- College of Chemistry and Environmental Engineering, Yangtze University, Jingzhou, China
The exploitation of petroleum, the production and use of petroleum products causes pollution that is harmful to the ecology and environment. At present, petroleum hydrocarbon pollution has become a universal concern in the world. As one of the bioremediation methods, plant root exudate repair has the effect of eliminating toxic substances in the environment. To explore the role of root exudates glycine in phytoremediation of petroleum-contaminated soil, seed germination and pot experiments were carried out to study the effects of glycine on the degradation of petroleum hydrocarbons in different plants and soil types. The results showed that when the concentration of petroleum pollutants was constant, the order of seed germination rate of the eight plants was ryegrass > sudan grass > white clover > tall fescue > alfalfa > pennisetum > canine root > maize grass.1000 mg L−1 of glycine could effectively promote plant biomass and the petroleum hydrocarbons degradation rate. The degradation effect was ryegrass + glycine > ryegrass > sudan grass + glycine > sudan grass > white clover + glycine > white clover. The degradation rate of ryegrass + glycine in 18,000 mg kg−1 petroleum-contaminated soil reached 55.7%. All plants had the highest biomass and plant height in loamy soil, while the highest degradation rate of petroleum hydrocarbons was observed in sandy soil, ranging from 50.36% to 59.36%. Among them, ryegrass combined with 1000 mg L−1 of glycine reached the highest petroleum hydrocarbons degradation rate of 59.36% in sandy soil. Ryegrass, sudan grass and white clover had the potential to remediate petroleum-contaminated soils. In the three types of soil, ryegrass had a strong remediation efficiency, and the degradation effect to petroleum hydrocarbons was more significant after the addition of glycine. Therefore, ryegrass can be planted with glycine as a priority for remediation in oil field areas contaminated by petroleum hydrocarbons.
Introduction
Nowadays, one of the most serious pollution types on the earth is petroleum pollution, with countries paying an increasing amount of attention to the technology that can help in the remediation of petroleum pollution. Due to the extensive amount of production and use of petrochemical products, a widespread problem of soil contamination by organic pollutants has developed all over the world. Furthermore, fuel transportation has caused an increase in environmental risks, such as spills, leaks, and discharges (Al-Mansoory et al., 2017). After petroleum hydrocarbons enter the soil, they begin by causing changes in the physical structure and the chemical properties of the soil. Such changes include destroying clogging of soil pores, significantly diminishing soil aeration and water permeability, disturbing the balance and ratio of carbon, nitrogen, and phosphorus, all the while reducing soil nutrients. At the same time, the living environment of soil microorganisms are being also changed, resulting in the change of the microbial community and its quantity in the soil microenvironment (Pacwa-Płociniczak et al., 2019). Furthermore, the deterioration of the soil ecosystem leads to a significant decline in the yield and quality of crops grown in it, leading to a serious decline in farmers’ economic income (Shah and Wu, 2019). Critically, crops such as rice and wheat absorb some of the toxic hydrocarbons when they grow in hydrocarbon-contaminated soils, which then directly harm people and the planet as they enter into the food chain (Khan et al., 2018).
The detoxification of petroleum-contaminated soils can be carried out by physical, chemical and biological remediation strategies and their comprehensive methods. Traditional physical-chemical methods including excavation, desorption, thermal destruction, soil washing, soil flushing, stabilization, soil vapor extraction, and many others. Although these methods are fast and effective, they have some limitations, such as high cost, high labor intensity and they tend to compromise the environmental matrices (Zuzolo et al., 2021). Bioremediation for petroleum contaminated soil mainly include phytoremediation, microbial remediation, and microbial-plant combined remediation. Phytoremediation takes an advantage of the interaction between plants, rhizosphere microorganisms, and soil environment to remove, decompose, absorb or adsorb soil pollutants, so as to restore the soil environment. Phytoremediation has been proven to be effective in removing organic pollutants from soil acting as a low-cost green solution with low ecological risks and side effects to humans and the environment (Kurade et al., 2021). Binet et al. (2000) studied the degradation of three to six cyclic polycyclic aromatic hydrocarbons (PAHs) (anthracen, phenanthrene, fluoranthene, benzo [a] fluoranthene, dibenzo [a, h] anthracene) by ryegrass. In their study, it was found that ryegrass has a great potential for the degradation of most PAHs including five and six rings. Reilley et al. (1996) chose four plants (Fectuca Ovina Schreb, Medicago Sativa, Sorghum Sudanense, and Trifolium) to study PAHs degradation in petroleum-contaminated soil, which found that the degradation rate of anthracene and pyrene in plants increased significantly. Furthermore, in a microcosm experiment involving Medicago sativa L. and the isolated bacterial consortium, M. sativa + Consortium resulted in 91% removal of diesel hydrocarbons in just 60 days (Eze et al., 2022). Although the research on the direction of phytoremediation has been recently relatively growing unto maturity or increased with vast literature, the application scope of phytoremediation is still quite limited. Due to the limitation of plant root length, its remediation area can only be in the shallow soil layer of 0–50 cm, while the deeper contaminated soil cannot be reached and remediated by plant roots. Therefore, other remediation technologies are required to be investigated for the joint treatment of soils that are contaminated by petroleum hydrocarbons at a deeper level.
Root exudates refer to the organic matter secreted to the surrounding environment by some tissues and organs of plants with vitality under certain conditions (Hassan and Nawchoo, 2022). It can be divided into saccharide, amino acid, organic acid, phenolic acid, fatty acid, sterol, protein, growth factor, etc. Root exudates can produce obvious effects on the rhizosphere environment. This includes changing soil pH, increasing soil cation exchange capacity (CEC), stimulating the rapid reproduction and growth of rhizosphere microorganisms, and promoting the release of nutrients in the soil. A large number of studies have shown that root exudates can effectively promote the degradation of organic pollutants in soils, strengthen the activation and fixation of inorganic pollutants. Wild et al. (2005) used summer pea and white mustard to repair heavily petroleum-contaminated soil through pot experiments. In their experiment they found that the removal rate of petroleum pollutants in non-rhizosphere soil was 59%, while that in rhizosphere soil was 71%, which was 20.3% higher than that of the former. Kirk et al. (2005) found that the number of ryegrass rhizosphere heterotrophic bacteria and petroleum hydrocarbon-degrading bacteria was 233.37 times higher than that of untouched soil control. Lee et al. (2007) applied four Korean native plants to study the degradation of PAHs and found that the degradation of PAHs by plants mainly depended on the improvement of microbial activity and the action of enzymes released by plants. The activities of phenolic compounds (root exudates) and peroxidase in the experimental soil were significantly increased. According to literature, there are four ways that root exudates can promote petroleum hydrocarbons degradation. First, enzymes surrounding root exudates can directly degrade petroleum hydrocarbons pollutants. Secondly, root exudates can separate petroleum hydrocarbons from soil substrate and improve the bioavailability of organic matter. Thirdly, root exudates can enhance the elimination of petroleum hydrocarbon pollutants by stimulating enzyme channels and intensifying the synergistic metabolic process. Fourthly, root exudates provide nutrients and energy for microbial growth and reproduction (Ruley et al., 2022).
In continuation, through an extensive investigation on phytoremediation of organic-contaminated soils, it is found that the contribution rate of absorption or fixation of organic pollutants by plants is not high during the remediation process. On the contrary, the rhizosphere environment of plants effectively promotes the transfer of organic pollutants and microbial degradation (Euliss et al., 2008; Gerhardt et al., 2009). Substances produced and secreted by plant roots select and reshape microbial communities in rhizosphere soils (Tartaglia et al., 2022). Hence, the selection of appropriate root exudates and their concentrations are vital in rhizosphere restoration and phytoremediation. In addition, the physico-chemical properties and parameters of different soil types are varied, which also affect the root tissue of plants and surrounding microorganisms. Therefore, it is of great significance to find a suitable soil type for plant growth and total petroleum hydrocarbons removal. At present, there is no systematic study on the direct effect of plant root exudates on petroleum-contaminated soil. Furthermore, if root exudates with obvious remediation effect on petroleum-contaminated soil can be screened out, it will create great economic and social benefits. Doing so will also open up a new way for the remediation of petroleum-contaminated soil. Glycine is among one of the most commonly detected amino acids in forest soil. It is of small molecular weight and simple structure, which can be used as a nitrogen source for absorption and utilization by plants and microorganisms (Lipson and Näsholm, 2001). The preliminary experiment showed that 1000 mg kg−1 glycine could effectively promote the degradation of petroleum hydrocarbons in soil.
In this study, based on field investigation and analysis of petroleum-contaminated soil, plants that can repair petroleum-contaminated soil were screened out through pot experiments to study the adaptive growth of plants under different petroleum pollution conditions. According to the selected specific plants, the effects of glycine and different soil types on petroleum hydrocarbons degradation were studied, and the synergistic promoting effect of glycine on phytoremediation of petroleum-contaminated soil was explored.
Experimental materials and methods
Materials
All the herbal seeds were purchased from Huazhong Agricultural University, including Lolium perenne L., Sorghum sudanense (Piper) Stapf., Trifolium repens, Festuca arundinacea L., Medicago sativa, Pennisetum alopecuroides (L.) Spreng., Cynodon dactylon (L.) Pers., and Dracocephalum moldavica. The clean soil used in the experiment was taken from farmland near Yangtze University, and different types of soil samples were taken from Changning-weiyuan area of Sichuan province. The physical and chemical properties of the soils are in Table 1. The petroleum used in the experiment was obtained from Jianghan oilfield in Hubei, Qianjiang city, Hubei province. The glycine (C2H5NO2, 99.5%–100%) reagent was obtained from Sinophanic Chemical Reagents (Shanghai) Co., Ltd. Only distilled water was used in this study.
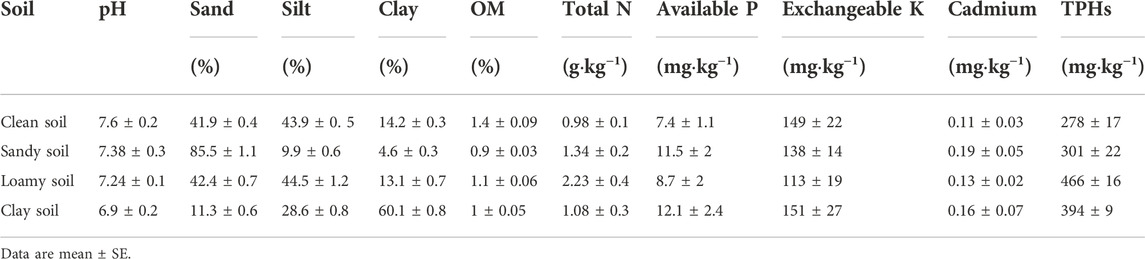
TABLE 1. Physico-chemical characteristics of experimental soils (0–30 cm), including pH, Sand, Silt, Clay, Organic Matter (OM), Total Nitrogen (Total N), Available Phosphorus (Available P), Exchangeable Potassium (Exchangeable K), Cadmium and Total Petroleum Hydrocarbons (TPHs).
RQH-450 Series Climate Incubator were purchased from Shanghai Jinghong Co., Ltd. (Shanghai, China).
Test design
Seed germination test of plants
As per a field investigation of the soil environment from the shale gas well site in Changning-Weiyuan showed that the petroleum content was unevenly distributed, with a maximum value of about 18,000 mg kg−1. Air-dried clean soil was sieved (2 mm). A certain quality of petroleum was weighed and dissolved in petroleum ether. Each 1 kg of clean soil and 500 ml of petroleum ether were fully mixed, and prepared respectively into five petroleum hydrocarbons concentrations of polluted soil (6000 mg kg−1, 9000 mg kg−1, 12,000 mg kg−1, 15,000 mg kg−1, 18,000 mg kg−1), and clean soil was set aside as a control group. Plant seeds were soaked for 24 h before sowing. 110 g of soil was weighed in each Petri dish, and 20 seeds of each tested plant was arranged neatly in the Petri dish with a diameter of 12 mm. After seed germination, all the Petri dishes were moved into an artificial climate box with humidity set at 70%, temperature at 25°C, and light adjusted to 80 Lx. A total of eight herbs were tested, and each groups experiment was repeated three times with a total of 144 treatments. Water was added to the bed surface with a dropett to keep it wet. Seeds were germinated with obvious radicle cracking (white) and rotten seeds were removed soon as they were observed to be rotting on time. When no new germinated seeds appeared for three consecutive days, the germination process was regarded to have been completed. Finally, we counted the number of seeds germinated and calculated the germination rate.
Effects of glycine on petroleum hydrocarbons degradation in soil under different plant types conditions
Through the Petri dish germination test, three plants with strong resistance to petroleum hydrocarbons were initially screened, namely white clover, ryegrass, and sudan grass (Table 2). Air-dried clean soil was sieved (2 mm). A certain quality of petroleum was weighed and dissolved in petroleum ether. Each 1 kg of clean soil and 500 ml of petroleum ether was fully mixed, and respectively prepared into five petroleum hydrocarbons concentrations of polluted soil (6000 mg kg−1, 9000 mg kg−1, 12,000 mg kg−1, 15,000 mg kg−1, 18,000 mg kg−1). The contaminated soils were put in a cool environment for 15 days, and waited for the volatilization of petroleum ether.
A plastic flowerpot with a height of 15 cm and a diameter of 12 cm was selected, and each pot contained 700 g of soil. The plants with good germination were transplanted into the flowerpot, and 10 plants were transplanted into each pot. The flowerpots were placed randomly and the positions of the flowerpots were exchanged regularly to avoid the influence of light on plant growth. A total of seven treatments were set up in the experiment (Table 3). The blank control group was to continually water the sample without adding root exudates or planting plants. The phytoremediation group was white clover, ryegrass, and sudan grass. The root exudate group was white clover + glycine, ryegrass + glycine, and sudan grass + glycine. The concentration of glycine was 1000 mg L−1. In the root exudate group, each flowerpot had a glycine solution added to it once and watered once for each 2 days. In the blank control group and the phytoremediation group, no root exudate was added and water was poured once for every 2 days. After watering, the weight of the whole pot was made up to the initial range. The temperature was maintained at 20–25°C and the soil water content was kept above 60%. Three replicated experiments were performed for each treatment, with a total of 105 parallel samples. The pot experiment was carried out in the greenhouse of Yangtze University from 16 April 2021, to 26 June 2021, for a total of 71 days.
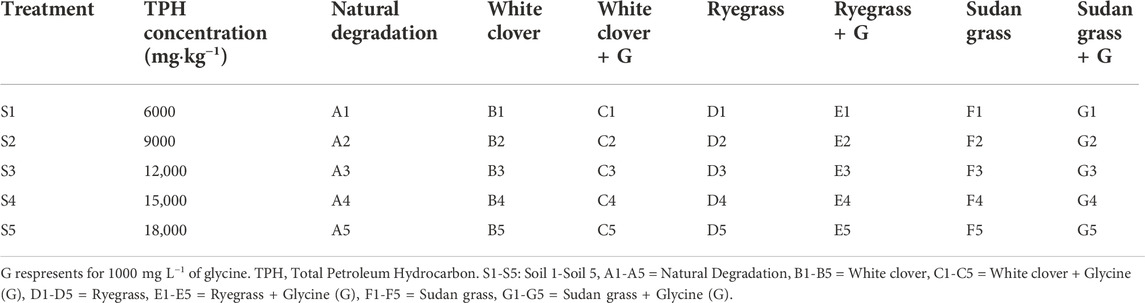
TABLE 3. Experimental design of glycine on petroleum hydrocarbons degradation in soil under different plant types conditions.
Effects of glycine on petroleum hydrocarbons degradation in soil under different soil conditions
Three soil types were set up in the experiment, namely sandy soil, loamy soil, and clay soil. The total petroleum hydrocarbons content of the soils was fixed at 12,000 mg kg−1 (in order to simplify the experiment, the average pollution concentration of 12,000 mg kg−1 obtained from the field investigation was taken as a representative concentration), and the three plants selected were white clover, ryegrass, and sudan grass, using glycine (1000 mg L−1) as the root exudate.
The sandy soil, loamy soil, and clay soil were separately placed on plastic film to a thickness of about 2 cm and were later placed in a cool place to dry naturally. A certain quality of petroleum was weighed and dissolved in petroleum ether. Each 1 kg of clean soil and 500 ml of petroleum ether was fully mixed, and respectively prepared into sandy soil, loamy soil, and clay soil with petroleum hydrocarbon content of 12,000 mg kg−1. The contaminated soils were put in a cool environment for 15 days, and waited for the volatilization of petroleum ether.
A plastic flowerpot with a height of 15 cm and a diameter of 12 cm were selected, and each pot contained 700 g of soil. The plants with good germination were transplanted into the flowerpot, and 10 plants were transplanted into each pot. Seven treatments were set in the pot experiment (Table 4). In the blank control group, the samples were only watered without adding root exudates. The phytoremediation group included white clover, ryegrass, and sudan grass. The root exudate group were white clover + glycine, ryegrass + glycine, and sudan grass + glycine. The concentration of glycine was 1000 mg L−1. In the root exudate group, each flowerpot had a glycine solution added once and watered once for each 2 days. In the blank control group and the phytoremediation group, no root exudate was added and water was poured once every 2 days. After watering, the weight of the whole pot was made up to the initial range. The temperature was maintained at 20–25°C and the soil water content was kept above 60%. Three replicates were performed for each treatment and 63 parallel experiments were conducted in total. The experiment lasted from 8 August 2021 to 18 September 2021, lasting a total of 71 days.

TABLE 4. Experimental design of glycine on petroleum hydrocarbons degradation in soil under different soil conditions.
Measurement and calculation of plant indicators
The germination number of seeds in contaminated soil is compared with the germination number of seeds in clean soil to calculate the relative germination rate of seeds under petroleum hydrocarbon pollution. The calculation formula is as follows:
After 71 days, the plant height and root length of white clover, ryegrass, and sudan grass were measured after harvesting the aboveground and underground parts of the three plants. Root dry weight and plant biomass were determined after drying.
Measurement and calculation of total petroleum hydrocarbons in soil
5 g of air-dried soil samples screened by 2 mm of sieve were accurately weighed and placed in a 50 ml centrifuge tube, followed by a 15 ml dichloromethane extractant, shaken, and mixed evenly. Then ultrasonic extraction was conducted for 20 min (min). After extraction, the centrifuge tube was put into a centrifuge and it was centrifuged at a 3000 r·min−1 for 10 min. After that, the tube was placed standing vertically, the liquid was separated, and the supernatant was poured into a triangle flask with constant weight. The above steps were repeated three times. All the supernatant was poured into the triangle bottle, which was finally put in a water bath at 60°C to steam petroleum ether. Thereafter, it was put in a constant temperature box of 60°C to bake for 1 h. Finally, it was weighed after being placed into the dryer to cool down for about 10 min. The calculation was done using the
Where, M1 is the mass of the triangle bottle, g; M2 is the total mass of the triangle bottle and TPHs, g; m is the mass of soil samples, g; P is the moisture content of soil samples, %.
Statistical analysis
The data were analyzed using IBM SPSS version 24.0 and Origin Pro 8.0. The presented data are means ± standard error of replicates. The differences between treatments for each experiment variant were compared using the one-way analysis of variance (ANOVA) followed by Duncan’s multiple range test at p ≤ 5% level. Petroleum hydrocarbons concentrations, soil types, glycine, and their interactions were analyzed by univariate multi-way ANOVA (p < 0.05).
Results and analysis
Results of the germination rate test
Different plant species had different responses to the same concentration of petroleum hydrocarbons pollution. Table 5 lists the seed germination rates of eight herbaceous plants under petroleum hydrocarbons pollution stress. The results showed that when the concentration of petroleum hydrocarbons in the test soil was 0 mg kg−1 (blank test), the seed germination rate of four plants exceeded 75%, among which ryegrass seed germination rate was the highest, reaching 90.8 ± 1.4%, followed by sudan grass seed germination rate at 80.7 ± 1.3%. The seed germination rates of tall fescue and white clover were 79.5 ± 1.9% and 76.2 ± 0.7%, respectively. The seed germination rates of canine root and maize grass were the lowest, which were only 32.3 ± 1.7% and 20.4 ± 0.8%, respectively. This indicates that there were problems in the germination rates of purchased canine root and maize grass seeds under the experimental conditions. If the seed germination rate of ryegrass was regarded as 100%, when the petroleum hydrocarbons concentration was 6000 mg kg−1, the seed germination rate of eight plants decreased by 12.2%–45.1% compared with that of clean soil. When the petroleum hydrocarbons concentration was 9000 mg kg−1, the germination rate decreased by 12.2%–38.7%. Further, when the petroleum hydrocarbons concentration increased to 12,000 mg kg−1, the germination rate decreased by 17.1%–8.3%. When the petroleum hydrocarbons concentration was 15,000 mg kg−1, the germination rate decreased by 20.0%–59.1%. When the petroleum hydrocarbons concentration continued to increase to 18,000 mg kg−1, the germination rate decreased by 22.6%–74.0%, indicating that the inhibition rate of seeds of all tested plants at a specific concentration was widely distributed, and there was a great difference in the tolerance of different herbaceous plants to petroleum hydrocarbons.
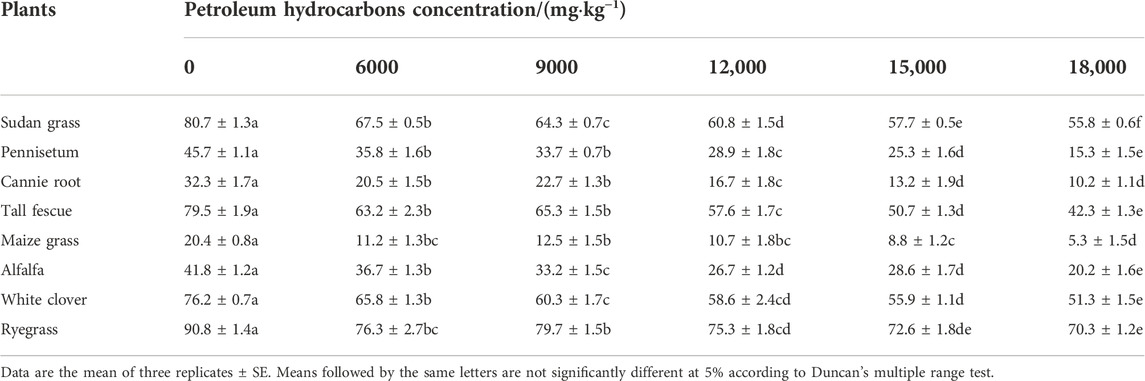
TABLE 5. Germination rate of eight plant seeds at different petroleum hydrocarbons concentrations (%).
Although most of the tested plants could not withstand high concentrations of petroleum hydrocarbon pollution, several plants showed strong tolerance to petroleum hydrocarbon pollution. In this experiment, ryegrass was least affected by petroleum hydrocarbons pollutants, and its germination rate did not change very much under the five petroleum hydrocarbons concentrations. If the germination rate of ryegrass seeds under blank conditions was regard as 100%, at petroleum hydrocarbons concentrations of 9000 mg kg−1, 12,000 mg kg−1, 15,000 mg kg−1, 18,000 mg kg−1, its germination rate decreased by 16.0%, 12.2%, 17.1%, 20.0%, and 22.6%, respectively. This was followed by sudan grass which was less affected by petroleum hydrocarbons pollutants. White clover and tall fescue showed strong resistance to petroleum hydrocarbons pollution, while other plants were significantly inhibited. The germination rate of maize grass decreased by 45.1%, 38.7%, 47.6%, 56.9%, and 74.0% compared with clean soil under five petroleum hydrocarbons concentrations, respectively.
Although the germination rate of seeds was correlated with the level of petroleum hydrocarbons pollution in soil, it still had a certain germination rate. As it can be seen from Figure 1, the seed germination rate of most tested plants was significantly affected by different concentrations of petroleum hydrocarbons. The seed germination rate decreased to different degrees with the increase of petroleum hydrocarbons concentration, showing an exponential relationship between them. The germination rates of ryegrass, sudan grass, and white clover were much higher than other plants in the range of tested pollutant levels. Ryegrass and sudan grass had strong seed germination ability even in 12,000 mg kg−1 petroleum hydrocarbons contaminated soil. The germination rate of them was about 19.3%–85.8% and 3.6%–82.4% higher than other plants under the same pollution condition, respectively.
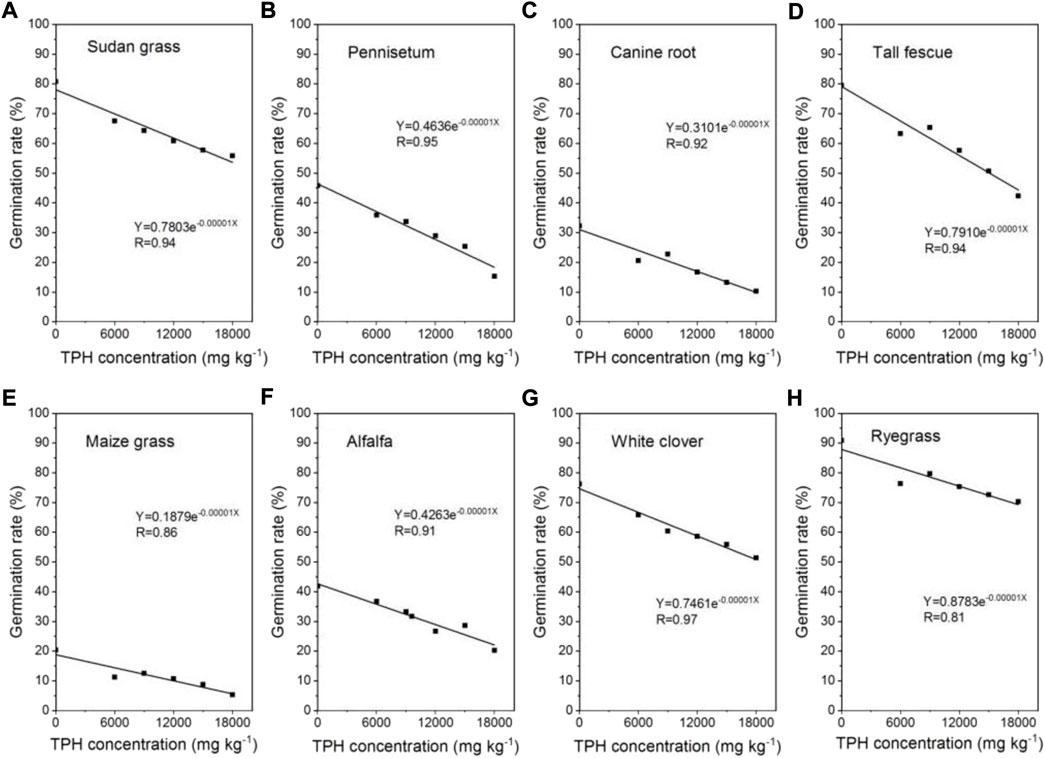
FIGURE 1. Correlation between germination rate of eight plant seeds and petroleum hydrocarbons concentrations.
The germination rates of ryegrass and sudan grass were 11.5%–85.3% and 2.5%–83.4% higher than those of other plants in 6000 mg kg−1 petroleum hydrocarbons contaminated soil, respectively. Under the petroleum hydrocarbons pollution level of 9000 mg kg−1, the germination rates of ryegrass and tall fescue were 18.1%–84.3% and 1.5%–80.9% higher than that of other plants, respectively. Under the condition of 15,000 mg kg−1 petroleum hydrocarbons pollution, the germination rates of sudan grass and white clover were 3.1%–84.7% and 9.3%–84.3% higher than those of other plants (excluding ryegrass), respectively. The germination rates of ryegrass, sudan grass and white clover were 20.6%–92.5%, 8.1%–90.5%, and 17.5%–89.7% higher than those of other plants in 18,000 mg kg−1 petroleum hydrocarbons contaminated soil, respectively. When the content of petroleum hydrocarbons in soil increased from 6000 mg kg−1–18,000 mg kg−1, the germination rate of ryegrass, sudan grass, and white clover decreased only by 7.9%, 17.3%, and 22.0%, respectively, indicating that the tolerance of ryegrass was higher than sudan grass and white clover.
Effects of glycine on petroleum hydrocarbons degradation in soil under different plant types conditions
Effects of different petroleum hydrocarbons concentrations and glycine on plant height
Figure 2 showed that, under the five pollution concentration levels, the plant heights of white clover, ryegrass, and sudan grass from small to large are: white clover < ryegrass < sudan grass. Compared with ryegrass and sudan grass, white clover had the smallest plant height, and its maximum plant height was only 9.4 ± 0.9 cm. When the petroleum hydrocarbons content in the soil was 6000 mg kg−1, the plant height of white clover was only 6.7 ± 0.8 cm. When the petroleum hydrocarbon content in the soil increased to 9000 mg kg−1 and 12000 mg kg−1, the plant height of white clover was 9.4 ± 0.9 cm and 6.3 ± 0.7 cm, respectively. This indicates that the lower concentration of petroleum hydrocarbons had a certain promotion effect on the plant height of white clover. When the petroleum hydrocarbons content in the soil continued to increase to 18,000 mg kg−1, the plant height of white clover was only 4.0 ± 0.4 cm, which was reduced by 2.7 cm compared with the white clover growing at the minimum petroleum hydrocarbons pollution level, indicating that the accumulation of petroleum hydrocarbons concentration in the soil to a certain extent would inhibit the growth of white clover. Compared with the white clover group, the plant height of the white clover with glycine even decreased to a certain extent, indicating that the addition of glycine did not directly promote the growth of white clover.
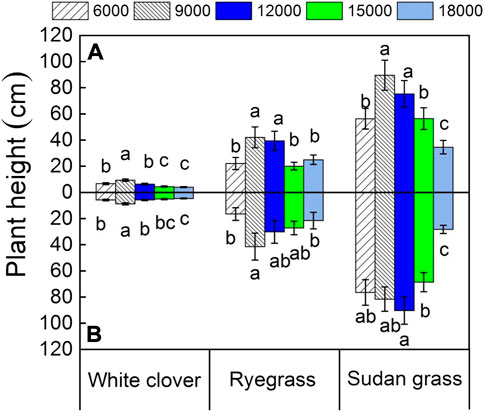
FIGURE 2. Plant height under different petroleum hydrocarbons concentrations. The values shown are the mean ± SE (n = 3). The same letters among specific treatments are not significantly different according to Duncan’s (0.05). Thus, (A): treatments without glycine; (B): treatments with glycine. 6000, 6000 mg kg−1 petroleum hydrocarbons contaminated soil; 9000, 9000 mg kg−1 petroleum hydrocarbons contaminated soil; 12,000, 12,000 mg kg−1 petroleum hydrocarbons contaminated soil; 15,000, 15,000 mg kg−1 petroleum hydrocarbons contaminated soil; 18,000, 18,000 mg kg−1 petroleum hydrocarbons contaminated soil.
When the petroleum hydrocarbons content in the soil was only 6000 mg kg−1, the plant height of ryegrass was 22.1 ± 4.6 cm. The plant height of ryegrass at the other four petroleum hydrocarbons concentrations was 42.1 ± 7.9 cm, 39.5 ± 7.3 cm, 20.1 ± 2.9 cm, and 25.0 ± 3.6 cm, respectively. It could be concluded that the growth of ryegrass was stimulated significantly when the petroleum hydrocarbons concentration was less than 18,000 mg kg−1. Among them, when the petroleum hydrocarbons concentration was between 9000 mg kg−1 ∼ 2000 mg kg−1, the ryegrass had the strongest excitation effect. Compared with the ryegrass group, the plant height of the ryegrass with glycine had no significant change under the five pollution levels, and the addition of glycine had no obvious effect on the growth of ryegrass.
As can be seen from Figure 2, the plant height of sudan grass is the largest. When the petroleum hydrocarbons content in the soil is 6000 mg kg−1, the plant height of sudan grass is 8.4 and 2.5 times greater than that of white clover and ryegrass, respectively, showing strong potential for growth and remediation of petroleum hydrocarbons contaminated soil. When the content of petroleum hydrocarbons in the soil increased to 9000 mg kg−1, 12,000 mg kg−1, and 15,000 mg kg−1, the plant height of sudan grass was 89.6 ± 11.5 cm, 75.3 ± 10.2 cm, and 56.4 ± 8.3 cm, respectively, it indicated that the growth of sudan grass was well promoted. However, when the petroleum hydrocarbons content in the soil increased to 18,000 mg kg−1, the plant height of sudan grass was only 34.6 ± 5.2 cm, indicating that the high petroleum hydrocarbons concentration had a strong inhibition effect on the plant height of sudan grass. At five petroleum hydrocarbons concentrations, compared to the sudan grass group, the plant height of sudan grass with glycine showed a certain degree of growth, indicating that glycine has promoting effect on the plant height of sudan grass.
Effects of different petroleum hydrocarbons concentrations and glycine on plant biomass
Figure 3 indicates that the effects of glycine addition and different petroleum hydrocarbons concentrations on the biomass of all tested plants showed significant differences. With the increase of petroleum hydrocarbons concentration, plant biomass first increased and then decreased. In the white clover group, when the petroleum hydrocarbons content in the contaminated soil was 6000 mg kg−1, its biomass was 0.56 ± 0.13 g and when the petroleum hydrocarbons content in the contaminated soil was 9000 mg kg−1, its biomass increased by 57.1%. In the white clover + glycine group, the biomass of white clover increased by 52.2% when the petroleum hydrocarbons content in soil increased from 6000 mg kg−1–9000 mg kg−1, indicating that 9000 mg kg−1 petroleum hydrocarbons contaminated soil could promote the biomass growth of white clover to a certain extent. When the petroleum hydrocarbons content in soil increased to 12,000 mg kg−1, 15,000 mg kg−1, 18,000 mg kg−1, the biomass of white clover was 0.37 ± 0.09 g, 0.30 ± 0.08 g, and 0.25 ± 0.06 g, respectively. Compared with the initial condition, the biomass decreased by 33.9%, 46.4%, and 55.4%, respectively. In the white clover + glycine group, the biomass decreased by 17.9%, 35.8%, and 43.3%, respectively, indicating that when the content of petroleum hydrocarbons in soil was in the range of 9000–18,000 mg kg−1, the biomass of white clover would be significantly inhibited. Comparing the biomass of white clover + glycine group with that of white clover group, the biomass of plants in the former group was larger than that in the white clover group, indicating that glycine addition could positively promote the biomass of white clover to a certain extent.
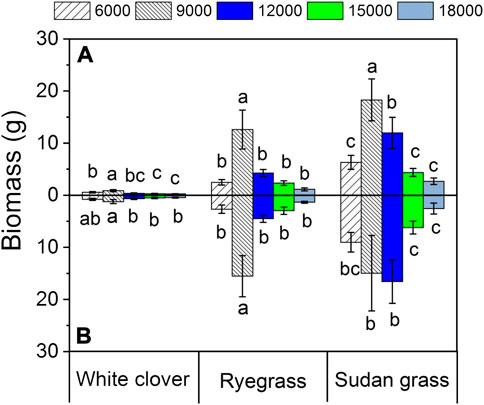
FIGURE 3. Biomass under different petroleum hydrocarbons concentrations. The values shown are the mean ± SE (n = 3). The same letters among specific treatments are not significantly different according to Duncan’s (0.05). Thus, (A): treatment without glycine; (B): treatment with glycine. 6000, 6000 mg kg−1 petroleum hydrocarbons contaminated soil; 9000, 9000 mg kg−1 petroleum hydrocarbons contaminated soil; 12,000, 12,000 mg kg−1 petroleum hydrocarbons contaminated soil; 15,000, 15,000 mg kg−1 petroleum hydrocarbons contaminated soil; 18,000, 18,000 mg kg−1 petroleum hydrocarbons contaminated soil.
In the ryegrass group, the biomass of ryegrass was 2.48 ± 0.52 g when the petroleum hydrocarbons content in the contaminated soil was 6000 mg kg−1, but when the petroleum hydrocarbons content in the contaminated soil increased from 6000 mg kg−1–9000 mg kg−1 and 12,000 mg kg−1, the biomass of ryegrass was 5.08 and 1.72 times that of the initial condition, respectively. In the ryegrass + glycine group, the biomass of ryegrass was 5.80 times and 1.68 times higher than under the initial condition, respectively, indicating that ryegrass would be strongly stimulated when growing in the soil contaminated by petroleum hydrocarbons in the range of 6000–12,000 mg kg−1. When the content of petroleum hydrocarbons in the contaminated soil was 15,000 mg kg−1, the biomass of ryegrass in the ryegrass group was 94.4% of the initial condition, and that of the ryegrass + glycine group was 111.2% of the initial condition. There was no significant change in the biomass of the two groups, indicating that the higher concentration of petroleum hydrocarbons contaminated soil had no discernible effect on ryegrass biomass. When the petroleum hydrocarbons content in the contaminated soil increased to 18,000 mg kg−1, the plant biomass in the ryegrass group was 1.14 ± 0.29 g. Compared with the initial condition, the mass of biomass decreased by 54.0%. The biomass of ryegrass in the ryegrass + glycine group was also 49.8% lower than that in the initial condition, indicating that the high concentration of petroleum hydrocarbons contaminated soil could inhibit the plant biomass of ryegrass. Comparing the plant biomass of the ryegrass + glycine group with that of the ryegrass group, it was found that the biomass difference between the two groups was almost negligible. Therefore, glycine addition had no significant effect on the plant biomass of ryegrass.
In the sudan grass formation, the biomass of sudan grass was 6.31 ± 1.34 g when the petroleum hydrocarbons content in the contaminated soil was 6000 mg kg−1, but when the petroleum hydrocarbons content in the contaminated soil increased from 6000 mg kg−1–9000 mg kg−1 and 12,000 mg kg−1, its biomass increased 190.0% and 89.7%, respectively, compared with the initial condition. The biomass of sudan grass + glycine group increased by 198.8% and 83.8%, respectively, compared with the initial condition, indicating that the biomass of sudan grass growing in the petroleum hydrocarbons contaminated soil of 6000–12,000 mg kg−1 could be significantly promoted. When the content of petroleum hydrocarbons in contaminated soil increased from 15,000 mg kg−1–18,000 mg kg−1, the plant biomass of sudan grass group and sudan grass + glycine groups decreased to a large extent, indicating that a high concentration of petroleum hydrocarbons had a certain reverse inhibition effect on the plant biomass of sudan grass. Comparing the plant biomass of sudan grass + glycine group with that of sudan grass group, it was found that the plant biomass of sudan grass under five pollution levels increased significantly, indicating that glycine addition had a significant positive effect on the biomass of sudan grass.
Effects of glycine on degradation rate of total petroleum hydrocarbons in soil under different petroleum hydrocarbons concentrations
According to Figure 4, when the petroleum hydrocarbons content in the contaminated soil was 6000 mg kg−1, the degradation rate of TPHs in the soil of the blank control group was 35.43 ± 2.93%, after 71 days. After 71 days treatment with white clover, the degradation rate of TPH in soil was 41.56 ± 3.84%, which was 17.3% higher than that under blank conditions. When 1000 mg kg−1 of glycine was added to the white clover pot, the degradation rate of TPHS in the soil was 42.93 ± 3.17% after 71 days of repair, which was only 3.3% higher than that of white clover. When the petroleum hydrocarbons content in contaminated soil was 9000 mg kg−1, the degradation rate of white clover and white clover with glycine was 1.18 times and 1.24 times higher than that of the blank control group, respectively. When the petroleum hydrocarbons content in contaminated soil was 12,000 mg kg−1, the degradation rate of the blank control group, phytoremediation group, and root exudate group decreased by 10.0%, 14.7%, and 7.6%, respectively, compared with the degradation rate of petroleum hydrocarbons content of 6000 mg kg−1. When the petroleum hydrocarbons content in the contaminated soil increased to 18000 mg kg−1, the degradation rate of TPHS in the blank control soil was 20.39 ± 2.39%. The degradation rate of TPHs in the white clover group was 29.34 ± 4.38%, which was 30.5% higher than that in the blank condition. The degradation rate of TPHs in the white clover + glycine group was 31.46 ± 4.67%, which was 7.2% higher than that in the white clover group. The results showed that although petroleum-contaminated soil had a certain degree of natural degradation ability, the bioremediation effect of plants and glycine was more significant.
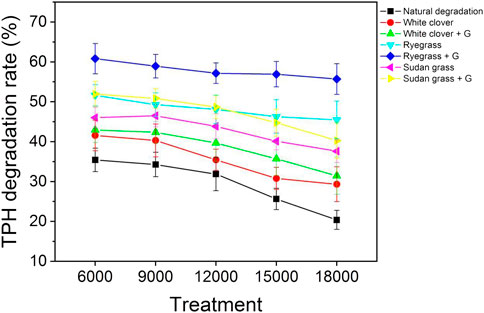
FIGURE 4. Degradation rate of total petroleum hydrocarbons in soil under blank control, plants, and plants with glycine (%).
As can be seen from Figure 4, when the petroleum hydrocarbons content in the contaminated soil was only 6000 mg kg−1, the degradation rate of TPHs in the soil was 51.63 ± 2.67% after 71 days of ryegrass restoration, which was 24.2% higher than that of white clover cultivation. After adding glycine to ryegrass for 71 days, the degradation rate of TPHs in soil was 60.82 ± 3.78%, which is 17.8% higher than that of the ryegrass group. When the petroleum hydrocarbons content in contaminated soil was 12,000 mg kg−1, the degradation rate of ryegrass and ryegrass with glycine was 1.51 and 1.79 times higher than that of the blank control group, respectively. When the petroleum hydrocarbons content in contaminated soil increased to 15,000 mg kg−1, the degradation rate of the ryegrass group and ryegrass group with glycine increased by 50.3% and 59.4% compared with the white clover group and white clover group with glycine, respectively. When the petroleum hydrocarbons content in the contaminated soil was 18,000 mg kg−1, the degradation rate of TPHs in the soil was 45.47 ± 4.69% after 71 days of ryegrass restoration. Furthermore, the degradation rate of TPHs in soil was 55.72 ± 3.85% after 71days restoration by adding glycine to ryegrass. The results showed that the repair ability of ryegrass was much better than that of white clover, and the repair efficiency of ryegrass and glycine was higher than that of white clover and glycine.
When the petroleum hydrocarbons content in the contaminated soil was 6000 mg kg−1, the degradation rate of TPHs in the soil was 46.03 ± 2.63% after 71 days of sudan grass restoration, which was 1.11 times and 0.89 times higher than that of white clover and ryegrass, respectively. After 71 days treatment with glycine, the degradation rate of TPHs in soil was 51.97 ± 3.15%, which was 1.21 times and 0.85 times higher than that of the white clover and ryegrass with glycine, respectively. When the petroleum hydrocarbons content of the contaminated soil was 9000 mg kg−1, the degradation rate of sudan grass restoration group and sudan grass with glycine had little change. When the petroleum hydrocarbons content in contaminated soil was 12,000 mg kg−1, the degradation rate of TPHs in the plant group and root exudate group was 1.38 and 1.53 times higher than that in the blank control group, respectively. When the petroleum hydrocarbons content in the contaminated soil increased to 18,000 mg kg−1, the degradation rate of TPHs in the soil was 37.62 ± 2.85% after 71 days of sudan grass treatment, and 40.27 ± 4.48% after 71 days of glycine treatment, compared with the soil with petroleum hydrocarbons content of 6000 mg kg−1, the degradation rate decreased by 18.3% and 22.5%, respectively. The results showed that the repair ability of sudan grass to total petroleum hydrocarbons was stronger than that of white clover and lower than that of ryegrass. The joint action ability of sudan grass and glycine was weaker than that of ryegrass and glycine, but stronger than that of white clover and glycine.
Analysis of petroleum hydrocarbons concentrations, glycine addition and their interaction
Analysis of petroleum hydrocarbons concentrations, glycine addition and their interaction (Table 6) showed that petroleum hydrocarbon concentrations had significant influence on biomass, root length, root dry weight and TPHs degradation rate of the three plants. Adding glycine significantly impacted degradation rate of TPHs of all the plant species. Petroleum hydrocarbons concentrations and its interaction with glycine had no significant effect on plant indicators.
Effects of glycine on petroleum hydrocarbons degradation under different soil types
Effects of glycine on plant height under different soil types
It can be seen from Figure 5 that, under these three soil conditions, white clover, ryegrass, and sudan grass all had the maximum plant height in loamy soil, followed by sandy soil, and finally clay soil. Under the same soil conditions, 1000 mg L−1 of glycine promoted the plant height of sudan grass to a certain extent.
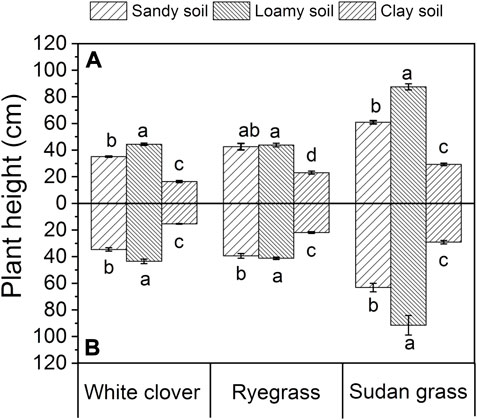
FIGURE 5. Plant height of plants under different soil conditions. The values shown are the mean ± SE (n = 3). The same letters among specific treatments are not significantly different according to Duncan’s (0.05). (A): treatment without glycine; (B): treatment with glycine.
The plant height of white clover, ryegrass and sudan grass were 16.4 cm, 23.1 cm, and 29.3 cm in clay soil, those are 44.4 cm, 43.8 cm, and 87.5 cm in loamy soil, while 35.1 cm, 42.6 cm, and 60.9 cm in sandy soil, respectively. When the growth medium changed from loamy soil to sandy soil, the plant height of white clover, ryegrass, and sudan grass decreased by 20.9%, 2.7%, and 30.4%, respectively. Compared with the sharp decrease of sudan grass and white clover, the plant height of ryegrass was very stable and almost did not decrease.
After adding glycine, the plant heights of white clover in sandy soil, loamy soil, and clay soil were 34.6 cm, 43.5 cm, and 15.4 cm, respectively. The plant heights of ryegrass in sandy soil, loamy soil, and clay soil were 39.4 cm, 41.2 cm, and 21.9 cm, respectively. Under these three soil conditions, the plant heights of white clover and ryegrass did not change significantly as compared with those before glycine addition. The plant heights of sudan grass in sandy soil and loamy soil increased by 4.9% and 4.4%, respectively, as compared with those before glycine addition, and the plant height in clay soil almost remained unchanged.
Effects of glycine on plant biomass under different soil types
It can be seen from Figure 6 that under the three soil types, the biomass of white clover, ryegrass, and sudan grass has a significant difference, and the order of plant biomass in the three soils is sandy soil < clay soil < loamy soil. Loamy soil was suitable for the growth of all three kinds of plants. 1000 mg L−1 of glycine significantly promoted the fresh weight of white clover.
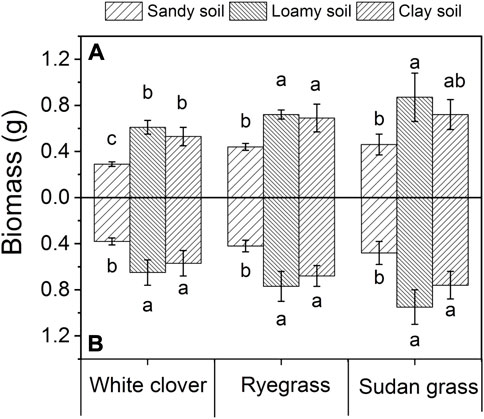
FIGURE 6. Plant biomass under different soil conditions. The values shown are the mean ± SE (n = 3). The same letters among specific treatments are not significantly different according to Duncan’s (0.05). Thus, (A): treatment without glycine; (B): treatment with glycine.
The biomass of white clover in sandy soil and clay soil was 0.29 and 0.53 g respectively, while the maximum biomass in loamy soil was 0.61 g. Similarly, the biomass of ryegrass in sandy soil was 0.44 g and that in clay soil was 0.69 g. The biomass of ryegrass increased by 56.8%, while the biomass of ryegrass in loamy soil reached the maximum value of 0.72 g which increased by 63.6% compared with that in sandy soil. The biomass of sudan grass in sandy soil was 0.46 g that in clay soil was 0.72 g and that in loamy soil was 0.87 g the maximum of the three treatments.
After adding glycine, the biomass of white clover in sandy soil was 0.38 g significantly increased by 31% compared with that before adding glycine. The biomass in clay soil and loamy soil was 0.57 g and 0.65 g respectively, significantly increased by 5.7% and 6.6% compared with that before glycine addition, respectively. Similarly, the biomass of ryegrass in sandy soil was 0.42 g and that in clay soil was 0.68 g. The biomass of ryegrass increased by 61.9%, while the biomass of ryegrass in loamy soil reached the maximum value of 0.77 g which increased by 13.2% as compared with that in clay soil. The biomass of sudan grass in sandy soil was 0.48 g, that in clay soil was 0.76 g, and that in loamy soil was 0.95 g the maximum of the three treatments. This indicates that loamy soil is the suitable soil type for the growth of all three plants, followed by clay soil, and finally sandy soil. Under the three soil conditions, there was no significant difference in biomass of ryegrass and sudan grass before and after adding glycine.
Effects of glycine on petroleum hydrocarbons degradation rate under different soil types
Figure 7 shows that among the three types of soils, sandy soil performs the best, loamy soil is the second, and clay soil is the worst. The natural degradation rates of sandy soil, loamy soil, and clay soil were 38.26%, 29.75%, and 12.13%, respectively. In sandy soil, white clover, ryegrass, and sudan grass could effectively remove total petroleum hydrocarbons, with degradation rates of 50.36%, 54.17%, and 50.59%, respectively. In loamy soil, the degradation rates of white clover, ryegrass, and sudan grass were 41.07%, 52.65%, and 34.42%, respectively, which were 0.68–0.97 times higher than that of sandy soil. In clay soil, the degradation rates of white clover, ryegrass, and sudan grass ranged from 14.13% to 26.53%, which was 0.28–0.49 times higher than that of sandy soil. After adding glycine, the degradation rates of white clover, ryegrass, and sudan grass to petroleum hydrocarbons in sandy soil increased to 55.27%, 59.36%, and 52.78%, respectively. In loamy soil, the degradation rates of white clover, ryegrass, and sudan grass reached 43.65%, 53.28%, and 37.36%, which were 0.71–0.9 times of those in sandy soil. In clay soil, the degradation rates of white clover, ryegrass, and Sudan grass reached 13.93%–31.02%, which was 0.26–0.52 times of those in sandy soil. The results indicated that sandy soil was suitable for petroleum hydrocarbon-contaminated soil remediation by all three plants.
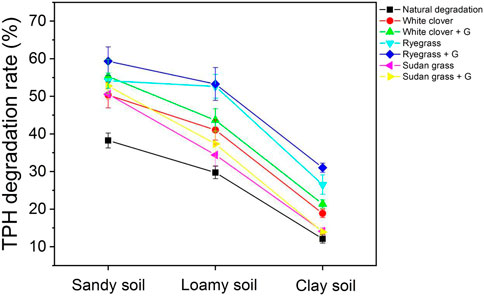
FIGURE 7. Degradation rate of total petroleum hydrocarbons under different soil conditions. G represents for 1000 mg kg−1 of glycine.
By comparing the degradation rates of petroleum hydrocarbons in the blank control group, phytoremediation group, and root exudate remediation group under three soil conditions, it can be seen that in sandy soil, the degradation rates of petroleum hydrocarbons in white clover, ryegrass and sudan grass in root exudate group were 1.38–1.68 times and 1.05–1.1 times respectively as those in blank control group and phytoremediation group. In loamy soil, the degradation rates of white clover, ryegrass, and sudan grass in the root exudate group were 1.26–1.79 times of that in the blank control group and 1.01–1.09 times of that in the phytoremediation group, respectively. In clay soil, the degradation rates of white clover, ryegrass, and sudan grass in the root exudate group were 1.15–2.56 times of that in the blank control group and 0.99–1.17 times of that in the phytoremediation group, respectively, indicating that glycine can effectively promote the degradation of petroleum hydrocarbon pollutants in soil.
Analysis of soil types, glycine addition and their interaction
Analysis of soil types, glycine addition and their interaction (Table 7) showed that soil types had significant influence on biomass, plant height, and TPHs degradation rate of all the plant species. Glycine and its interaction with soil types had no significant effect on plant indicators.
Discussion
Seed germination and early growth are critical developmental stages in plant life cycle. As the starting point of plant life cycle, the germination process can first feel the stress of adversity and is very sensitive to environmental factors such as oil content (Seddighinia et al., 2020). In this experiment, the seed germination ability of eight plants was found to have decreased in petroleum-contaminated soil, which is consistent with other studies’ findings that petroleum hydrocarbons pollution significantly decreased the germination ability of Festuca Arundinacea L., Lolium perenne L., Medicago, Oryza sativa L., Zea Mays L. and wheat (Wei et al., 2014; Afegbua and Batty, 2018). One possible reason for the inhibitory effect of petroleum on seed germination is the high hydrophobicity of petroleum compounds. The oil film around the seed can act as a physical barrier, preventing or reducing water and oxygen from entering the seed and thus inhibiting germination. In addition, oil is a complex combination of hydrocarbons, in which high concentrations of volatile compounds and aliphatic compounds may be responsible for its higher toxicity (Haider et al., 2021). The findings of this experiments showed that ryegrass, sudan grass, white clover, and tall fescue had strong resistance to petroleum hydrocarbon pollution. These results are in agreement with the results of Smith et al. (2006) which also showed that PHS pollution had no significant adverse effects on seed germination of tall fescue, perennial ryegrass, Dactylis glomerata L., Festuca Rubra L., Trifolium repens L., Trifolium pratense L., and Lotus Corniculatus L. This could further be explained with the fact that seeds have a major line of defense that prevents petroleum oil from penetrating the seed coat and reducing embryo growth activity. The integrity and hardness of the seed coat affect the rate of oil penetration (Amakiri and Onofeghara, 1984), and tissue penetration is a prerequisite for oil damage to embryos. The seed coat that is resistant to oil infiltration is almost unaffected and thus exhibits strong resistance (Haider et al., 2021).
Plants grown in PHs contaminated soils showed various abnormalities in their growth and metabolic indices, including decreased chlorophyll content, increased starch metabolic enzymes, antioxidant activities, production of total protein, carbohydrates, and amino acids, as well as significant decreases in overall productivity and plant yield (Achuba and Ja-anni, 2018; Odukoya et al., 2019). Adieze et al. (2012) reported that maize plant height and plant biomass were reduced by 6.4 and 21.9%, respectively, after soil was contaminated by 1% W/W crude oil. While, Panicum maximum L. Tropical plants such as Centrosema Angustifolium L. and Centrosema Angustifolium L. also showed significant reductions in plant height and crop biomass. The toxicity of 10% diesel in soil reduced the biomass of sorghum and pearl millet by 67.0 and 74.0%, respectively (Luhach and Chaudhry, 2012). In this experiment, the biomass of the three plants increased greatly in the soil contaminated by petroleum hydrocarbon at a lower concentration, and the plants were stimulated by petroleum hydrocarbon contaminated soil. When petroleum hydrocarbon contaminated soil increased to 12,000 mg kg−1, the plant growth was quickly inhibited. The irritating effect of petroleum compounds may be due to the decomposition of hydrocarbons by microorganisms such as bacteria, as other scholars have stated it (Odukoya et al., 2019). Other possible causes of plant growth promotion may be the release of nutrients from oil, or the effects of hormones such as petroleum auxin (naphthenic acid), which increase the yield of various crops by stimulating photosynthesis and protein-nitrogen in plants (Adieze et al., 2012). However, the negative effects of oil pollution on plant root nutrient acquisition and associated microbial activity may result in poor growth of plant seedlings in contaminated soils due to increased concentrations of hydrocarbon pollution (Baoune et al., 2018). Due to the toxicity and hydrophobicity of low molecular weight hydrocarbons, oily overburden on contaminated soil surfaces prevents water from penetrating the soil layer, and lack of water affects leaf growth, seedling development height, and root elongation. Studies on the growth of gramineous and leguminous plants in oil-contaminated soils have shown that rhizobium nodulation has considerable inhibition (Haider et al., 2021). The lack of Rhizobium nodules reduces nutrient availability and results in lower seedling growth and development. In addition, a decrease in the stomatal index in leaves, amylase, and starch phosphorylase activity in seedling cotyledon, and a decrease in meristem mitosis activity and root size with an increase in soil crude oil concentration may also contribute to poor seedling growth in oil-contaminated soil (Tran et al., 2018).
Existing studies confirm that various types of plants including legumes and grasses are successful in removing organic soil pollutants (Asghar et al., 2017). PHs levels in various soils planted with ryegrass were significantly reduced—up to 20% of the initial PHs concentration (Pawlik et al., 2020). Vitiveria Zizanioides L. effectively removing polycyclic aromatic hydrocarbons (PAHs) and diesel oil from contaminated sediments in India and Pakistan (Nisa et al., 2015). Poaceae and Fabaceae resulted in remarkable remediation potential for TPH-contaminated soil (Zuzolo et al., 2021). In phytoremediation of organic pollutants, they are removed through different pathways: plant degradation, plant volatilization, plant extraction and rhizosphere degradation (Ossai et al., 2020). There are three main mechanisms for plants to remove petroleum hydrocarbons pollutants from soil: 1) direct absorption and degradation by plants; 2) By releasing secretions and enzymes, plants can stimulate and enhance the activity of microorganisms in the root zone and enhance biotransformation; 3) Plants enhance mineralization in the root region. Plant absorption is the result that plants absorb petroleum pollutants directly from the soil through metabolic activities and accumulate these harmful substances in some parts of themselves without having lethal toxic effects on themselves. Plant degradation refers to the conversion of pollutants into less toxic or non-phytotoxic metabolites by plants through metabolic action (Ite and Ibok. 2019). It takes only 2–3 days for gramineous plants to degrade phenylene and xylene, 4–5 days for maize plants, and 5–6 days for root crops (Janse 2005). The enzymes secreted from plant roots to the rhizosphere can directly participate in the biochemical process of degradation of petroleum hydrocarbon pollutants. Studies have shown that nitrate reductase and laccase can degrade trinitrotoluene, and dehalogenase can degrade trichloroethylene to produce chloride ions, water, and carbon dioxide (Kiamarsi et al., 2020). Root exudates are released by different parts of biologically active plant roots. The active period of root cap tissue is short and easy to dissociate, and the golgi apparatus in cells is easy to release a large amount of mucus, so it is easy to secrete a large amount of mucinous substances. Root secretion is an adaptive response of plant roots to environmental changes. For specific exudates, it is usually induced by nutrient stress and is an active response (Stassen et al., 2021). When a specific matrix is lacking and causes stress to the plant, the plant will synthesize this matrix through its regulation and secrete it into the soil through the root system. This characteristic substance can promote the activation of the deficient nutrients and improve the utilization efficiency of the plant, to alleviate the stress of this substance. However, most of the non-specific root exudates are considered to be passive secretions caused by the destruction of plant plasma membrane structure (Scavo et al., 2019). Whether active or passive secretion is dominant in plants often depends on the environmental conditions of the rhizosphere. Generally healthy plants also have high root secretion activity, which peaks at the peak of plant life activity and then declines (Gregory 2008). Amino acids in root exudates provide carbon and nitrogen sources for microbial growth and induce the formation of mycorrhizal signaling substances (Canarini et al., 2019). In this study, glycine on plant biomass showed the promoting effect of plant height, and the mechanism may be adding glycine is absorbed by plant roots of microorganisms, microorganisms have enough nutrients after a large number of growth and breeding has carried on the adjustment to root zone of the surrounding environment optimization, to promote the growth of plants. In addition, the diversity and specificity of root exudates result in a large number of microorganisms, such as bacteria and fungi, gathering in the root region. These microorganisms are extremely active due to the activation of root exudates, and they degrade or partially degrade organic pollutants by using them as energy or by co-metabolizing with root exudates (Vergani et al., 2017). Root exudates themselves can also react with organic or inorganic pollutants by oxidation, reduction, complexation, or chelation, thus promoting the degradation, transformation, or fixation of pollutants (Ye et al., 2017).
Soil physical structure and chemical conditions can be changed by plants and their roots, thus affecting the degradation effect. The organic matter required by the soil is provided by the shedding of root cap cells and the secretion of mucinous gel. Organic matter can reduce the bioavailability (the degree to which pollutants interact with living organisms) of some petroleum hydrocarbons, especially the lipid solubility and compounds bound to organic matter (Cunningham et al., 1996). Due to its limited density, low emulsifying potential, and high viscosity, toxic compounds in petroleum can easily penetrate the soil surface and affect the porosity and permeability of soil (Ossai et al., 2020). Organic pollutants such as PHs are rich in high carbon (C) ratio and limited nitrogen (N), so they can significantly change soil organic matter composition and structure, and affect soil C/N ratio, C/P ratio, pH, electrical conductivity (EC) and soil salinity (Farouq et al., 2022). Additionally, PHs pollution leads to anaerobic conditions in the soil by blocking air diffusion and suffocating soil particles in the porous structure of the soil, and ultimately affects the soil microbial community (Devatha et al., 2019). Terzaghi et al. (2017) reported that the absorption of organic pollutants by plants from contaminated soil is affected by a series of abiotic factors, such as clay, iron oxide, and dead organic matter content. In this study, sandy soil had high sand content, mainly quartz, few nutrients, and poor fertility retention. It is difficult for plants to obtain effective nutrients conducive to their growth during the growth period, resulting in slow growth and small biomass. Loamy soil is characterized by a uniform distribution of various components, good nutrients, good aeration, and strong water retention ability. It is very suitable for plant growth, with rapid plant growth and large biomass. The plant heights of white clover, ryegrass, and sudan grass are all small in clay soil, which may be due to poor aeration in clay soil, slow decomposition of organic matter, and poor water permeability in soil, making it difficult for plants to absorb nutrients and water. Amellal et al. (2001) studied the interaction between soil particle size, contamination, and the functionality of microorganisms and pointed out that the sandy texture soils showed higher microorganism populations and activity. Huesemann et al. (2004) also demonstrated that soils with low percentages of fine silt and clay showed higher degradation rates of hydrocarbons. In this study, the three plants have the highest degradation rates of petroleum hydrocarbons in sandy soil, which may be because sandy soil has a loose soil structure, coarse soil particles, and good soil aeration. Microorganisms in plant roots can easily obtain oxygen and energy in sandy soil, so they can quickly remove a large number of organic pollutants in the soil. In addition, sandy soil is more porous, and when watering plants, some of the total petroleum hydrocarbons may be lost with rain. The soil gap in clay soil is very small, and the aqueous solution of root exudates can hardly flow to the root surface of plants through the pores, so the use of glycine is of limited help to the degradation of total petroleum hydrocarbons.
Conclusion
The seeds of ryegrass, sudan grass and white clover still have strong vitality under the stress of petroleum hydrocarbons pollution, demonstrating the potential of phytoremediation of petroleum contaminated soil. Ryegrass had good adaptability to petroleum hydrocarbons-contaminated soil. Sudan grass and white clover were more affected by petroleum hydrocarbons-contaminated soil. Ryegrass had a more significant effect on the remediation of petroleum hydrocarbons after adding glycine, and its degradation rates were 60.82%, 57.17%, and 55.72% when the concentration of petroleum hydrocarbons was 6000 mg kg−1, 12,000 mg kg−1, and 18,000 mg kg−1, respectively. Loamy soil is suitable for the growth of three kinds of plants. Under the same soil condition, 1000 mg L−1 of glycine has a great influence on the degradation rate of plants. Through the phytoremediation experiment with root exudates, it was found that white clover, ryegrass, and sudan grass had a strong degradation efficiency of total petroleum hydrocarbons in sandy soil. Ryegrass was preferentially planted for remediation in oil fields polluted by petroleum hydrocarbons.
Data availability statement
The original contributions presented in the study are included in the article/Supplementary Material, further inquiries can be directed to the corresponding author.
Author contributions
All authors contributed to the study conception and design. Conceptualization, methodology, and writing of the original draft: MH, Review, supervision: PM and ZL; Data curation: MH; Visualization and editing: MH; All authors revised the manuscript and approved the final version.
Funding
This work was funded by the “13th five-year” National Major Science and Technology Project of China (grant no. 2016ZX05040-002).
Conflict of interest
The authors declare that the research was conducted in the absence of any commercial or financial relationships that could be construed as a potential conflict of interest.
Publisher’s note
All claims expressed in this article are solely those of the authors and do not necessarily represent those of their affiliated organizations, or those of the publisher, the editors and the reviewers. Any product that may be evaluated in this article, or claim that may be made by its manufacturer, is not guaranteed or endorsed by the publisher.
References
Achuba, F. I., and Ja-anni, M. O. (2018). Effect of abattoir waste water on metabolic and antioxidant profiles of cowpea seedlings grown in crude oil contaminated soil. Int. J. Recycl. Org. Waste Agric. 7, 59–66. doi:10.1007/s40093-017-0190-6
Adieze, I. E., Orji, J. C., Nwabueze, R. N., and Onyeze, G. O. C. (2012). Hydrocarbon stress response of four tropical plants in weathered crude oil contaminated soil in microcosms. Int. J. Environ. Stud. 69, 490–500. doi:10.1080/00207233.2012.665785
Afegbua, S. L., and Batty, L. C. (2018). Effect of single and mixed polycyclic aromatic hydrocarbon contamination on plant biomass yield and PAH dissipation during phytoremediation. Environ. Sci. Pollut. Res. 25, 18596–18603. doi:10.1007/s11356-018-1987-1
Al-Mansoory, A. F., Idris, M., Abdullah, S. R. S., and Anuar, N. (2017). Phytoremediation of contaminated soils containing gasoline using Ludwigia octovalvis (Jacq.) in greenhouse pots. Environ. Sci. Pollut. Res. 24, 11998–12008. doi:10.1007/s11356-015-5261-5
Amakiri, J. O., and Onofeghara, F. A. (1984). Effects of crude oil pollution on the germination of Zea mays and Capsicum frutescens. Environ. Pollut. Series A, Ecol. and Biol. 35 (2), 159–167. doi:10.1016/0143-1471(84)90136-3
Amellal, N., Portal, J. M., Vogel, T., and Berthelin, J. (2001). Distribution and location of polycyclic aromatic hydrocarbons (PAHs) and PAH degrading bacteria within polluted soil aggregates. Biodegradation 12, 49–57. doi:10.1023/A:1011909107858
Asghar, H. N., Rafique, H. M., Khan, M. Y., and Zahir, Z. A. (2017). Phytoremediation of light crude oil by maize (Zea mays L.) bio-augmented with plant growth promoting bacteria. Soil Sediment. Contam. Int. J. 26 (7-8), 749–763. doi:10.1080/15320383.2017.1414771
Baoune, H., El Hadj-Khelil, A. O., Pucci, G., Sineli, P., Loucif, L., and Polti, M. A. (2018). Petroleum degradation by endophytic Streptomyces spp. isolated from plants grown in contaminated soil of southern Algeria. Ecotoxicol. Environ. Saf. 147, 602–609. doi:10.1016/j.ecoenv.2017.09.013
Binet, P., Portal, J. M., and Leyval, C. (2000). Dissipation of 3~6-ring polycyclic aromatic hydrocarbons in the rhizosphere of ryegrass. Soil Biol. Biochem. 32, 2011–2017. doi:10.1016/S0038-0717(00)00100-0
Bing (2022a). 1827128500.jpg (JPEG Image, 1280 × 960 pixels) — scaled (71%). from: http://g1010.jinnong.cn/a/20210301/427912/1827128500.jpg (Retrieved September 23, 2022).
Bing (2022b). 20180310225330_53650.jpg (JPEG Image, 768 × 686 pixels) — scaled (99%). from: http://cdn055.yun-img.com/static/upload/duoyouqi/team/20180310225330_53650.jpg (Retrieved September 23, 2022).
Bing (2022c). ccvy1y5bsxj.jpg (JPEG Image, 650 × 420 pixels). from: https://res.nyw58.com/img/2020/x041514/ccvy1y5bsxj.jpg (Retrieved September 23, 2022).
Canarini, A., Kaiser, C., Merchant, A., Richter, A., and Wanek, W. (2019). Root exudation of primary metabolites: mechanisms and their roles in plant responses to environmental stimuli. Front. Plant Sci. 10, 157. doi:10.3389/fpls.2019.00157
Cunningham, S. D., Anderson, T. A., Schwab, A. P., and Hsu, F. C. (1996). Phytoremediation of soils contaminated with organic pollutants. Adv. Agron. 56 (1), 503–516. doi:10.1002/9780470944479.ch20
Devatha, C. P., Vishnu Vishal, A., and Purna, C. R. J. (2019). Investigation of physical and chemical characteristics on soil due to crude oil contamination and its remediation. Appl. Water Sci. 9, 89. doi:10.1007/s13201-019-0970-4
Euliss, K., Ho, C. H., Schwab, A. P., Rock, S., and Banks, M. K. (2008). Greenhouse and field assessment of phytoremediation for petroleum contaminants in a riparian zone. Bioresour. Technol. 99, 1961–1971. doi:10.1016/j.biortech.2007.03.055
Eze, M. O., Thiel, V., Hose, G. C., George, S. C., and Daniel, R. (2022). Enhancing rhizoremediation of petroleum hydrocarbons through bioaugmentation with a plant growth-promoting bacterial consortium. Chemosphere 289, 133143. doi:10.1016/j.chemosphere.2021.133143
Farouq, A. A., Ismail, H. Y., Rabah, A. B., Muhammad, A. B., Ibrahim, U. B., and Fardami, A. Y. (2022). Cowpea induced physicochemical and biological rhizosphere changes in hydrocarbon contaminated soil. Plant Soil 477, 759–777. doi:10.1007/s11104-022-05460-y
Gerhardt, K. E., Huan, X-D., Glick, B. R., and Greenberg, B. M. (2009). Phytoremediation and rhizoremediation of organic soil contaminants: potential and challenges. Plant Sci. 176, 20–30. doi:10.1016/j.plantsci.2008.09.014
Gregory, P. J. (2008). Plant roots: growth, activity and interactions with the soil. Oxford: John Wiley & Sons.
Haider, F. U., Ejaz, M., Cheema, S. A., Khan, M. I., Zhao, B., Liqun, C., et al. (2021). Phytotoxicity of petroleum hydrocarbons: Sources, impacts and remediation strategies. Environ. Res. 197, 111031. doi:10.1016/j.envres.2021.111031
Hassan, A., and Nawchoo, I. A. (2022). Rhizosphere improvement role of biotechnology and microbioengineering. Core Microbiome Improv. Crop Qual. Prod., 268–287. doi:10.1002/9781119830795.ch16
Huesemann, M. H., Hausmann, T. S., and Fortman, T. J. (2004). Does bioavailability limit biodegradation? a comparison of hydrocarbon biodegradation and desorption rates in aged soils. Biodegradation 15, 261–274. doi:10.1023/B:BIOD.0000042996.03551.f4
Ite, A. E., and Ibok, U. J. (2019). Role of plants and microbes in bioremediation of petroleum hydrocarbons contaminated soils. Int. J. Environ. Bioremediation Biodegrad. 7 (1), 1–19. doi:10.12691/ijebb-7-1-1
Khan, M. A. I., Biswas, B., Smith, E., Naidu, R., and Megharaj, M. (2018). Toxicity assessment of fresh and weathered petroleum hydrocarbons in contaminated soil-a review. Chemosphere 212, 755–767. doi:10.1016/j.chemosphere.2018.08.094
Kiamarsi, Z., Kafi, M., Soleimani, M., Nezami, A., and Lutts, S. (2020). Conjunction of Vetiveria zizanioides L. and oil-degrading bacteria as a promising technique for remediation of crude oil-contaminated soils. J. Clean. Prod. 253, 119719. doi:10.1016/j.jclepro.2019.119719
Kirk, J. L., Klironomos, J. N., Lee, H., and Trevors, J. T. (2005). The effects of perennial ryegrass and alfalfa on microbial abundance and diversity in petroleum contaminated soil. Environ. Pollut. 133 (3), 455–465. doi:10.1016/j.envpol.2004.06.002
Kurade, M. B., Ha, Y. H., Xiong, J. Q., Govindwar, S. P., Jang, M., and Jeon, B. H. (2021). Phytoremediation as a green biotechnology tool for emerging environmental pollution: A step forward towards sustainable rehabilitation of the environment. Chem. Eng. J. 415, 129040. doi:10.1016/j.cej.2021.129040
Lee, S-H., Lee, W-S., Lee, C-H., and Kim, J. G. (2007). Degradation of phenanthrene and pyrene in rhizosphere of grasses and legumes. J. Hazard. Mater. 153 (1/2), 892–898. doi:10.1016/j.jhazmat.2007.09.041
Lipson, D., and Näsholm, T. (2001). The unexpected versatility of plants: organic nitrogen use and availability in terrestrial ecosystems. Oecologia 128 (3), 305–316. doi:10.1007/s004420100693
Luhach, J., and Chaudhry, S. (2012). Effect of diesel fuel contamination on seed germination and growth of four agricultural crops. Univers. J. Environ. Res. Technol. 2 (4), 311–317.
Nisa, W., Rashid, A., Aziz, N. B., Mahmood, T., Islam, K., and Kazmi, S. K. (2015). Potential of vetiver (Vetiveria Zizanioides L.) grass in removing selected pahs from diesel contaminated soil. Pak. J. Bot. 47 (1), 291–296.
Odukoya, J., Lambert, R., and Sakrabani, R. (2019). Understanding the impacts of crude oil and its induced abiotic stresses on agrifood production: a review. Horticulturae 5, 47. doi:10.3390/horticulturae5020047
Ossai, I. C., Ahmed, A., Hassan, A., and Hamid, F. S. (2020). Remediation of soil and water contaminated with petroleum hydrocarbon: A review. Environ. Technol. Innov. 17, 100526. doi:10.1016/j.eti.2019.100526
Pacwa-Płociniczak, M., Czapla, J., Płociniczak, T., and Piotrowska-Seget, Z. (2019). The effect of bioaugmentation of petroleum-contaminated soil with Rhodococcus erythropolis strains on removal of petroleum from soil. Ecotoxicol. Environ. Saf. 169, 615–622. doi:10.1016/j.ecoenv.2018.11.081
Pawlik, M., Płociniczak, T., Thijs, S., Pintelon, I., Vangronsveld, J., and Piotrowska-Seget, Z. (2020). Comparison of two inoculation methods of endophytic bacteria to enhance phytodegradation efficacy of an aged petroleum hydrocarbons polluted soil. Agronomy 10 (8), 1196. doi:10.3390/agronomy10081196
Reilley, K., Banks, M. K., and Schwab, A. P. (1996). Dissipation of polycyclic aromatic hydrocarbons in the rhizosphere. J. Environ. Qual. 25, 212–219. doi:10.2134/jeq1996.00472425002500020002x
Ruley, J. A., Amoding, A., Tumuhairwe, J. B., and Basamba, T. A. (2022). Rhizoremediation of petroleum hydrocarbon–contaminated soils: A systematic review of mutualism between phytoremediation species and soil living microorganisms. Phytoremediation, 263–296. doi:10.1016/B978-0-323-89874-4.00008-X
Scavo, A., Abbate, C., and Mauromicale, G. (2019). Plant allelochemicals: Agronomic, nutritional and ecological relevance in the soil system. Plant Soil 442 (1), 23–48. doi:10.1007/s11104-019-04190-y
Seddighinia, F. S., Iranbakhsh, A., Oraghi Ardebili, Z., Nejad Satari, T., and Soleimanpour, S. (2020). Seed priming with cold plasma and multi-walled carbon nanotubes modified growth, tissue differentiation, anatomy, and yield in bitter melon (Momordica charantia). J. Plant Growth Regul. 39 (1), 87–98. doi:10.1007/s00344-019-09965-2
Shah, F., and Wu, W. (2019). Soil and crop management strategies to ensure higher crop productivity within sustainable environments. Sustainability 11 (5), 1485. doi:10.3390/su11051485
Smith, M. J., Flowers, T. H., Duncan, H. J., and Alder, J. (2006). Effects of polycyclic aromatic hydrocarbons on germination and subsequent growth of grasses and legumes in freshly contaminated soil and soil with aged PAHs residues. Environ. Pollut. 141 (3), 519–525. doi:10.1016/j.envpol.2005.08.061
Stassen, M. J., Hsu, S. H., Pieterse, C. M., and Stringlis, I. A. (2021). Coumarin communication along the microbiome–root–shoot axis. Trends Plant Sci. 26 (2), 169–183. doi:10.1016/j.tplants.2020.09.008
Tartaglia, M., Zuzolo, D., Postiglione, A., Prigioniero, A., Scarano, P., Sciarrillo, R., et al. (2022). Biotechnological combination for co-contaminated soil remediation: focus on tripartite “meta-enzymatic” activity. Front. Plant Sci. 13, 852513. doi:10.3389/fpls.2022.852513
Terzaghi, E., Zanardini, E., Morosini, C., Raspa, G., Borin, S., Mapelli, F., et al. (2017). Rhizoremediation half-lives of PCBs: Role of congener composition, organic carbon forms, bioavailability, microbial activity, plant species and soil conditions, on the prediction of fate and persistence in soil. Sci. Total Environ. 612, 544–560. doi:10.1016/j.scitotenv.2017.08.189
Tran, T. H., Gati, E. M., Eshel, A., and Winters, G. (2018). Germination, physiological and biochemical responses of acacia seedlings (Acacia raddiana and Acacia tortilis) to petroleum contaminated soils. Environ. Pollut. 234, 642–655. doi:10.1016/j.envpol.2017.11.067
Vergani, L., Mapelli, F., Zanardini, E., Terzaghi, E., Di Guardo, A., Morosini, C., et al. (2017). Phyto-rhizoremediation of polychlorinated biphenyl contaminated soils: An outlook on plant-microbe beneficial interactions. Sci. Total Environ. 575, 1395–1406. doi:10.1016/j.scitotenv.2016.09.218
Wei, H., Son, g. S., Tian, H., and Liu, T. (2014). Effects of phenanthrene on seed germination and some physiological activities of wheat seedling. C. R. Biol. 337, 95–100. doi:10.1016/j.crvi.2013.11.005
Wild, E., Dent, J., Thomas, G. O., and Jones, K. C. (2005). Direct observation of organic contaminant uptake, storage, and metabolism within plant roots. Environ. Sci. Technol. 39, 3695–3702. doi:10.1021/es048136a
Ye, S., Zen, g. G., Wu, H., Zhan, g. C., Lian, g. J., Dai, J., et al. (2017). Co-occurrence and interactions of pollutants, and their impacts on soil remediation—a review. Crit. Rev. Environ. Sci. Technol. 47 (16), 1528–1553. doi:10.1080/10643389.2017.1386951
Keywords: root exudate, glycine, phytoremediation, ryegrass, petroleum hydrocarbons contaminated soil
Citation: He M, Li Z and Mei P (2022) Root exudate glycine synergistically promotes phytoremediation of petroleum-contaminated soil. Front. Environ. Sci. 10:1033989. doi: 10.3389/fenvs.2022.1033989
Received: 01 September 2022; Accepted: 28 September 2022;
Published: 12 October 2022.
Edited by:
Amin Mojiri, Hiroshima University, JapanReviewed by:
Carmine Guarino, University of Sannio, ItalyHossein Farraji, University of Canterbury, New Zealand
Copyright © 2022 He, Li and Mei. This is an open-access article distributed under the terms of the Creative Commons Attribution License (CC BY). The use, distribution or reproduction in other forums is permitted, provided the original author(s) and the copyright owner(s) are credited and that the original publication in this journal is cited, in accordance with accepted academic practice. No use, distribution or reproduction is permitted which does not comply with these terms.
*Correspondence: Ping Mei, bWVpcGluZ2hiQDEyNi5jb20=