- 1Global Science Team, World Wildlife Fund, Washington, DC, United States
- 2Lawrence Berkeley National Laboratory, Berkeley, CA, United States
- 3PSR Energy Consulting and Analytics, Rio de Janeiro, Brazil
- 4Natural Capital Project and the Woods Institute for the Environment, Stanford University, Stanford, CA, United States
- 5School of Earth, Environmental, and Marine Sciences, The University of Texas Rio Grande Valley, Edinburg, TX, United States
- 6Onward Energy, Charlotte, NC, United States
- 7Department of Ecology and Evolutionary Biology, Cornell University, Ithaca, NY, United States
- 8WWF-Asia Pacific, Ho Chi Minh City, Vietnam
- 9Confluvio, Montreal, QC, Canada
- 10Department of Mechanical, Aerospace and Civil Engineering, The University of Manchester, Manchester, United Kingdom
- 11Department of Civil, Environmental and Geomatic Engineering, University College London, London, United Kingdom
- 12Sustainable Water & Energy LLC, Estes Park, CO, United States
- 13The Nature Conservancy, Arlington, VA, United States
- 14Renewable and Appropriate Energy Laboratory, University of California, Berkeley, Berkeley, CA, United States
- 15The Nature Conservancy, Bogota, Colombia
- 16WWF-Nepal, Kathmandu, Nepal
- 17World Wildlife Fund-US, Washington, DC, United States
- 18Independent Consultant, Boulder, CO, United States
As governments and non-state actors strive to minimize global warming, a primary strategy is the decarbonization of power systems which will require a massive increase in renewable electricity generation. Leading energy agencies forecast a doubling of global hydropower capacity as part of that necessary expansion of renewables. While hydropower provides generally low-carbon generation and can integrate variable renewables, such as wind and solar, into electrical grids, hydropower dams are one of the primary reasons that only one-third of the world’s major rivers remain free-flowing. This loss of free-flowing rivers has contributed to dramatic declines of migratory fish and sediment delivery to agriculturally productive deltas. Further, the reservoirs behind dams have displaced tens of millions of people. Thus, hydropower challenges the world’s efforts to meet climate targets while simultaneously achieving other Sustainable Development Goals. In this paper, we explore strategies to achieve the needed renewable energy expansion while sustaining the diverse social and environmental benefits of rivers. These strategies can be implemented at scales ranging from the individual project (environmental flows, fish passage and other site-level mitigation) to hydropower cascades to river basins and regional electrical power systems. While we review evidence that project-level management and mitigation can reduce environmental and social costs, we posit that the most effective scale for finding balanced solutions occurs at the scale of power systems. We further hypothesize that the pursuit of solutions at the system scale can also provide benefits for investors, developers and governments; evidence of benefits to these actors will be necessary for achieving broad uptake of the approaches described in this paper. We test this hypothesis through cases from Chile and Uganda that demonstrate the potential for system-scale power planning to allow countries to meet low-carbon energy targets with power systems that avoid damming high priority rivers (e.g., those that would cause conflicts with other social and environmental benefits) for a similar system cost as status quo approaches. We also show that, through reduction of risk and potential conflict, strategic planning of hydropower site selection can improve financial performance for investors and developers, with a case study from Colombia.
1 Introduction
To maintain a stable climate, most of the world’s governments, along with non-state actors, have committed to reducing greenhouse gas emissions, including through electrification of several currently non-electrified energy uses while simultaneously decarbonizing electricity systems. Hydropower is currently the world’s leading source of renewable and low-carbon electricity and, although wind and solar photovoltaic (PV) are projected to become the dominant forms of generation by 2050, many influential energy institutions forecast that global hydropower capacity will double in that time period (IEA, 2021; IRENA, 2021). The need to dramatically expand renewable generation to meet the challenge of curbing climate change triggers a related challenge: sustainably deploying the new energy projects by minimizing conflicts with other social and environmental resources and objectives, such as those articulated in the Sustainable Development Goals (SDGs). Because conventional hydropower requires the damming of rivers, it poses particular challenges to achieving this balancing of objectives. For example, because the reservoirs behind hydropower dams can trap rivers’ sediment supplies, hydropower expansion can accelerate the degradation of heavily populated and agriculturally crucial deltas, such as the Mekong (Kondolf et al., 2022), with negative impacts for SDGs such as those for safe and resilient cities (SDG 11) and food supplies (SDG 2). In this paper we explore how hydropower development and management can be balanced with other objectives, at scales ranging from individual dams to river basins to regional power systems.
Our central hypothesis is that while there are solutions across these spatial scales, the greatest potential for achieving this balance will occur at the scale of power systems. That is, the pursuit of “sustainable hydropower” is most effectively defined, and pursued, within a broader concept of sustainable power systems. This broader framing provides an overarching structure within which to compare tradeoffs, identify options, and define a sustainable role for hydropower within an overall power system.
In addition to reviewing a set of spatially nested solutions (from dams to river basins to electrical grids), we also explore the hypothesis that system-scale approaches can reduce a range of risks for various actors (e.g., governments, companies, and communities). While attempts to improve the sustainability of hydropower often focus on minimizing risks to rural communities or ecosystems, broad uptake of the approaches we describe will hinge on them also reducing risks for actors who typically have a strong influence on decisions about water-management and power systems, including investors, developers and government agencies. Thus, we explore how pursuing more balanced outcomes from hydropower development and operation—evaluated within overall power systems—can reduce risks not just for communities and ecosystems but also for these influential actors. We test these hypotheses through a combination of literature review and data analysis.
2 Hydropower in power systems: Services and risks
Hydropower, or hydroelectric power, represents approximately 16 percent of global electricity generation from a global hydropower capacity of 1,230,000 MW (IRENA, 2020). In addition to generation, hydropower projects can also offer various ancillary energy services that benefit grid reliability. Further, hydropower projects that store water in a reservoir are essentially storing potential energy that can relatively quickly be converted to electrical energy that is dispatchable to a grid, allowing services such as peaking and load following (see discussion below of types of hydropower). Through these services, hydropower can help balance the intermittent generation from renewables, such as wind and solar PV. Thus, hydropower can contribute to mitigating climate change in two ways: as a direct source of low-carbon generation and, through a range of grid services, facilitating a greater proportion of variable renewables into a power system (Montero and Perez, 2009).
Conventional hydropower projects, which generally require a dam across a river, can be classified as either storage or run-of-river. By maintaining water in a reservoir, storage projects reduce flow variability during the year and can allow a more consistent and predictable flow through turbines. Large reservoirs can store sufficient water to reduce the variability between dry years and wet years. Hydropower dams with storage reservoirs are often multi-purpose, in that they generate electricity but are also operated to achieve other purposes such as flood-risk management and storage for irrigation or municipal water supply. Of the approximately 10,000 hydropower dams in the database of the International Commission on Large Dams, approximately 60% are considered single purpose and 40% multipurpose (Branche, 2015).
Run-of-river projects have minimal storage and thus do not alter seasonal patterns of flow, and generation may vary considerably across the year (e.g., operating at 100% capacity factor during the rainy season and often considerably lower capacity factors during the dry season). Run-of-river projects can be operated for short-term storage, allowing “hydropeaking” operations (e.g., storing water for 20 hours and then releasing a high discharge for 4 hours during a period of peak demand). Note that run-of-river projects are often incorrectly generalized as having considerably lower environmental impacts than dams with reservoirs, or even that run-of-river projects do not require building a dam (e.g., see the blog post on Forbes.com in which Katusa (2010) states that run-of-river hydropower does not require a dam). However, although run-of-river projects do not alter seasonal patterns of flow, they can be operated to have considerable daily and sub-daily flow fluctuations (e.g., when used for hydropeaking (Almeida et al., 2020)) and they can also have major impacts on longitudinal connectivity for aquatic species, coarse sediment, and river processes. For example, two run-of-river dams on the Madeira River in the Amazon Basin have been shown to impact downstream hydrology and populations of gilded catfish (Brachyplatystoma rousseauxii), a migratory species important for regional fisheries (Damme et al., 2019; Almeida et al., 2020). Hydropower dams on the mainstem Mekong have also been predicted to have major impacts on fish migration and biomass (International Centre for Environmental Management, 2010) and sediment transport (Schmitt et al., 2019); as some mainstem dams have now been completed, these predicted impacts are increasingly observable. These Mekong dams, such as Xayaboury (operational) and Sambor (proposed), are classified as run-of-river projects because their storage is small compared to the river’s large discharge, yet they are large structures (dam heights of 32 m and 56 m, respectively) capable of major disruptions to longitudinal connectivity, illustrating that run-of-river projects should not be assumed to have minimal impacts on riverine ecosystems and services.
Unlike conventional hydropower, pumped storage hydropower (PSH) is not a source of generation but rather storage, representing more than 95% of storage available to grids worldwide (Blakers et al., 2021). In a PSH project, water is pumped uphill, generally when electricity is plentiful and lower cost, and stored in an upper reservoir. The upper reservoir can then release water back downhill through turbines to rapidly generate power when electricity demand is high, providing load balancing to a power system (Hunt et al., 2020; Gilfillan and Pittock, 2022). Note that some PSH can be developed “off channel,” cycling water between two reservoirs and not directly affecting river connectivity or flows (Blakers et al., 2021).
While generally considered a low-carbon source of generation (the IPCC estimates that life-cycle emissions from hydropower are five percent that of natural gas and three percent that of coal (Schlömer et al., 2014)), the reservoirs associated with hydropower projects can be a source of greenhouse gas emissions, including carbon dioxide and methane (Deemer et al., 2016; Ocko and Hamburg, 2019). For example, Räsänen et al. (2018) found that while 107 out of 141 (76%) of hydropower reservoirs in the Mekong basin had life cycle emissions in the range of other renewables, the others had higher emissions, including 14 (10%) with emissions comparable to those of fossil fuel plants. The risk factors for high emissions from reservoirs are relatively well known, including shallow reservoirs, tropical environments, reservoirs where vegetation was not cleared prior to filling, and upstream sources of nutrients (Deemer et al., 2016). Emissions from reservoirs is a focus of ongoing research and, clearly, the potential for a hydropower project to have higher emissions than other renewables should be a key screening criterion for any proposed project (Almeida et al., 2019). The Hydropower Sustainability Standard, developed through a process led by the International Hydropower Association (IHA), includes standards to ensure that hydropower projects are “consistent with low carbon power generation” (Hydropower Sustainability Secretariat, 2021).
Global capacity of hydropower grew rapidly in the first decade of this century, with annual capacity additions in 2013 being four times greater than the annual additions at the beginning of the century (Figure 1). Annual capacity additions have declined since 2013, with average additions since 2017 at less than half the 2013 peak. Despite the recent decline in annual investment, several of the leading organizations that publish forecasts of how the world can meet climate targets estimate a doubling or near-doubling of global hydropower capacity by 2050. For example, IRENA (2021) projects that to meet the 1.5°C target, the world will need approximately 2,500,000 MW of hydropower by 2050 and IEA (2021) projects 2,599,000 MW of hydropower by 2050 will be required to meet that target. From today’s total of 1,230,000 MW of global capacity, achieving that projected capacity in 2050 would require annual capacity additions of approximately 45,000 MW (e.g., annual investment would need to rapidly return to the level of the 2013 peak and then hold there for 30 years; Figure 1). Asia is projected to see the largest growth, with more than half of the projected global capacity growth, while Africa is projected to have the highest percentage increase, with capacity anticipated to nearly triple by 2050 (Opperman et al., 2017).
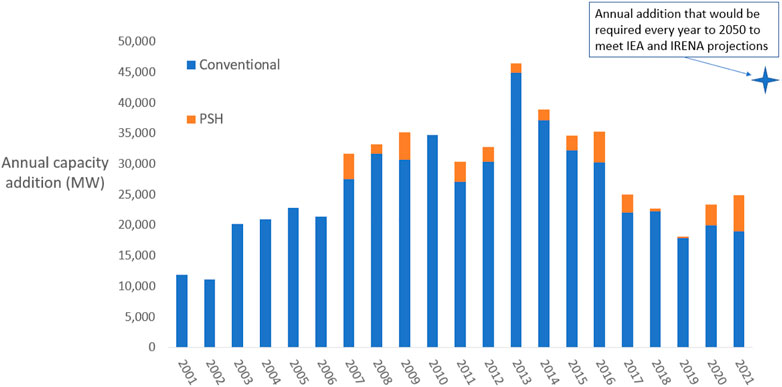
FIGURE 1. Annual global capacity additions for conventional hydropower (blue) and pumped storage hydropower (orange) for the past two decades (data from IRENA, 2022). Note that PSH data were not available before 2007. The blue star in the upper right indicates the level of annual addition that would need to be reached, and then sustained for 30 years, to achieve the forecasts from IEA (2021) and IRENA (2021) for 2050.
2.1 Risks associated with hydropower development and operation
Although hydropower is a major generation source and can support low-carbon power systems, hydropower development and operation can contribute to a range of negative impacts on people and river resources. Collectively, these potential negative impacts represent a set of interacting risks that decision makers pursuing sustainable power systems will need to address. A thorough review of the risks to ecosystems and human communities from hydropower development and management is beyond the scope of this paper, but we do provide a review, with extensive references, in the Supplementary Material. In brief, these impacts include displacement of communities and the loss of agricultural lands, conversion of flowing river habitats to reservoirs, barriers to migration of fish and other aquatic species, alterations in downstream flow patterns and water quality, and the capture of sediment within reservoirs leading to downstream changes in channel morphology and accelerated erosion of deltas (Ligon et al., 1995; Collier et al., 1996; World Commission on Dams, 2000; Thayer Scudder, 2005). In this section we examine how these environmental and social risks contribute to a set of financial risks, such as delays and cost overruns and regulatory uncertainty, that negatively impact developers and investors. We then describe how all of these risks translate into economic risks for governments who seek to achieve a broad set of objectives for their people. These economic risks arise when social and environmental impacts diminish resources important for livelihoods, food, and other resources valued by people and when financial risks constrain the efficient expansion of power systems.
The impacts to environmental and social resources can translate into controversy and social conflict over both proposed and existing dams. In turn, social conflict can result in reputational and regulatory risks for dam owners and developers and contribute to delays and cost overruns for dams or even cancellations. Studies have found that hydropower projects have more delays and cost overruns than other large infrastructure projects (Ansar et al., 2014). Sovacool et al. (2014) found that hydropower projects had a mean cost escalation of 71 percent and that cost overruns affected 75 percent of projects. They reported that among various project types—including solar, wind, nuclear and thermal energy projects—hydropower projects had the longest average construction period (118 months), longest time overrun (43 months) and largest total cost overrun (median of US$100 million per project). Similarly, a study by the consulting firm EY (2016) reported that most hydropower projects (80 percent) experienced cost overruns with an average overrun of 60 percent. Both of these proportions were the highest among the types of large infrastructure projects they surveyed, including gas, coal, and nuclear power plants, water treatment facilities, and offshore wind projects (Figure 2). They also reported that 60 percent of hydropower projects experienced delays with an average delay of nearly three years—among the highest among the categories of infrastructure projects (coal projects had slightly longer average delays).
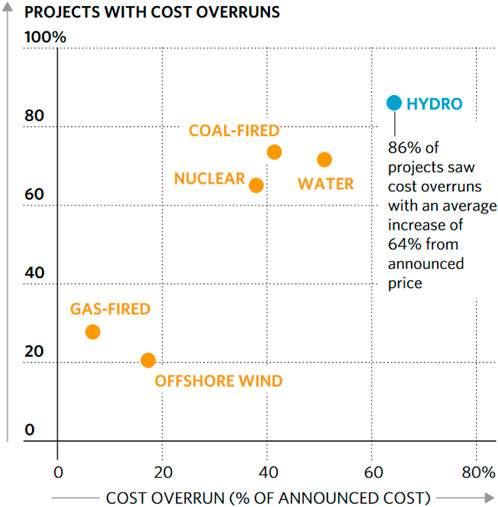
FIGURE 2. Proportion of projects with cost overruns and average cost overrun among categories of large infrastructure and energy projects. “Water” refers to water treatment plants (Figure used with permission from Opperman et al. (2017), adapted from EY (2016)).
These studies do not categorize the reasons for delay and, beyond environmental and social issues, hydropower projects do have a range of site-specific risks and uncertainties, such as geotechnical challenges, that can lead to delays and cost overruns. However, hydropower projects, particularly large ones, can cause relatively large negative impacts on ecosystems and communities (e.g., other renewable technologies generally do not have the issues of displacement of communities that large hydropower projects can have), and some projects’ delays and/or suspensions have been directly attributed to these risks and impacts. In the past decade, several major projects were suspended or cancelled, including HidroAysén in Chile (2.75 GW; US$320 million invested), Myitsone in Myanmar (6 GW; suspended after US$800 million had been invested), and São Luiz do Tapajós in Brazil (8 GW, US$150 million invested), along with several projects in India, including the 780 MW Nyamjang Chu project which was suspended by the National Green Tribunal based on potential impacts to the black-necked crane (Opperman et al., 2017). Social and environmental impacts were major factors in all of these decisions.
While the cancellation or suspension of a project can avoid major social and environmental negative impacts, from the perspective of developers and investors it would clearly be preferable to have had earlier decisions about the acceptability of the project, prior to the outlay of “sunk investment” costs. Similarly, project cancellations can cause economic disruptions and losses for a country that was counting on the cancelled project to help deliver power in the future. The cancellation can lead to short-term higher power costs and the economic costs of “unserved power” that reduces other investment and economic activity; future investors may require a higher “risk premium” for investment in major projects. Thus, from the perspective of achieving national economic goals for power system development, earlier decisions, arising through strategic system-scale planning, could avoid the economic losses and disruptions of project cancellation.
A 2011 special report on renewable technologies from the IPCC concluded that “various environmental and social concerns [represent] perhaps the largest challenges to continued deployment [of hydropower], if not carefully managed” (Kumar et al., 2011). Thus, it is likely that a range of environmental and social impacts are contributing to hydropower’s delivery and cost challenges and that improved management of these risks (from avoidance to mitigation) could not only improve sustainability performance but also improve hydropower from an investment perspective (e.g., reducing uncertainty, improving return on investment) and an economic perspective (efficient delivery of energy objectives balanced with other economic and social objectives). These hypotheses are examined in more detail in Section 4.
3 Managing risks in hydropower from project to system scales
The environmental and social risks from hydropower can be addressed at scales ranging from the project to sector-scale power system planning.
3.1 Managing environmental and social risks at the project scale
A range of tools and methods have been developed and applied to manage environmental and social risks from hydropower at the project scale. As observed by Ledec and Quintero (2003), site selection is the most important step for addressing an individual hydropower dam’s impacts, but we will cover site selection in the following section on strategic planning. Here we describe ways to reduce negative impacts or manage ecosystem functions and services through water quality management, fish passage, sediment passage and environmental flows at the scale of a single dam. Note that both the design (e.g., the presence of a fish ladder) and operations of dams (e.g., the release of “attraction flows” to increase fish use of a passage structure) can be involved in these strategies—and that the ability to carry out some types of operations depends on the physical infrastructure of the dam—and thus dam design is also crucial for dam operations for environmental mitigation.
Dams can negatively impact downstream water quality, such as when they release water with low dissolved oxygen or temperatures outside of historic ranges for a river. Dissolved oxygen can be managed through technologies such as aerating turbines. Some aspects of water quality can be managed through selection of the depth in the reservoir that releases are drawn from, such as a multi-level outlet structures that allow dam operators to manage water temperature of the flows that are released (Cassidy, 2018). Dams with sluice gates built into the bottom of dam are capable of passing some sediment, which will generally require a drawing down of the reservoir to generate sufficient water velocities to mobilize and transport the sediment that has built up behind the dam (Kondolf et al., 2014).
Fish passage structures are intended to reduce negative impacts from dams on fish movement and migration. Structures become ineffective above a certain height of dam and, for these larger structures, some managers have tried assisted migration (e.g., “trap and truck” methods) that capture fish at the base of a dam and transport them over to a location upstream of the dam. Both trucks and barges have been used to transport juvenile fish back downstream, such as for salmon in the Columbia River system (Washington state, United States).
Most examples of fish passage structures—and thus most research on the efficacy of those structures—have come from temperate rivers, often where strong-swimming and leaping salmonids are the primary target fish. Relatively limited applications and research has come from tropical rivers which, in contrast to temperate rivers, often have a much wider range of fish species that migrate. In contrast to rivers in North America or Europe, which may have less than 10 migratory species with similar swimming behavior, tropical rivers, such as the Amazon or Mekong, can have hundreds of species with a broad range of swimming behavior (e.g., those that tend to swim on the surface vs the bottom, those able to leap and those not able to leap) with sizes that range from a few centimeters up to the Mekong giant catfish (400 kg).
A review of the efficacy of fish passage structures reported an average upstream passage efficiency of 62% for salmonids and 21% for non-salmonids, although there were extremely limited studies from rivers such as the Mekong available for the review (Noonan et al., 2012). Rivers often have multiple dams in sequence; with an average passage efficiency of 21%, even just 2 dams will reduce by 96% the proportion of the original population size of migratory fish that are able to reach an upstream habitat. Although Noonan et al. (2012) found that downstream passage efficiency at a dam was generally higher than upstream passage efficiency, fish must first move downstream through the reservoir to reach the dam. The downstream movement of larval fish or eggs requires a current and so dams with long reservoirs with low velocity water can be an impassable barrier (Pompeu et al., 2012). Juvenile fish are often inefficient swimmers and so long reservoirs can also be a barrier to the downstream movement of juveniles. Further, reservoirs can often host native or non-native piscivorous fish that prey on native fish, further illustrating that, in addition to the dams themselves, reservoirs can function as barriers (Pelicice et al., 2015).
Dams can release environmental flows to address some of the impacts from flow alteration. There is now extensive guidance on methods to prescribe environmental flows to promote social and environmental objectives (Tharme, 2003; Opperman et al., 2018; World Bank Group, 2018; Hartmann, 2020) along with case studies, reports and research papers on the results of environmental flow implementation (Harwood, 2017). For example, on the Skagit River (Washington, United States), managers of a hydropower dam adjusted flow releases to improve spawning habitat for salmon, resulting in a significant increase in successful spawning (Connor and Pflug, 2004). The Three Gorges Dam on China’s Yangtze River has also implemented environmental flows to improve fish spawning. Beginning in 2011, managers have released a managed flood pulse in late spring to mimic conditions that trigger spawning in native carp species (Cheng et al., 2018). Beyond fish, environmental flows can be targeted to promoting social and economic objectives such as food production and livelihoods. For example, a managed flood release from the Manantali Dam on the Senegal River was implemented to partially restore the productivity, and associated livelihoods, of the river’s delta in Senegal, which had declined due to dam operations that had greatly reduced the annual flood pulse (Hamerlynck and Duvail, 2003).
Many of the issues summarized above, including sediment, flow regime, reservoir emissions and displacement, have corresponding criteria among the 12 “hydropower performance requirements” in the Hydropower Sustainability Standard (Hydropower Sustainability Secretariat, 2021). Through its “San Jose Declaration,” the IHA has required that its members, which include hydropower developers and operators, apply these standards (International Hydropower Association, 2021). The performance requirements apply primarily to single projects, however there are criteria that a project meet a “demonstrated need and strategic fit” for specific water and energy services. In theory, meeting these criteria would compel proponents of an individual dam to perform some level of system-scale assessment to show that the project is the best way to provide electricity or other services within the context of the power system to which it will contribute. A requirement that a project validate its “demonstrated need and strategic fit” evokes the recommendation of the World Commission on Dams (2000) that decisions about dam development take place within a Needs Assessment to determine if a dam is the best way to meet an energy or water-management need. If pursued in a comprehensive manner, the requirement for demonstrated need and strategic fit, or a Needs Assessment, links the project scale with the system scale, potentially helping to address the limitations of project-scale management of hydropower risks, discussed in the next section.
3.1.1 Limitations of project-scale management of hydropower risks
The project-scale actions described above can mitigate some of the negative environmental and social impacts from dams, although due to a range of constraints, for many impacts this mitigation is only partial. Further, these actions are often expensive and can be difficult or impossible to implement due to cost and/or physical constraints. For example, many of the actions require specific design features, such as fish passage structures, sluice gates for sediment passage, or multi-level outlets for managing water temperatures. If not included in the original design of a dam, these structures can be extremely expensive and cost prohibitive in most situations. A multi-level outlet structure added to the Shasta Dam on the Sacramento River (California, United States) to improve temperatures for salmon below the dam cost 80 million USD.
Fish passage and sediment passage both become more difficult with increasing height of a dam and increasing length of a reservoir. Larger grain sizes of sediment (e.g., coarse sand, gravel and cobble) will deposit at the head of a long reservoir, tens of kilometers away from the dam and a downstream reach of flowing water. Passing those sediment sizes through a long reservoir can only be accomplished with extremely expensive solutions such as dredging and barging. In some systems, managers have added large sediment sizes (e.g., gravels of the size used by salmon for spawning) below dams to replace the sediment trapped in the upstream reservoir, but this requires ongoing management (e.g., replenishment of sediment as it is transported by flows) (Kondolf et al., 2014). Fish passage also becomes extremely difficult and/or expensive as dam height increases and, even with lower dams, fish passage structures have very low effectiveness for some commercially important species (Noonan et al., 2012). Even successful upstream passage at a dam comes with limitations, such as the prevalence of predators in a reservoir—to the extent that some reservoirs can be considered an ecological “trap” for migratory fish (Pelicice and Agostinho, 2008).
Design and operational constraints can also limit the effectiveness of environmental flows or the range of flow releases that are possible. The size of outlets can limit the ability to release an ecologically important flow level, such as the discharge needed to inundate downstream floodplain wetlands. Further, a dam’s economic purposes can also constrain some types of flows, such as when a needed magnitude or duration of flow would result in too much water being “spilled”—bypassing turbines and reducing generation and revenue for a dam operator.
Three key conclusions can be drawn based on these various limitations to mitigating impacts at the scale of an individual dam:
• A dam’s design is crucial to the ability to pursue environmental and social objectives, such as whether it was designed and built to include fish passage, sediment passage, and multi-level outlets for temperature management. Turbine design can influence the economic feasibility of releasing some environmental flows because certain choices of turbine design (e.g., a range of turbine sizes) may be able to generate power over a wider range of discharges, providing greater flexibility for environmental flow management and potentially reduced associated losses to generation and revenue (Balc˘iūnas and Ždankus, 2007; Garrett et al., 2023). Oversizing outlet capacity can also provide greater management flexibility for releasing a range of flow levels. These design solutions will generally be far more affordable to incorporate during original design than through retrofitting.
• The location of a dam will generally be the single most important decision in terms of how it will impact social and environmental resources. In a World Bank report, Ledec and Quintero (2003) emphasized that a comprehensive process for site selection is by far the best “mitigation” strategy during dam development: minimizing or avoiding negative impacts through careful site selection—such as avoiding sites that would have high impacts on migratory fish or sediment transport—could potentially diminish the need for accomplishing mitigation through design and operation. Effective site selection processes could also direct new storage dams away from locations which are likely to have high levels of greenhouse gas emissions from reservoirs (Almeida et al., 2019), such as where upstream land use will produce high levels of nutrients that could lead to elevated levels of methanogenesis in the reservoir (Deemer et al., 2016).
• The benefits of good site selection for a single dam can be applied, and scaled up, through system-scale planning for hydropower. System-scale planning can also overcome limitations inherent to project-level planning and design, including its limited ability to quantify or address cumulative impacts and failure to account for system-level synergies. The remainder of this paper focuses on system-scale approaches and examines the hypotheses that: (1) for planning and/or management, system-scale approaches offer the greatest potential for balancing energy benefits with social and environmental resources; and (2) beyond improved outcomes for social and environmental resources, system-scale approaches can reduce risks for the key actors that generally control decision making in the hydropower sector: governments, developers and investors.
3.2 Hydropower system design
The limitations of mitigating environmental impacts through project-scale approaches (see Section 3.1.1) along with the recognition that site selection is generally the most important decision that will influence the social and environmental performance of hydropower (Ledec and Quintero, 2003) has led conservation organizations to recommend that hydropower be pursued through system-scale planning. For example, The Nature Conservancy has developed a system-scale approach to hydropower planning and management called Hydropower by Design (HbD; Opperman et al., 2015; Opperman et al., 2017), with support from partners including the Inter-American Development Bank (Hartmann et al., 2013) and with current applications in Mexico. WWF and partners have explored system-scale hydropower planning for Nepal, funded by the U.S. Agency for International Development (WWF and DAI Global, 2021) and the Mekong region, in collaboration with the Mekong River Commission and the Asian Development Bank (Mekong River Commission et al., 2016). In parallel, researchers were working on similar ideas with a focus on analytical methods (Hurford and Harou, 2014; Hurford et al., 2014) and also working with organizations such as the World Bank (Karki, 2015). In this section we review the potential for system-scale approaches to identify options for development or management of hydropower systems that potentially provide for a broader range of benefits than could be achieved through project-by-project decision making, development and management.
Basin- or system-scale planning for hydropower and other water-management infrastructure is not a new concept; in the case of the Tennessee Valley Authority (TVA), it is at least a century old (Boccaletti, 2021) and hydropower has been planned at the basin scale in Brazil for six decades. These examples of system-scale planning have generally focused on either maximizing the hydropower capacity and/or generation available from a basin and, to the extent planning objectives were more comprehensive, they sought to optimize across a range of traditional water-management sectors and services derived from river regulation (e.g. hydropower and flood management). A primary distinction between these approaches and HbD is that HbD strives to also include a more diverse range of social and environmental benefits derived from river ecosystems (e.g., the ecosystem services derived from free-flowing rivers).
In this paper we use the term ‘Hydropower by Design’ (HbD), or more generically “hydropower system design,” as shorthand for the recent proposals from NGOs and academics for strategic and system-scale approaches to hydropower planning and management that more fully account for the environmental and social benefits of river ecosystems. Note that with the term ‘hydropower system design’ we mean approaches to planning and management that are multi-project and multi-objective, encompassing resources from both river regulation and river ecosystems.
In most regions undergoing hydropower development currently, decisions about dams, such as siting, are made at the scale of single projects rather than through strategic planning processes (Hartmann et al., 2013; Almeida et al., 2022b). Similarly, environmental review, such as through an Environmental Impact Assessment (EIA), also typically takes place at the scale of an individual dam and often is triggered after many key decisions, such as location, have already been made. Further, Sadler et al. (2000) reported that EIAs rarely result in the rejection of a project because they often occur after considerable investment and political momentum have already occurred. Thus, the EIA process is generally ineffective at influencing the single most important characteristic of a dam in terms of environmental impact—its location (Ledec and Quintero, 2003)—and, instead, the review process generally results in minor modifications and a set of mitigation requirements. Based on these limitation of EIAs, the World Commission on Dams (2000) recommended that project review should occur sufficiently early so that it could realistically reject or relocate inappropriate projects. Proposals for HbD and similar approaches—which emphasize decision processes to inform site selection at an early stage, with explicit recognition of cumulative impacts—can be seen as responses to the limitations of project-by-project dam planning and review diagnosed by the World Commission on Dams.
Recommendations for HbD, or hydropower system design, focus not only on addressing limitations of environmental review processes to better manage negative impacts at a system scale, they also emphasize that system-scale approaches can identify overlooked opportunities to achieve multiple benefits (Opperman et al., 2017; Hurford et al., 2020b). These include opportunities to achieve better social and environmental outcomes for any given level of hydropower development—but also opportunities to achieve a broader range of benefits arising from water infrastructure development and regulation, such as hydropower and flood management (i.e., the motivation for traditional hydropower system planning such as that of the TVA).
Almeida et al. (2022b) provides a comprehensive review of the most recent proposals for hydropower system design, describing four basic steps:
1) Characterize and quantify social, environmental, and economic values that will serve as performance metrics (e.g., fisheries, livelihoods and hydropower generation);
2) Generate a set of options or dam portfolios (ranging from a few to potentially millions);
3) Quantify the performance of various portfolios against those metrics of social, environmental and economic values;
4) Identify portfolios that perform well across a range of values, meet objectives, and/or have acceptable tradeoffs.
Almeida et al. (2022b) recommend stakeholder participation throughout those steps and Opperman et al. (2017) describe a system for stakeholder engagement throughout a HbD process. Stakeholder participation is particularly important for the process of defining the social, environmental and economic values to measure and track (in terms of how different portfolios perform; Step 1) and it can be useful to define measures in ways that are easily understandable to stakeholders and decision makers (The Nature Conservancy and WWF, 2016).
The number of options or portfolios to generate (Step 2) will vary based on the geographic extent of the area under consideration and the objectives of the process. A small area may include only a few projects and it will be possible to identify all potential portfolios (i.e., all potential combinations of those projects). When considering a larger area, with many potential dams, the number of combinations can become enormous (if projects are not mutually exclusive, the number grows factorially and can be calculated as 2n with n representing the number of dams under consideration). For comparison, The Nature Conservancy and WWF (2016) explored the potential for a system-scale approach to improve outcomes from hydropower development for the Myitnge River basin, a tributary to the Irrawaddy River in Myanmar, with four potential dams, or 16 potential combinations or portfolios of dams. Flecker et al. (2022) focused on the Amazon River basin with 509 total dams (including 351 proposed dams), representing 10153 potential combinations or portfolios. To reduce computational complexity when working in an area with a large number of potential dams, it may be useful to only include those dams considered likely to be built by planners and decision makers, or only those dams most relevant to system performance (e.g., above a threshold of capacity or impacts; Schmitt et al., 2018), which may also increase the utility of results to stakeholders and decision makers.
Steps 3 and 4 from the list above are a multi-objective optimization problem (sensu Hurford and Harou, 2014; Hurford et al., 2014). Quantifying the performance of a very large number of portfolios (Step 3) can be accomplished through a genetic algorithm that can explore optimal space (e.g., a Pareto frontier) without generating all possible combinations of portfolios to identify a set of near-optimal options (Figure 3). Geressu and Harou (2015) showed how this approach can consider conjunctively new hydropower dams and their operating rules. Flecker et al. (2022) demonstrated that dynamic programming can be used to find dam portfolios that are guaranteed to be Pareto optimal without the need to evaluate all impacts and benefits for all portfolios (10153 in their study). When more complex, non-linear, models are needed to evaluate impacts of a dam portfolio, genetic algorithms or similar heuristics can find portfolios that are likely Pareto optimal (but without a full analytical proof) (Schmitt et al., 2019).
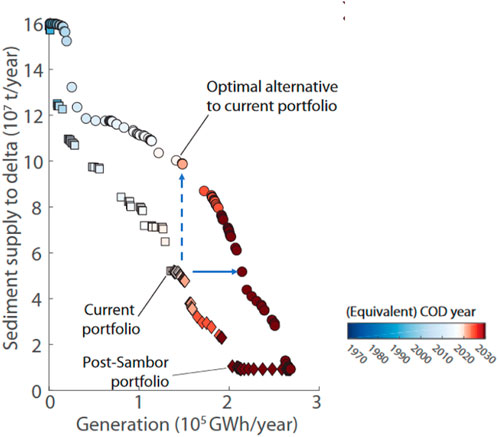
FIGURE 3. A tradeoff plot for sediment supply in the Mekong River (y axis) and hydropower generation within the Mekong River basin (x axis) for many different dam portfolios. The circles show Pareto optimal portfolios, resulting in the optimal trade-off between those conflicting objectives. The squares show how site-by-site additions of dams led to the “current portfolio.” The current portfolio is Pareto dominated, meaning that an alternative dam portfolio could have achieved the same level of hydropower generation with considerably better environmental performance in terms of sediment supply to the delta (e.g., moving from “current portfolio” which corresponds to approximately 5 × 107 tons/year up to “optimal alternative” with approximately twice as much sediment for the same total generation; dashed vertical arrow). The diamonds show the current planned sequence of dams which will continue to depart from the Pareto optimal frontier. However, hydropower capacity sufficient to generate an additional 100 TWh/year could be developed with no further impact on sediment to the delta (e.g., by building dams upstream of existing dams and avoiding building dams on tributaries that still contribute sediment); see the solid horizontal arrow that moves from the current portfolio to a portfolio on the Pareto Frontier. Figure used with permission from Schmitt et al. (2019); in legend, COD refers to “commercial operation date.”
To assess how different portfolios perform and to visualize tradeoffs, results can be presented as a tradeoff plot (Figure 3). Because system-planning processes often track a range of measures to represent diverse resources and values, plots that can show how portfolios perform across multiple variables can be useful, such as a parallel axis plot (e.g., see Geressu and Harou, 2015 and Figure 4).
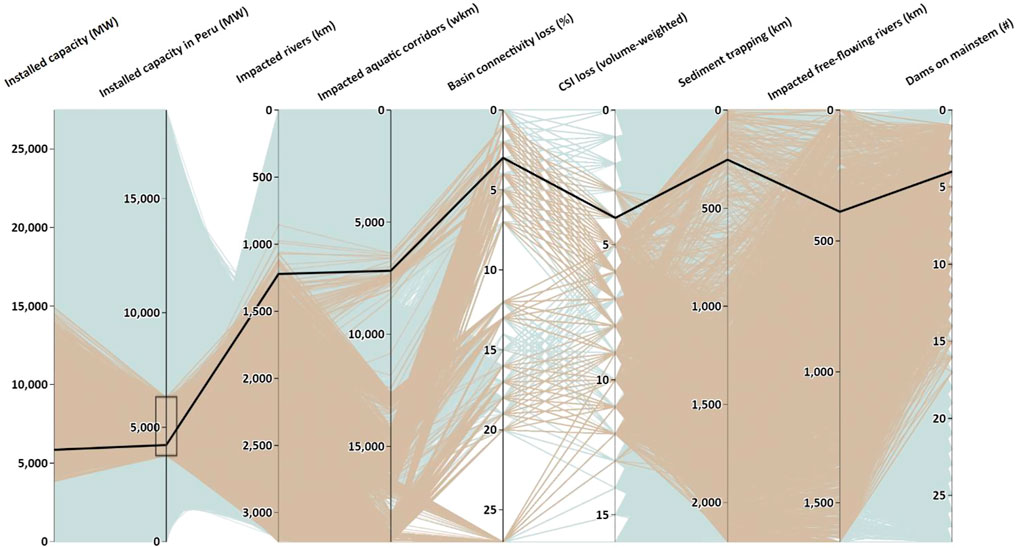
FIGURE 4. A parallel axis plot showing how a large number of dam portfolios perform across a range of metrics (from a large basin in Peru with unpublished data, used here to illustrate the utility of this type of figure). Each portfolio is represented by a gray path that crosses the axes, with one axis per metric (in most of the figure, the high number of gray lines gives the appearance of gray shading). Where each path crosses an axis depicts the corresponding portfolio’s performance against that metric. For each metric, the “desired” performance is at the top of the axis (e.g., for negative impacts, such as “impacted kilometers,” zero is at the top of the axis; for positive outcomes, such as capacity, zero is at the bottom). Here, a single portfolio is selected and shown as a black line. This plot is from a decision support tool that allows users to select ranges for a metric, here indicated by a square along the axis for installed capacity. All portfolios that fall within that range are shown in brown. By selecting ranges across multiple axes, users can quickly filter through tens of thousands of portfolios to find the sub-set that fulfills the selected ranges.
These types of plots, combined with other quantitative results, can be used within stakeholder and dialogue processes to identify those options that effectively accomplish a range of objectives (Step 4). For example, in a partially developed basin, a system-scale process could identify a portfolio of dams that would minimize additional losses of sediment by selecting dams that are upstream of existing dams and avoiding new dams on tributaries that still contribute sediment (Figure 3). In theory, the portfolios identified through this process can be translated into a set of options for decision makers. This approach can also be used to identify individual projects that are rarely or never part of portfolios that perform well across multiple objectives, helping to screen out potentially problematic projects. Opperman et al. (2017) review how the results of HbD processes can be integrated into planning, project identification and approval, licensing, and investment decisions. These approaches can also be used to improve planning under uncertainty, such as ensuring that planners can select portfolios of dams that will be robust to climate change (Hurford et al., 2020a).
Assessing tradeoffs across multiple objectives can allow system-scale guidance to move beyond rules of thumb and toward recommendations that are system specific. The models used to examine tradeoffs and options do require data, and high-resolution data may not be available for all objectives. However, system-scale analyses are generally not intended to find the “right” answer but rather to identify a set of options that may merit further study. In their study on hydropower development options in the Myitnge River basin, Myanmar, The Nature Conservancy and WWF (2016) showed how, for the purposes of identifying illustrative options, globally available data could be used as preliminary placeholders within multi-objective tradeoff models. These models can also seek to use the same datasets that are being used by government agencies and multilateral financial institutions to plan hydropower, as was done in a WWF-led study of hydropower options and tradeoffs for Nepal (WWF and DAI Global, 2021).
Although examples of hydropower system design can increasingly be found in research (Ziv et al., 2012; Hurford et al., 2014; Schmitt et al., 2018; Almeida et al., 2019; Flecker et al., 2022) and applications with NGO participation (Opperman J. J. et al., 2015; The Nature Conservancy and WWF, 2016; WWF and DAI Global, 2021), decision making for hydropower still rarely is based on system planning (Hartmann et al., 2013; Almeida et al., 2022b), though some examples exist (Karki, 2015). This lack of uptake may be partly explained by the trend that research on system-scale planning often emphasizes the potential for better outcomes for environmental resources and communities (as reviewed in Section 3.2.1 below) as opposed to better outcomes for those parties that are most influential for decisions on reviews, licenses and the flow of money toward projects, such as investors, developers, and government agencies. Section 4 of this paper focuses on the potential benefits to those actors.
3.2.1 Hypothesis: Hydropower system design can produce more balanced outcomes across multiple social, economic and environmental objectives
There is both empirical and analytical support for the hypothesis that hydropower system design can produce more balanced outcomes from hydropower development and management. For example, a range of studies have shown that, for a given level of hydropower development, a system-scale approach to hydropower project selection could have produced considerably improved environmental outcomes for riverine resources than what was produced through existing decision processes, which were project-by-project (Figures 3, 5).
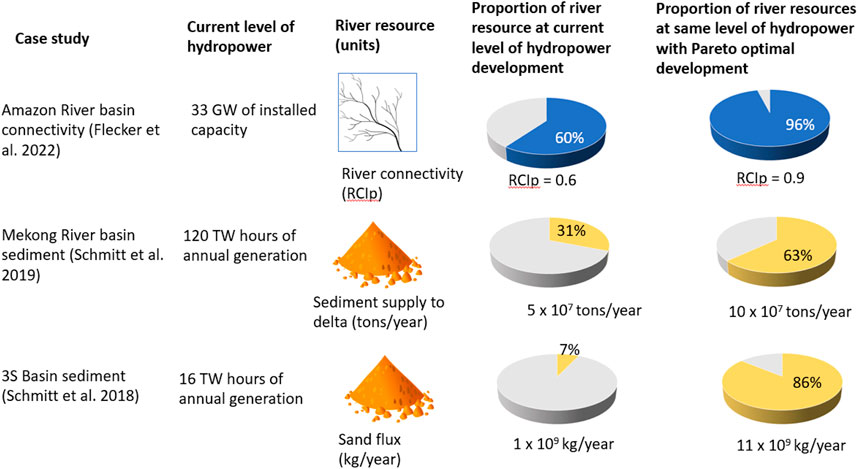
FIGURE 5. Comparing outcomes for river resources for equivalent levels of hydropower development between the actual current level of development and the environmental outcome that could have been achieved through a hydropower system design that identified Pareto optimal options. For reference, the difference between current outcomes and that which would be possible from Pareto optimal selection for sediment in the Mekong basin (dashed vertical line from Figure 3) is shown in the middle row.
For example, in the Amazon, the current level of hydropower capacity has reduced basin-scale connectivity by 40% from its original level (as measured by a dendritic river connectivity index that quantifies drainage network fragmentation (RCIp; Flecker et al., 2022)). These existing projects were not developed through any sort of comprehensive system-scale process. Had they been developed through a system-scale process that sought to optimize connectivity and hydropower capacity, the same level of capacity could have been developed with just a 4% reduction in basin-wide connectivity (Figure 5). Similarly, Schmitt et al. (2018) found that the current set of hydropower dams in the 3S basin (three main tributaries to the Mekong River) generates 16,000 GWh/year and has reduced the basin’s output of sand by 91%; a system-scale approach to site selection that identified a Pareto-optimal development option that balanced hydropower generation and sand supply could have achieved the same generation for only a 15% reduction in sand (Figure 5). Considering the entire Mekong basin, Schmitt et al. (2019) found that the current portfolio of hydropower development has reduced sediment supply to the agriculturally crucial delta by 70%; a system-scale approach could have identified a portfolio that produced equal generation but maintained twice as much sediment (Figures 3, 5). In all three cases, the standard pattern of development produced results that were far from optimal. The more balanced outcomes, identified through the various analyses, could only have been developed by seeking efficient (optimized) outcomes at the basin-scale; river basin development that proceeds project-by-project could not realistically achieve such improved outcomes.
These same studies have modeled potential future expansion of hydropower in those basins and also found that a system-scale approach could identify much more balanced outcomes than would be likely with a project-by-project approach. For example, Schmitt et al. (2019) found that a system-scale approach could identify portfolios that increase hydropower generation from the Mekong basin by 70% with almost no further impact on sediment supply (see horizontal arrow in Figure 3), whereas the planned sequence of dams, planned and developed as individual projects, would reduce today’s already depleted sediment level by an additional 80% (leaving the basin with only 6% of its original supply of sediment).
In addition to river basins under development, a system-scale approach is also capable of producing more balanced outcomes when applied to the “re-optimization” of a mature hydropower basin (i.e., a basin that will not have new hydropower dams developed), demonstrated by the Penobscot River in Maine (United States). Non-federal hydropower dams in the United States are periodically required to go through a process to receive a new license, referred to as “relicensing.” During earlier relicensing processes for various hydropower dams in the Penobscot, Tribal governments, conservation organizations, and state and federal resource agencies sought to improve fish passage for the basin’s 12 migratory fish species, including Atlantic salmon, Atlantic shad, and river herring. However, these single-project relicensing processes had generally failed to resolve conflicts between migratory fish and hydropower dams. An opportunity arose to relicense seven of the basin’s primary hydropower dams at one time. In 2005, stakeholders, agencies and the dams’ owner came to a settlement agreement that focused on the dams as a system. The agreement included the sale of three dams to a newly formed Penobscot Restoration Trust (composed of the Penobscot Tribe and several conservation organizations). The Trust then raised funds and removed two of the dams and built a nature-like fish passage channel around a third. The owner of the other dams received new licenses and permission to change equipment and operations at remaining dams. Due to these changes, the total generation from dams in the basin will somewhat increase compared to pre-project conditions while, due to dam removal (and improved fish passage at remaining dams, required as a license condition) approximately 1,000 km of additional habitat will be available for migratory fish. River herring using the basin for spawning have already increased from a few thousand to a few million fish (Opperman et al., 2011; Opperman et al., 2017).
The outcomes in the Penobscot basin—dam removal to improve fish habitat coupled with changes at other dams to increase basin-scale generation—could only have occurred through an approach that included multiple projects together. Outcomes of these magnitudes would have been highly unlikely or impossible through a sequence of individual project relicensing processes.
Thus far, we have primarily focused on the potential for system-scale approaches to produce more balanced outcomes between hydropower and the social and environmental resources derived from river ecosystems. However, a system-scale approach to planning and management of hydropower and other infrastructure can also identify portfolios that produce more balanced outcomes across various traditional water-management purposes (Lee et al., 2009; Jeuland et al., 2014; Opperman et al., 2017), explored further in Section 4.2. The re-optimization of mature basins could also include analyses for how existing hydropower systems could be reoperated to facilitate greater expansion of variable sources such as wind and solar PV.
Thus, in both developing and already developed basins, there is strong support for the hypothesis that moving planning and management of hydropower from the project scale to the system scale can produce more balanced outcomes across multiple benefits. Further, Flecker et al. (2022) found that the opportunity for balanced outcomes increases with spatial scale of the planning area.
3.3 Power system planning to balance low-carbon energy with river conservation
Increasing the scale of hydropower planning and management can expand the range of potential options, increasing the opportunity to identify those capable of providing more balanced outcomes relative to status quo planning and management; this section explores the hypothesis that further expansion—from hydropower systems to power systems—can further increase the range of potential options and the ability to find solutions that balance multiple objectives, such as providing low carbon, low cost power while maintaining free-flowing rivers.
The hydropower system design discussed in Section 3.2 has two major limitations. First, the modeling for hydropower system design generally does not consider hydropower dams in the way that energy planners or grid operators view them. The studies described above sought to optimize system-scale river values, such as connectivity or sediment, with system-scale hydropower capacity (MW) or generation (TW hours). However, focusing on capacity or generation oversimplifies how individual hydropower projects perform within power systems -- and thus how projects are selected and valued from an energy perspective. For example, two dams with similar capacities may play different roles in a grid (e.g., if one had storage and the other did not). This simplification of hydropower characteristics limits the utility of the results and recommendations from these hydropower system design applications from the perspectives of energy planners, project investors and developers, and grid operators.
A second limitation is that by using tradeoff analyses where the energy objective is hydropower capacity or generation, the analysis limits the “energy solution” to what hydropower can provide. In actual power systems, capacity and/or generation (or other grid services) can come from a variety of other technologies or solutions (e.g., other renewables, batteries, grid management). This focus on hydropower for meeting energy objectives narrows the range of potential solutions, including the potential for greater protections for river values, limiting the utility of the results and recommendations for river-dependent communities, resource management agencies and conservation organizations.
Ultimately, people use energy, not hydropower. Thus, the goal of achieving multiple objectives simultaneously—for climate, energy, biodiversity conservation and other SDGs—is not likely to be achieved with an analytical or planning focus on a single energy technology, such as hydropower. Instead, the goal is to meet the needs for power of people and economies in ways that do not contribute to climate change, are economically feasible and affordable (and thus politically viable), and that minimize conflicts with other objectives, such as livelihoods, food production, and healthy ecosystems. Conservation organizations have referred to this challenge as the need to identify power systems that are “low carbon, low cost, and low conflict” or “LowCx3” for shorthand (Opperman, 2019), pointing toward broader energy planning processes.
However, typical methods for power system planning, such as capacity expansion modeling, also have a major limitation: they generally do not incorporate diverse environmental and social resources and values into their analyses. Thus, while methods like HbD strive to fully account for those diverse values but do not produce results that are directly usable by energy planners, capacity expansion models produce useful results for energy planners but risk perpetuating processes that first identify projects and then only consider environmental and social values during project-level review—rather than using environmental and social information to guide site selection at early stages of planning.
Integration of more holistic hydropower planning processes, such as HbD, with power system capacity expansion models can contribute to identifying power systems that are LowCx3. However, to date there are few examples of the integration of capacity expansion models with the type of river basin and hydropower models that underpin HbD (Almeida et al., 2022b); below we explore some early applications. We begin with two approaches that achieve some level of integration of hydropower, rivers, and capacity expansion without fully integrating the river basin and power system models.
First, a power systems model can be used to develop a target range of generation from hydropower and then a river systems model can be used to develop an optimized hydropower dam portfolio that meets that target with least impacts on social and environmental resources, such as minimizing capture of sediment or loss of migratory fish habitat. For example, Schmitt et al. (2021) focused on the future power system and large rivers of Myanmar (the Irrawaddy and Salween), a country with Business as Usual (BAU) projections for grid expansion that are dominated by hydropower and, to a lesser extent, coal. They used a simple capacity expansion model (aggregated over the whole country with limited representation of temporal demand dynamics) to develop two alternative least-cost power systems that included targets for additional generation technologies, including wind and solar. The two alternatives resulted in much lower targets for hydropower than the BAU (17%—26% of the BAU target), mostly because they assumed more realistic cost decreases for wind and solar technology then Myanmar’s existing energy master plans. They then used a river systems model and the Borg multi-objective evolutionary algorithm (MOEA) to identify Pareto-optimal options for meeting the hydropower generation target from each scenario while minimizing capture of sediment behind dams. The alternative scenarios were able to avoid any dam construction on the mainstem Irrawaddy and Salween rivers, with dramatically lower loss of sediment relative to the BAU. One reason for that was that, even when optimized, the BAU portfolios required mainstem dams to meet the projected major increase in hydropower demand. The alternative scenarios had much greater diversification in their mixes of generation technology and had lower overall costs (5%—7% lower) than the BAU because of their higher proportion of low-cost wind and solar. Further, the more diverse mix of generation sources will be less vulnerable to disruption during droughts than would be a more hydropower-dominant system, and wind and solar resources are located closer to demand centers and existing power lines, reducing the need for new transmission.
One limitation of this approach is that the screening-level capacity expansion model produces a relatively coarse objective of an overall generation target from the hydropower sector, whereas higher resolution capacity expansion models treat each hydropower project as a distinct power plant, with cost and performance characteristics that are used as criteria in the selection of a least-cost power system. This simplification may result in some loss of precision in terms of the dams selected through the hydropower portfolio analysis and how they would perform, in terms of cost and grid services, within an actual power system. Additional iteration between models could address this limitation.
A second approach for partial integration can be to use a capacity expansion model and compare least-cost energy scenarios with and without various policy constraints, such as policies to protect certain rivers or types of rivers. An unconstrained first run of a capacity expansion model can establish a baseline or reference least-cost power system (e.g., a system selected only to minimize costs). Then a river systems analysis can be used to identify a set of river-defined objectives, ranging from specific objectives such as “avoid dams on River X” or a categorical objective such as “avoid dams on free-flowing rivers” or “avoid dams in national parks.” This type of objective can then be translated into a constraint for a second run of the capacity expansion model by, for example, removing as an option for the model those potential dams that would conflict with the policy constraint. The capacity expansion model is then re-run with these constraints. Because the policy constraint filtered the pool of dams that the model could select (e.g., after filtering, the model is not able to select any dam within a national park), the resulting alternative power system will be the least-cost option given the policy constraint. The options can then be compared in terms of system cost.
This second approach was explored by Opperman et al. (2019). They developed a BAU reference least-cost power system expansion for Chile up to the year 2045, using the SWITCH model. Then, based on an analysis of free-flowing rivers in Chile (sensu Grill et al., 2019), they also developed two least-cost scenarios that included river protections that could be expressed as constraints within the SWITCH model. To be consistent with the challenge of achieving grids that are LowCx3, these river-protection scenarios also avoided new fossil fuels, while the BAU was free to select fossil fuels. The river-protection scenarios included:
1) Basin-constrained scenario (“basin”), which did not allow new hydropower dams within undeveloped basins (i.e., it only allows new projects in basins with current operational projects: approximately 35% of potential hydropower projects remained eligible after this constraint).
2) Free-flowing river-constrained scenario (“FFR”), which did not allow new hydropower dams on rivers defined as free-flowing (approximately 15% of potential hydropower projects remained eligible).
Thus, the basin scenario would allow development of a hydropower dam on a free-flowing river if that river was within a basin that already had dams, while the river scenario protected any river that was classified as free-flowing.
The reference or BAU scenario for Chile included a major expansion of coal generation from 4 GW in the first period to over 17 GW by 2045, while hydropower increased by about 35% by 2045. The two river-protection scenarios included no additional coal and about half as much hydropower. The river-protection scenarios included dramatically more solar PV, representing approximately 2/3 of installed capacity by 2045, relative to the BAU. To account for this higher level of variable renewable generation, the river-protection scenarios include three times as much storage as does the BAU (Figure 6).
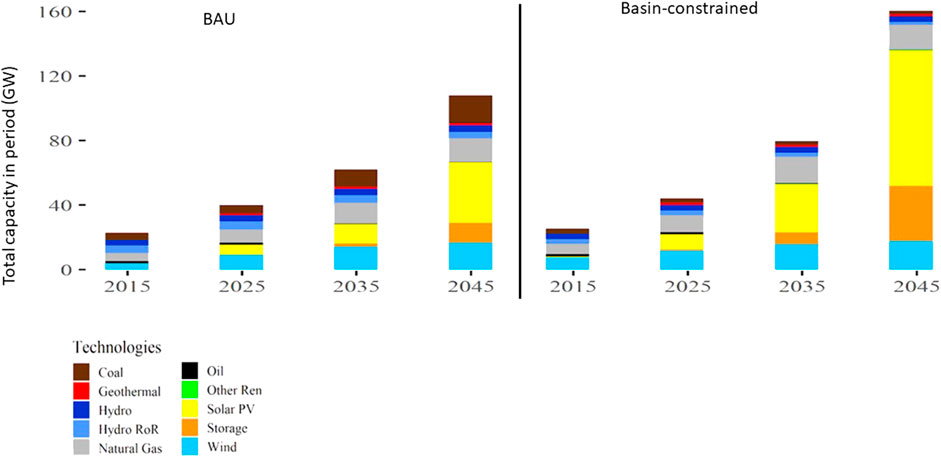
FIGURE 6. Installed capacity for Chile, by period and technology, for reference scenario (BAU) and a scenario that avoided new dams in undeveloped basins (basin-constrained scenario). The river-constrained scenario had a very similar pattern of investment as did the basin-constrained scenario. Figure adapted with permission from Opperman et al. (2019)
The river-protection scenarios are much more consistent with a LowCx3 grid than is the BAU, with a carbon intensity and emissions per capita that are approximately ¼ of the BAU. The BAU results in the damming of 58 free-flowing rivers (FFR), with a total of 3,850 km changing from free-flowing to non-free-flowing. The basin scenario resulted in the damming of 14 FFR and 1,960 km while, as intended, the FFR scenario resulted in the loss of no FFR (Figure 7; note, however, that in both scenarios negative impacts will still occur on the non-FFR rivers where new projects are built). In terms of the cost, the three scenarios were nearly equal, with the basin scenario cost being 1.5% more expensive than the BAU and the FFR scenario being 2.1% more expensive.
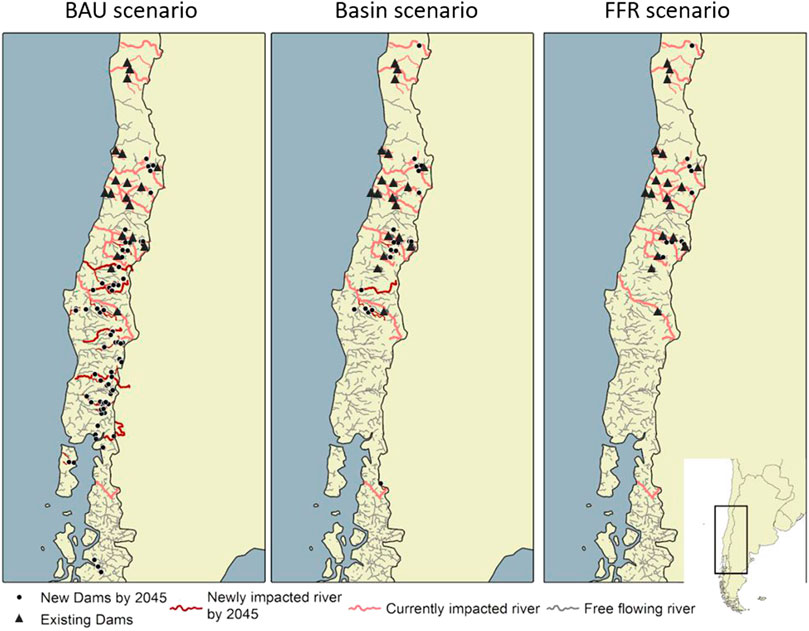
FIGURE 7. Location of dams and free-flowing rivers in Chile under three power system scenarios. Used with permission from Opperman et al. (2019)
The considerably larger expansion of solar-PV in both cases above (Myanmar and Chile) could result in a range of negative impacts from solar development. However, there is a large potential for solar PV on already degraded lands in both Chile and Myanmar (Baruch-Mordo et al., 2019) and careful planning of solar expansion can minimize negative impacts on people and nature (Kiesecker et al., 2010). Solar PV projects can be built in a range of applications with low impacts on environmental and social resources, such as on rooftops, within agricultural settings, or floating on reservoirs (Almeida et al., 2022a). In contrast, hydropower is often constrained to few large projects at sites where dams are technically feasible.
Thus, both approaches above use a sequence of energy systems and river models (e.g., one model is run first and its results are used as inputs to the other model) and so there is no dynamic feedback between the models. Gonzalez et al. (2023) provides an example of an application with fully integrated river basin and power system simulators, such that the spatial and temporal interdependencies of the two systems can be represented when evaluating the expansion and operation of one or both systems. For example, the model is able to ensure that the hydropower generation required by the power system simulator is in fact deliverable given river flows and reservoir storage levels. An optimization algorithm is connected to the integrated water-energy model in order to search for those water and/or energy upgrades and policy changes (e.g., operation rules, regulatory design, etc.) which can best meet one or more criteria. This approach can answer strategic planning questions, such as which specific power generation mix (including locations of generators and transmission lines) enables a portfolio of hydropower dams which cumulatively have acceptable water-resource implications for other water users and the environment? Or, what hydropower release rules will enable power mixes with substantial variable sources such as solar and wind power?
Until recently this approach had only been tried on a simple test case by Gonzalez et al. (2020). More recently, Gonzalez et al. (2023) applied a full water-energy co-design capacity expansion approach to Ghana’s energy system and the Volta River basin. The study uses a multi-objective search approach to identify the most efficient (“Pareto-optimal”) power-water system designs and quantify the trade-offs and synergies in performance that they imply. The case study’s design problem was to meet Ghana’s future energy demands and emission targets by expanding the use of variable renewables (solar PV and wind), bioenergy and new transmission lines, but without expanding conventional (high emission) thermal power sources and without new hydropower dams—only re-operation of dams was allowed. The paper shows that variable renewables could be increased by 38%, but at large cost to other sectors—the seasonality of hydropower releases would decrease irrigated food production while the sub-daily variability of river flows downstream of dams (“hydropeaking”) would increase substantially (by a factor of 22) to enable the grid to accommodate the variability of the new solar and wind generation. However, the study demonstrates that if the whole power system is redesigned to aid in this transition—i.e., the dams do not have to do all the adaptation work—then the energy and emission goals could be achieved at an acceptable cost to the environment and food security. The power system redesign included optimizing where new transmission lines were developed and where new bioenergy, wind and solar generators were placed in the grid. This analysis demonstrates the value of interconnecting the river basin and power system models as only when these systems were co-designed and managed synergistically could multi-sector conflicts be decreased to acceptable levels (see FutureDAMS project website for further information, such as software implementation).
3.3.1 Hypothesis: Planning at the scale of an overall power system has the greatest potential to identify a broader set of options that meet multiple social, economic and environmental objectives
The examples reviewed in the previous section provide support for the hypothesis that, by expanding the range of potential options, planning expanded to whole power systems has greater potential to meet objectives for both low-carbon energy and river conservation compared to planning limited to hydropower systems. For the Myanmar case, meeting a national energy target primarily through hydropower planning—even with a HbD approach to find Pareto-optimal options—would require dams on the mainstem Irrawaddy and Salween (Schmitt et al., 2021), the only remaining long free-flowing rivers in south or southeastern Asia (Grill et al., 2019). Only by expanding the range of available options by including other technologies could a solution be identified that both met national power targets with low-carbon energy and maintained the long free-flowing rivers—at a somewhat lower cost than the hydropower-dominant approach of the BAU. Similarly, Siala et al. (2021) found that promoting greater expansion of solar PV provides the best opportunity to meet power demands in Southeast Asia with low-carbon electricity while avoiding some of the more harmful dams on the mainstem Mekong River. Shirley and Kammen (2015) explored options for meeting 2030 power demand for Sarawak (Malaysia), for which the government was planning to build two coal plants and 12 large hydropower dams. They found that two hydropower dams already under construction plus decentralized generation (solar PV and biomass) could meet future demand at a competitive cost.
By planning at the power system scale with the integration of capacity expansion models and river models, planners are able to successfully avoid the limitations of hydropower system design described above. By using a capacity expansion model, the results are expressed in terms that are used by energy planners and grid operators; a model such as SWITCH identifies individual projects, the sequence of their construction, and system costs. By expanding to other technologies, a broader range of solutions are available, increasing the probability of finding LowCx3 power systems consistent with maintaining a significant amount of undammed rivers valued by river-dependent communities, resource agencies and conservation organizations.
4 Beyond improved environmental and social outcomes, system-scale approaches provide benefits to investors and governments
The previous sections support the hypotheses that system-scale approaches to hydropower planning and management are more likely to find options that perform well across multiple dimensions, and that planning at the scale of whole power systems provides the greatest range of options and thus the greatest likelihood of finding solutions that work well for multiple objectives (e.g., river conservation and power).
Thus far, we have mostly focused on the social and environmental benefits of meeting energy targets in ways consistent with maintaining high value or free-flowing rivers. However, achieving widespread uptake of these approaches will likely require a clear demonstration of associated benefits for a range of key actors and decision makers in the hydropower and energy sectors, including governments, investors and developers. In this section we explore two hypotheses about the potential benefits of system-scale planning approaches for these actors.
4.1 Hypothesis: Hydropower system design can reduce risks and improve returns for investors and developers
In areas where hydropower development is still happening, hydropower system design and analyses can identify portfolios of projects that can be consistent with LowCx3 power systems. Such a system can only become a reality if investment flows toward those specific projects—which in many places means that investors and developers choose to pursue them. In this section we explore how projects that emerge through a hydropower system design process can have improved financial performance for developers and investors through two key pathways: 1) system-design optimization and 2) improved risk management to reduce delays triggered by social and environmental impacts and associated cost overruns.
To explore these sources of financial value, we modeled several alternative approaches for project selection for new hydropower dams in the Magdalena River basin, Colombia. The river, the fifth largest in South America, flows for 1,500 km from the Andes to the Caribbean Sea. At 273,000 km2, its basin spans approximately one-quarter of Colombia’s land area, encompassing a population of 36 million people (75% of Colombia’s population), most (86%) of its GDP and 70% of its hydropower generation. The basin also is home to approximately 140,000 indigenous people, who live primarily within 143 indigenous reserves. The Magdalena basin currently has 35 medium to large hydropower dams, producing approximately 33,000 GWh/year from a total installed capacity of 6,673 MW. Approximately 100 other potential sites in the Magdalena, with an aggregate capacity of 24,000 MW, were identified through a basin study in the 1970s (Study of the Electric Energy Sector; ESEE, 1979).
This analysis of hydropower site selection is offered as a purely illustrative exploration of the hypothesis that system-scale processes have the potential to deliver benefits to developers and investors, alongside improved outcomes for social and environmental resources. The results are not a recommendation or plan for the Magdalena basin. The basin was selected because it had a range of available data that facilitated this type of analysis and not necessarily to reflect current demand for or likelihood of hydropower development.
4.1.1 System design optimization
In many river basins, projects are selected by developers who will generally emphasize relatively short-term financial targets. Projects that emerge from this developer-driven model will not necessarily be those that will work together most effectively as a system. Each project selected and built has the potential to change conditions for subsequent projects, including changes in flow patterns, potentially affecting operations of future projects, and impacts to communities and ecosystems, potentially affecting the social context and mitigation requirements for future projects. Selection of single projects in isolation miss opportunities to identify synergies among groups of projects, such as integrated operations, collective mitigation, and location of roads and transmission lines. A system-scale approach using optimization can identify a set of projects most effective at capturing system-level financial efficiencies. In addition to system-scale benefits, the individual projects selected through this approach can potentially have greater average financial performance than those selected through the BAU approach.
To explore the potential for improvement in project financial performance from system design optimization, we compared two development scenarios for the Magdalena basin: BAU and a system optimization scenario. Both development scenarios used the HERA model, from PSR (2020), and scenario-specific criteria, described below, to 1) select individual projects from among 97 potential dam sites (as catalogued in the 1979 hydropower master plan Study of the Electric Energy Sector) to meet a given generation target and 2) to calculate financial data on those projects. The HERA model is a computational model used to identify potential project sites from various physical and hydrological characteristics of a river basin (for full methods, see Supplementary Material).
• Business as usual—This scenario reflects a developer-driven approach in which projects are sequentially selected based on the Net Present Value (NPV) of each individual project. The BAU was intended to represent how development decisions are currently made in Colombia, with independent developers proposing sites in response to periodic government auctions for power capacity. The BAU algorithm sequentially selects sites based on the highest project-level NPV, reflecting the tendency for developers to prioritize projects based on NPV. Because construction of a project potentially changes the conditions for some or all future sites, the river cascade topology within HERA (e.g., how project location affects flow regime) is updated after each project is constructed. HERA then recalculates NPV for remaining sites and selects the project with the next-highest NPV, continuing until a target generation level is reached.
• System engineering—This scenario selects projects based on financial criteria that incorporated system-scale efficiencies. Rather than selecting individual sites in sequence, maximizing project-level NPV at each step, this scenario maximizes NPV for an overall system that achieves the same generation level as the BAU. This scenario captures system-level efficiencies by considering project interactions (e.g., through alterations of the flow regime) and through a comprehensive assessment of dam design and costs. The system engineering scenario maximizes basin-level economic benefits of hydropower and does not consider environmental or social impacts nor how those impacts affect project-level risks or financial performance.
Due to various system-level efficiencies, the system engineering scenario selected a portfolio of projects with a 9.3 percent greater expected NPV for developers relative to the BAU (defined as revenues minus costs with a discount rate of 9% over 35 years). The median NPV for system engineering (from among simulations developed through a Monte Carlo approach with a sample of 50 cashflows for each scenario) was 6.3 billion USD compared to 5.8 billion USD for the BAU. A related measure, Internal Rate of Return (IRR), was also greater for system engineering (28.5%) compared to BAU (25.1%) (Figure 8 and Table 1). Both results suggest that a portfolio of dams selected through project-level decisions fails to capture a range of system-scale efficiencies, resulting in reduced financial performance. A comprehensive basin planning process is capable of capturing these efficiencies and delivering greater financial performance.
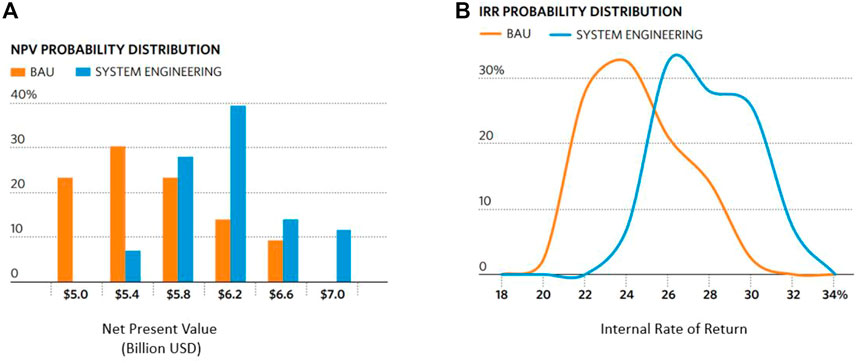
FIGURE 8. Distribution of Net Present Value (NPV); (A) and Internal Rate of Return (IRR); (B) for BAU vs. system engineering scenarios for hydropower development in Colombia’s Magdalena basin. Both figures show values of the aggregated cashflows from all projects in a scenario, with distributions of values reflecting 50 simulations of cashflows for each scenario.
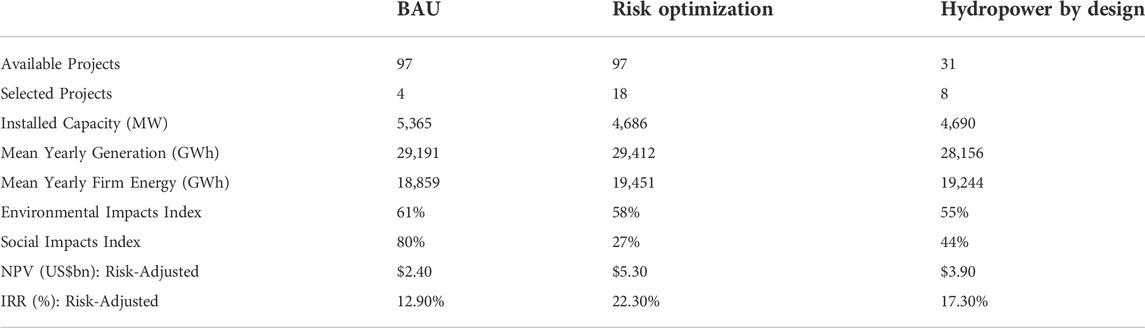
TABLE 1. System-scale performance of the BAU, risk optimization and Hydropower by Design scenarios for hydropower development in the Magdalena basin. Higher percentages on the Environmental and Social Impacts Indices indicate greater negative impacts.
4.1.2 Improved risk management
The second source of potential financial value captures benefits from improved risk management by focusing site selection, and project design, on avoiding or reducing negative impacts on communities and environmental resources. These impacts can trigger social conflicts and/or regulatory actions leading to delays and cost overruns. Because each month of delay leads to increased costs and foregone revenue, delays can reduce a project’s IRR. As discussed previously, environmental and social conflicts are relatively common to hydropower and contribute to the hydropower sector having among the highest rates and levels of both time delays and cost overruns. Hydropower system design can seek a portfolio of projects that minimize social and environmental conflicts, potentially resulting in a portfolio composed of projects less likely to have associated delays and cost overruns, improving the distribution of projects’ IRR relative to those selected through a BAU approach.
To explore the potential benefits to developers and investors of improved risk management, we ran an additional development scenario that combined system optimization with risk optimization and then a Hydropower by Design scenario that sought to ensure specific environmental outcomes.
• System engineering plus risk optimization (“risk optimization”). This scenario selected projects using the system engineering approach, described above, but added consideration of social and environmental and social risks to provide information that could reduce the probability of associated delays and cost overruns. To frame this analysis, we derived a simple relationship between environmental and social impacts and time overruns associated with increased costs. We drew on data from Sovacool et al. (2014) about overall construction cost and time overruns for hydropower projects and then transformed the associated distribution curve based on a factor reflecting the relative contribution of social and environmental and social risks to project delays; for this analysis, we assumed this contribution factor to be 30 percent.
We then assigned an “environmental and social risk score” to each potential hydropower project in the Magdalena that reflected the potential magnitude of time delays (and thus associated cost overruns). The risk scores were based on data collected by The Nature Conservancy in the Magdalena Basin, including a range of environmental, social, demographic and economic variables, such as location of protected areas, sensitive ecosystem zones (such as dry forest), potential displacement of people, and indigenous community territories. The risk score was weighted strongly toward social risks (80%) relative to environmental risks (20%), reflecting that social conflicts are more likely to contribute to project delays than are environmental impacts (note, however, that social conflicts are often triggered by, or are intertwined with, environmental impacts).
Selection of projects in the first two scenarios (BAU and system engineering) were “risk blind” in that a given project’s estimates for NPV and IRR did not reflect potential social and environmental risks. With the risk optimization scenario, we sought to understand how a more comprehensive assessment of risk could affect project financial performance, and how risk-adjusted financial performance estimates would affect project selection. A risk penalty (the environmental and social risk score) was applied to the projects selected by the BAU approach to model the potential for those projects to have environmental and social impacts that could translate into delays and cost overruns. Through this, we developed a set of “risk-adjusted” scores for NPV and IRR for the BAU portfolio (Figure 9).
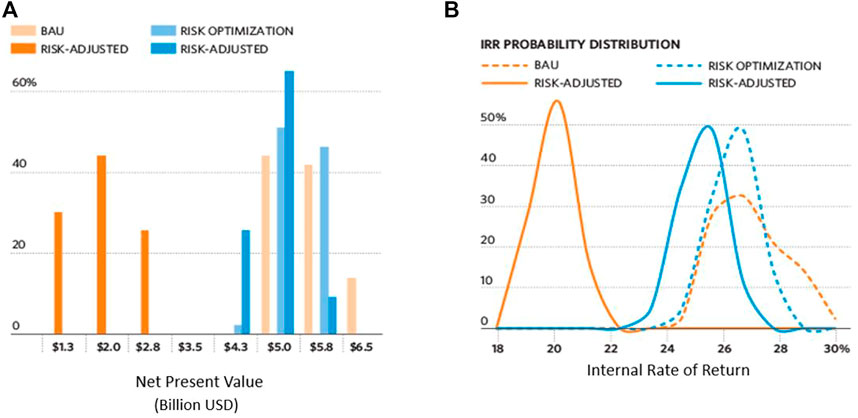
FIGURE 9. Distribution of Net Present Value (NPV) (A) and Internal Rate of Return (IRR) (B) for BAU compared to risk optimization scenarios for hydropower development in the Magdalena Basin, Colombia. Both figures show values of the aggregated cashflows from all projects in a scenario, with distributions of values reflecting 50 simulations of cashflows for each scenario.
We then applied the risk optimization scenario—also intended to meet the same generation target as the BAU—to select a portfolio of projects based on their risk-adjusted NPV values (i.e., on an ex-ante basis). Note that the risk optimization selection process also used the basin-scale efficiencies from the system engineering scenario. See Supplementary Material for the full methods of this analysis.
We then modeled the impact of risk on project performance, and thus scenario performance, through a probabilistic application of the environmental and social impact indices to individual projects within the Monte Carlo simulations. Because they had been selected without consideration of environmental and social risks, this modeling of risk had a strong impact on project and scenario performance in the BAU with a considerable decline in NPV and IRR values (see the leftward shift from BAU to risk-adjusted BAU in Figure 9). The BAU was composed of a few large, complex projects that carried high risks for conflicts and associated delays and cost overruns. In contrast, the risk optimization scenario selected a higher number (18) of smaller projects. Because the risk optimization scenario used a project selection process that incorporated projections of risk, the modeling of the impacts of those risks to the risk optimization scenario resulted in a much smaller decline in NPR and IRR (Figure 9 and Table 1).
• Hydropower by Design (HBD). This scenario selected projects using both system engineering and risk optimization and included criteria to fulfill specific conservation goals (e.g., maintain connected river systems for migratory fish). In this case, the scenario included constraints that removed projects from the pool the model could select from, including rules to avoid dams on the mainstem Magdalena and a set of free-flowing tributaries (Saldaña, Carare, Cesar, and San Jorge rivers).
The HbD scenario resulted in lower levels of negative impacts compared to the BAU, particularly for social impacts. Because the HbD scenario selected projects based on financial estimates that reflected potential risk, it also had improved NPV and IRR compared to the BAU (Figure 10 and Table 1).
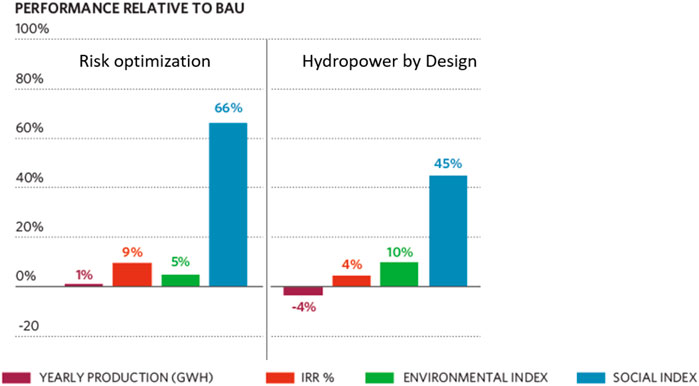
FIGURE 10. Performance relative to the BAU scenario for financial, power, social and environmental metrics for the risk optimization scenario and the HbD scenario. Note that “positive” bars indicate improved performance relative to the BAU (e.g., a positive bar on “environmental index” indicates better environmental performance and reduced negative impacts on environmental values).
As mentioned above, this is an illustrative analysis and results should be interpreted with caution. However, the analysis relied on modeling tools that are commonly used by the hydropower sector. The results provide support for the assertion that hydropower system design can offer financial benefits to investors and developers through both capturing system engineering efficiencies (i.e., favoring projects that take advantage of system-scale synergies) and through reducing risk (i.e., favoring projects that are less likely to trigger conflicts that lead to delays and cost overruns). In the past 15 years, hydropower projects, including the Porce IV and Cañafisto projects, have been suspended due to social and environmental issues, after significant preparation costs had been incurred, illustrating the relationship between these risks and investment risk in this basin. The results of these illustrative analyses warrant further research into the financial benefits of hydropower system design.
4.2 Hypothesis: Comprehensive power system planning can help governments achieve multiple objectives simultaneously and avoid conflicts
Ensuring that power systems are expanded and maintained to provide reliable electricity service capable of meeting demand over time is a primary objective for governments. However, governments have a range of other objectives. Here we explore the hypothesis that power system planning that fully integrates objectives for rivers and/or other important social and environmental resources—i.e., the pursuit of grids that are LowCx3—can provide direct benefits to governments. This comprehensive planning is focused on achieving climate and energy goals while avoiding, minimizing or mitigating conflicts to the extent possible. The reduction of risk of conflict has two primary benefits for governments. First, reducing the risk of conflict over projects reduces the likelihood of delays, cost over-runs and cancellations, which avoids unexpected impacts to the power system which could negatively affect cost and system reliability. Second, a planning approach that seeks to balance energy objectives with other objectives can help identify options that satisfy multiple public policy goals.
4.2.1 Uganda case study
To explore these two potential sources of benefit, we modeled options for power system development in Uganda, a country with an electrification rate of 26% and where hydropower provides 90% of installed capacity. Projections for 2045 include an increase in total grid capacity of approximately five times, with multiple large hydropower dams among the planned capacity additions. Several of the potential hydropower dams are within national parks. Tourism represents an important part of Uganda’s economy, contributing approximately 8% of Gross Domestic Product and 7% of employment, with much of that tourism driven by national parks and wildlife viewing (Centre for the Promotion of Imports, 2020).
In 2019 the Ugandan government announced feasibility studies for a 360-MW hydropower dam on Uhuru Falls of the Nile River, approximately 500 m from the popular tourist site of Murchison Falls and within Murchison Falls National Park, the most visited national park in Uganda. The proposal triggered objections from conservation organizations and disagreement within the government; the approval to pursue feasibility studies has been given, then rescinded and then restarted (Mongabay.com, 2019). It is not clear whether the dam will move forward, but the proposals have highlighted that several of Uganda’s options for new hydropower come from dams within national parks, thus setting up a conflict between the governments’ objectives for power development and for protecting national parks, which are important for wildlife and tourism.
To identify potential solutions for resolving this conflict, we used a capacity expansion model, SWITCH, to compare two power system development scenarios up to 2045: 1) the BAU that was free to select new hydropower projects within national parks; and 2) a “LowCx3” option that avoids any new dams in national parks. SWITCH is a state-of-the-art planning model for a regional or national power system that identifies the least cost expansion of supply side resources and transmission to meet prescribed levels of hourly load, subject to a host of power system technical constraints (He et al., 2016). The model includes an hourly dispatch of representative days to capture the synergies between hourly production of variable renewable resources and load, in addition to complying with resource adequacy needs for a power system with variable resources. SWITCH is calibrated with a portfolio of existing generation units and transmission lines. The model selects generation and transmission expansion projects from a portfolio of potential projects with characteristics supplied by the user. The list of potential projects that SWITCH could select from and data sets (e.g., solar radiation patterns for Uganda) can be found in the Supplementary Material.
The least-cost “reference scenario” included an expansion of hydropower capacity from 1.5 GW today to 2.1 GW by 2045 and the model selected two new hydropower dams located within national parks. In the “LowCx3” scenario, which was prevented from selecting projects within national parks, greater investment in solar PV and storage replaced the 2 dams that were selected with the reference scenario (Figure 11).
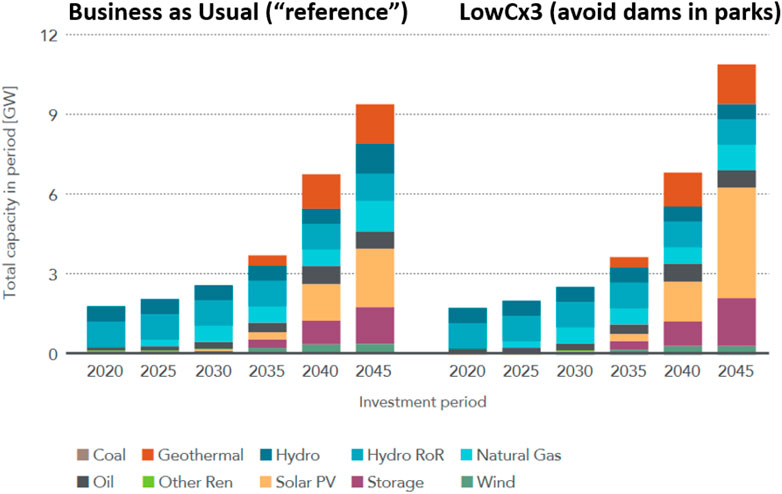
FIGURE 11. Installed capacity, by technology and time period, for the BAU scenario (“reference”) and a “LowCx3” scenario that avoided hydropower dams in national parks.
Both scenarios had very low carbon intensities (<0.04 kgCO2/MWh) and emissions per capita (0.01 kgCO2/person). Importantly, costs for the two scenarios were nearly identical, suggesting that Uganda could build a low-carbon power system by 2045 that avoids further damming within national parks for no additional cost to its people or economy. Although this was a modeling exercise with some use of data from other regions as estimates, it shows that the Ugandan government can likely avoid trading off the integrity of its national parks in order to deliver power to its people. This type of strategic planning offers the government the opportunity to identify options that avoid conflicts and perform well for multiple objectives (e.g., power expansion, climate objectives, tourism).
4.2.2 Benefits to governments of power system planning
The Uganda case study illustrates how system-scale planning, in the pursuit of power systems that are LowCx3, can provide benefits to governments by identifying development options that perform well across multiple objectives. The ability of a system-scale approach to provide multiple benefits has been well established, at least as far back as the coordinated basin planning and development of the TVA (Boccaletti, 2021). More recent studies have found that hydropower system design can identify better outcomes for multiple traditional government objectives, such as irrigation and flood management (Opperman et al., 2017). Jeuland et al. (2014) reported that coordinated management of hydropower reservoirs in the Nam Ngum river basin in Laos could increase net benefits from irrigation and hydropower by 3%—12%. Lee et al. (2009) found that a system-scale approach for prescribing operations across the multipurpose hydropower dams on the Columbia River could allow the system to provide better outcomes across multiple objectives—and be more resilient to climate change. Compared to the existing operation, which used fixed flood-control release curves for each dam, optimized curves developed for the whole system could generate more hydropower while maintaining equivalent levels of flood-risk reduction. In addition, this system-scale approach to operations would also increase reservoir storage in the late summer, increasing the ability to maintain appropriate flows and temperatures for salmon.
In addition to providing multiple benefits, a system planning approach can reduce conflicts—including those that could interfere with power system expansion through delays, cost overruns and cancellations. Proposals for hydropower in Chile, particularly those on the wild rivers of Patagonia, have often led to conflict and protests, including the cancellation of HidroAysén after US$320 million had already been invested. The Chilean example, reviewed in Section 3.3, showed that Chile could meet its low-carbon power targets without damming any more free-flowing rivers, with minimal impact on system cost, and thus avoid the conflicts, delays and cancellations likely to be triggered by development of pristine river basins. Similarly, modeling to find a LowCx3 option suggests that Uganda can meet future power demands without building dams in national parks, which would likely lead to conflicts (prior to construction) and negative impacts on ecosystems and tourism (if constructed).
Overall, these various studies suggest that governments have much to gain by power system planning that seeks LowCx3 options, both in terms of avoiding conflict and disruption and by achieving multiple objectives.
5 Conclusion
Governments confront a considerable challenge in meeting future demands for power while dramatically decarbonizing power systems. Although annual investments in wind and solar now far outpace those for hydropower, hydropower is still the leading source of low-carbon electricity today and it will continue to play a key role in future low-carbon power systems. However, hydropower projects have the potential to cause considerable negative impacts on rivers and the communities and ecosystems that depend on them. Projections for a doubling of global hydropower capacity would likely lead to a dramatic loss of those large, free-flowing rivers that remain (Thieme et al., 2021). Further, these negative impacts can trigger social conflicts, or regulatory scrutiny, that leads to delays, cost overruns and even cancellations—all of which negatively impact developers and investors and disrupt governments seeking to reliably expand their national or regional power systems.
Thus, many different actors—ranging from communities to investors to ministries of energy and ministries of the environment—have a stake in finding solutions for hydropower development and management that reduce impacts, avoid conflicts, and achieve a better balance between various stakeholders’ priority objectives. Here we summarize the support this paper found for several hypotheses about these solutions.
• First, while project-scale mitigation can address some of the negative impacts associated with hydropower, the best opportunity for more balanced outcomes will emerge from system-scale planning and management.
• More balanced outcomes from hydropower systems include better performance for a range of environmental (e.g., fish populations) social (e.g., livelihoods), and economic (e.g., other water-management sectors, such as water supply) objectives.
• Integrating planning for hydropower and river resources with energy modeling (e.g. a capacity expansion model) can provide results relevant to energy planners and operators and has the best opportunity to identify options that meet climate and energy goals while maintaining healthy rivers.
• Hydropower system design offers two pathways for benefit for developers and investors: (1) operational synergies achieved through system optimization; and (2) better management of risk to reduce delays and cost overruns. Both pathways can improve financial performance for projects that emerge from a system planning process relative to those that emerge through single-project decision making commonly pursued by developers.
• Energy system planning can provide benefits to governments because it can help them to achieve multiple objectives simultaneously and to avoid the disruptions that come from conflict around power system expansion.
Despite these multiple benefits, hydropower system design, or energy system planning that fully integrates environmental and social resources, remains relatively rare. Current instruments aimed at promoting “sustainable hydropower,” such as the Hydropower Sustainability Standard (Hydropower Sustainability Secretariat, 2021), are focused on individual projects and therefore tend to overlook both system-scale costs and opportunities; without this perspective, sustainability assessments are missing the most important scale for identifying options for a sustainable power system (However, note that the Standard’s requirements that projects show their “demonstrated need and strategic fit” can potentially provide a linkage between individual project assessment and system planning).
System-scale planning is certainly more complicated and requires more participants than single project planning. Thus, to support system planning, various actors need to see real value in it. System approaches may be relatively rare in part because, to date, relevant analyses have focused on the potential for better outcomes for environmental and social resources through system-scale approaches. In many cases, these outcomes will not be the top priority of the most influential decision makers. To address that potential constraint, this paper examined hypotheses that these approaches will also have measurable benefits to key actors such as investors, developers and government agencies, finding support for those benefits. Further research to explore and validate these benefits, coupled with effective communication to key audiences, may increase the adoption of system-scale approaches. Beyond research, innovative implementation mechanisms of system planning should also be aimed at demonstrating value to diverse actors, with subsequent project-level investments aligned with system plans. Multilateral institutions, such as the World Bank, that have multiple institutional objectives (e.g., energy, food, and biodiversity) could support comprehensive energy planning processes and signal that they will invest in those projects that are consistent with power systems that are LowCx3. Although these multilateral institutions fund a relatively small proportion of new projects, their investments can be catalytic and influence other funding streams.
While we emphasize that power system planning offers the most effective scale for achieving balanced outcomes for multiple objectives, the various approaches described in this paper can work in a complementary fashion and can be applied at different scales and times during the planning, development and operation phases. For example, power system planning can compare the tradeoffs and benefits of grids with different mixes of generation technologies. For systems where hydropower will play a role, and where there are high number of potential projects, hydropower system design can be applied to identify a portfolio of projects that minimize conflicts. For the projects selected from this process, project-level standards, such as the Hydropower Sustainability Standard, and best practices in design and operation (e.g., environmental flows) can then be applied. Collectively, these approaches can help governments, communities, and developers identify, and then achieve, power systems that will contribute to climate objectives and maintain the multiple values of free-flowing rivers.
Data availability statement
The raw data supporting the conclusions of this article will be made available by the authors, without undue reservation.
Author contributions
All authors contributed to research, applications, and discussions that developed the concepts offered in this paper. JO conceptualized this article and did the primary drafting of text with additional primary writing from JC, RK, RSc, JrH, JuH, JR, and DH. JO, JC, JuH, RK, RSa, RA, EC, AF, GG, JnH, DK, EM, TM, CR, and JR contributed to analyses. RK, RSc, RA, JuH, AN, MT, DH, AF, MG, EM, RSa, and JrH provided review and editing.
Conflict of interest
Author JO was employed by World Wildlife Fund. Authors RK and TM was employed by PSR Energy Consulting and Analytics. Author EC was employed by Onward Energy. Author MG was employed by WWF-Asia Pacific. Author GG was employed by Confluvio. Authors JrH was employed by Sustainable Water & Energy LLC. Authors JnH, EM, AN, JR, and CR were employed by The Nature Conservancy. Authors RS was employed by WWF-Nepal. Author MT was employed by World Wildlife Fund-US.
The remaining authors declare that the research was conducted in the absence of any commercial or financial relationships that could be construed as a potential conflict of interest.
Publisher’s note
All claims expressed in this article are solely those of the authors and do not necessarily represent those of their affiliated organizations, or those of the publisher, the editors and the reviewers. Any product that may be evaluated in this article, or claim that may be made by its manufacturer, is not guaranteed or endorsed by the publisher.
Supplementary material
The Supplementary Material for this article can be found online at: https://www.frontiersin.org/articles/10.3389/fenvs.2022.1036653/full#supplementary-material
References
Almeida, R. M., Hamilton, S. K., Rosi, E. J., Barros, N., Doria, C. R. C., Flecker, A. S., et al. (2020). Hydropeaking operations of two Run-of-River mega-dams alter downstream hydrology of the largest Amazon tributary. Front. Environ. Sci. 8. doi:10.3389/fenvs.2020.00120
Almeida, R. M., Schmitt, R., Grodsky, S. M., Flecker, A. S., Gomes, C. P., Zhao, L., et al. (2022a). Floating solar power could help fight climate change — let’s get it right. Nature 606, 246–249. doi:10.1038/d41586-022-01525-1
Almeida, R. M., Schmitt, R. J., Castelletti, A., Flecker, A. S., Harou, J. J., Heilpern, S. A., et al. (2022b). Strategic planning of hydropower development: Balancing benefits and socioenvironmental costs. Curr. Opin. Environ. Sustain. 56, 101175. doi:10.1016/j.cosust.2022.101175
Almeida, R. M., Shi, Q., Gomes-Selman, J. M., Wu, X., Xue, Y., Angarita, H., et al. (2019). Reducing greenhouse gas emissions of Amazon hydropower with strategic dam planning. Nat. Commun. 10, 4281. doi:10.1038/s41467-019-12179-5
Ansar, A., Flyvbjerg, B., Budzier, A., and Lunn, D. (2014). Should we build more large dams? The actual costs of hydropower megaproject development. Energy Policy 69, 43–56. doi:10.1016/j.enpol.2013.10.069
Balc˘iūnas, P., and Ždankus, N. (2007). Harmonization of hydropower plant with the environment. Renew. Sustain. Energy Rev. 11, 1260–1274. doi:10.1016/j.rser.2005.09.005
Baruch-Mordo, S., Kiesecker, J. M., Kennedy, C. M., Oakleaf, J. R., and Opperman, J. J. (2019). From paris to practice: Sustainable implementation of renewable energy goals. Environ. Res. Lett. 14, 024013. doi:10.1088/1748-9326/aaf6e0
Blakers, A., Stocks, M., Lu, B., and Cheng, C. (2021). A review of pumped hydro energy storage. Prog. Energy 3, 022003. doi:10.1088/2516-1083/abeb5b
Cassidy, R. A. (2018). “Water temperature, dissolved oxygen, and turbidity control in reservoir releases,” in Alternatives in regulated river management (Boca Raton, FL, USA: CRC Press), 27–62.
Centre for the Promotion of Imports (2020). Analysis of the tourism value chain in Uganda. The Netherlands. Available at: https://www.cbi.eu/market-information/tourism/vca-uganda-tourism-2020 (Accessed August 5, 2022).
Cheng, L., Opperman, J. J., Tickner, D., Speed, R., Guo, Q., and Chen, D. (2018). Managing the three Gorges dam to implement environmental flows in the Yangtze River. Front. Environ. Sci. 6. doi:10.3389/fenvs.2018.00064
Collier, M., Webb, R. H., and Schmidt, J. C. (1996). Dams and rivers: A primer on the downstream effects of dams. Reston, Virginia: US Department of the Interior, US Geological Survey.
Connor, E. J., and Pflug, D. E. (2004). Changes in the distribution and density of pink, chum, and chinook salmon spawning in the upper Skagit River in response to flow management measures. North Am. J. Fish. Manag. 24, 835–852. doi:10.1577/m03-066.1
Damme, P. A. V., Córdova-Clavijo, L., Baigún, C., Hauser, M., Doria, C. R. da C., and Duponchelle, F. (2019). Upstream dam impacts on gilded catfish Brachyplatystoma rousseauxii (Siluriformes: Pimelodidae) in the Bolivian Amazon. Neotrop. Ichthyol. 17. doi:10.1590/1982-0224-20190118
Deemer, B. R., Harrison, J. A., Li, S., Beaulieu, J. J., DelSontro, T., Barros, N., et al. (2016). Greenhouse gas emissions from reservoir water surfaces: A new global synthesis. BioScience 66, 949–964. doi:10.1093/biosci/biw117
EY (2016). Spotlight on power and utility megaprojects - formulas for success. Part 1, financing and delivery. London, UK: EY.
Flecker, A. S., Shi, Q., Almeida, R. M., Angarita, H., Gomes-Selman, J. M., García-Villacorta, R., et al. (2022). Reducing adverse impacts of Amazon hydropower expansion. Science 375, 753–760. doi:10.1126/science.abj4017
Garrett, K. P., McManamay, R. A., and Witt, A. (2023). Harnessing the power of environmental flows: Sustaining river ecosystem integrity while increasing energy potential at hydropower dams. Renewable Sustainable Energy Rev. 173, 113049. doi:10.1016/j.rser.2022.113049
Geressu, R. T., and Harou, J. J. (2015). Screening reservoir systems by considering the efficient trade-offs—Informing infrastructure investment decisions on the blue nile. Environ. Res. Lett. 10, 125008. doi:10.1088/1748-9326/10/12/125008
Gilfillan, D., and Pittock, J. (2022). Pumped storage hydropower for sustainable and low-carbon electricity grids in pacific rim economies. Energies 15, 3139. doi:10.3390/en15093139
Gonzalez, J. M., Tomlinson, J. E., Martínez Ceseña, E. A., Basheer, M., Obuobie, E., Padi, P. T., et al. (2023). Designing diversified renewable energy systems to balance multisector performance. Nat. Sustain. doi:10.1038/s41893-022-01033-0
Gonzalez, J. M., Tomlinson, J. E., Harou, J. J., Martínez Ceseña, E. A., Panteli, M., Bottacin-Busolin, A., et al. (2020). Spatial and sectoral benefit distribution in water-energy system design. Appl. Energy 269, 114794. doi:10.1016/j.apenergy.2020.114794
Grill, G., Lehner, B., Thieme, M., Geenen, B., Tickner, D., Antonelli, F., et al. (2019). Mapping the world’s free-flowing rivers. Nature 569, 215–221. doi:10.1038/s41586-019-1111-9
Hamerlynck, O., and Duvail, S. (2003). The rehabilitation of the delta of the Senegal River in Mauritania: Fielding the ecosystem approach. Gland, Switzerland: IUCN.
Hartmann, J. (2020). How-to guide: Hydropower downstream flow regimes. London: International Hydropower Association. Available at: https://www.hydropower.org/publications/how-to-guide-hydropower-downstream-flow-regimes.
Hartmann, J., Harrison, D., Opperman, J., and Gill, R. (2013). The new frontier of hydropower sustainability: Planning at the system scale. Washington, DC: Inter-American Development Bank.
He, G., Avrin, A. P., Nelson, J. H., Johnston, J., Mileva, A., Tian, J., et al. (2016). SWITCH-China: A systems approach to decarbonizing China’s power system. Environ. Sci. Technol. 50, 5467–5473. doi:10.1021/acs.est.6b01345
Hunt, J. D., Byers, E., Wada, Y., Parkinson, S., Gernaat, D. E. H. J., Langan, S., et al. (2020). Global resource potential of seasonal pumped hydropower storage for energy and water storage. Nat. Commun. 11, 947. doi:10.1038/s41467-020-14555-y
Hurford, A. P., and Harou, J. J. (2014). Balancing ecosystem services with energy and food security – assessing trade-offs from reservoir operation and irrigation investments in Kenya’s Tana Basin. Hydrology Earth Syst. Sci. 18, 3259–3277. doi:10.5194/hess-18-3259-2014
Hurford, A. P., Harou, J. J., Bonzanigo, L., Ray, P. A., Karki, P., Bharati, L., et al. (2020a). Efficient and robust hydropower system design under uncertainty - a demonstration in Nepal. Renew. Sustain. Energy Rev. 132, 109910. doi:10.1016/j.rser.2020.109910
Hurford, A. P., Huskova, I., and Harou, J. J. (2014). Using many-objective trade-off analysis to help dams promote economic development, protect the poor and enhance ecological health. Environ. Sci. Policy 38, 72–86. doi:10.1016/j.envsci.2013.10.003
Hurford, A. P., McCartney, M. P., Harou, J. J., Dalton, J., Smith, D. M., and Odada, E. (2020b). Balancing services from built and natural assets via river basin trade-off analysis. Ecosyst. Serv. 45, 101144. doi:10.1016/j.ecoser.2020.101144
Hydropower Sustainability Secretariat (2021). Hydropwer sustainability standard. London: Hydropwer Sustainability Secretariat.
International Energy Agency (IEA) (2021). Net zero by 2050 - a roadmap for the global energy sector. Paris: International Energy Agency.
International Centre for Environmental Management (2010). MRC strategic environmental assessment (SEA) of hydropower on the Mekong mainstream: Summary of the final report. Tây Hô, Hà Nôi, Vietnam: International Centre for Environmental Management.
International Hydropower Association (2021). The san Jose declaration on sustainable hydropwer. London: IHA.
International Renewable Energy Agency (IRENA) (2021). World energy transitions outlook: 1.5°C pathway. Abu Dhabi, UAE: International Renewable Energy Agency. Available at: https://www.irena.org/publications/2021/Jun/World-Energy-Transitions-Outlook (Accessed November 8, 2021).
International Renewable Energy Agency (IRENA) (2020). Global renewables outlook: Energy transformation 2050. Abu Dhabi, UAE: International Renewable Energy Agency.
International Renewable Energy Agency (IRENA) (2022). IRENA RE time series. Mountain View, CA, USA: Tableau Software. Available at: https://www.irena.org/Data/View-data-by-topic/Capacity-and-Generation/Statistics-Time-Series (Accessed August 30, 2022).
Jeuland, M., Baker, J., Bartlett, R., and Lacombe, G. (2014). The costs of uncoordinated infrastructure management in multi-reservoir river basins. Environ. Res. Lett. 9, 105006. doi:10.1088/1748-9326/9/10/105006
Karki, P. (2015). South asia-investment decision making in hydropower: Decision tree case study of the upper arun hydropower project and koshi basin hydropower development in Nepal. Washington, DC, USA: The World Bank.
Katusa, M. (2010). Hydroelectric revolution: a river runs through it. Jersey City, NJ, USA: Forbes. Available at: https://www.forbes.com/sites/energysource/2010/06/15/hydroelectric-revolution-a-river-runs-through-it/ (Accessed June 10, 2022).
Kiesecker, J. M., Copeland, H., Pocewicz, A., and McKenney, B. (2010). Development by design: Blending landscape-level planning with the mitigation hierarchy. Front. Ecol. Environ. 8, 261–266. doi:10.1890/090005
Kondolf, G. M., Gao, Y., Annandale, G. W., Morris, G. L., Jiang, E., Zhang, J., et al. (2014). Sustainable sediment management in reservoirs and regulated rivers: Experiences from five continents. Earth's. Future 2, 256–280. doi:10.1002/2013EF000184
Kondolf, G. M., Schmitt, R. J. P., Carling, P. A., Goichot, M., Keskinen, M., Arias, M. E., et al. (2022). Save the Mekong delta from drowning. Science 376, 583–585. doi:10.1126/science.abm5176
Kumar, A., Schei, T., Ahenkorah, A., Caceres Rodriguez, R., Devernay, J.-M., Freitas, M., et al. (2011). “Hydropower,” in IPCC special report on renewable energy sources and climate change mitigation, 437–496.
Ledec, G., and Quintero, J. D. (2003). Good dams and bad dams: Environmental criteria for site selection of hydroelectric projects.
Lee, J., Hamlet, A., Asce, M., Fitzgerald, C., Burges, S., and Asce, F. (2009). Optimized flood control in the Columbia River Basin for a global warming scenario. J. Water Resour. Plan. Manag. 135, 440–450. doi:10.1061/(asce)0733-9496(2009)135:6(440)
Ligon, F. K., Dietrich, W. E., and Trush, W. J. (1995). Downstream ecological effects of dams. BioScience 45, 183–192. doi:10.2307/1312557
Mekong River CommissionAsia Development BankWWF (2016). Rapid basin-Wide hydropower sustainability assessment tool (RSAT). Vientiane, Laos: Mekong River Commission.
Mongabay.com (2019). In a flip-flop, Uganda says it’ll allow a study for a dam at Murchison Falls. Menlo Park, CA, USA: Mongabay Environmental News. Available at: https://news.mongabay.com/2019/12/in-a-flip-flop-uganda-says-itll-allow-a-study-for-a-dam-at-murchison-falls/ (Accessed August 5, 2022).
Noonan, M. J., Grant, J. W. A., and Jackson, C. D. (2012). A quantitative assessment of fish passage efficiency. Fish. Fish. (Oxf). 13, 450–464. doi:10.1111/j.1467-2979.2011.00445.x
Ocko, I. B., and Hamburg, S. P. (2019). Climate impacts of hydropower: Enormous differences among facilities and over time. Environ. Sci. Technol. 53, 14070–14082. doi:10.1021/acs.est.9b05083
Opperman, J., Hartmann, J., Lambrides, M., Carvallo, J., Chapin, E., Baruch-Mordo, S., et al. (2019). Connected and flowing: A renewable future for rivers, climate, and people. Washington, D.C.: WWF and The Nature Conservancy.
Opperman, J. J., Hartmann, J., Raepple, J., Angarita, H., Beames, P., Chapin, E., et al. (2017). The power of rivers: A business case. Arlington, VA: The Nature Conservancy.
Opperman, J. J., Grill, G., and Hartmann, J. (2015). The power of rivers. Arlington, VA: The Nature Conservancy.
Opperman, J. J., Kendy, E., Tharme, R. E., Warner, A. T., Barrios, E., and Richter, B. D. (2018). A three-level framework for assessing and implementing environmental flows. Front. Environ. Sci. 6. doi:10.3389/fenvs.2018.00076
Opperman, J. (2019). Powering the world and saving rivers with low carbon, low cost and low conflict energy grids. Film City, Noida: ET Energy World. Available at: https://energy.economictimes.indiatimes.com/energy-speak/powering-the-world-and-saving-rivers-with-low-carbon-low-cost-and-low-conflict-energy-grids/3592 (Accessed July 30, 2022).
Opperman, J., Royte, J., Banks, J., Rose Day, L., and Apse, C. (2011). The Penobscot River, Maine, USA: A basin-scale approach to balancing power generation and ecosystem restoration. Ecol. Soc. 16, art7. doi:10.5751/ES-04117-160307
Pelicice, F. M., and Agostinho, A. A. (2008). Fish-passage facilities as ecological traps in large neotropical rivers. Conserv. Biol. 22, 180–188. doi:10.1111/j.1523-1739.2007.00849.x
Pelicice, F. M., Pompeu, P. S., and Agostinho, A. A. (2015). Large reservoirs as ecological barriers to downstream movements of Neotropical migratory fish. Fish. Fish. (Oxf). 16, 697–715. doi:10.1111/faf.12089
Pompeu, P. S., Agostinho, A. A., and Pelicice, F. M. (2012). Existing and future challenges: The concept of successful fish passage in South America. River Res. Appl. 28, 504–512. doi:10.1002/rra.1557
PSR (2020). HERA user manual. Rio de Janeiro, RJ- Brasil: PSR. Available at: https://www.psr-inc.com/wp-content/uploads/softwares/herausreng.pdf
Räsänen, T. A., Varis, O., Scherer, L., and Kummu, M. (2018). Greenhouse gas emissions of hydropower in the Mekong River Basin. Environ. Res. Lett. 13, 034030. doi:10.1088/1748-9326/aaa817
Sadler, B., Verocai, I., and Vanclay, F. (2000). Environmental and social impact assessments for large dams. Cape Town, South Africa: The World Commission on Dams.
Schlömer, S., Bruckner, T., Fulton, L., Hertwich, E., McKinnon, A., Perczyk, D., et al. (2014). “Annex III: Technology-specific cost and performance parameters,” in Climate change 2014: Mitigation of climate change: Contribution of working group III to the fifth assessment report of the Intergovernmental Panel on Climate Change (Cambridge: Cambridge University Press), 1329–1356.
Schmitt, R., Bizzi, S., Castelletti, A., and Kondolf, G. (2018). Improved trade-offs of hydropower and sand connectivity by strategic dam planning in the Mekong. Nat. Sustain. 1, 96–104. doi:10.1038/s41893-018-0022-3
Schmitt, R. J. P., Bizzi, S., Castelletti, A., Opperman, J. J., and Kondolf, G. M. (2019). Planning dam portfolios for low sediment trapping shows limits for sustainable hydropower in the Mekong. Sci. Adv. 5, eaaw2175. doi:10.1126/sciadv.aaw2175
Schmitt, R. J. P., Kittner, N., Kondolf, G. M., and Kammen, D. M. (2021). Joint strategic energy and river basin planning to reduce dam impacts on rivers in Myanmar. Environ. Res. Lett. 16, 054054. doi:10.1088/1748-9326/abe329
Shirley, R., and Kammen, D. (2015). Energy planning and development in Malaysian Borneo: Assessing the benefits of distributed technologies versus large scale energy mega-projects. Energy Strategy Rev. 8, 15–29. doi:10.1016/j.esr.2015.07.001
Siala, K., Chowdhury, A. K., Dang, T. D., and Galelli, S. (2021). Solar energy and regional coordination as a feasible alternative to large hydropower in Southeast Asia. Nat. Commun. 12, 4159. doi:10.1038/s41467-021-24437-6
Sovacool, B. K., Nugent, D., and Gilbert, A. (2014). Construction cost overruns and electricity infrastructure: An unavoidable risk? Electr. J. 27, 112–120. doi:10.1016/j.tej.2014.03.015
Tharme, R. E. (2003). A global perspective on environmental flow assessment: Emerging trends in the development and application of environmental flow methodologies for rivers. River Res. Appl. 19, 397–441. doi:10.1002/rra.736
Thayer Scudder (2005). The future of large dams: Dealing with social, environmental, institutional and political costs. London: Earthscan.
The Nature Conservancy and WWF (2016). Improving hydropower outcomes through system-scale planning: An example from Myanmar. Arlington, VA: The Nature Conservancy and WWF.
Thieme, M. L., Tickner, D., Grill, G., Carvallo, J. P., Goichot, M., Hartmann, J., et al. (2021). Navigating trade-offs between dams and river conservation. Glob. Sustain. 4, e17. doi:10.1017/sus.2021.15
World Bank Group (2018). Environmental flows for hydropower projects: Guidance for the private sector in emerging markets. Washington, DC: World Bank. doi:10.1596/29541
World Commission on Dams (2000). Dams and development: A new framework for decision-making: The report of the world commission on dams. London: Earthscan.
WWF and Dai Global (2021). System-scale planning to support sustainable energy systems and conservation of freshwater resources for people and nature. report funded by USAID.
Keywords: rivers, renewable energy, system-scale planning, environmental mitigation, migratory fish
Citation: Opperman JJ, Carvallo JP, Kelman R, Schmitt RJP, Almeida R, Chapin E, Flecker A, Goichot M, Grill G, Harou JJ, Hartmann J, Higgins J, Kammen D, Martin E, Martins T, Newsock A, Rogéliz C, Raepple J, Sada R, Thieme ML and Harrison D (2023) Balancing renewable energy and river resources by moving from individual assessments of hydropower projects to energy system planning. Front. Environ. Sci. 10:1036653. doi: 10.3389/fenvs.2022.1036653
Received: 04 September 2022; Accepted: 14 November 2022;
Published: 04 January 2023.
Edited by:
Silvia Quadroni, University of Insubria, ItalyReviewed by:
Paolo Espa, University of Insubria, ItalyFrank Onderi Masese, University of Eldoret, Kenya
Francesco Caponi, ETH Zürich, Switzerland
Giuseppe Roberto Pisaturo, Free University of Bozen-Bolzano, Italy
Copyright © 2023 Opperman, Carvallo, Kelman, Schmitt, Almeida, Chapin, Flecker, Goichot, Grill, Harou, Hartmann, Higgins, Kammen, Martin, Martins, Newsock, Rogéliz, Raepple, Sada, Thieme and Harrison. This is an open-access article distributed under the terms of the Creative Commons Attribution License (CC BY). The use, distribution or reproduction in other forums is permitted, provided the original author(s) and the copyright owner(s) are credited and that the original publication in this journal is cited, in accordance with accepted academic practice. No use, distribution or reproduction is permitted which does not comply with these terms.
*Correspondence: Jeffrey J. Opperman, amVmZi5vcHBlcm1hbkB3d2Yub3Jn