- 1Henan Engineering Research Center of Industrial Enzymes, Institute of Biology Co., Ltd., Henan Academy of Sciences, Zhengzhou, China
- 2Anyang Academy of Agricultural Sciences, Anyang, China
3-Methylindole is a major component of organic pollutants in livestock compost, which can contribute to the deterioration of the environment in livestock farms and their surrounding areas. This study demonstrates that using microorganisms to degrade 3-methylindole is an effective method for energy conservation and environmental protection. The microbe capable of efficiently degrading 3-methylindole was isolated and screened from fecal samples. The isolated bacteria were identified as Acinetobacter oleivorans after morphological characterization and 16S rRNA sequencing. This project demonstrated that 3-methylindole was completely degraded under optimal conditions (initial concentration of 3MI: 100 mg/L, 30°C, pH8.0, and shaking at 160 rpm for 48 h). N2-Acetyl-L-ornithine, Phenylacetaldehyde, Phenylacetic acid, Indole-3-carboxylic acid, and Indole-3-carboxaldehyde were the primary metabolites of this degradation process. This study provides a theoretical foundation for other microbe-mediated environmental remediation approaches as well as a basis for future work to apply bacteria that degrade 3-methylindole for the purification of polluted environments. It has a promising application in the control of malodorous gas pollution in the large-scale livestock and poultry breeding industries.
1 Introduction
Animal husbandry efforts have advanced rapidly in scale and complexity in China over recent years owing to the progressive improvement of national standards of living. Because of the increasing demand for meat, milk, and eggs among the Chinese population, the number of livestock and poultry herds that are kept in China has steadily increased, which has led to the production of a significant amount of malodorous fecal waste. China produced 3.884 billion tons of livestock and poultry manure and sewage in 2015 alone, including 636, 565, and 2,633 million tons of fresh manure, urine, and sewage, respectively (Qian et al., 2018; Zou et al., 2020). However, our understanding of the generation, identification, prevention, and abatement/treatment of unpleasant odors is incomplete. Therefore, the treatment of livestock and poultry manure odor gas pollution has become increasingly important and urgent (Cheng et al., 2018).
3-Methylindole (3MI) is a common and well-known N-heterocyclic aromatic compound with a fecal odor that can be found in a variety of environmental matrices. 3MI is found naturally in animal faeces, coal tar, beetroot, and camphor wood, and it is used as a precursor in the production of many food additives and chemicals used in industrial and agricultural settings. Animal manure and wastewater treatment plant samples have been the focus of the majority of studies on the detection and quantification of skatole. However, skatoles can enter various environments via waste stream discharge, gas emissions, manure fertilizer application, wildlife excretion, and alga decomposition. Skatoles can also be produced by indigenous microorganisms (Ma et al., 2021).
As a harmful odorous compound released by poultry and livestock, 3MI has a strong fecal odor with an odor threshold of less than 0.003 mg/m3, making it detectable at extremely low concentrations (Liu et al., 2018). In addition to having an unpleasant odor, 3MI has been associated with the development of pulmonary edema and emphysema in sheep and cattle. It can also increase the risk of developing a tumor in the respiratory tract and contribute to the development of neuroendocrine and immunological diseases in humans. It can further attract flies, mosquitoes, and beetles, thereby contributing to the spread of diseases transmitted by insects (Yang et al., 2019; Zhang et al., 2022). Consequently, it is urgently necessary to conduct a thorough investigation of 3MI environmental pollution.
To date, effective physical adsorption and chemical oxidation methods have been developed for the elimination of 3MI contamination. However, physical adsorption approaches are expensive, energy-intensive, and susceptible to poor environmental conditions. In addition to these limitations, chemical oxidation techniques can also contribute to secondary pollution (Luo, 2015). Biodegradation is a viable and eco-friendly method of removing skatole (Qu, 2020). Biodegradation has emerged as an attractive alternative method for removing 3MI and other environmental contaminants that do not entail the same range of drawbacks. Several 3MI-degrading microbes have since been identified (Ma et al., 2021; Wu et al., 2021), including Clostridium maleominatum A-3 under anaerobic conditions (Kohda et al., 1997), methanogenic bacteria in the context of sulfur reduction (Gu et al., 2002), and Pseudomonas putida lpc24 under conditions of oxygen restriction. Moreover, aerobic bacterial species such as Lactobacillus brevis (Meng et al., 2013), Rhodopseudomonas palustris wku-kdns3 (Sharma et al., 2015), Acinetobacter toweneri NTA1-2A, Acinetobacter gullouiae tat1-6 (Tesso et al., 2019), Burkholderia sp. IDO3, Rhodococcus sp. dmu1 and Rhodococcus pyridinvorans rp3 (Wu et al., 2021) can degrade this compound. However, these microbes generally exhibit only limited 3MI degradation activity, which is impaired in the presence of high 3MI concentrations. These microorganisms may grow under strict conditions that prevent their use in production settings because they are unable to break down this compound effectively. Future efforts to screen for bacteria that degrade 3MI are thus obviously necessary.
A bacterial strain with 3MI-degrading activity was isolated from pig manure compost soil in this study through screening efforts. Its capacity to break down manure as well as its growth performance and degradation activity under various culture conditions (inoculum amount, temperature, pH, and initial manure concentration) were then evaluated. In addition, metabolites produced by this strain were identified using ultra-high performance liquid chromatography-mass spectrometry (UHPLCQ-TOF MS). The purpose of this study was to develop effective strain resources for fecal odor degradation and to create a foundation suitable for using this strain to purify odor-polluted environments.
2 Materials and methods
2.1 Test materials
2.1.1 Microbial strain origins
The microbial strains screened in this study were isolated from samples of pig feces, fattening pig feces, sow feces, and pig plant manure collected in Luoshan County, Xinyang, Henan Province, China.
2.1.2 Culture medium
Inorganic salt medium (g/L): (NH4)2SO4 1.0, KH2PO4 0.2, K2HPO4 0.8, MgCl2·7H2O 1.1, FeCl2·4H2O 0.016, NaCl 0.5, pH7.0 ± 0.2. LB medium was prepared as follows (g/L): NaCl 10.0, peptone 10.0, yeast powder 5.0, pH 7.0, and Agar 15.0 was utilized to produce solidified medium. PBS was prepared as follows (g/L): Na2HPO4·12H2O 10.8, NaH2PO4·2H2O 2.3, pH 7.0.
2.1.3 Reagents and equipment
In this study, the analyses were performed using pure 3MI standards, pure methanol, anhydrous ethanol, glacial acetic acid, and NaCl. Equipment and approaches used for this study included high-performance liquid chromatography (HPLC), liquid chromatography-mass spectrometry (LC-MS), a UV spectrophotometer, PCR, scanning electron microscopy, an AB Triple TOF 6600 Mass Spectrometer (AB SCIEX)/Q Exactive HF Mass Spectrometer (Thermo), an Agilent 1290 Infinity LC Ultra High-Pressure Liquid Chromatography (Agilent)/Thermo Ultra High-Pressure Liquid Chromatography (Thermo) system, a low-temperature high-speed centrifuge (Eppendorf 5430R), an ACQUITY UPLC BEH Amide column (1.7 μm, 2.1 mm × 100 mm), acetonitrile (Merck,1499230-935), ammonium acetate (Sigma,70221), methanol (Fisher, A456-4), and ammonia (Fisher, A470-500).
2.1.4 Bacterial suspension
Initial screening efforts resulted in the isolation of a single bacterial strain, which was chosen and grown for 24 h at 30°C with constant shaking in 150 ml of sterile liquid LB medium (160 rpm). The culture medium was then centrifuged for 10 min at 6,000 rpm at 4°C, the pellet was washed 2-3 times with PBS (pH 6.8), and the centrifugation process was repeated. Then PBS was used to suspend the cells.
3 Methods
3.1 3MI standard preparation
A 5 g/L 3MI standard solution was produced by dissolving 0.5 g of 3MI standard in 100 ml of anhydrous ethanol. This mixture was then sterilized with an organic filter membrane of 0.2 μm and stored at 4°C for subsequent use.
3.2 Isolation and screening of 3MI-degrading bacteria
In total, 1.0 g of pig manure compost soil was added to a sterile enriched liquid medium and cultured for 24 h at 30°C with constant shaking at 180 rpm. This medium was then used to inoculate (5%) a flask of inorganic salt medium supplemented with 100 mg/L 3MI, followed by 24 h of incubation at 30°C with 180 rpm. This subculturing procedure was repeated four times under identical conditions. Following this, 1 ml of the enriched solution was diluted 10-fold to 10−5 10−6, and 10−7 dilution levels, and 100 μl of each of these diluted samples was then spread on an enrichment solid medium. After incubation, the color, morphology, and size of the colonies were evaluated, and single colonies were selected to ensure that they possessed consistent morphological characteristics. Beef extract peptone solid (LB) medium was then used to perform further purification to yield colonies with a single morphological profile. An inclined culture medium was inoculated with the isolated bacterial strains that could grow when 3MI was the only carbon source available, and they were stored at 4°C.
3.3 Identification of 3MI-degrading bacteria
Identification of isolated 3MI-degrading bacteria was carried out using morphological, physiological, and biochemical methods, as in previous studies (Tesso et al., 2019; Whitman et al., 2012).
3.4 16S rRNA sequencing
The bacterial 16S rRNA gene sequence was amplified with the following universal primers: forward 27F:5′-AGAGTTTGATCATGGCTCAG-3′,reverse1492R:5′-ACGG TTACCTTACCTTGTTACGACTT-3′. All amplifications were carried out in 50 μl reactions containing 2 μl of gDNA, 2 μl of suitable primers (10 μmol/L), 25 μl of 2Taq PCR Mix, and 19 μl of purified water. The settings for the thermocycler were as follows: 98°C for 2 min; 98°C for 10 s, 55°C for 15 s, and 72°C for 90 s across 38 cycles; 72°C for 5 min.
Amplicons were sequenced by a commercial company, and the obtained sequences were compared with those in the NCBI GenBank. The isolated strains were identified by constructing a phylogenetic tree using the neighbor-joining method and the MEGA 6 software.
3.5 HPLC measurement of 3MI concentrations
For the HPLC-based detection of sample 3MI concentrations, 10 mg of 3MI was suspended at 1 mg/ml in anhydrous ethanol and diluted to standard concentrations of 50, 100, 200, 300, and 500 μg/ml. These standards were passed through a 0.2 µm organic filter membrane, and their peak area values were determined using HPLC, with three measurements per concentration level. The sample concentrations and peak area values were used to establish a linear regression. Chromatographic separation was carried out utilizing an Agilent 5 TC-C18 (250 mm × 4.6 mm, 5 µm) column at 30°C with a UV detection wavelength of 260 nm and a mobile phase of 1% acetic acid: water (1:1). The flow rate was maintained at 1.0 ml/min, while the injection volume was 20 µl.
3.6 Analyses of bacterial growth
The isolated AO-06 experimental strain of highly efficient 3MI-degrading bacteria was selected as the primary focus of this study. These bacteria were used to inoculate inorganic salt medium (10% inoculum), which was then cultured at 30°C in the presence of 100 mg/L 3MI as a carbon source while being continuously shaken at 140 rpm. Bacteria were cultured in triplicate for 72 h, and their growth was evaluated every 12 h. As a blank solution, an inorganic salt medium containing 3MI was used, and the absorbance at 600 nm (OD600) in each sample was measured using a UV spectrophotometer, with samples being thoroughly mixed with a whirlpool oscillator before measurement.
3.7 Analysis of 3MI degradation activity
To evaluate the ability of isolated bacteria to degrade 3MI, these cells were cultured for 72 h at 30°C and 140 rpm in liquid inorganic salt medium (10% inoculum) containing 500 mg/L 3MI. No bacteria were added to a control sample that served as a blank. The 3MI concentrations in these culture flasks were measured every 12 h in triplicate.
3.8 Analysis of the growth and degradation activity of strain AO-06
The ability of strain AO-06 to grow and degrade 3MI when 3MI (100 mg/L) was the only carbon source available was tested using a 10% inoculation, with cells being cultured at a natural pH at 30°C (180 rpm). Samples were collected every 12 h in triplicate, and OD600 values were measured as described above to monitor bacterial growth. The liquid phase samples were then subjected to 3MI extraction by combining them with an equal volume of methanol, centrifuging them at 5,000 rpm for 10 min, passing 1 ml of solution through a 0.22 μm organic filter membrane, and storing it at 4°C for testing. Samples with excessive concentrations were diluted before HPLC analysis.
3.9 The impact of environmental conditions on the 3MI-degrading activity of strain AO-06
The effect of various environmental conditions on the degradation of 3MI was studied. The following were the basic culture conditions: Medium with a natural pH containing 100 mg/L 3MI; temperature: 30°C; shaking speed: 180 rpm; inoculum concentration: 5%. Periodically, samples were collected to evaluate 3MI concentrations and bacterial growth, as described above. Rates of 3MI degradation were the primary metric of interest, and they were computed as follows:
3MI degradation rate (%) = (3MI content in uninoculated medium—3MI content in inoculated medium)/3MI content in uninoculated medium ×100%
The effects of different starting concentrations of 3MI (100, 150, 200, 250, 300 mg/L), pH levels (4.0, 5.0, 6.0, 8.0, 9.0), temperatures (20, 25, 30, 37, 42°C), and shaking speeds (0, 60, 120, 180, 240 rpm) on 3MI degradation were evaluated, with samples collected every 12 h in triplicate. The 3MI concentration was fixed at 100 mg/L for all assays unless otherwise indicated.
3.10 Metabolite detection
The preliminary analysis of metabolites produced by strain AO-06 when it degrades 3MI over a 48 h period was performed using a UHPLCQ-TOF MS approach. The experimental procedure is as follows:
1) based on the above analyses of growth and 3MI degradation characteristics, a sample collection time point of 48 h was selected, as bacteria were at a stable level with relatively vigorous growth and the highest observed 3MI degradation activity, allowing for more robust analyses of metabolite production. Three replicate samples were established in both the control and culture groups.
2) The samples were thawed at 4°C, combined with an equal amount of chilled methanol/acetonitrile/aqueous solution (2:2:1, v/v), mixed by vortexing, subjected to low-temperature ultrasonication for 30 min, allowed to stand for 10 min at −20°C, and centrifuged at 14,000 xg for 20 min at 4°C. The supernatants were then vacuum-dried, redissolved in a 100 μl acetonitrile aqueous solution (acetonitrile: water, 1:1, v/v), vortexed, centrifuged for 15 min at 14,000xg at 4°C, and the supernatants were utilized for subsequent procedures.
3) All samples were separated using an Agilent 1290 Infinity LC ULTRA high-performance liquid chromatography (UHPLC) HILIC column. The column was maintained at 25°C with a flow rate of 0.5 ml/min, an injection volume of 2 μl, and a mobile phase of water containing 25 mM ammonium acetate and 25 mM ammonia (A) and acetonitrile (B). Linear gradient elution was conducted as follows: 0–0.5 min, 95% B; 0.5–7 min, 95%–65% B; 7–8 min, 65%–40% B; 8–9 min, 40% B; 9–9.1 min, 40%–95% B; 9.1–12 min, 95% B. During this analytical procedure, samples were placed in an automatic sampler at 4°C. Random sequences were utilized for continuous sample analysis to control for the influence of instrument detection signal fluctuations. Quality control (QC) samples were added at random to the sample queue to monitor system stability and result reliability.
4) An AB Triple TOF 6600 mass spectrometer was used to collect a sample of first- and second-order spectrograms. Samples were separated using an Agilent 1290 Infinity LC UHPLC system, as described above, before being analyzed with the Triple TOF 6600 mass spectrometer (AB SCIEX). Positive and negative electrospray ionization (ESI) ion modes were used for detection, with the following ESI source parameters: Atomizing gas auxiliary heating 1 (Gas1): 60, auxiliary heating 2 (Gas2): 60, Air curtain gas (CUR): 30P SI, ion source temperature: 600°C, spray voltage (ISVF) ±5500 V (positive and negative modes); Primary mass charge ratio detection range: 60–1000 Da, secondary ion mass charge ratio detection range: 25–1000 Da, primary mass spectrometry scanning cumulative time: 0.20 s/spectra, secondary mass spectrometry scanning cumulative time: 0.05 s/spectra. Secondary mass spectrometry data were collected in data-dependent acquisition mode (IDA) using peak intensity value screening mode. The cluster removal voltage (DP) was ±60 V (positive and negative modes), and the collision energy was 35 ± 15eV. The IDA settings used a dynamic exclusion isotope ion range of 4 Da and 10.
4 Results and discussion
4.1 Screening identifies bacteria capable of degrading 3-methylindole
Three bacterial strains capable of growth when 3MI was the only available carbon source were isolated through repeated dilution and screening assays, indicating that these bacteria could degrade 3MI. Analyses of the 3MI degradation activity of these strains using HPLC revealed that one strain, tentatively designated AO-06, displayed a more robust activity than the other two. As a result, strain AO-06 was selected for additional experimental characterization. Functional microorganisms play an important role in the biological deodorization of livestock and poultry faces, so the key to Biological deodorization is used to identify strains that have a high deodorization efficiency (Hort et al., 2009). Chinese researchers have been working on microbial screening for deodorants for a very long time, but due to the limitations of screening techniques, microbial specificity, and other factors, the deodorant microorganisms being screened have issues with poor adaptability, single effects, and low efficiency. The deodorizing strain was therefore very important because it was chosen among those with strong adaptability and high efficiency.
4.2 Morphological, physiological, biochemical, and molecular characterization of strain AO-06
Following plate streaking separation, strain AO-06 was discovered to have round bacterial colonies with a smooth, white, protruding surface that appeared moist and shiny, with clearly defined edges (Figure 1). Bacteria were rod-shaped, Gram-negative, and non-sporulating upon microscopic examination. According to the “Manual of Systematic Identification of Common Bacteria,” starch hydrolysis, gelatin hydrolysis, and glucose/mannose utilization assays were performed, with the results of such biochemical and physiological characterization summarized in Table 1.
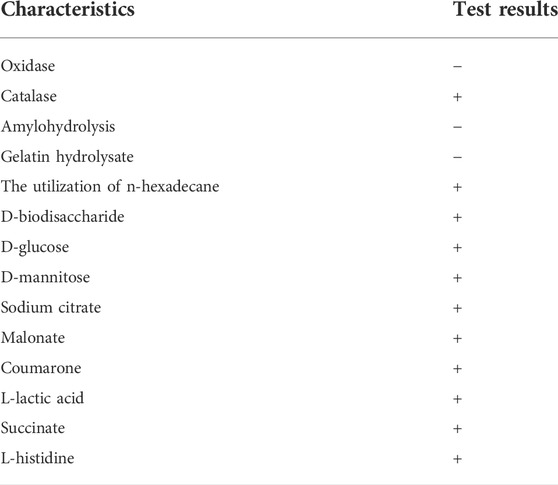
TABLE 1. The microbiological characteristics of strain AO-06 capable of growing in the presence of 3MI as the only available carbon source.
A 16S rRNA sequencing approach produced a 1434 bp gene product, which was compared to known sequences in the NCBI GenBank database before constructing a phylogenetic tree using the neighbor-joining method. This strain exhibited 99.9% sequence homology and 99.9% sequence similarity with Acinetobacter oleivorans (Figure 2). As a result, A. oleivorans was tentatively identified. There are many microorganisms in the environment, and these indigenous microorganisms play an important role in environmental purification. Because of the high variability of microorganisms, a large number of microorganisms suitable for survival in this environment can be left to be selected through natural domestication (Verschuere et al., 2001). The samples collected in this experiment were composted pig manure and activated sludge from pig farms. The reason for this is that pig manure in the composting process contains a large number of microorganisms and is rich in species.
4.3 Characterization of the growth of strain AO-06
To facilitate the accurate quantification of 3MI concentrations in culture samples, 50, 100, 200, 300, and 500 μg/ml 3MI standards were prepared and detected via HPLC. Then, a standard curve was constructed by plotting concentrations against peak area values (Figure 3). The retention time of 3MI in liquid chromatograms at 270 nm was 5.092 min (Figure 4). The linear regression equation for the 3MI standard curve was y = 23.931x–118.28 (R2 = 0.9917).
According to OD600 measurements, AO-06 exhibited a logarithmic growth phase during which 3MI concentrations gradually decreased. After 48 h, maximum growth and complete 3MI degradation were evident. Thereafter, the growth rate decreased gradually (Figure 5).
4.4 The impact of culture conditions on the growth and 3Midegrading activity of strain AO-06
4.4.1 The effects of 3MI concentration on bacterial growth and 3MI degradation
In this study, a variety of starting 3MI concentrations (0, 50, 100, 150, 200, 250, and 300 mg/L) were used to evaluate the effect of these concentrations on 3MI degradation activity. Complete 3MI degradation was observed after 48 h at a starting 3MI concentration of 100 mg/L (Figure 6A), whereas higher 3MI concentrations required a longer period. This may be the result of a delay in degradation timing caused by continuous bacterial adaptation to environmental toxicity during the growth process.
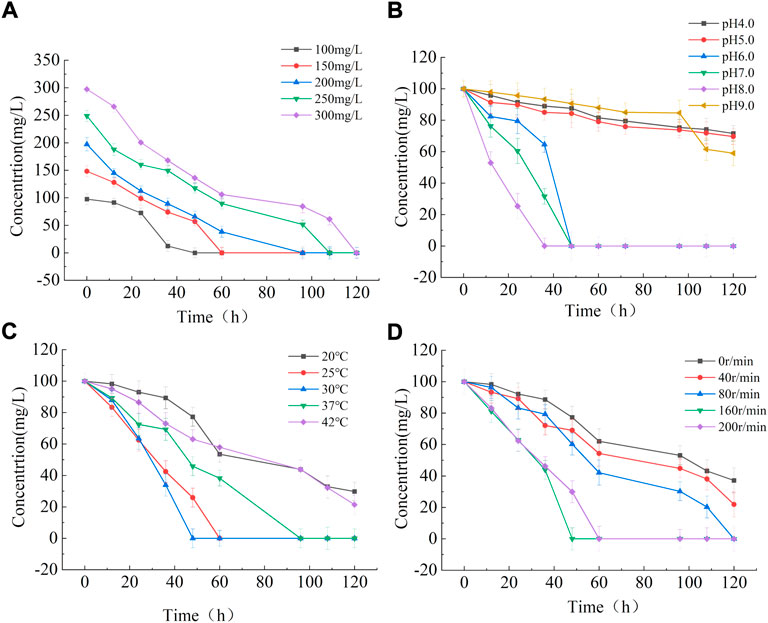
FIGURE 6. The impact of starting 3MI concentrations (A), pH (B), temperature (C), and shaking speed (D) on 3MI degradation.
4.4.2 The impact of pH on bacterial growth and 3MI degradation
A range of pH values (4.0, 5.0, 6.0, 8.0, and 9.0) was then used to investigate the relationship between this variable and 3MI degrading activity changes in pH can affect nutrient availability, membrane charge and stability, membrane permeability, the ability of cells to absorb specific compounds, and the efficacy of certain intracellular reactions (Zhang et al., 2022). At a pH of 8.0, A. oleivorans AO-06 displayed optimal 3MI degrading activity, achieving 100% degradation in 36 h (Figure 6B). This activity was significantly inhibited by excessively acidic or alkaline conditions, with degradation rates of <50% even after 120 h.
4.4.3 The impact of temperature on bacterial growth and 3MI degradation
Temperature effects on 3MI degradation were studied at five different temperatures: 20°C, 25°C, 30°C, 37°C, and 42°C. At 30°C, Acinetobacter Oleivorans AO-06 had the highest degradation efficiency for 3MI (Figure 6C), and the degradation rate reached 100% within 48 h. The temperature range that allowed Acinetobacter Oleivorans AO-06 to achieve a 100% degradation rate in 48 h was 25–37°C (Figure 6C). The temperature had an effect on the degradation efficiency of Acinetobacter Oleivorans AO-06, indicating that 30°C was the optimal growth temperature for strain AO-06.
4.4.4 The impact of shaking speed on 3MI degradation
Five different shaking speeds (0, 40, 80, 160, and 200 rpm) were investigated to see if they had an effect on 3MI degradation. Complete 3MI degradation was observed at speeds ranging from 80 to 200 rpm (Figure 6D), with a speed of 160 rpm exhibiting 100% degradation after 48 h. The rate of shaking affects the dissolved oxygen content of the media, which can indirectly influence bacterial growth. As a result, faster-shaking results in higher oxygen levels in the culture medium. However, once a certain speed is reached, no further increase in dissolved oxygen levels can be achieved.
The optimal culture conditions for strain AO-06 to most efficiently degrade 3MI (starting concentration: 100 mg/L) were pH 8.0 at 30°C with 160 rpm shaking. These conditions allow for 100% degradation efficiency. Therefore, this strain may be well-suited for the biodegradation of 3MI-contaminated environments in the field. Future studies will focus on elucidating the toxicity and metabolic characteristics of strain AO-06 to provide a theoretical basis for the future industrial-scale removal of 3MI from the environment, particularly in pig farm waste.
4.5 Metabolite detection
Analyses of metabolomics provide a method for quantifying all metabolites present in specific biological samples. Potentially harmful metabolites produced by strain AO-06 during 3MI degradation under optimized conditions were then assessed after 48 h and compared to a blank control sample using UHPLC-MS (Figure 7).
This analysis found 605 metabolites in total, with 388 and 217 detected in positive and negative ion modes, respectively (Table 2). These metabolites were identified using an in-house database (Shanghai Applied Protein Technology), with structures identified by matching the retention time, molecular weight (molecular mass error <10 ppm), secondary fragmentation spectrum, collision energy, and other information for these metabolites. In total, 6 putative metabolites associated with 3MI metabolism were identified, including 3-methyloxindole,1H-Indole-2,3-dione, Phenylacetaldehyde, Phenylacetic acid, 1H-indole-3-carboxaldehyde, and indole-3-carboxylic acid (Figure 8).
The absence of systematic studies evaluating the 3MI metabolism pathway has resulted in the identification of only putative metabolites based on the observations of individual studies. Fukuoka (Fukuoka et al., 2015) also detected 1H-Indole-3-carboxaldehyde and Indole-3-carboxylic acid when identifying the metabolites of 3MI, whereas 3-methyloxindole has frequently been reported in the literature as a metabolite produced from 3MI by methanogens enriched from wetland soil (Gu and Berry, 1992). There have also been reports of indole-3-carboxylic acid, which was identified as a byproduct of 3MI degradation mediated by Pseudomonas aeruginosa, along with indoline-3-ol (Yin and Gu, 2006). The presence of 1H-indole-2,3-dione (indigo red) was also detected in this experiment, possibly as a result of further oxidation of 3-methyl hydroxyindole. Indigo red has been identified as a common indole metabolite, and may therefore be the subsequent byproduct of indole metabolism following the degradation of 3MI to indole.
Previous studies on 3MI-degrading bacteria have been carried out, and some strains with degradation ability, such as Pseudomonas putida LPC24, Lactobacillus brevis 1.12 (Meng et al., 2013), Rhodopseudo-monas palustris WKU-KDNS3 (Sharma et al., 2015), Acinetobacter toweneri NTA1-2A (Tesso et al., 2019), Rhodococcus sp. DMU1 and DMU2, and Burkholderia sp. IDO3, have been reported. Acinetobacter species are widespread in natural environments and have been implicated as important oil degradation mediators (Fukuoka et al., 2015) (Table 3). In the present study, A. oleivorans AO-06 was isolated and it could degrade 3MI below 300 mg/L completely within a short time. This study provides a theoretical foundation for other microbe-mediated environmental remediation approaches as well as a basis for future work to apply bacteria that degrade 3-methylindole for the purification of polluted environments. It has a promising application in the control of malodorous gas pollution in the large-scale livestock and poultry breeding industries.
5 Conclusion
In conclusion, the present screening efforts identified Acinetobacter oleivorans strain AO-06 as capable of degrading 3MI with a high degree of efficiency. The 3MI degradation of this strain was independent of the initial 3MI concentration but was affected by pH, temperature, and shaking speed; optimal culture conditions were established (initial 3MI concentration: 100 mg/L, pH: 8.0, shaking speed: 160 rpm, 30°C for 48 h). An LC-MS approach was used to identify putative 3MI metabolites produced by strain AO-06, yielding N2-acetyl-L-Ornithine, Phenylacetaldehyde, Phenylacetic acid, Indole-3-carboxylic acid, and indole-3-carboxaldehyde. However, more investigation is needed to determine the mechanisms by which strain AO-06 metabolizes 3MI.
Data availability statement
The original contributions presented in the study are included in the article/Supplementary Material, further inquiries can be directed to the corresponding author.
Author contributions
The authors have contributed to this article as follows: Conceptualization, HH and XZ; methodology, HH and XZ; formal analysis, SQ; writing-original draft, FG and FF; investigation, WD, HM, and LX writing-review and editing, XZ, LL, and HH; funding acquisition, LL and HH. All authors have read and agreed to the published version of the article.
Funding
This research was funded by the Project of Outstanding Talents Training Program of Henan Academy of Sciences (Grant Number 210405004), Central Plains Science and technology innovation leader Project (Grant Number 214200510011), and Outstanding Talents Training Program of Henan Academy of Sciences (Grant Number 210405002).
Conflict of interest
Authors HH, LL, WD, HM, FF, SQ, and XZ were employed by the company Institute of Biology Co., Ltd.
The remaining author declares that the research was conducted in the absence of any commercial or financial relationships that could be construed as a potential conflict of interest.
Publisher’s note
All claims expressed in this article are solely those of the authors and do not necessarily represent those of their affiliated organizations, or those of the publisher, the editors and the reviewers. Any product that may be evaluated in this article, or claim that may be made by its manufacturer, is not guaranteed or endorsed by the publisher.
References
Cheng, S., Li, Z., Uddin, S. M. N., Mang, H. P., Zhang, L., Zhang, J., et al. (2018). Toilet revolution in China. J. Environ. Manage. 216, 347–356. doi:10.1016/j.jenvman.2017.09.043()
Fukuoka, K., Ozeki, Y., and Kanaly, R. A. (2015). Aerobic biotransformation of 3-methylindole to ring cleavage products by Cupriavidus sp. strain KK10. Biodegradation 26, 359–373. doi:10.1007/s10532-015-9739-0
Gu, J. D., and Berry, D. F. (1992). Metabolism of 3-methylindole by a methanogenic consortium. Appl. Environ. Microbiol. 58, 2667–2669. doi:10.1128/aem.58.8.2667-2669.1992
Gu, J. D., Fan, Y. Z., and Shi, H. C. (2002). Relationship between structures of substituted indolic compounds and their degradation by marine anaerobic microorganisms. Mar. Pollut. Bull. 45, 379–384. doi:10.1016/S0025-326X(02)00091-7
Hort, C., Gracy, S., Platel, V., and Moynault, L. (2009). Evaluation of sewage sludge and yard waste compost as a biofilter media for the removal of ammonia and volatile organic sulfur compounds (VOSCs). Chemical Engineering Journal 152 , 1, 44–53.
Kohda, C., Ando, T., and Nakai, Y. (1997). Isolation and characterization of anaerobic indole- and skatole-degrading bacteria from composting animal wastes. J. Gen. Appl. Microbiol. 43, 249–255. doi:10.2323/jgam.43.249
Li, P., Wang, Y. H., Jiang, Z., and Tong, L. (2010). Bioaugmentation of cellulose degradation in swine wastewater treatment with a composite microbial consortium. Fresenius Environ. Bull. 19, 3107–3112. doi:10.1007/978-90-481-9558-9-10
Liu, D. Z., Wei, Y. F., Liu, X. Y., Zhou, Y., Jiang, L., Yin, J. Y., et al. (2018). Indoleacetate decarboxylase is a glycyl radical enzyme catalysing the formation of malodorant skatole. Nat. Commun. 9, 4224. doi:10.1038/s41467-018-06627-x
Luo, H. E. (2015). Study on the screening and biodegradation characteristics of indole-degrading bacteria. dissertation/master’s thesis (China (Guodong): Guangdong University of Technology).
Ma, Q., Meng, N., Li, Y. J., and Wang, J. W. (2021). Occurrence, impacts, and microbial transformation of 3-methylindole (skatole): A critical review. J. Hazard. Mat. 416, 126181. doi:10.1016/j.jhazmat.2021.126181
Ma, Q., Qu, H., Meng, N., Li, S. Z., Wang, J. W., Liu, S. W., et al. (2020). Biodegradation of skatole by Burkholderia sp. Ido3 and its successful bioaugmentation in activated sludge systems. Environ. Res. 182, 109123. doi:10.1016/j.envres.2020.109123
Meng, X., He, Z. F., Li, H. J., and Zhao, X. (2013). Removal of 3-methylindole by lactic acid bacteria in vitro. Exp. Ther. Med. 6, 983–988. doi:10.3892/etm.2013.1251
Qian, Y., Song, K., Hu, T., and Ying, T. (2018). Environmental status of livestock and poultry sectors in China under current transformation stage. Sci. Total Environ. 622–623, 702–709. doi:10.1016/j.scitotenv.2017.12.045
Sharma, N., Doerner, K. C., Alok, P. C., and Choudhary, M. (2015). Skatole remediation potential of Rhodopseudomonas palustris WKU-KDNS3 isolated from an animal waste lagoon. Lett. Appl. Microbiol. 60, 298–306. doi:10.1111/lam.12379
Tesso, T. A., Zheng, A., Cai, H., and Liu, G. (2019). Isolation and characterization of two Acinetobacter species able to degrade 3-methylindole. PLoS One 14, 0211275. doi:10.1371/journal.pone.0211275
Verschuere, L., Rombaut, G., Sorgeloos, P., and Verstraete, W. (2000). Probiotic bacteria as biological control agents in aquaculture. Microbiology and molecular biology reviews 64 , 4, 655, 671.
Whitman, W. B., Goodfellow, M., Kämpfer, P., Busse, H. J., Trujillo, M. E., and Suzuki, K. I. (2012). Bergey’s manual of systematic bacteriology: volume 5: the Actinobacteria. Springer Science and Business Media.
Wu, Y. H., Zhang, S. C., Tian, Q., Ma, G. Z., Guo, R. J., Li, S. D., et al. (2021). Isolation and identification of a high-efficiency bacterial strain Rp3 to degrade skatole: An odor chemical in compost. J. Agric. Resour. Environ. 38, 576–584.
Yang, B. Y., Lin, Y. X., Dai, C. X., Yan, Y. Q., Piao, S. Y., Liu, X. Y., et al. (2018). Degradation characteristics of indole by Alcaligenes sp. YBY. Environ. Sci. Technol. 41, 1–6.
Yang, G., Zhang, P., Liu, H., Zhu, X., and Dong, W. (2019). Spatial variations in intestinal skatole production and microbial composition in broilers. Anim. Sci. J. 90, 412–422. doi:10.1111/asj.13164
Yin, B., and Gu, J. D. (2006). Aerobic degradation of 3-methylindole by Pseudomonas aeruginosa gs isolated from mangrove sediment. Hum. Ecol. Risk Assess. Int. J. 12, 248–258. doi:10.1080/10807030500531539
Zhang, Z. Y., Zhou, L. Z., Yue, D. D., Guo, W. Y., Pan, M. S., Qi, L., et al. (2022). Isolation, identification and degradation characteristics of skato-degrading bacteria YKSW-6. Bull. Microbiol. 49, 2486–2499. doi:10.13344/j.microbiol.china.211012
Keywords: biodegradation, 3-methylindole, degradation characteristics, screen, Acinetobacter olivaceus
Citation: Hu H, Li L, Gao F, Diao W, Ma H, Feng F, Quan S, Xiang L and Zhang X (2022) Screening, identification, and degradation characteristics of 3-methylindole degrading bacteria. Front. Environ. Sci. 10:1028699. doi: 10.3389/fenvs.2022.1028699
Received: 26 August 2022; Accepted: 18 October 2022;
Published: 01 November 2022.
Edited by:
Hongbiao Cui, Anhui University of Science and Technology, ChinaReviewed by:
Lei Xu, Nanyang Normal University, ChinaRuibo Sun, Anhui Agricultural University, China
Copyright © 2022 Hu, Li, Gao, Diao, Ma, Feng, Quan, Xiang and Zhang. This is an open-access article distributed under the terms of the Creative Commons Attribution License (CC BY). The use, distribution or reproduction in other forums is permitted, provided the original author(s) and the copyright owner(s) are credited and that the original publication in this journal is cited, in accordance with accepted academic practice. No use, distribution or reproduction is permitted which does not comply with these terms.
*Correspondence: Xiujiang Zhang, MTM5MDM4NDQ0NzJAMTM5LmNvbQ==
†These authors have contributed equally to this work and share the first authorship