimental benthocosm system to mimic crude-oil or gas-condensate spills and examined the response of benthic microbial populations. We hypothesized that crude-oil or gas-condensate amendments will lead to increased heterotrophic microbial activity, especially of hydrocarbon-degrading bacteria, at the expense of autotrophs, such as benthic algae and cyanobacteria. We also hypothesized that the effect of crude oil on benthic microbes will be stronger and more prolonged than the effect of gas-condensate, as the volatile compounds in gas-condensate can rapidly dissipate, without forming substantial MOSSFA, as in the case of crude-oil contamination.
Materials and methods
Experimental setup
Intertidal sediments (mainly quartz sand) were collected from the southeastern Mediterranean coast (Lat. 32° 47′52N; Lon. 34° 57′20E) in the winter of 2019. The sediments were transferred to nine benthocosm tanks (∼140 L each), and the thickness of the sediment layer in each benthocosm tank was ∼20 cm. Ambient seawater was added to the benthocosm tanks so that sediments were submerged (water height was ∼25 cm above the sediments; Figure 1). To maintain ambient sea surface temperatures, the tanks were immersed in an elongated seawater tank (5 m3) at the Israel Oceanographic & Limnological Research (IOLR) Institute (southeastern Mediterranean coast). Porewater was collected using a custom-made extraction apparatus placed in the middle of each tank by a tube connected to a syringe. Following 3 days of acclimation, the nine benthocosms were assigned to three treatments (scattered randomly in the raceway pool): 1) crude-oil, 2) gas-condensate and 3) unamended control. A ∼500 µm thick layer of cru oil or gas condensate was established at the water surface, representative of a moderate-high pollution scenario based on the Israeli Ministry of Environmental Protection criteria (www.sviva.gov.il). The top sediments and porewater were sampled from each experimental benthocosm once every 7–14 days for >6 months (n = 17 samplings).
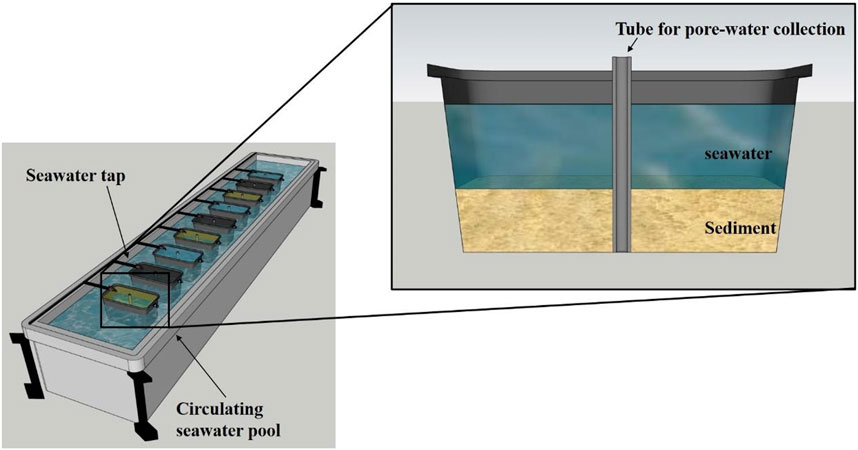
FIGURE 1. Illustration showing the benthocosm experimental design used in this study. Each benthocosm was filled with surface sediment (20 cm) and overlying water (25 cm) collected from the littoral zone of the southeastern Mediterranean Sea in February 2019. Crude oil or gas condensate were added to the overlying water, mimicking a moderate pollution scenario (<500 µm thick layer). Three benthocosms remained unamended (hydrocarbons were not added). The porewater and top sediment were sampled every 7–14 days for 6 months (n = 17 sampling events).
Inorganic nutrients in porewater
Pore-water samples were extracted using an acid-clean syringe and tubing through the collection apparatus (Figure 1), filtered through an 0.45 µm syringe filter, and added to acid-washed 20 ml plastic scintillation vials. The samples were kept at −20°C until analysis. NO2− + NO3− (nitrate + nitrite) and orthophosphate (PO43+) were determined using a segmented flow Seal Analytical AA-3 system. All measurements were well above the limit of detection; 80 μmol kg−1 for NO2− + NO3− and 8 μmol kg−1 for PO43+ (Sisma-Ventura et al., 2021).
Total organic carbon (TOC) and total polycyclic aromatic hydrocarbons (∑PAHs)
TOC was measured by wet oxidation as in Gaudette et al. (1974) with minor modifications following Astrahan et al. (2017). Briefly, samples were oxidized with potassium dichromate and sulfuric acid after which the excess dichromate was potentiometrically titrated with ammonium ferrous sulfate using a Metrohm 785 DMP titrino auto-burette. The level of quantitation (LOQ) is 0.06%.
Total PAHs were analyzed using a GC-MS according to EPA8270 method. The LOQ for each different PAH measured was 5 ng gr−1, while the detection levels varied between 0.46 and 1.4 ng gr−1, depending on the molecule in question.
Sediment surface phytoplankton and bacterial abundance
Samples were collected from the upper 1 cm of the benthocosm sediment (∼3 gr), placed in sterile plastic tubes containing 6 ml of pre-filtered seawater (0.2 µm) and were immediately fixed with a glutaraldehyde solution (1% final concentration, Sigma, G7651). At the lab, the chelating agent sodium pyrophosphate (final concentration 0.01 M) and the detergent Tween 20 (final concentration 0.5%) were added to reduce adhesion of bacterial cells to the sediment grains. The tube was then mixed for 30 min using vortex at 700 rpm before being placed in an ultrasonic water bath (Symphony, VWR) for 1 min to disperse the attached bacterial cells into the liquid phase. The tubes were left overnight at 4°C and subsamples were either stained with 1 μl SYBR green (Applied Biosystems cat #S32717) per 100 µl of sample in the dark (10 min) for heterotrophic bacterial counts or left unstained for picophytoplankton quantification. The samples were analyzed by an Attune® acoustic focusing flow cytometer. Cell abundance was normalized to the sediment’s dry weight.
Sediment surface chlorophyll.a concentration
Samples were collected from the upper 1 cm of the benthocosm sediment (∼10 gr), transferred to glass scintillation vials (20 ml) and stored at −20°C until analysis. Chlorophyll. a was extracted from the sediments using 90% acetone overnight at 4°C. The extracts were filtered through a 0.45 μm Teflon syringe filter and transferred into new glass vials. Chlorophyll. a concentrations were determined from the extracts by the non-acidification method (Welschmeyer, 1994) using a Turner Designs Trilogy® fluorometer at 436 nm excitation filter and 680 nm emission filter. Liquids were decanted from the sediment samples to determine their weight, and the values were used to calculate the amount of chlorophyll.a per gram of sediment.
Bacterial production (BP) in sediment
Samples were collected from the upper 1 cm of the benthocosm sediment (∼3 gr), re-suspended in 3.5 ml of pre-filtered seawater (0.2 µm) and spiked with 500 nmol L−1 (final concentration) of [4,5-3H]-leucine (Perkin Elmer United States, specific activity 160 Ci mmol−1) as previously described for sediments (Frank et al., 2019). The samples were incubated in the dark for 4–8 h under in-situ temperatures. At the end of the incubation, the assimilation of leucine was halted by the addition of 100 µl of 100% trichloroacetic acid solution. The sediment samples were then sonicated (Symphony, VWR) for 10 min to remove the bacterial biomass from the sediments (Frank et al., 2017). The bacterial biomass extract was divided into three 1 ml aliquots and the micro-centrifugation technique was applied (Smith et al., 1992). Disintegration per minute (DPM) from each sample was read using a liquid scintillation counter (Packard Tri carb 2100). A conversion factor of 1.5 kg C mol−1 with an isotope dilution factor of 2.0 was used to calculate the bacterial carbon assimilation rate (Simon and Azam, 1989). Blank samples (n = 3 in each sampling event) included sediments randomly collected from the benthocosms added with the [4,5-3H]-leucine and immediately with trichloroacetic acid, and incubated under the same conditions as described above.
DNA extraction and amplicon sequencing
DNA was extracted from the sediment samples (∼500 mg wet weight) with the PowerSoil DNA bead tubes (Qiagen, United States), using the FastPrep-24™ Classic (MP Biomedicals, United States) bead-beating to disrupt the cells (2 cycles at 5.5 m sec–1, with a 5 min interval). The V4 region (∼ 300 bp) of the 16S rRNA gene was amplified from the DNA (∼50 ng) using the 515Fc/806Rc primers amended with CS1/CS2 tags (5′- ACACTGACGACATGGTTCTACAGTGYCAGCMGCCGCGGTAA, 5′- TACGGTAGCAGAGACTTGGTCTGGACTACNVGGGTWTCTAAT, (Apprill et al., 2015; Parada et al., 2016). PCR conditions were as follows: initial denaturation at 94°C for 45 s, 30 cycles of denaturation (94°C for 15 s), annealing (15 cycles at 50°C and 15 cycles at 60°C for 20 s) and extension (72°C for 30 s). Two annealing temperatures were used to account for the melting temperature of both forward (58.5–65.5°C), and reverse (46.9–54.5°C), primers. Library preparation from the PCR products and sequencing of 2 × 250 bp Illumina MiSeq reads was performed by HyLabs (Israel). The reads were deposited to the NCBI SRA archive under project number PRJNA882580.
Bioinformatics
Demultiplexed paired-end reads were processed in QIIME2 V2022.2 environment (Bolyen et al., 2019). Primers and sequences that did not contain primers were removed with cutadapt (Martin, 2011). Reads were truncated based on quality plots, checked for chimeras, merged and grouped into amplicon sequence variants (ASVs) with DADA2 (Callahan et al., 2016). The amplicons were classified with scikit-learn classifier that was trained on Silva database V138 (16S rRNA, Glöckner et al., 2017). Mitochondrial and chloroplast sequences were removed from the 16S rRNA amplicon dataset. Pathogen sequences were detected with 16SPIP (Miao et al., 2017).
Statistical analyses
Nutrients, cells abundance and activity were compared among the different hydrocarbon treatments using analysis of variance (ANOVA) followed by a Student-Newman-Keuls (SNK) post-hoc test (p < 0.05). Before analyses, the data were log-transformed to ensure the normality distribution of residuals (Shapiro-Wilk test) and checked for homogeneity of variance. The analyses were done by using the Microsoft add-in XLSTAT. We calculated beta diversity indices in R V3.6.3 (R Core Team 2021), using phyloseq (McMurdie and Holmes, 2013). Indispecies package was used to identify microbial lineages associated with treatment (Cáceres and Legendre, 2009).
Results
Porewater’s chemical characteristics in response to crude-oil and gas-condensate amendments
Inorganic nutrient levels from the porewater exhibited large temporal variability in all treatments and controls (Figure 2). In the unamended control benthocosms, NO2− + NO3− ranged from 0.44–4.85 μmol L−1 and PO43+ from 0.65–4.40 μmol L−1, resulting in an average N:P ratio of ∼0.8:1. The NO2− + NO3− levels drastically declined in both the crude-oil and gas-condensate treatments in the first ∼100 days post hydrocarbon addition by up to 85%, and then gradually increased by 2–3 fold relative to the controls in the next ∼100 days (Figures 2A,B). Overall, the temporal alterations in NO2− + NO3− levels were similar between treatments statistically-wise, namely the control ≈ crude-oil ≈ gas-condensate (p> 0.05, ANOVA, Supplementary Table S1). PO43+ was rapidly consumed throughout the experimental duration in both treatments relative to the controls till it was nearly fully depleted (Figures 2C,D). Overall, the PO43+ levels in the control mesocosm > crude-oil ≈ gas-condensate (p< 0.05, ANOVA, Supplementary Table S1). The resulting N:P ratio was ∼2.8:1 in both the crude oil and the gas-condensate treatments. Additional chemical measurements directly related to the hydrocarbon additives in the top sediment included total organic carbon (TOC) and total polycyclic aromatic hydrocarbons (∑PAHs) concentrations that were measured at discrete time points along the experiment (days 0, 4, 74, and 207). The TOC level in the unamended control was ∼0.6% at all sampling points and increased to 1.17–1.32% in the crude oil and 0.76–1.02% in the gas-condensate benthocosms (Table 1). This resulted in ∼2-fold and ∼1.5-fold higher TOC values in the crude-oil and gas-condensate benthocosms, respectively, than the unamended controls. ∑PAHs levels were ∼18 ng gr−1 in the control benthocosms and increased by as much as 6-fold higher concentrations in the hydrocarbon treatments (Table 1; Supplementary Table S2). Naphthalene and benzo(a)pyrene were the dominant species comprising the PAHs at all sampling points in both the crude-oil and gas-condensate treatments (29–43% and 26–35%, respectively) (Supplementary Table S2). Other molecules such as Benzo (g,h,i)perylene (4–12%), Phenanthrene (7–13%), Fluoranthene (0–12%), 2-Methynaphthalene (1–6%), Benz(a)anthracene (0–5%), Benzo(b)fluoranthene (0–3%), Dibenzofuran (0–3%), Chrysene (0–5%), and Indeno (1,2,3-cd)pyrene (0–7%) were also detectable in some time points\treatments but not in all, and were often below the limit of quantification (Supplementary Table S2). Overall, TOC in the control < crude oil ≈ gas condensate, while for ∑PAHs the levels in the control < gas-condensate < crude-oil (p< 0.05, ANOVA; Supplementary Table S2).
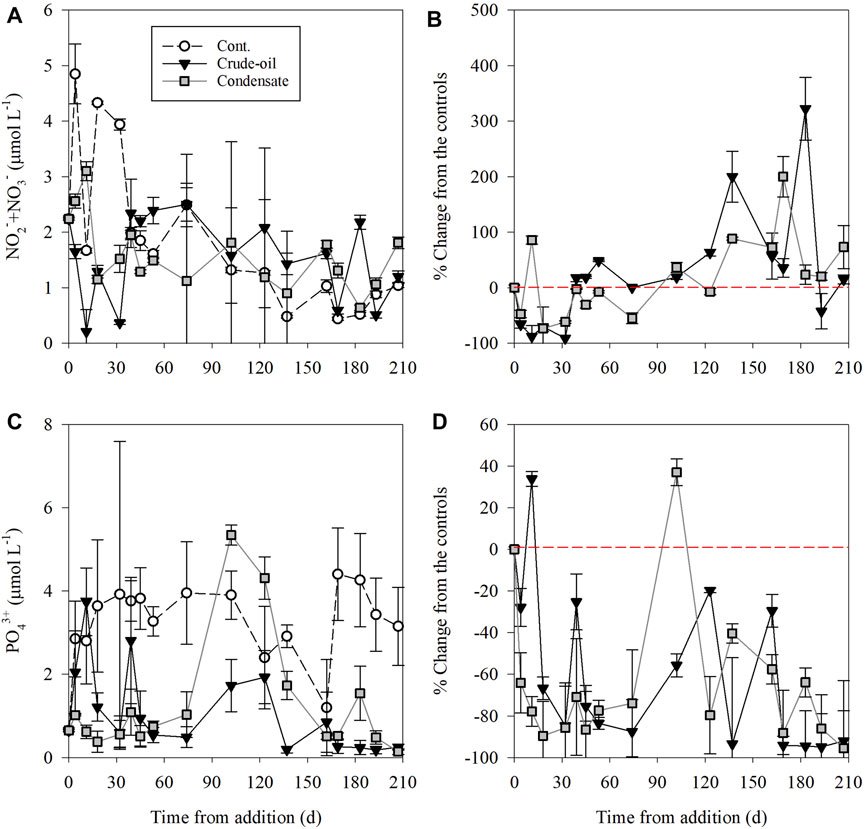
FIGURE 2. The temporal variability of porewater NO2− + NO3− (A, B) and PO43+ (C, D) following crude oil (black triangle) and gas condensate (grey square) additions and in the unamended control benthocosms (white dots). Values shown are the average of three independent ‘biological’ replicates and their standard deviations. The red dashed line indicates no change between the treatments and the unamended controls at each time-point.
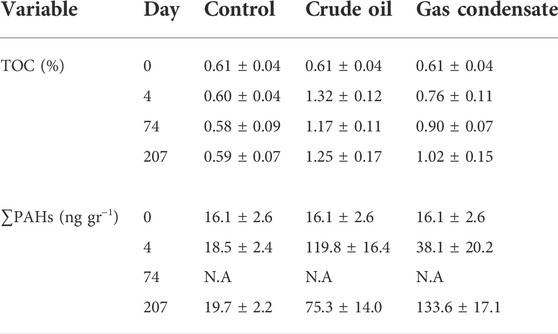
TABLE 1. Values of total organic carbon (TOC) and total polycyclic aromatic hydrocarbons (∑PAHs) in the surface sediments following crude oil or gas condensate additions at discrete time points. N.A-not available.
Changes in bacterioplankton biomass, activity and diversity in response to the addition of crude oil and gas condensate
Heterotrophic bacterial abundances in sediments showed little and insignificant difference between treatments throughout the experiment with scattered temporal variability (Figure 3A; Supplementary Table S1). The bacterial cell number in the crude oil treatment ranged from ∼1 × 105 cells gr−1 to ∼12 × 105 cells gr−1, while in the gas-condensate treatment it ranged from ∼0.4 × 105 cells gr−1 to ∼10 × 105 cells gr−1. Similar abundances were recorded in the control benthocosms, with heterotrophic bacterial abundance ranging from ∼0.3 × 105 to ∼9 × 105 cells gr−1 (Figure 3A). The resulting heterotrophic bacterial abundance in the crude oil or gas condensate treatments relative to the controls showed scattered temporal variability, except from days 137–210 where lower abundances were found (Figure 3B), following the increase in NO2− + NO3− (Figure 2B). Unlike the slight variations detected in heterotrophic bacterial abundances (Figures 3A,B), heterotrophic bacterial production rates (BP) almost immediately (within a few hours) and significantly increased post-crude oil or gas condensate additions relative to the controls (Figures 3C,D, Supplementary Table S1). BP ranged from 0.3–1.7 μg C gr−1 h−1 in the control benthocosms, whereas in the crude oil and gas condensate these ranged from 0.7–6.9 μg C gr−1 h−1 and 0.7–2.9 μg C gr−1 h−1, respectively (Figure 3C). The increase in BP rates relative to the unamended controls was highest in the crude oil (maximal change ∼1,600%) and lower in the gas condensate (maximal change ∼800%) treatments (Figure 3D). Overall, the BP rates in the control < gas condensate < crude oil (p< 0.05, ANOVA; Supplementary Table S1).
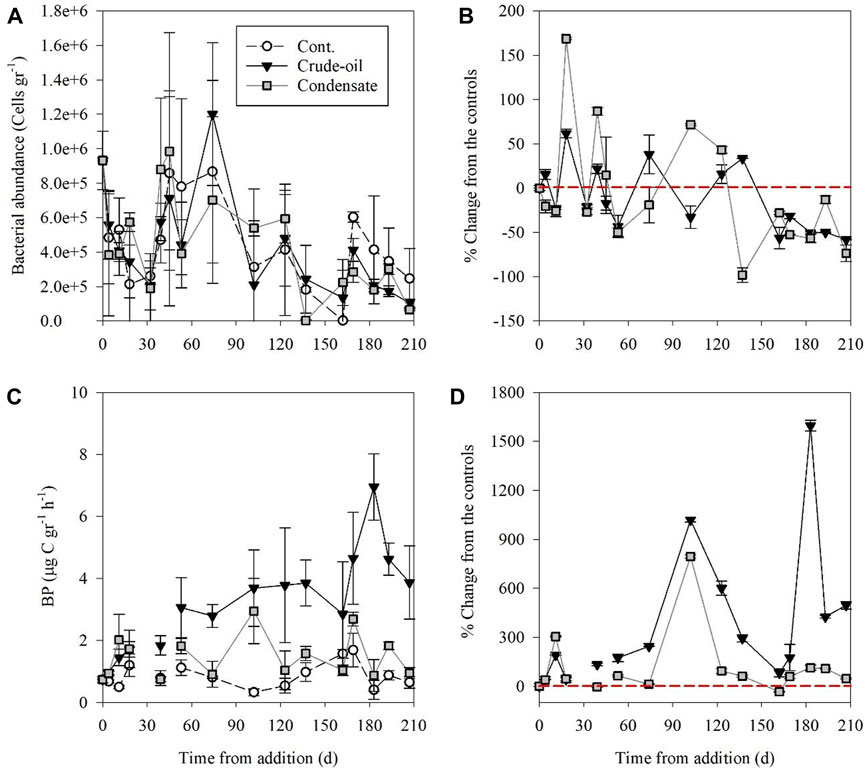
FIGURE 3. The temporal variability of surficial sediment bacterial abundance (A, B) and bacterial production (C, D) rates following crude oil (black triangle) and gas condensate (grey square) additions and in the unamended control benthocosms (white dots). The values shown are the average of three independent “biological” replicates and their standard deviations. The red dashed line indicates no change between the treatments and the unamended controls at each time point.
Benthic phytoplankton biomass derived from chlorophyll. a measurements showed little and insignificant differences between treatments, ranging from 0.002 to 0.02 µg gr−1 (Figure 4A; Supplementary Table S1). Chlorophyll. a level in the gas condensate benthocosms was usually lower than the controls throughout the experiment duration (maximal decrease of ∼50%) (Figure 4B). In contrast, an increase in chlorophyll. a was recorded in the first few days post crude oil addition (∼50%) followed by a gradual decrease until the conclusion of the experiment (maximal decrease of ∼85%) (Figure 4B). Benthic cyanobacterial abundance ranges were in the same order of magnitude between treatments; control (1.4 × 103–18 × 103 cells gr−1), crude oil (1.1 × 103–45 × 103 cells gr−1) and gas condensate (1.2 × 103–22 × 103 cells gr−1) treatments (Figure 4C), yet exhibited different temporal variability (Figure 4D; Supplementary Table S1). Crude oil addition lead to an increase in cyanobacteria during the first 40 days compared to the controls (maximal change ∼85%), followed by a continuous decrease throughout the remaining experimental period (Figure 4B). Gas condensate addition lead to an immediate decrease in cyanobacterial abundance (by ∼20%), which remained below the respective control benthocosms throughout most of the sampling points (Figure 4D). Picoeukaryotic phytoplankton abundance was low in all treatments; 0.003–0.017 cells gr−1 in comparison to the controls (Figure 4E). The gas condensate addition lead to an overall decrease in picoeukaryotes throughout the whole experiment relative to the controls (maximal ∼80%), while crude oil amendments resulted in a rapid abundance decrease in the first 120 days post-addition (maximal ∼75%) followed by a moderate increase (maximal 40%) (Figure 4F; Supplementary Table S1).
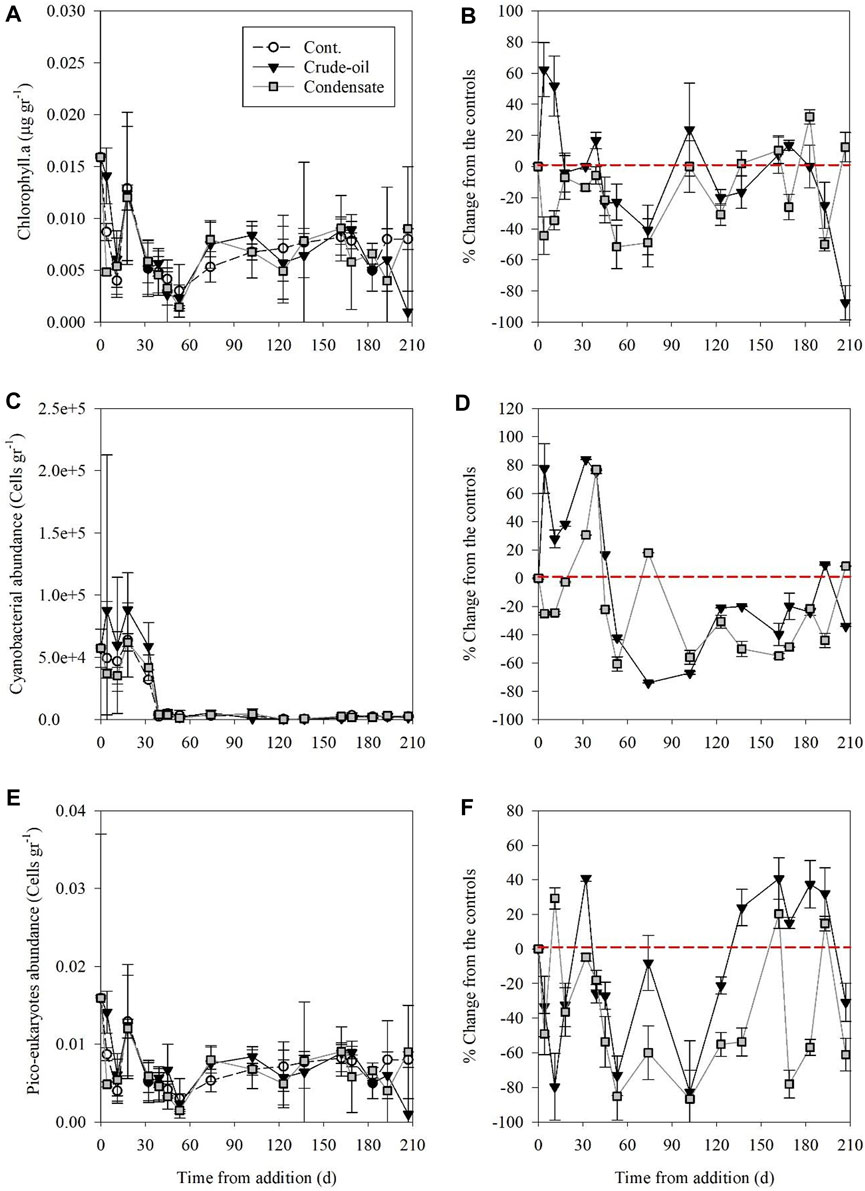
FIGURE 4. The temporal variability of surface sediment chlorophyll.a (A, B), cyanobacteria (C, D) and picoeukaryotic algae (E, F) following crude oil (black triangle) and gas condensate (grey square) additions and in the unamended control benthocosms (white dots). The values shown are the average of three independent “biological” replicates and their standard deviations. The red dashed line indicates no change between the treatments and the unamended controls at each time point.
Amplicon sequencing of the 16S rRNA gene showed that overall the benthic microbial communities were similar between treatments and the controls, complex and highly heterogeneous. Whereas alpha diversity appeared to increase in all the treatments compared to t0, no clear trends in alpha diversity were observed among the treatments, and there was ssubstantal noise in the data (Supplementary Figure S1). The dominant bacterial lineages were Alphaproteobacteria, Gammaproteobacteria, Bacteroidia and Planctomycetes (>10% of all ASVs each) (Supplementary Figure S2A). We observed drift of population structure in all treatments, in particular, at later stages, after a 48-h incubation (Supplementary Figure S2B). Despite the high heterogeneity of the samples, indicator species analysis revealed the enrichment of hydrocarbonoclastic bacteria following oil amendments (considering only the first two time points, before the drift in community composition) including Methylophaga, Ponticaulis and Alcanivorax genera (Table 2). Gas condensate amendments enriched a limited number of lineages whose metabolism is unknown as well as an Actinobacterota 67–14 lineage that has been associated with heavy metal contaminations in sediments (Table 2).

TABLE 2. Indicator species analysis of microbial lineages that were enriched in the sediments following crude oil or gas condensate addition 48 h after the beginning of the experiment. More details are in Supplementary Figure S1.
Discussion
Chronic hydrocarbon pollution alters porewater quality and microbial communities in the coastal sediments
The ambient ∑PAHs level in the unamended (control) littoral sediments was higher (yet insignificantly, t-test, p = 0.057) than the values reported over the shelf (∼30–200 m) and slope (200–1,000 m) of the eastern Mediterranean Sea in pristine areas (Barakat et al., 2011; Kucuksezgin et al., 2013; Astrahan et al., 2017). This suggests that the littoral sediments used in the benthocosms were moderately polluted by hydrocarbons, to begin with, regardless of any experimental manipulation. The source of this chronic-low level pollution maybe be tracked to the 1970-80s as discussed above (Golik et al., 1988). Note, however, that the ∑PAHs values we measured, which represent current-nowadays conditions in the littoral zone, are below the corresponding Effects Range Low (ERL) standards (Long et al., 1995), and are consistent with other observations from the eastern Mediterranean (Mandalakis et al., 2014).
The TOC levels measured in this study are in agreement with previously reported TOC values from the shelf of the southeastern Mediterranean Sea (Astrahan et al., 2017). The variation in TOC values may be attributed to differences in grain sizes (de Haas et al., 2002) and other land-derived processes (Herut et al., 2000; Efrati et al., 2013). PAHs are often positively correlated with TOC in sediments (Lohmann, 2003; Oleszczuk and Baran, 2015). In the control benthocosms there was no clear relationship between TOC and ∑PAHs, in agreement with the different natural sources for these molecules (that is, PAHs are generally associated with anthropogenic pollution).
The ambient NO2− + NO3− and PO43+ concentrations in the porewater are in agreement with the low nutrient levels characteristic of this oligotrophic region (Sisma-Ventura et al., 2021). The corresponding average ∼1:1 N:P ratio of the inorganic fraction suggests N-limiting conditions for autotorphic microbial populations (Redfield, 1934; Moore et al., 2013). The ambient concentrations of hydrocarbons in eastern Mediterranean sediments (Golik et al., 1988) suggest that in the case of a hydrocarbon spill there may be a microbial inoculum of hydrocarbon degraders able to use and decompose some of the hydrocarbons. Our molecular results support this notion, showing the presence of hydrocarbon degraders such as Methylophaga and Alcanivorax in the control benthocosms, though in low abundances (Supplementary Figure S2). This is not surprising since hydrocarbon-degrading bacteria such as Alcanivorax were previously reported in the southeastern Mediterranean Sea, both in the sediments (Guy-Haim et al., 2020; Rubin-Blum et al., 2022) and in the water column (Keuter et al., 2015).
Hydrocarbons reach nearshore sediments and alter microbial activity and diversity
Our experiments simulated a moderate/high hydrocarbon spill in a coastal environment without physical mixing by wave movement. Under such conditions, most hydrocarbons likely bypass the 25 cm water layer as MOS and reach the sediments as sporadically-dispersed particles. This may explain the considerable heterogeneity in our results, as there likely was no consistent diffusion of hydrocarbons from the slick, but mainly formation and sinking of hydrocarbon-containing particles, that reach the sediments sporadically. Nonetheless, crude oil and gas condensate amendments resulted in a significant increase in ∑PAHs and TOC levels (Table 1). These enrichments were more pronounced in crude oil amendments, whereas gas condensate may have triggered a delayed response (i.e., the increase in ∑PAHs was observed only at the end time-point). A positive-linear relationship between TOC and ΣPAHs was observed (Pearson, p < 0.05), suggesting a common source or the existence of carbon moieties in the PAHs molecules. A similar positive relationship was also found in several stations on the southern part of the Israeli coast in proximity to gas drilling sites and production platforms (Astrahan et al., 2017), suggesting that in both cases TOC and PAHs are linked. However, the ∑PAHs concentration was below its ERL standards for coastal sediments (Long et al., 1995), and significantly lower than some chronically polluted sediments in the Mediterranean Sea such as Alexandria harbor (Barakat et al., 2002) and El-Arish or El-Mex (Aly Salem et al., 2013). This is in line with the moderate, yet prolonged, effects we observed in the incubations.
Our results indicate that both crude oil and gas condensate may trigger similarly prolonged (months and possibly more), yet usually moderate changes in benthic microbial abundances and diversity. This is surprising considering gas condensate consists of many volatile compounds and should potentially be less toxic on long timescales than crude oil (Øksenvåg et al., 2017). This suggests that the remaining non-volatile PAH molecules in gas condensate, either solely or cumulatively, impact benthic algae and bacteria. We also propose that volatile molecules from gas condensate attached to aerosols may be redeposited in the surface water downwind of the spill site with toxic effects. This issue should be examined in future studies.
The overall minor changes in heterotrophic microbial variables exposed to hydrocarbons may be explained in several not mutually exclusive ways: 1) Hydrocarbon degraders that were present before the amendments may become active at the expense of non-hydrocarbon degrading bacteria. Under these circumstances, bacterial abundance may not necessarily change, but selection of more tolerant microbes may occur, and bacterial activity is expected to increase; 2) Macronutrients such as N or P may limit bacterial growth post hydrocarbon additions (Shai et al., 2021) and 3) toxic effects of some PAH molecules (e.g., Naphthalene and\or benzo(a)pyrene, Supplementary Table S2) reduce bacterial growth variables (excluding hydrocarbon degraders). The decrease in NO2− + NO3− levels following crude oil or gas condensate additions relative to the controls in the first ∼100 days post-addition (Figures 2A,B and Supplementary Table S1), was likely due to increased bacterial use through assimilation and denitrification, accompanied by elevated bacterial production rates (Figures 3C,D and Supplementary Table S1). Indeed, crude oil amendments were previously shown to stimulate denitrification (Zhao et al., 2020). We may also consider the role of benthic dinitrogen (N2) fixation, which could be responsible for the increase in NO2− + NO3− levels relative to the controls in the remaining days till the conclusion of the experiment (Figures 2A,B; Supplementary Table S1), as diazotrophs were found to tolerate oil-polluted sediments in an estuary system (Chronopoulou et al., 2013). Given the nitrogen limitation of hydrocarbon degradation (Musat et al., 2006), and the increased budget of energy-rich compounds that can support the costly N2 fixation, diazotrophs may indeed have an advantage in the long term, producing nitrogen that can yield new NO2− + NO3−. Notably, we did not measure denitrification or N2 fixation rates or detect specific-related genes such as the nirK or nifH. Therefore, we cannot ascertain whether this interplay between different biochemical processes occurred. However, many denitrifying bacteria and heterotrophic diazotrophs comprise the bacterial classes we found using 16S rDNA analyses (Table 2, Supplementary Figure S2), so it is likely. Future investigation of physiological rates and microbial functions is needed to investigate the N budgets following hydrocarbon introduction to the sediments, either directly or through MOS.
Along with nitrogen, phosphorus is likely to be a co-limiting nutrient for hydrocarbon degraders in this area, exemplified by the increased bacterial activity coupled with the decline in PO43+. Unlike inorganic nitrogen, porewater PO43+ is rapidly consumed by benthic microorganisms, highlighting the P-limiting conditions for bacteria in this oligotrophic area (Sisma-Ventura and Rahav, 2019) and hence the commonly observed P influx from the water column to the sediment (Sisma-Ventura et al., 2021; Rubin-Blum et al., 2022). Given that orthophosphate has very few external and sporadic sources (e.g., dust deposition, Guieu et al., 2014), hydrocarbon-degrading bacteria in the eastern Mediterranean Sea are expected to face severe P-limitation. Contrary, nitrogen can be fixed biologically by benthic diazotrophs (Musat et al., 2006) therefore compensating for the low nitrogen availability.
Observations made in a temporal study following the Prestige oil spill off northwestern Spain (Alonso-Gutiérrez et al., 2009) indicated that bacterial abundances almost did not vary, following our results (Figures 3A,B, Supplementary Table S1). On the other hand, bacterial productivity increased significantly (Figures 3C,D), and bacterial diversity changed substantially (Supplementary Figure S1) in the treated benthocosms compared to the controls (Supplementary Table S1). One such alteration could be explained by the interplay between denitrifying bacteria and diazotrophs discussed above. Our results, therefore, suggest that monitoring bacterial activity is a powerful tool to pinpoint hydrocarbon pollution, whereas bacterial abundance measurements may be misleading or not indicative.
Given that PAHs, such as benzo(a)pyren and naphthalene that were prominent in our experiments (Supplementary Table S2) and are considered toxic to autotrophic microbes (Hylland, 2006; Ozhan and Bargu, 2014), the significant decrease in benthic picoeukaryote abundances following the addition of crude oil or gas condensate is not surprising. However, picoeukaryotes may be also outcompeted for nutrients (e.g., N, P) by the fast-growing hydrocarbon-degrading heterotopic bacteria. In addition, hydrocarbon-degrading bacteria may not only benefit from the increased availability of metabolic substrates but also “enjoy” the limited grazing pressure due to the toxic effects of the PAHs (Chronopoulou et al., 2013). Moreover, the increase in the ∑PAHs after the experiment in the gas condensate benthocosms (day 207, Table 2) may suggest increased toxicity to infaunal organisms, including microbes.
Alphaproteobacteria, Deltaproteobacteria and Gammaproteobacteria play a role in crude oil degradation due to their ability to use aliphatic and aromatic compounds (David et al., 2005; Joel et al., 2011; Doyle et al., 2018). Bacteroidetes that can also use hydrocarbons occur in oil-contaminated sediments (Paissé et al., 2008; Sun et al., 2013; Korlević et al., 2015) (Yakimov et al., 2007; Quero et al., 2015). At higher taxonomic ranks, hydrocarbon-degrading bacteria were represented by Oceanospirillales, Cellvibrionales, Alteromonadales, Sphingomonadales, Steroidobacterales, and Flavobacteriales orders, and particularly by Methylophaga and Alcanivorax genera, in both the hydrocarbon-added benthocosms and the controls. This suggests that the benthic microbial communities in our study site routinely encounter hydrocarbons, as discussed above.
Summary and future ramifications
This study adds to our understanding of the complex response of benthic microbial populations in “low nutrients low chlorophyll” (LNLC) benthic marine environments to crude oil and gas condensate spills. The southeastern Mediterranean Sea has experienced a vast increase in seabed exploitation, development of offshore platforms and massive oil transport, thereby posing many risks to this ecologically-sensitive environment. This is especially true for the seabed which is a long-term repository for contaminants, different from the water column where pollutants are eventually diluted and interact with oxygen and light.
Our results suggest that crude oil and gas condensate spills may lead to prolonged responses in benthic microbial productivity and diversity. In agreement with previous studies, we show that autotroph abundance declined following hydrocarbon addition. Our results also suggest that hydrocarbon pollution may alter nutrient budgets affecting geochemical cycles (e.g., denitrification vs. N2 fixation), thereby changing the nutrient regime for benthic heterotrophic bacteria and autotrophic microbes. We show that while heterotrophic bacterial abundances and diversity remain stable for several months post hydrocarbon addition (Supplementary Figure S1), their production rates rapidly increase, suggesting that activity measures can be useful tools for monitoring crude oil or gas condensate pollution. Measurement of microbial activity can be supported by an array of molecular (e.g., “omics”) and biochemical (e.g., denitrification vs. N2 fixation) assays, identifying the metabolic potential and directly measuring macromolecule degradation.
We surmise that while crude oil produces MOS that can enrich hydrocarbon degraders, gas condensate may reduce MOS fluxes due to its toxicity to microorganisms involved in the formation of marine snow. This could be the reason for the lower increase in bacterial production rates as well as the delayed transport of PAHs to the sediment. A few days or weeks after gas condensate spills, these become less toxic due to the release of volatile short-chain hydrocarbons to the atmosphere, thus potentially favoring flocculation and eventually MOS. These aspects should be determined in future dedicated studies.
One of the ways to mitigate hydrocarbon pollution in surface seawater is the use of dispersants (Dave and Ghaly, 2011). These surfactant agents increase the accumulation of PAHs in the sediments (Cai et al., 2014), and thus impact sediment ecosystem functions and pose significant health risks as hydrocarbon derivatives enter the food web (Montagna et al., 2013). Concerning our results, the use of dispersants may further increase bacterial activity and function. However, the effects of dispersants are ambiguous and more studies are needed to fully appreciate their effects on the benthic environment (Ferguson et al., 2017).
Outcomes from this study will enable the development of science-based monitoring tools aimed to detect the effects of crude oil and gas condensate spills in the benthic environment, in line with UNEP’s Integrated Monitoring and Assessment Program for the Mediterranean Sea (IMAP) as part of the Barcelona Convention Mediterranean Action Plan.
Data availability statement
The datasets presented in this study can be found in online repositories. The names of the repository/repositories and accession number(s) can be found in the article/Supplementary Material.
Author contributions
This work was conceived and performed by EK, DA, and ER. MR-B conducted the microbial diversity and bioinformatic analyses, NB and ER analyzed the microbial abundance and production; EK, NB, and ER analyzed the bacterial abundance and chlorophyll.a measurtments; GS-V analyzed the porewater inorganic nutrients. ER wrote the manuscript with substantial contributions from all co-authors.
Funding
This study was supported by the Ministry of Science and Technology (Grant # 001126) to MR-B, GS-V, and ER. MR-B is also funded by the Israeli Science Foundation (Grant 913/19). This work is in partial fulfillment of the M. Sc. thesis for EK (University of Haifa).
Acknowledgments
We would like to thank Yaron Gertner from the Israel Oceanographic & Limnological Research for his help in the TOC analyses. We also wish to thank Dar Golomb for making the illustration shown in Figure 1
Conflict of interest
The authors declare that the research was conducted in the absence of any commercial or financial relationships that could be construed as a potential conflict of interest.
Publisher’s note
All claims expressed in this article are solely those of the authors and do not necessarily represent those of their affiliated organizations, or those of the publisher, the editors and the reviewers. Any product that may be evaluated in this article, or claim that may be made by its manufacturer, is not guaranteed or endorsed by the publisher.
Supplementary material
The Supplementary Material for this article can be found online at: https://www.frontiersin.org/articles/10.3389/fenvs.2022.1051460/full#supplementary-material
References
Abbriano, R. M., Carranza, M. M., Hogle, S. L., Levin, R. A., Netburn, A. N., Seto, K. L., et al. (2011). Deepwater Horizon oil spill: A review of the planktonic response. Oceanogr. Wash. D. C). 24, 294–301. doi:10.5670/oceanog.2011.80
Almeda, R., Connelly, T. L., and Buskey, E. J. (2016). How much crude oil can zooplankton ingest? Estimating the quantity of dispersed crude oil defecated by planktonic copepods. Environ. Pollut. 208, 645–654. doi:10.1016/j.envpol.2015.10.041
Alonso.Gutiérrez, J., Antonio, F., Joan, A., Núria, J., and Marc, V., (2009). Bacterial communities from shoreline environments (Costa da Morte, Northwestern Spain) affected by the Prestige Oil Spill. Appl. Environ. Microbiol. 75, 3407–3418. doi:10.1128/AEM.01776-08
Aly Salem, D. M. S., Khaled, A., and El Nemr, A. (2013). Assessment of pesticides and polychlorinated biphenyls (PCBs) in sediments of the Egyptian Mediterranean Coast. Egypt. J. Aquatic Res. 39, 141–152. doi:10.1016/j.ejar.2013.11.001
Apprill, A., Mcnally, S., Parsons, R., and Weber, L. (2015). Minor revision to V4 region SSU rRNA 806R gene primer greatly increases detection of SAR11 bacterioplankton. Aquat. Microb. Ecol. 75, 129–137. doi:10.3354/ame01753
Astrahan, P., Silverman, J., Gertner, Y., and Herut, B. (2017). Spatial distribution and sources of organic matter and pollutants in the SE Mediterranean ( Levantine basin ) deep water sediments. Mar. Pollut. Bull. 116, 521–527. doi:10.1016/j.marpolbul.2017.01.006
Bacosa, H. P., Kamalanathan, M., Cullen, J., Shi, D., Xu, C., Schwehr, K. A., et al. (2020). Marine snow aggregates are enriched in polycyclic aromatic hydrocarbons (Pahs) in oil contaminated waters: Insights from a mesocosm study. J. Mar. Sci. Eng. 8, 781–816. doi:10.3390/jmse8100781
Banerjee, A., Ghosh, D., and Das, S. (2018). Modified firefly algorithm for area estimation and tracking of fast expanding oil spills. Appl. Soft Comput. 73, 829–847. doi:10.1016/j.asoc.2018.09.024
Barakat, A. O., Moonkoo, K., Yoarong, Q., and Wade, T. L. (2002). Organochlorine pesticides and PCB residues in sediments of Alexandria Harbour, Egypt. Mar. Pollut. Bull. 44, 1426–1434. doi:10.1016/s0025-326x(02)00313-2
Barakat, A. O., Mostafa, A., Wade, T. L., Sweet, S. T., and El Sayed, N. B. (2011). Distribution and characteristics of PAHs in sediments from the Mediterranean coastal environment of Egypt. Mar. Pollut. Bull. 62, 1969–1978. doi:10.1016/j.marpolbul.2011.06.02410.1016/j.marpolbul.2011.06.024
Bienhold, C., Zinger, L., Boetius, A., and Ramette, A. (2016). Diversity and biogeography of bathyal and abyssal seafloor bacteria. PLoS One 11, 0148016. doi:10.1371/journal.pone.0148016
Bolyen, E., Rideout, J. R., Dillon, M. R., Bokulich, N. A., Abnet, C. C., Al-Ghalith, G. A., et al. (2019). Reproducible, interactive, scalable and extensible microbiome data science using QIIME 2. Nat. Biotechnol. 37, 852–857. doi:10.1038/s41587-019-0209-9
Brussaard, C. P. D., Peperzak, L., Beggah, S., Wick, L. Y., Wuerz, B., Weber, J., et al. (2016). Immediate ecotoxicological effects of short-lived oil spills on marine biota. Nat. Commun. 7, 11206–11211. doi:10.1038/ncomms11206
Cáceres, M. De, and Legendre, P. (2009). Associations between species and groups of sites: Indices and statistical inference. Ecology 90, 3566–3574. doi:10.1890/08-1823.1
Cai, X., Zhang, Q., Wang, S., Peng, J., Zhang, Y., Ma, H., et al. (2014). Surfactant-assisted synthesis of reduced graphene oxide/polyaniline composites by gamma irradiation for supercapacitors. J. Mat. Sci. 49, 5667–5675. doi:10.1007/s10853-014-8286-0
Callahan, B. J., McMurdie, P. J., Rosen, M. J., Han, A. W., Johnson, A. J. A., and Holmes, S. P. (2016). DADA2: High-resolution sample inference from Illumina amplicon data. Nat. Methods 13, 581–583. doi:10.1038/nmeth.3869
Carpenter, A., and Kostianoy, A. G. (2018). in Oil pollution in the Mediterranean Sea: Part I: The international context. Editors A. Carpenter, and A. G. Kostianoy (Berlin: Springer).
Chanton, J., Zhao, T., Rosenheim, B. E., Joye, S., Bosman, S., Brunner, C., et al. (2015). Using natural abundance radiocarbon to trace the flux of petrocarbon to the seafloor following the deepwater Horizon oil spill. Environ. Sci. Technol. 49, 847–854. doi:10.1021/es5046524
Chronopoulou, P.-M., Fahy, A., Coulon, F., Païssé, S., Goñi-Urriza, M., Peperzak, L., et al. (2013). Impact of a simulated oil spill on benthic phototrophs and nitrogen-fixing bacteria in mudflat mesocosms. Environ. Microbiol. 15, 242–252. doi:10.1111/j.1462-2920.2012.02864.x
Cravo-Laureau, C., and Duran, R. (2014). Marine coastal sediments microbial hydrocarbon degradation processes: Contribution of experimental ecology in the omics’era. Front. Microbiol. 5, 39–48. doi:10.3389/fmicb.2014.00039
Daly, K. L., Passow, U., Chanton, J., and Hollander, D. (2016). Assessing the impacts of oil-associated marine snow formation and sedimentation during and after the Deepwater Horizon oil spill. Anthropocene 13, 18–33. doi:10.1016/j.ancene.2016.01.006
Dave, D., and Ghaly, A. E. (2011). Remediation technologies for marine oil spills: A critical review and comparative analysis. Am. J. Environ. Sci. 7, 423–440. doi:10.3844/ajessp.2011.423.440
David, G., Bruno, D., Philippe, P., Guillemette, J., and Philippe, D. (2005). Structure of sediment-associated microbial communities along a heavy-metal contamination gradient in the marine environment. Appl. Environ. Microbiol. 71, 679–690. doi:10.1128/AEM.71.2.679-690.2005
de Haas, H., van Weering, T. C. E., and de Stigter, H. (2002). Organic carbon in shelf seas: Sinks or sources, processes and products. Cont. Shelf Res. 22, 691–717. doi:10.1016/s0278-4343(01)00093-0
Doyle, S. M., Whitaker, E. A., Pascuale, V. De, Wade, T. L., Knap, A. H., Santschi, P. H., et al. (2018). Rapid formation of microbe-oil aggregates and changes in community composition in coastal surface water following exposure to oil and the dispersant corexit. Front. Microbiol. 9, 689–716. doi:10.3389/fmicb.2018.00689
Efrati, S., Lehahn, Y., Rahav, E., Kress, N., Herut, B., Gertman, I., et al. (2013). Intrusion of coastal waters into the pelagic eastern mediterranean: In situ and satellite-based characterization. Biogeosciences 10, 3349–3357. doi:10.5194/bg-10-3349-2013
Faramawy, S., Zaki, T., and Sakr, A. A.-E. (2016). Natural gas origin, composition, and processing: A review. J. Nat. Gas. Sci. Eng. 34, 34–54. doi:10.1016/j.jngse.2016.06.030
Feng, H., Han, X., Zhang, W., and Yu, L. (2004). A preliminary study of heavy metal contamination in Yangtze River intertidal zone due to urbanization. Mar. Pollut. Bull. 49, 910–915. doi:10.1016/j.marpolbul.2004.06.014
Ferguson, R. M. W., Gontikaki, E., Anderson, J. A., and Witte, U. (2017). The variable influence of dispersant on degradation of oil hydrocarbons in subarctic deep-sea sediments at low temperatures (0-5 °C). Sci. Rep. 7, 2253–2313. doi:10.1038/s41598-017-02475-9
Frank, H., Fussmann, K. E., Rahav, E., and Bar Zeev, E. (2019). Chronic effects of brine discharge from large-scale seawater reverse osmosis desalination facilities on benthic bacteria. Water Res. 151, 478–487. doi:10.1016/j.watres.2018.12.046
Frank, H., Rahav, E., and Bar-Zeev, E. (2017). Short-term effects of SWRO desalination brine on benthic heterotrophic microbial communities. Desalination 417, 52–59. doi:10.1016/j.desal.2017.04.031
Gieg, L. M., Kolhatkar, R. V., McInerney, M. J., Tanner, R. S., Harris Steve, H., Sublette, K. L., et al. (1999). Intrinsic bioremediation of petroleum hydrocarbons in a gas condensate-contaminated aquifer. Environ. Sci. Technol. 33, 2550–2560. doi:10.1021/es981349d
Glöckner, F. O., Yilmaz, P., Quast, C., Gerken, J., Beccati, A., Ciuprina, A., et al. (2017). 25 years of serving the community with ribosomal RNA gene reference databases and tools. J. Biotechnol. 261, 169–176. doi:10.1016/j.jbiotec.2017.06.1198
Gobet, A., Böer, S. I., Huse, S. M., van Beusekom, J. E. E., Quince, C., Sogin, M. L., et al. (2012). Diversity and dynamics of rare and of resident bacterial populations in coastal sands. ISME J. 6, 542–553. doi:10.1038/ismej.2011.132
Golik, A., Weber, K., Salihoglu, I., Yilmaz, A., and Loizides, L. (1988). Pelagic tar in the Mediterranean Sea. Mar. Pollut. Bull. 19, 567–572. doi:10.1016/0025-326x(88)90020-3
Gregson, B. H., McKew, B. A., Holland, R. D., Nedwed, T. J., Prince, R. C., and McGenity, T. J. (2021). Marine oil snow, a microbial perspective. Front. Mar. Sci. 8. doi:10.3389/fmars.2021.619484
Guieu, C., Aumont, O., Paytan, A., Bopp, L., Law, C. S., Mahowald, N., et al. (2014). The significance of the episodic nature of atmospheric deposition to Low Nutrient Low Chlorophyll regions. Glob. Biogeochem. Cycles 28, 1179–1198. doi:10.1002/2014GB004852
Hasse, L., and Liss, P. S. (1980). Gas exchange across the air — sea interface. Tellus B 32, 470–481. doi:10.3402/tellusa.v32i5.10602
Hatcher, P. G., Obeid, W., Wozniak, A. S., Xu, C., Zhang, S., Santschi, P. H., et al. (2018). Identifying oil/marine snow associations in mesocosm simulations of the Deepwater Horizon oil spill event using solid-state 13C NMR spectroscopy. Mar. Pollut. Bull. 126, 159–165. doi:10.1016/j.marpolbul.2017.11.004
Hazen, T. C., Dubinsky, E. a., DeSantis, T. Z., Andersen, G. L., Piceno, Y. M., Singh, N., et al. (2010). Deep-sea oil plume enriches indigenous oil-degrading bacteria. Science 330, 204–208. doi:10.1126/science.1195979
Herut, B., Almogi-Labin, A., Jannink, N., and Gertman, I. (2000). The seasonal dynamics of nutrient and chlorophyll a concentrations on the SE Mediterranean shelf-slope. Oceanol. Acta 23, 771–782. doi:10.1016/S0399-1784(00)01118-X
Hylland, K. (2006). Polycyclic aromatic hydrocarbon (PAH) ecotoxicology in marine ecosystems. J. Toxicol. Environ. Health Part A 69, 109–123. doi:10.1080/15287390500259327
Joel, K., Om, P., Gina, F., and Andy, C., (2011). Hydrocarbon-degrading bacteria and the bacterial community response in Gulf of Mexico beach sands impacted by the Deepwater Horizon oil spill. Appl. Environ. Microbiol. 77, 7962–7974. doi:10.1128/AEM.05402-11
Johnston, E. L., and Roberts, D. A. (2009). Contaminants reduce the richness and evenness of marine communities: A review and meta-analysis. Environ. Pollut. 157, 1745–1752. doi:10.1016/j.envpol.2009.02.017
Keuter, S., Rahav, E., Herut, B., and Rinkevich, B. (2015). Distribution patterns of bacterioplankton in the oligotrophic south-eastern Mediterranean Sea. FEMS Microbiol. Ecol. 91, fiv070. doi:10.1093/femsec/fiv070
Korlević, M., Zucko, J., Dragić, M. N., Blažina, M., Pustijanac, E., Zeljko, T. V., et al. (2015). Bacterial diversity of polluted surface sediments in the northern Adriatic Sea. Syst. Appl. Microbiol. 38, 189–197. doi:10.1016/j.syapm.2015.03.001
Kress, N., Shoham-Frider, E., and Galil, B. S. (2016). Twenty two years of sewage sludge marine disposal monitoring in the Eastern Mediterranean Sea: Impact on sediment quality and infauna and the response to load reduction. Mar. Pollut. Bull. 110, 99–111. doi:10.1016/j.marpolbul.2016.06.076
Kucuksezgin, F., Pazi, I., Tolga Gonul, L., and Duman, M. (2013). Distribution and sources of polycyclic aromatic hydrocarbons in Cilician Basin shelf sediments (NE Mediterranean). Mar. Pollut. Bull. 71, 330–335. doi:10.1016/j.marpolbul.2013.01.026
Laflamme, R. E., and Hites, R. A. (1978). The global distribution of polycyclic aromatic hydrocarbons in recent sediments. Geochimica Cosmochimica Acta 42, 289–303. doi:10.1016/0016-7037(78)90182-5
Lee, K., and Levy, E. M. (1989). Enhancement of the natural biodegradation of condensate and crude oil on beaches of Atlantic Canada. Int. Oil Spill Conf. Proc. 1, 479–486. doi:10.7901/2169-3358-1989-1-479
Lohmann, R. (2003). The emergence of black carbon as a super-sorbent in environmental chemistry: The end of octanol? Environ. Forensics 4, 161–165. doi:10.1080/713848510
Long, E. R., Macdonald, D. D., Smith, S. L., and Calder, F. D. (1995). Incidence of adverse biological effects within ranges of chemical concentrations in marine and estuarine sediments. Environ. Manag. 19, 81–97. doi:10.1007/BF02472006
Luna, G. M., Manini, E., and Danovaro, R. (2002). Large fraction of dead and inactive bacteria in coastal marine sediments: Comparison of protocols for determination and ecological significance. Appl. Environ. Microbiol. 68, 3509–3513. doi:10.1128/AEM.68.7.3509-3513.2002
Mandalakis, M., Polymenakou, P. N., Tselepides, A., and Lampadariou, N. (2014). Distribution of aliphatic hydrocarbons, polycyclic aromatic hydrocarbons and organochlorinated pollutants in deep-sea sediments of the southern cretan margin, eastern Mediterranean Sea: A baseline assessment. Chemosphere 106, 28–35. doi:10.1016/j.chemosphere.2013.12.081
Martin, M. (2011). Cutadapt removes adapter sequences from high-throughput sequencing reads. EMBnet. J. 17, 10. doi:10.14806/ej.17.1.200
Mason, O. U., Scott, N. M., Gonzalez, A., Robbins-Pianka, A., Bælum, J., Kimbrel, J., et al. (2014). Metagenomics reveals sediment microbial community response to Deepwater Horizon oil spill. ISME J. 8, 1464–1475. doi:10.1038/ismej.2013.254
McMurdie, P. J., and Holmes, S. (2013). Phyloseq: An R package for reproducible interactive analysis and graphics of microbiome census data. PLoS One 8, 61217. doi:10.1371/journal.pone.0061217
Miao, J., Han, N., Qiang, Y., Zhang, T., Li, X., and Zhang, W. (2017). 16SPIP: A comprehensive analysis pipeline for rapid pathogen detection in clinical samples based on 16S metagenomic sequencing. BMC Bioinforma. 18, 568. doi:10.1186/s12859-017-1975-3
Montagna, P. A., Baguley, J. G., Cooksey, C., Hartwell, I., Hyde, L. J., Hyland, J. L., et al. (2013). Deep-sea benthic footprint of the deepwater Horizon blowout. PLoS One 8, 70540. doi:10.1371/journal.pone.0070540
Moore, C. M., Mills, M. M., Arrigo, K. R., Berman-Frank, I., Bopp, L., Boyd, P. W., et al. (2013). Processes and patterns of oceanic nutrient limitation. Nat. Geosci. 6, 701–710. doi:10.1038/ngeo1765
Musat, F., Harder, J., and Widdel, F. (2006). Study of nitrogen fixation in microbial communities of oil-contaminated marine sediment microcosms. Environ. Microbiol. 8, 1834–1843. doi:10.1111/j.1462-2920.2006.01069.x
Øksenvåg, J. H. ., Daling, P. S., Hellstrøm, K. C., Merethe-Bakken, O., and Johnsen, M. (2017). Sigyn condensate properties and behaviour at sea. Torgarden Available at: 978-82-7174-305-5.
Oleszczuk, P., and Baran, S. (2015). Degradation of individual polycyclic aromatic hydrocarbons (PAHs) in soil polluted with aircraft fuel. Pol. J. Environ. Stud. 12, 431–437.
Ozhan, K., and Bargu, S. (2014). Can crude oil toxicity on phytoplankton be predicted based on toxicity data on benzo(a)pyrene and naphthalene? Bull. Environ. Contam. Toxicol. 92, 225–230. doi:10.1007/s00128-013-1181-6
Paissé, S., Coulon, F., Goñi-Urriza, M., Peperzak, L., McGenity, T. J., and Duran, R. (2008). Structure of bacterial communities along a hydrocarbon contamination gradient in a coastal sediment. FEMS Microbiol. Ecol. 66, 295–305. doi:10.1111/j.1574-6941.2008.00589.x
Parada, A. E., Needham, D. M., and Fuhrman, J. A. (2016). Every base matters: Assessing small subunit rRNA primers for marine microbiomes with mock communities, time series and global field samples. Environ. Microbiol. 18, 1403–1414. doi:10.1111/1462-2920.13023
Peterson, C. H., Rice, S. D., Short, J. W., Esler, D., Bodkin, J. L., Ballachey, B. E., et al. (2003). Long-term ecosystem response to the Exxon Valdez oil spill. Science 302, 2082–2086. doi:10.1126/science.1084282
Quero, G. M., Cassin, D., Botter, M., Perini, L., and Luna, G. M. (2015). Patterns of benthic bacterial diversity in coastal areas contaminated by heavy metals , polycyclic aromatic hydrocarbons ( PAHs ) and polychlorinated biphenyls ( PCBs ). Front. Microbiol. 6, 1053–1115. doi:10.3389/fmicb.2015.01053
Rahsepar, S., van Eenennaam, J. S., Radović, J. R., Oldenburg, T. B. P., Rijnaarts, H. H. M., Murk, A. J., et al. (2022). Marine snow-oil interaction affects n-alkane biodegradation in sediment. Water, Air, Soil Pollut. 233, 84. doi:10.1007/s11270-022-05557-1
Redfield, A. (1934). “On the proportions of organic derivatives in sea water and their relation to the composition of phytoplankton,” in James johnstone memorial volume. Editor R. J. Daniel (Liverpool: University Press of Liverpool), 177–192.
Rocchetti, L., Beolchini, F., Hallberg, K. B., Johnson, D. B., and Dell’Anno, A. (2012). Effects of prokaryotic diversity changes on hydrocarbon degradation rates and metal partitioning during bioremediation of contaminated anoxic marine sediments. Mar. Pollut. Bull. 64, 1688–1698. doi:10.1016/j.marpolbul.2012.05.038
Rohal, M., Barrera, N., Van Eenennaam, J. S., Foekema, E. M., Montagna, P. A., Murk, A. J., et al. (2020). The effects of experimental oil-contaminated marine snow on meiofauna in a microcosm. Mar. Pollut. Bull. 150, 110656. doi:10.1016/j.marpolbul.2019.110656
Ross, J., Hollander, D., Saupe, S., Burd, A. B., Gilbert, S., and Quigg, A. (2021). Integrating marine oil snow and MOSSFA into oil spill response and damage assessment. Mar. Pollut. Bull. 165, 112025. doi:10.1016/j.marpolbul.2021.112025
Rubin-Blum, M., Sisma-Ventura, G., Yudkovski, Y., Belkin, N., Kanari, M., Herut, B., et al. (2022). Diversity, activity, and abundance of benthic microbes in the Southeastern Mediterranean Sea. FEMS Microbiol. Ecol. 98, fiac009–14. doi:10.1093/femsec/fiac009
Shaffer, B. (2011). Israel—new natural gas producer in the mediterranean. Energy Policy 39, 5379–5387. doi:10.1016/j.enpol.2011.05.026
Shai, Y., Rubin-blum, M., Angel, D. L., Sisma-ventura, G., Zurel, D., Astrahan, P., et al. (2021). Response of oligotrophic coastal microbial populations in the SE Mediterranean Sea to crude oil pollution ; lessons from mesocosm studies. Estuar. Coast. Shelf Sci. 249, 107102. doi:10.1016/j.ecss.2020.107102
Simon, M., and Azam, F. (1989). Protein content and protein synthesis rates of planktonic marine bacteria. Mar. Ecol. Prog. Ser. 51, 201–213. doi:10.3354/meps051201
Sisma-Ventura, G., Herut, B., Silverman, J., Katz, T., Rubin-Blum, M., and Rahav, E. (2021). P fluxes and prokaryotic cycling at benthic boundary layer in the deep southeastern Mediterranean Sea. J. Geophys. Res. Biogeosci. 126, 1–11. doi:10.1029/2020JG006110
Sisma-Ventura, G., and Rahav, E. (2019). DOP stimulates heterotrophic bacterial production in the oligotrophic southeastern mediterranean coastal waters. Front. Microbiol. 10, 1913. doi:10.3389/fmicb.2019.01913
Smith, D. C., Smith, D. C., Azam, F., and Azam, F. (1992). A simple, economical method for measuring bacterial protein synthesis rates in seawater using 3H-leucine. Mar. Microb. food web 6, 107–114.
Sun, M. Y., Dafforn, K. A., Johnston, E. L., and Brown, M. V ( (2013). Core sediment bacteria drive community response to anthropogenic contamination over multiple environmental gradients. Environ. Microbiol. 15, 2517–2531. doi:10.1111/1462-2920.12133
Valentine, D. L., Fisher, G. B., Bagby, S. C., Nelson, R. K., Reddy, C. M., Sylva, S. P., et al. (2014). Fallout plume of submerged oil from Deepwater Horizon. Proc. Natl. Acad. Sci. U. S. A. 111, 15906–15911. doi:10.1073/pnas.1414873111
Welschmeyer, N. A. (1994). Fluorometric analysis of chlorophyll a in the presence of chlorophyll b and pheopigments. Limnol. Oceanogr. 39, 1985–1992. doi:10.4319/lo.1994.39.8.1985
Yakimov, M. M., Timmis, K. N., and Golyshin, P. N. (2007). Obligate oil-degrading marine bacteria. Curr. Opin. Biotechnol. 18, 257–266. doi:10.1016/j.copbio.2007.04.006
Keywords: crude-oil, gas-condensate, hydrocarbon pollutants, benthic bacteria, benthic alga, eastern mediterranean sea
Citation: Kababu E, Angel DL, Sisma-Ventura G, Belkin N, Rubin-Blum M and Rahav E (2022) Effects of crude oil and gas condensate spill on coastal benthic microbial populations. Front. Environ. Sci. 10:1051460. doi: 10.3389/fenvs.2022.1051460
Received: 23 September 2022; Accepted: 03 November 2022;
Published: 15 November 2022.
Edited by:
Nsikak U. Benson, Covenant University, NigeriaReviewed by:
José Q. García-Maldonado, National Council of Science and Technology (CONACYT), MexicoRagaa Hamouda, University of Sadat City, Egypt
Agung Dhamar Syakti, Raja Ali Haji Maritime University, Indonesia
Copyright © 2022 Kababu, Angel, Sisma-Ventura, Belkin, Rubin-Blum and Rahav. This is an open-access article distributed under the terms of the Creative Commons Attribution License (CC BY). The use, distribution or reproduction in other forums is permitted, provided the original author(s) and the copyright owner(s) are credited and that the original publication in this journal is cited, in accordance with accepted academic practice. No use, distribution or reproduction is permitted which does not comply with these terms.
*Correspondence: E. Rahav, ZXlhbC5yYWhhdkBvY2Vhbi5vcmcuaWw=