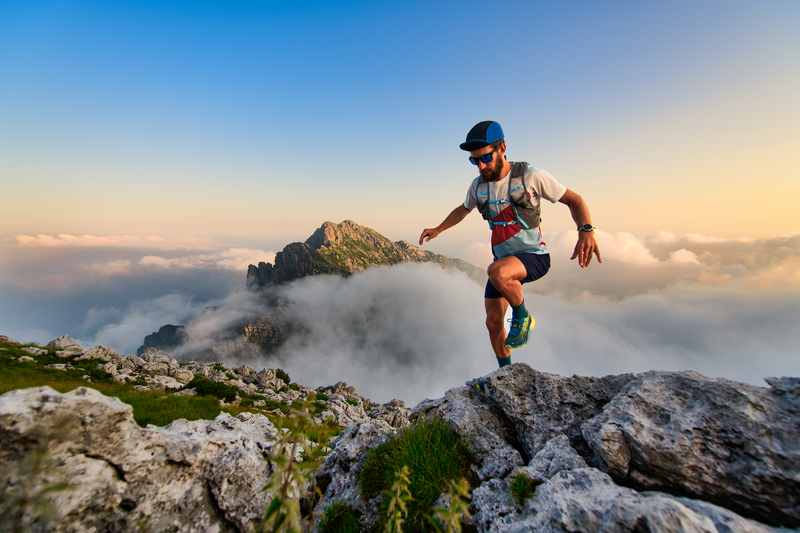
95% of researchers rate our articles as excellent or good
Learn more about the work of our research integrity team to safeguard the quality of each article we publish.
Find out more
ORIGINAL RESEARCH article
Front. Environ. Sci. , 07 November 2022
Sec. Soil Processes
Volume 10 - 2022 | https://doi.org/10.3389/fenvs.2022.1023831
Litter decomposition is the main driver of nutrient cycling process in terrestrial ecosystems. Afforestation completely altered vegetation composition and litter species, disrupting the long-term carbon balance in grassland ecosystem. However, there is a lack of understanding of how litter mixing effect (LME) affects soil carbon cycling in afforested ecosystem. Here, we investigated the effects of litter richness and quality of tree, shrub, and grass species and their litter mixture on soil CO2 fluxes. The results showed that cumulative soil CO2 flux in the early stage (1–28 days) was 1.75 times higher than that in the late stage (29–113 days), indicating litter decomposition was intensive at first and then decreased with time. Soil carbon flux changed with decomposition stages. In the early-stage of decomposition, soil CO2 flux increased with the concentrations of litter carbon, nitrogen and condense tannin. In the late phase of decomposition, all litter chemical traits were negatively related to the soil carbon flux. Additionally, plant litter richness was negatively correlated to early-stage soil CO2 flux, whereas it was positively related to late-stage soil carbon flux. Our results provide evidence that long-term carbon balance in grassland ecosystems was interrupted by afforestation, and the dominant litter chemical traits that controlling soil carbon cycling changed over time.
Litter decomposition is the primary engine of nutrient recycling in terrestrial ecosystems, influencing nutrient availability and soil carbon dynamics (Swift et al., 1979; Aerts, 1997; Mao et al., 2015). Over the past few decades, studies have focused on the decay of single litter species (Gartner and Cardon, 2004). Indeed, the decomposition process in natural ecosystems is a mix of various plant litter species (Hättenschwiler et al., 2005). Studies suggest that litter species interact with each other when decomposing, implying that the decomposition rate of litter mixtures are accelerated or decelerated by litter mixing, namely litter mixing effects (LME) (Gartner and Cardon, 2004; Singhal et al., 2021).
Studies have generalized that LME can be either synergistic or antagonistic, which means the decomposition dynamics of litter mixture was faster or slower than expected (Gartner and Cardon, 2004; Hättenschwiler et al., 2005). The underlying hypotheses of LME include 1) nutrient transfer among litter types, 2) complementary resource use, and 3) stimulatory or inhibitory influences of specific litter compounds (Gessner et al., 2010). Some studies showed that non-additive litter effect of the mixed-species would increase with litter species richness (Hättenschwiler et al., 2005). Other studies reported that species identity within litter mixtures surpassed litter richness as the main driver of LME (Wardle et al., 1997; Zak et al., 2003). For example, it has been revealed that antagonistic LME was strongest in three most-recalcitrant litter species along the diversity gradient from one to six species litter mixtures (Hättenschwiler and Gasser, 2005). More importantly, evidence suggested that LME may not occur if litter mixtures are composed of chemically similar species, it occurs frequently in chemically dissimilar species (Meier and Bowman, 2008).
Litter quality was generally represented by the concentration of nitrogen (N), carbon (C) and lignin (L) (Haettenschwiler and Jorgensen, 2010). Litter N accelerated the decomposition process, and litter L was partially retained and converted into humus stored in the soil (Taylor et al., 1989; Cotrufo et al., 2013; Li et al., 2021). However, a study showed that increasing lignin concentration increased photodegradation process (Austin and Ballare, 2010). This discrepancy might because of the fact that other litter chemical traits, such as cellulose (Ce), phenols (Phe), and condensed tannin (Ct), could also be important in driving decomposition process (Hättenschwiler and Vitousek, 2000; Kraus et al., 2004; Yang et al., 2021). Indeed, there is evidence suggest that micronutrients prevent plant residue from being decayed, although they are presented in rather small amounts (Berg and Mcclaugherty, 2003). Additionally, although there are a great number of studies focusing on the effect of litter mixture on litter mass loss, little is known about on mutli-trophic soil processes, such as soil carbon cycling. Therefore, to better understand the underlying mechanistic links between litter mixture chemistry and soil carbon dynamics, LME studies should assess many more litter chemical traits, particular for those that are not commonly considered.
Unfortunately, litter chemical components differed by tens to hundreds of orders, the role of these micronutrient was usually masked by macronutrients (Berg and Mcclaugherty, 2003). Additionally, litter chemical traits themselves interacted with each other. For instance, cellulose can be bound by lignin to form stable compounds and tannins can precipitate N substrates in protein-tannin complexes (Kraus et al., 2004; Talbot et al., 2012). Therefore, to better understand how litter chemical traits influencing LME, we employed principal component analysis (PCA) to characterize litter chemical composition, which has been carried out effective in several studies (Epps et al., 2007; Meier and Bowman, 2008; Meier and Bowman, 2010). PCA was adopted because it 1) included the presence and quantity of various litter chemical properties; 2) scaled the values of variables to one before PCA analysis to increase sensitivity of micronutrients; 3) considered interactions among litter chemical traits.
Northern China’s agro-pastoral ecotone is a typical ecologically fragile semi-arid zone, which is a key area for the ambitious large-scale afforestation efforts Three-North Shelterbelt Development Program (TNSDP) (Hong et al., 2018; Wang et al., 2020). The tree Populus simonii, shrubs Artemisia halondendron and Caragana microphylla were commonly planted either singly or together during afforestation because of their high tolerance against drought, wind erosion, and sand burial (Li et al.,2013b; Li et al., 2017; Luo et al., 2020). Afforestation and shelterbelt establishment has greatly reduced soil erosion and sandstorms (Zeng et al., 2008; Wang et al., 2019), however, the plantation of trees and shrubs thoroughly changed the vegetation composition, affected soil C availability, induced litter mixing interaction, and consequently altered the long-term C balance. This critical afforestation in grassland also provides a rare opportunity to investigate effects of tree, shrub, and grass litter mixing effect on soil carbon cycling. Following nutrients transfer and complementary concept (Meier and Bowman, 2008), we hypothesized that LME intensity would increase with litter species richness, and LME is strongest in four litter mixtures. As for litter chemical trats, we hypothesized that similar to litter C and N content, litter micronutrients such as litter Phe and Ct are also negatively related to the late-stage soil C flux.
The study site is situated in Naiman Desertification Research Station, Chinese Academy of Sciences, an area within the agro-grazing ecotone in eastern Inner Mongolia, China (42°55′N and 120°41′E, 350 m above sea level) (Supplementary Figure S1). The long-term mean annual temperature and mean annual precipitation are 6.4°C and 360 mm, respectively. The mean annual wind speed is 3.4–4.1 m s−1 (Zhao et al., 2006). The soil consists mainly of chestnut soil according to the Chinese classification and is a Haplic Calcisol according to the FAO (Su et al., 2006). Therefore, litter input and decomposition are important for improving soil quality and nutrient status in the region (Li Y. L. et al., 2013). Over the last 40 years, many agroforestry practices have been conducted to counteract soil erosion and reduce land degradation in this area (Li et al., 2017). Shrubs such as Artemisia halodendron and Caragana microphylla play an important role in the re-establishment of degraded ecosystems (Zhu et al., 1989). Artemisia halodendron, a typical sub-shrub with a high ability to capture deep water, grows and propagates rapidly in sandy ecosystems due to its low nutrient requirements and capacity for vegetative propagation (Luo et al., 2020). Caragana microphylla, a pioneer leguminous shrub species for vegetation re-establishment, has been widely reported for its adaptation to windy and sandy environment (Zhang et al., 2006). The vegetation is thus characterized by grasses (e.g., Pennisetum centrasiatium), with scattered shrubs (e.g., Artemisia halondendron and Caragana microphylla) and windbreak tree belts of Populus simonii.
In September 2018, we sampled litter material of Artemisia halodendron (Ah), Caragana microphylla (Cm), Pennisetum centrasiatium (Pc) and Populus simonii (Ps). The litter material was brought back to the laboratory and oven-dried at 65°C for 24 h. To test how litter species richness and chemical composition affects the decomposition of litter mixture, we mixed four litter materials in all possible 2- species (1:1), 3- species (1:1:1), and 4-species (1:1:1:1) ways. Therefore, there were fourteen litter material type (Ah, Cm, Pc, Ps, AhCm, AhPc, AhPs, CmPc, CmPs, PcPs, AhCmPc, AhCmPs, AhPcPs, and AhCmPcPs). Two grams of litter material, following the natural yearly litter quantity in this region (Wang et al., 2018), were ball-milled into powder (GT300 Ball Mill, POWTEQ, Beijing, China) and then homogenized by sieving through a 0.25 mm mesh to minimize effect of size and structure of the litter material. In addition, there were five replicates in each leaf litter treatment. We collected top soil (0–10 cm) in a dune meadow, and then sieved (2 mm mesh), homogenized in the laboratory.
The prepared 2 g litter powder was mixed thoroughly with 200 g dry-weight soil in a 500-ml canning jar, and there were five replicates without litter addition for the control treatment. Thus, there were 15 × 5 = 75 incubation jars. The incubation jars were kept at 21–25°C in an incubator (SPX-500; Jiangnan, Ningbo, China), and the soil-litter mixture inside the jar was maintained at 60% water-holding capacity through the gravimetric method. Moreover, the incubation here was kept for 113 days, which was based on our previous study showing that the CO2 release rate was decreased to 0 when incubation duration exceeded 100 days (Yang et al., 2019).
Litter C and N concentration were analyzed with element analyzer (Costech ECS 4010). Lignin concentration was analyzed using a modified acetyl bromide method (Iiyama and Wallis, 1990). Soluble sugar (Ss) concentration was determined using the anthrone method (Helbert and Brown, 2002). Litter cellulose content was measured according to the acid hydrolysis method (Updegraff, 1969). The concentration of phenols was determined using the Folin-Ciocalteu method (Waterman and Mole, 1998). The concentration of condensed tannins was measured following the acid butanol method described by Hagerman (Porter et al., 1986). These chemical traits were selected because they were shown to have great influence on litter decomposition and soil C cycling (Taylor et al., 1989; Hättenschwiler and Vitousek, 2000; Talbot and Treseder, 2012).
We evaluated soil C dynamics by measuring the C flux daily with an infra-red gas analyzer (Li-Cor 840A CO2/H2O) for the first 7 days, every second day from day 7 to day 21, and every 5th day from day 22 to day 72, and then every 15 days from day 73 to day 113. Data were collected every second for 2 min. Data from the middle 100 s were analyzed. The unary linear slope method was used to calculate the rate of CO2 increase in the sample. The CO2 release rate was calculated using the following formula:
Fc: CO2 release rate; V: volume of the jar from which the soil portion is removed; R: gas constant; dc'/dt: slope--adjusted rate of CO2 efflux change; Wav: water vapor partial pressure in the jar during measurement period; Pav: average atmospheric pressure in the jar; and Tav: The average temperature in the jar. The total CO2 emission efflux was calculated by plotting the CO2 release rate versus time and obtaining the area under the curve.
The CO2 release rate in 1–28 days was more than ten times that in 29–113 days. According to dynamics of CO2 release rate, the whole culture process was divided into two stages: early stage (0–28 days) and late stage (29–111 days).
We compared the observed values and expected values to evaluate non-additive litter mixing effects (LME) using the following formula:
The expected CO2 efflux of the litter mixture was the average CO2 release efflux of component litter species in the litter mixtures. The observed CO2 release efflux of the litter mixture was measured CO2 release efflux.
Significant differences in litter chemical traits, cumulative CO2 fluxes, and litter-mixing effects were detected by one-way analysis of variance (ANOVA) followed by least significant difference (LSD) in SPSS version 17.0 (SPSS Inc., Chicago, IL, United States). Principal component analysis (PCA) was carried out to show the litter chemical distance among litter species and mixtures. Structural equation model (SEM) was used to evaluate the direct and indirect effects of litter species richness and litter chemical traits on CO2 flux. The PCA and SEM were conducted using package “vegan” and “lavaan” by R software version 4.0.3 (R Development Core Team, 2014).
The initial nutrient content of single-species litters and litter mixtures are shown in Table 1 and Figure 1. All seven initial litter chemical properties significantly differed among four single-species. The N content in Cm plant litter was 36.84 ± 0.14 mg g−1 which was 2.1, 5, and 5.5 times higher than that in Ah, Pc, and Ps litter. The C content in Cm (445.22 ± 0.71 mg g−1) was also higher than that in Ah (441.59 ± 0.15 mg g−1), Pc (400.43 ± 0.59 mg g−1), and Ps (410.33 ± 0.17 mg g−1) litters. The L and Phe concentration of Ah litter (160.37 ± 2.13 mg g−1 and 61.66 ± 0.24 mg g−1, respectively) was higher than other three plant litter. The phenols concentration in Cm (36.76 ± 0.23 mg g−1) and Ps (13.83 ± 0.19 mg g−1) litter was 60% and 22% of that in Ah litter, respectively. The highest Ss and Ct content was observed in Ps litter. The Ss content in Pc (12.90 ± 0.65 mg g−1) was only 18% of that in Ps litter (71.12 ± 0.37 mg g−1), and the Ct in Cm litter (8.94 ± 0.24 mg g−1) was only 1/10 of that in Ps litter. The cellulose content was 401.22 ± 1.75 mg g−1 in Pc litter, which was 2.3 times as much as in Ps litter. The highest L and phenols content were observed in Ah litter, and the highest N and C content were observed in Cm litter, the highest cellulose content was observed in Pc litter, and the highest Ss and Ct content were shown in Ps litter.
FIGURE 1. Principal components plot of litter quality index measured for Artemisia halodendron (Ah), Caragana microphylla (Cm), Pennisetum centrasiatium (Pc), and Populus simonii (Ps), Artemisia halodendron+ Caragana microphylla (AhCm), Artemisia halodendron+ Pennisetum centrasiatium (AhPc), Artemisia halodendron+ Populus simonii (AhPs), Caragana microphylla+ Pennisetum centrasiatium (CmPc), Caragana microphylla+ Populus simonii (CmPs), Pennisetum centrasiatium+ Populus simonii (PcPs), Artemisia halodendron+ Caragana microphylla+ Pennisetum centrasiatium (AhCmPc), Artemisia halodendron+ Caragana microphylla+ Populus simonii (AhCmPs), Caragana microphylla+ Pennisetum centrasiatium+ Populus simonii (CmPcPs), Artemisia halodendron+ Caragana microphylla+ Pennisetum centrasiatium+ Populus simonii (AhCmPcPs).
Values of the litter mixtures’ chemical properties were between those of the single-species litters (Table 1; Figure 1). Among two-species litter mixtures, the N, C, and L content were highest in the AhCm litter mixture, and Ss, Phe, and Ct concentrations were highest in the AhPs litter mixture. The cellulose concetration in the AhPc litter mixture was 306.86 ± 1.45 mg g−1, which was 1.8 and 2.5 times higher than that in AhPs and AhCm litter mixtures. Among three and four-species litter mixtures, the N content was highest in AhCmPc litter, the C, L, Ss, Phe, and Ct content were highest in AhCmPs litter; the Phe and Ct content were highest in AhPcPs litter; and the L and cellulose content were highest in AhCmPcPs litter.
Litter chemical distance was shown by PCA (Figure 1). The first two principal components (PC1 and PC2) explained 85.9% of the variation in litter chemistry, PC3 accounted for 7.7% of the variation in chemical traits (data not shown). PC1 explained 51.8% of the variation in litter chemical traits and revealed a gradient in the Ce, Ss, Phe, L, and Ce concentration. PC2 accounted for 34.1% of the variation in litter chemical traits and was highly correlated with the Ct, C, and N content (Supplementary Figure S2).
Dynamics of CO2 release among different litter addition treatments are shown in Figure 2. The CO2 release rate in the control remained low during the whole incubation, which was approximately 1.54 ± 0.25 μg CO2-C g−1 soil. The CO2 release rate in litter input treatments showed a similar temporal pattern: increasing sharply to the maximum in the first 3 days after litter addition, and then decreasing for the next 4 days, thereafter decreasing gradually with time until the end of the incubation period (Supplementary Figure S3; Figure 2). For the first 3 days, the CO2 release rate in the Ah and Cm input treatments increased to 182.63 ± 20.01 μg CO2-C g−1 soil and 122.99 ± 26.98 μg CO2-C g−1 soil, whereas in the Pc and Ps addition treatments, the CO2 release rate was only increased to 42.48 ± 10.97 μg CO2-C g−1 soil and 60.00 ± 13.04 μg CO2-C g−1 soil, respectively. The CO2 release rate in AhCm was 146.64 ± 18.92 μg CO2-C g−1 soil, the CO2 release rate in PcPs was 37.29 ± 8.16 μg CO2-C g−1 soil. For three and four-species litter mixtures, the CO2 release rate ranged from 77.06 ± 12.45 μg CO2-C g−1 soil in the AhCmPc treatment to 104.96 ± 21.71 μg CO2-C g−1 soil in AhPcPs treatment. From day 3 to day 28, the CO2 release rate in single-species litter and litter mixtures ranged from 35.53 ± 6.70 μg CO2-C g−1 soil in AhPs litter to 62.38 ± 7.85 μg CO2-C g−1 soil in the Ah litter treatment. From day 28 to day 111, the CO2 release rate in single-species litters and litter mixtures ranged from 5.04 ± 1.63 μg CO2-C g−1 soil in Cm litter to 10.09 ± 2.65 μg CO2-C g−1 soil in PcPs litter. In sum, the CO2 release rate among the various litter inputs differed substantially in the early stage (1–28th day), but did not differ as much in the late stage (29th–113rd day).
FIGURE 2. Dynamics of CO2 emission fluxes in control and litter addition treatments for single species, two species mixtures, and three and four species mixtures. All means are ±SE, 5 replicates per treatment.
In accordance with CO2 release rate, total CO2 fluxes in control was low, which was only 7.7% of that in litter addition treatments. Among the single-species litters, the total CO2 fluxes in Ah litter was 1909.63 ± 127.37 μg CO2-C g−1 soil, which was significantly higher than that in Cm litter (1466.43 ± 60.04 μg CO2-C g−1 soil) and Ps litter (1341.22 ± 63.43 μg CO2-C g−1 soil). Interestingly, among two-species litter mixture treatments, the cumulative CO2 flux in CmPc litter was highest, at 1663.93 ± 72.09 μg CO2-C g−1 s, and cumulative CO2 fluxes in AhPs litter was lower than others, which was 1270.36 ± 49.45 μg CO2-C g−1 soil. As described above, we found that mixing of more diverse litters induced smaller cumulative CO2 fluxes, while the mixing of more similar litters produced higher cumulative CO2 fluxes. Among three and four-species litter mixtures, total cumulative CO2 fluxes in AhCmPcPs was 1432.67 ± 42.20 μg CO2-C g−1 soil, which was lower than that of AhCmPc (1667.47 ± 48.47 μg CO2-C g−1 soil) and AhCmPs (1664.86 ± 29.77 μg CO2-C g−1 soil) (Figure 3).
FIGURE 3. Total CO2 fluxes in control and litter treatments (A) and cumulative CO2 fluxes in different species richness treatments (B). All means are ±SE, 5 replicates per treatment. Capital letters indicate the difference in single litter difference; Lowercase letters indicate the difference of two litter species mixture; and the difference in CO2 fluxes among three and four litter mixtures was also labeled by capital letters (ANOVA followed by LSD test, p < 0.05).
At the beginning, litter-mixing effect (LME) was strong and negative, thereafter degree of intensity tapers off until the direction of LME in several litter treatments changed to positive before day 28. Specifically, the LMEs in CmPc, CmPs, and PcPs litter mixture treatments were negative at first but changed to positive at day 22; the LMEs were negative throughout the incubation period for AhCm, AhPc, and AhPs treatments. The LMEs were negative throughout the incubation period for AhCmPc, AhPcPs, and AhCmPcPs, in AhCmPs and CmPcPs, the LMEs were initially negative and then became positive after day 28. Therefore, total CO2 fluxes and the LME were divided into two stages: day 1 to day 28 was the early stage, and day 28 to day 113 was the late stage (Figure 4).
FIGURE 4. Dynamics of litter-mixing effects (LME) on CO2 emission rate for two species, three and four species mixtures. Dotted lines indicate the point at which observed values (O) equal to expected values (E).
The LME on cumulative CO2 emission was negative in the early stage, while some of the LME was positive in the late stage (Figure 5). For two-species litter mixtures in the early-stage, the litter-mixing intensity in AhPc and AhPs was −2.88 ± 0.15 and −2.99 ± 0.14, respectively, which was more strongly than that in AhCm (−1.14 ± 0.14) and CmPs (−1.42 ± 0.15). For three and four-species litter mixtures, the LME in AhCmPc, AhPcPs, and AhCmPcPs was −2.54 ± 0.16, −1.89 ± 0.15, and −2.23 ± 0.16, respectively, which was nearly 4–6 times stronger than that in CmPcPs and AhCmPs treatment. In contrast, the LME in the late-stage was 1.32 ± 0.04 in CmPc, which was significantly higher than that of CmPs (0.61 ± 0.09) and PcPs (0.71 ± 0.07). Conversely, the LME was antagonistic in AhCm, AhPc, and AhPs, and the intensity of mixing in AhPs was -2.13 ± 0.15, which was much stronger than that of AhCm litter (−1.15 ± 0.14). For three-species litter mixtures, the LME was synergistic in AhCmPs and CmPcPs, and there was no difference in the mixing intensity among them. Similarly, although the mixing effect was antagonistic in AhCmPc and AhPcPs, there was no difference in the mixing intensity among them. The mixing effect in the four-species litter mixture AhCmPcPs was −1.23 ± 0.13.
FIGURE 5. Litter-mixing effects (LME) on cumulative CO2 emission during (A,B) the Early stage (C,D) the Late stage for two, three and four species mixtures. All means are ±SE, 5 replicates per treatment. Lowercase letters indicate the difference in two litter species; Capital letters indicate the difference of three and four litter species mixture (ANOVA followed by LSD test, p < 0.05).
Structural equation model (SEM) suggested that the driving factors of early- and later-stage CO2 fluxes are completely different (Figure 6), indicating that the key litter chemistry factors affecting CO2 fluxes and LME varied with incubation time. The early-stage CO2 fluxes was directly affected by PC2 (litter C, N, and Ct concentration) and early-stage LME (R2 = 0.50), and the latter was mainly controlled by litter species richness. The late-stage CO2 fluxes was influenced by PC1 (litter Ce, Ss, Phe, and L concentration) and late-stage LME (R2 = 0.54).
FIGURE 6. Structural equation model (SEM) evaluating the direct and indirect effects of litter species richness and litter quality index on early-stage CO2 flux (A), late-stage CO2 flux (C), the standardized effects of these factors derived from the SEM (B,D). Red and black lines indicate negative and positive relationships, respectively; grey dotted lines indicate the relationship is not significant at p < 0.05 level; Number adjacent to arrows are standardized path coefficients, indicating the effect size of the relationship. * denotes significant difference at p < 0.5, ** denotes significant difference at p < 0.05, *** denotes significant difference at p < 0.01.
In line with previous studies (Meier and Bowman, 2010), our research showed that species richness was positively correlated to the short-term cumulative C mineralization, which was might because species mixing enhance the diversity of food resources and accelerate nutrient exchange (Harguindeguy et al., 2008; Liu et al., 2016). According to the nutrient transfer hypothesis, the high-quality litter allows nutrient transfer by fungal hyphae or leaching to the low-quality litter lead to a more rapid decomposition of poor-quality litter (Hättenschwiler et al., 2005). However, both additive LME and total CO2 fluxes were not linearly correlated to litter species richness (Epps et al., 2007; Harguindeguy et al., 2008). In this study, both synergistic and antagonistic mixing effects were observed in roughly equal proportions, implying that 1) Litter mixing was a short-term ecological process that tapers off over time (Chen et al., 2017); 2) Chemically similar litter mixing does not induce a litter mixing effect (Meier and Bowman, 2008). Hence, chemical variation in litter mixture determined magnitude and direction of non-additive LME, which might be the key factors driving CO2 fluxes (Epps et al., 2007; Zhang et al., 2014; Mao et al., 2015).
Within a given climate regime, litter quality is the main driver of the litter decomposition rate (Aerts, 1997). High-quality litter (high C and N concentration) accelerates the decomposition of low-quality litter and produces a positive mixing effect (Hoorens and Aerts, 2003; Zhang et al., 2014), our study provided evidence that litter C and N content were positively correlated to LME. Hard-to-decompose substances such as Ct within litter played a negative role in activating microbial activity (Coq et al., 2010). Furthermore, an increasing number of studies suggest that low-concentration litter compounds interact with other compounds accelerating or inhibiting microbial decomposition. For example, tannins and phenols can precipitate N-containing substrates in protein-tannin complexes (Kraus et al., 2004) and phenol-protein complexes (Hättenschwiler and Vitousek, 2000), which can only be utilized by fungi and earthworms with moderate levels of enzyme activity. Moreover, transfer of toxic compounds or phenolics between litter species is thought to inhibit decomposition via enzyme inhibition and substrate deprivation (Mutabaruka et al., 2007; Freschet et al., 2012). Fortunately, scaling the value of variables to one before PCA in our study have increased sensitivity to these compounds that would not otherwise be detectable (Meier and Bowman, 2008).
What is interesting is that in our study, effects of litter chemical compounds varied with decomposition stages (Berg, 2008). In early stage of decomposition, the large amount of litter C and N compounds accompanied with litter addition provided sufficient energy and nutrients to activate microorganisms (Vestgarden, 2001). However, in the later stage, litter C and N content was negatively correlated to total CO2 flux. Additionally, litter Ce, Ss, Phe, and L content, which have no relation to early-stage CO2 flux, were closely correlated to late-stage CO2 flux. This result was in line with the previous study showing that N control litter decay rates during first phase of decay, while L content becomes more important thereafter (Berg, 1984; Taylor et al., 1989). As decomposition proceeds, the C and N was depleted and the proportion of L and Ce increase, and at some point where L control begins and becomes stronger through time (Berg, 2008). Moreover, Staaf and Berg 1982 emphasized that in the late-stage, L-controlled phase were normally proportional to the litter decomposition and soil respiration (Staaf and Berg 1982). Together with previous studies, we provided evidence that effects of litter richness and chemical traits on soil C cycling changed over decomposition stages.
Afforestation in agro-pastoral ecotone was expected to be a good tool for restoring degraded soils and ecosystems (Watson et al., 2000). However, not all trees can be grown everywhere, interlaced planting of trees and shrubs on degraded grassland does not necessarily maximize soil C conservation (Deng et al., 2014; Liu et al., 2018). Artemisia halodendron is a sand-fixing shrub species that has been widely mixed planted with other trees or shrubs in severely degenerated sites in North China (Luo et al., 2020). Unfortunately, the results showed that cumulative CO2 fluxes in A. halodendron is relatively high, but CO2 fluxes is decreased and LME was intensive when A. halodendron is mixed with other species. The results suggest that the restoration of degraded land might be accelerated with widely plantations of exclusively A. halodendron but slowed down when mixed with other plant species. Therefore, afforestation species and management type should be carefully considered and planting should be guided by indigenous knowledge and local communities (Hong et al., 2020).
Our results revealed that tree and shrub litter addition to grasslands significantly increased the emission of CO2, especially in the early-stage that soil CO2 flux was 1.75 times higher than that in the late-stage. We found that both litter richness and litter quality affected LME and soil CO2 flux. Importantly, we provided evidence that dominant litter chemical factors that influence soil CO2 flux changed over time, with litter C, N and Ct controlled the early-stage CO2 flux, while all litter chemical traits drove the late-stage CO2 flux. Our result opens a new perspective for improving our understanding of soil C cycling in agro-pastoral ecotone after large-scale afforestation.
The original contributions presented in the study are included in the article/Supplementary Material, further inquiries can be directed to the corresponding author.
Conceptualization: HY and YLi; Methodology: HY and JZ; Writing-original draft preparation: HY; Writing-review and editing: HY, YLi, CB, and YLu. All authors have read and agreed to the published version of the manuscript.
Key Science and Technology Program of Inner Mongolia (2019ZD00704, 2021ZD001505), National Natural Science Foundation of China (32071845), Strategic Priority Research Program of the Chinese Academy of Sciences (XDA23060404).
The authors declare that the research was conducted in the absence of any commercial or financial relationships that could be construed as a potential conflict of interest.
All claims expressed in this article are solely those of the authors and do not necessarily represent those of their affiliated organizations, or those of the publisher, the editors and the reviewers. Any product that may be evaluated in this article, or claim that may be made by its manufacturer, is not guaranteed or endorsed by the publisher.
The Supplementary Material for this article can be found online at: https://www.frontiersin.org/articles/10.3389/fenvs.2022.1023831/full#supplementary-material
Aerts, R. (1997). Climate, leaf litter chemistry and leaf litter decomposition in terrestrial ecosystems: A triangular relationship. Oikos 79, 439–449. doi:10.2307/3546886
Austin, A. T., and Ballare, C. L. (2010). Dual role of lignin in plant litter decomposition in terrestrial ecosystems. Proc. Natl. Acad. Sci. U. S. A. 107, 4618–4622. doi:10.1073/pnas.0909396107
Berg, B., and Mcclaugherty, C. (2003). Plant litter. Decomposition, humus formation, carbon sequestration. Berlin, Germany: Springer-Verlag Berlin Heidelberg.
Berg, B. (2008). Nutrient release from litter and humus in coniferous forest soils—A mini review. Scand. J. For. Res. 1, 359–369. doi:10.1080/02827588609382428
Chen, Y. C., Ma, S. Q., Sun, J., Wang, X. D., Cheng, G. W., and Lu, X. Y. (2017). Chemical diversity and incubation time affect non -additive responses of soil carbon and nitrogen cycling to litter mixtures from an alpine steppe soil. Soil Biol. Biochem. 109, 124–134. doi:10.1016/j.soilbio.2017.02.007
Coq, S., Souquet, J. M., Meudec, E., Cheynier, V., and Hättenschwiler, S. (2010). Interspecific variation in leaf litter tannins drives decomposition in a tropical rain forest of French Guiana. Ecology 91, 2080–2091. doi:10.1890/09-1076.1
Cotrufo, M. F., Wallenstein, M. D., Boot, C. M., Denef, K., and Paul, E. (2013). The microbial efficiency-matrix stabilization (MEMS) framework integrates plant litter decomposition with soil organic matter stabilization: Do labile plant inputs form stable soil organic matter? Glob. Chang. Biol. 19, 988–995. doi:10.1111/gcb.12113
Deng, L., Liu, G. B., and Shangguan, Z. P. (2014). Land-use conversion and changing soil carbon stocks in China's 'grain-for-green' Program: A synthesis. Glob. Chang. Biol. 20, 3544–3556. doi:10.1111/gcb.12508
Epps, K. Y., Comerford, N. B., Reeves, J. B., Cropper, W. P., and Araujo, Q. R. (2007). Chemical diversity – highlighting a species richness and ecosystem function disconnect. Oikos 116, 1831–1840. doi:10.1111/j.0030-1299.2007.15853.x
Freschet, G. T., Aerts, R., and Cornelissen, J. H. C. (2012). A plant economics spectrum of litter decomposability. Funct. Ecol. 26, 56–65. doi:10.1111/j.1365-2435.2011.01913.x
Gartner, T. B., and Cardon, Z. G. (2004). Decomposition dynamics in mixed-species leaf litter. Oikos 104, 230–246. doi:10.1111/j.0030-1299.2004.12738.x
Haettenschwiler, S., and Jorgensen, H. B. (2010). Carbon quality rather than stoichiometry controls litter decomposition in a tropical rain forest. J. Ecol. 98, 754–763. doi:10.1111/j.1365-2745.2010.01671.x
Harguindeguy, N. P., Blundo, C. M., Gurvich, D. E., Diaz, S., and Cuevas, E. (2008). More than the sum of its parts? Assessing litter heterogeneity effects on the decomposition of litter mixtures through leaf chemistry. Plant Soil 303, 151–159. doi:10.1007/s11104-007-9495-y
Hättenschwiler, S., and Gasser, P. (2005). Soil animals alter plant litter diversity effects on decomposition. Proc. Natl. Acad. Sci. U. S. A. 102, 1519–1524. doi:10.1073/pnas.0404977102
Hättenschwiler, S., Tiunov, A. V., and Scheu, S. (2005). Biodiversity and litter decomposition in terrestrial ecosystems. Annu. Rev. Ecol. Evol. Syst. 36, 191–218. doi:10.1146/annurev.ecolsys.36.112904.151932
Hättenschwiler, S., and Vitousek, P. M. (2000). The role of polyphenols in terrestrial ecosystem nutrient cycling. Trends Ecol. Evol. 15, 238–243. doi:10.1016/s0169-5347(00)01861-9
Helbert, J. R., and Brown, K. D. (2002). Color reaction of anthrone with monosaccharide mixtures and oligo- and polysaccharides containing hexuronic acids. Anal. Chem. 29, 1464–1466. doi:10.1021/ac60130a020
Hong, S. B., Yin, G. D., Piao, S. L., Dybzinski, R., Cong, N., Li, X. Y., et al. (2020). Divergent responses of soil organic carbon to afforestation. Nat. Sustain. 3, 694–700. doi:10.1038/s41893-020-0557-y
Hong, S., Piao, S., Chen, A., Liu, Y., Liu, L., Peng, S., et al. (2018). Afforestation neutralizes soil pH. Nat. Commun. 9, 520. doi:10.1038/s41467-018-02970-1
Hoorens, B., Aerts, R., and Stroetenga, M. (2003). Does initial litter chemistry explain litter mixture effects on decomposition? Oecologia 137, 578–586. doi:10.1007/s00442-003-1365-6
Iiyama, K., and Wallis, A. F. A. (1990). Determination of lignin in herbaceous plants by an improved acetyl bromide procedure. J. Sci. Food Agric. 51, 145–161. doi:10.1002/jsfa.2740510202
Kraus, T. E. C., Zasoski, R. J., Dahlgren, R. A., Horwath, W. R., and Preston, C. M. (2004). Carbon and nitrogen dynamics in a forest soil amended with purified tannins from different plant species. Soil Biol. Biochem. 36, 309–321. doi:10.1016/j.soilbio.2003.10.006
Li, C. Y., Peng, F., Lai, C. M., Xue, X., You, Q. G., Chen, X. J., et al. (2021). Plant community changes determine the vegetation and soil δ 13 C and δ 15 N enrichment in degraded alpine grassland. Land Degrad. Dev. 32, 2371–2382. doi:10.1002/ldr.3912
Li, Y. L., Chen, J., Cui, J. Y., Zhao, X. Y., and Zhang, T. H. (2013a). Nutrient resorption in Caragana microphylla along a chronosequence of plantations: Implications for desertified land restoration in North China. Ecol. Eng. 53, 299–305. doi:10.1016/j.ecoleng.2012.12.061
Li, Y. Q., Brandle, J., Awada, T., Chen, Y. P., Han, J. J., Zhang, F. X., et al. (2013b). Accumulation of carbon and nitrogen in the plant-soil system after afforestation of active sand dunes in China's Horqin Sandy Land. Agric. Ecosyst. Environ. 177, 75–84. doi:10.1016/j.agee.2013.06.007
Li, Y. Q., Chen, Y. P., Wang, X. Y., Niu, Y. Y., and Lian, J. (2017). Improvements in soil carbon and nitrogen capacities after shrub planting to stabilize sand dunes in China's horqin sandy land. Sustainability 9, 662. doi:10.3390/su9040662
Liu, C. C., Liu, Y. G., Guo, K., Zhao, H. W., Qia, X. G., Wang, S. J., et al. (2016). Mixing litter from deciduous and evergreen trees enhances decomposition in a subtropical karst forest in southwestern China. Soil Biol. Biochem. 101, 44–54. doi:10.1016/j.soilbio.2016.07.004
Liu, X., Yang, T., Wang, Q., Huang, F., and Li, L. (2018). Dynamics of soil carbon and nitrogen stocks after afforestation in arid and semi-arid regions: A meta-analysis. Sci. Total Environ. 618, 1658–1664. doi:10.1016/j.scitotenv.2017.10.009
Luo, Y. Q., Zhao, X. Y., Ding, J. P., Li, Y. Q., Liu, X. P., Wang, T., et al. (2020). Soil respiration dynamics in a semi-fixed sand dune under the pioneer shrub Artemisia halodendron Turcz. ex Bess. in the Horqin sandy land, northeastern China. Arid Land Res. Manag. 34, 36–51. doi:10.1080/15324982.2019.1629675
Mao, B., Yu, Z. Y., and Zeng, D. H. (2015). Non-additive effects of species mixing on litter mass loss and chemical properties in a Mongolian pine plantation of Northeast China. Plant Soil 396, 339–351. doi:10.1007/s11104-015-2593-3
Meier, C. L., and Bowman, W. D. (2010). Chemical composition and diversity influence non-additive effects of litter mixtures on soil carbon and nitrogen cycling: Implications for plant species loss. Soil Biol. Biochem. 42, 1447–1454. doi:10.1016/j.soilbio.2010.05.005
Meier, C. L., and Bowman, W. D. (2008). Links between plant litter chemistry, species diversity, and below-ground ecosystem function. Proc. Natl. Acad. Sci. U. S. A. 105, 19780–19785. doi:10.1073/pnas.0805600105
Mutabaruka, R., Hairiah, K., and Cadisch, G. (2007). Microbial degradation of hydrolysable and condensed tannin polyphenol–protein complexes in soils from different land-use histories. Soil Biol. Biochem. 39, 1479–1492. doi:10.1016/j.soilbio.2006.12.036
Porter, L. J., Hrstich, L. N., and Chan, B. G. (1986). The conversion of procyanidins and prodelphinidins to cyanidin and delphinidin. Phytochemistry 25, 223–230. doi:10.1016/s0031-9422(00)94533-3
Singhal, V., Roy, T., Singh, C., and Ghosh, J. (2021). Effect of incubation time, litter diversity and species richness on decomposition dynamics of tree species from Western Himalayas. Catena 203, 105281. doi:10.1016/j.catena.2021.105281
Su, Y. Z., Li, Y. L., and Zhao, H. L. (2006). Soil properties and their spatial pattern in a degraded sandy grassland under post-grazing restoration, Inner Mongolia, northern China. Biogeochemistry 79, 297–314. doi:10.1007/s10533-005-5273-1
Swift, M. J., Heal, W. O., and Anderson, J. M. (1979). Decomposition in terrestrial ecosystems. Stud. Ecol. 5, 2772–2774.
Talbot, J. M., and Treseder, K. K. (2012). Interactions among lignin, cellulose, and nitrogen drive litter chemistry-decay relationships. Ecology 93, 345–354. doi:10.1890/11-0843.1
Talbot, J. M., Yelle, D. J., Nowick, J., and Treseder, K. K. (2012). Litter decay rates are determined by lignin chemistry. Biogeochemistry 108, 279–295. doi:10.1007/s10533-011-9599-6
Taylor, B. R., Parkinson, D., and Parsons, W. F. J. (1989). Nitrogen and lignin content as predictors of litter decay-rates - a microcosm test. Ecology 70, 97–104. doi:10.2307/1938416
Updegraff, D. M. (1969). Semimicro determination of cellulose in biological materials. Anal. Biochem. 32, 420–424. doi:10.1016/s0003-2697(69)80009-6
Vestgarden, L. S. (2001). Carbon and nitrogen turnover in the early stage of Scots pine (pinus sylvestris L.) needle litter decomposition: Effects of internal and external nitrogen. Soil Biol. Biochem. 33, 465–474. doi:10.1016/s0038-0717(00)00187-5
Wang, X. Y., Li, Y. G., Gong, X. W., Niu, Y. Y., Chen, Y. P., Shi, X. P., et al. (2019). Storage, pattern and driving factors of soil organic carbon in an ecologically fragile zone of northern China. Geoderma 343, 155–165. doi:10.1016/j.geoderma.2019.02.030
Wang, X. Y., Li, Y. Q., Gong, X. W., Niu, Y. Y., Chen, Y. P., Shi, X. P., et al. (2020). Changes of soil organic carbon stocks from the 1980s to 2018 in northern China's agro-pastoral ecotone. Catena 194, 104722. doi:10.1016/j.catena.2020.104722
Wang, X. Y., Li, Y. Q., Lian, J., Niu, Y., Gong, X. W., Yang, H., et al. (2018). Inter- and intra-annual dynamics of vegetation litter at different habitats in Horqin Sandy Land, China. Chin. J. Appl. Ecol. 29 (5), 1494–1502. doi:10.13287/j.1001-9332.201805.005
Wardle, D. A., Bonner, K. I., and Nicholson, K. S. (1997). Biodiversity and plant litter Experimental evidence which does not support the view that enhanced species richness improves ecosystem function. Oikos 79, 247. doi:10.2307/3546010
Waterman, P. G., and Mole, S. (1998). Analysis of phenolic plant metabolites. Blackwell Scientific Publication.
Watson, R. T., Noble, I. R., Bolin, B., Ravindranath, N. H., and Dokken, D. J. (2000). Land use, land-use change, and forestry : A special report of the IPCC.
Yang, H., Li, Y., Ning, Z., and Zhang, Z. Q. (2019). Effects of mixed litter on organic carbon mineralization in a dune grassland. Acta Ecol. Sin. 39, 2510–2519.
Yang, H. L., Li, Y. L., Wang, S. K., Zhan, J., Ning, Z. Y., and Han, D. (2021). The response of critical microbial taxa to litter micro-nutrients and macro-chemistry determined the agricultural soil priming intensity after afforestation. Front. Microbiol. 12, 730117. doi:10.3389/fmicb.2021.730117
Zak, D. R., Holmes, W. E., White, D. C., Peacock, A. D., and Tilman, D. (2003). Plant diversity, soil microbial communities, and ecosystem function: Are there any links? Ecology 84 (8), 2042–2050. doi:10.1890/02-0433
Zeng, D. H., Hu, Y. L., Chang, S. X., and Fan, Z. P. (2008). Land cover change effects on soil chemical and biological properties after planting Mongolian pine (Pinus sylvestris var. mongolica) in sandy lands in Keerqin, northeastern China. Plant Soil 317, 121–133. doi:10.1007/s11104-008-9793-z
Zhang, C. H., Li, S. G., Zhang, L. M., Xin, X. P., and Liu, X. R. (2014). Litter mixing significantly affects decomposition in the Hulun Buir meadow steppe of Inner Mongolia, China. J. Plant Ecol. 7, 56–67. doi:10.1093/jpe/rtt022
Zhang, T.-H., Su, Y.-Z., Cui, J.-Y., Zhang, Z.-H., and Chang, X.-X. (2006). A leguminous shrub (Caragana microphylla) in semiarid sandy soils of North China. Pedosphere 16, 319–325. doi:10.1016/s1002-0160(06)60058-1
Keywords: agro-grazing ecotone, non-additive litter-mixing effect, soil carbon dynamic, litter chemical traits, afforestation
Citation: Yang H, Li Y, Zhan J, Bao C and Luo Y (2022) Effects of litter chemical traits and species richness on soil carbon cycling changed over time. Front. Environ. Sci. 10:1023831. doi: 10.3389/fenvs.2022.1023831
Received: 20 August 2022; Accepted: 27 October 2022;
Published: 07 November 2022.
Edited by:
Dafeng Hui, Tennessee State University, United StatesReviewed by:
Rentao Liu, Ningxia University, ChinaCopyright © 2022 Yang, Li, Zhan, Bao and Luo. This is an open-access article distributed under the terms of the Creative Commons Attribution License (CC BY). The use, distribution or reproduction in other forums is permitted, provided the original author(s) and the copyright owner(s) are credited and that the original publication in this journal is cited, in accordance with accepted academic practice. No use, distribution or reproduction is permitted which does not comply with these terms.
*Correspondence: Yulin Li, bGl5bEBsemIuYWMuY24=
Disclaimer: All claims expressed in this article are solely those of the authors and do not necessarily represent those of their affiliated organizations, or those of the publisher, the editors and the reviewers. Any product that may be evaluated in this article or claim that may be made by its manufacturer is not guaranteed or endorsed by the publisher.
Research integrity at Frontiers
Learn more about the work of our research integrity team to safeguard the quality of each article we publish.