- 1Shaanxi Provincial Land Engineering Construction Group, Key Laboratory of Degraded and Unused Land Consolidation Engineering, Ministry of Natural Resources, Xi’an, China
- 2Shaanxi Engineering Research Center of Land Consolidation, Shaanxi Provincial Land Consolidation Engineering Technology Research Center, Xi’an, China
- 3Shaanxi Provincial Land Engineering Construction Group, Land Engineering Technology Innovation Center, Ministry of Natural Resources, Xi’an, China
- 4State Key Laboratory Base of Eco-Hydraulic Engineering in Arid Area, Xi’an University of Technology, Xi’an, China
Infiltration of stormwater through green surfaces is an important means of groundwater recharge. However, the increase in constructed impervious area caused by intensive urbanization has led to a reduction in rainwater infiltration over the past decade. The constructed rapid infiltration system (CRIS) with an ample open space plays an important role in groundwater recharge. This study aims to explore the influence of stormwater (roof runoff) concentration infiltration on the groundwater table and quality in the CRIS. Groundwater table monitoring is conducted for more than 2 years (October 2017–December 2019) by continuous online monitoring combined with manual sampling. Results show that the addition of zeolite to the CRIS has a good removal effect on rainfall runoff pollutants, and the influence of stormwater concentration infiltration on groundwater quality is small when the CRIS enters the stable running stage. The increased proportion of chemical oxygen demand, N, and P in J1 are all less than 10% from 2018 to 2019, and they are less than 20% for heavy metals. The stormwater concentration infiltration can recharge groundwater and increase the groundwater depth, and the groundwater depth varies from 0.5 m to 1.5 m during the monitoring period. The influence scope of the concentrated infiltration on the groundwater table and quality is between 25 and 45 m. The response of the groundwater table and quality to the stormwater concentration infiltration of J1, 25 m away from the CRIS, exhibits hysteresis, and the lag time is about 3–4 months. Conclusion from relevant research can provide important theoretical support for the further study of groundwater recharge by the CRIS.
Introduction
Urbanization has become a global trend with the rapid development of the economy and vast growth of the population, and coping with the extreme climate has been challenging for the urban storm drainage system. A series of serious ecological problems, such as urban flooding, waterlogging, no-point source pollution, and water shortage, have emerged from various activities. These problems have caused the greatest challenge for stormwater management (Guo et al., 2019). Infiltrating stormwater locally into the ground rather than discharging it to conventional pipe sewers is increasingly considered a means of controlling urban stormwater runoff. The utilization of stormwater is an effective measure to solve the overexploitation of groundwater and to prevent urban disasters (Bach et al., 2010; Zhou et al., 2017). As an important source, stormwater can alleviate the crisis of urban water shortage. At present, the comprehensive regulation of surface water, groundwater, and stormwater appears particularly important. Stormwater is frequently used to recharge groundwater and can increase groundwater levels and reduce rainfall runoff volume. The purpose of flood control and full utilization of the rainwater resource has been achieved (Zhai et al., 2017). Some industry-developed countries, such as Germany, Japan, Australia, and the United States, have formed mature rainwater utilization measures. Complete security systems have been established, and research on low-impact development has been gradually been conducted (Eisapour et al., 2010). China began to establish sponge cities in 2014 for achieving the optimal allocation of urban rainwater resources (Shao et al., 2016; Nguyen et al., 2019). At present, stormwater recharge measures mainly include surface infiltration, which allows rainwater to infiltrate the soil through permeable surfaces. Rain gardens, lawns, permeable pavements, river beaches, and the constructed rapid infiltration system (CRIS) are permeable surfaces with good infiltration conditions (Trowsdale and Simcock, 2011; Brown et al., 2012).
The CRIS is a sewage treatment technology developed from the sewage land treatment system (Yang et al., 2017; Su et al., 2020). It is usually filled with materials of good permeability, such as natural river sand, ceramsite, gravel, and coal gangue (Duan and Su. 2014; Zhao et al., 2019). The purpose is to replace the natural soil layer and to increase the hydraulic load of the system. The CRIS is operated in a dry and wet alternate manner. The pollutants in the CRIS are removed through the comprehensive action of filtration, adsorption and retention, physical and chemical reactions, and degradation of aerobic and anaerobic microorganisms (Kang, 2011).
The sewage treatment by the CRS to recharge groundwater has the risk of groundwater contamination (Al-Yamani et al., 2019; Vystavna et al., 2019). However, the use of stormwater to recharge groundwater is the most economical and safest means in terms of sewage infiltration. Recharge is what permits aquifers to remain active. It can fully utilize the limited water resources to achieve the purpose of raising the groundwater table and preventing land subsidence. Groundwater recharge considers the social, economic, and ecological benefits (Mensah et al., 2022). Substrate and depth-to-water-table determine how quickly surface water will enter the underground watercourse. Shallow, sandy aquifers close to the Earth’s surface recharge quicker than aquifers nestled hundreds of meters below the Earth’s surface (Gallagher, 2017). At present, subsurface infiltration structures, CRIS, are the most common types of infiltration systems. CRIS leads stormwater into aquifers through wells directly and eliminates the interception and adsorption of rainwater by plants and soil. The CRIS can easily cause groundwater pollution because most of its fillers are medium-coarse sand, and the water infiltration rate is relatively huge (Lee et al., 2012). Therefore, 5% of zeolite, as the improvement material, is added to the CRIS. the objectives of this project are as follows: 1) to explore the influence of stormwater concentration infiltration on groundwater quality in the CRIS; 2) to quantify the influence of stormwater concentration infiltration on the groundwater table; and 3) to estimate the rainfall runoff volume reduction rate and the pollutant removal rate with 5% of zeolite in the CRIS. Conclusions from the relevant research can provide important theoretical support for the further study of groundwater recharge by CRIS. They can also provide scientific and reasonable evaluation for the development of stormwater recharge measures.
Materials and methods
Description of the case study site and introduction of CRIS
The study area is located on a campus in Xianyang City, Shaanxi Province. The soil is mainly composed of silt loam. The bulk density is 1.35 g/cm3, comprising 9% clay, 80% sandy silt, and 10% sand. The city is situated on widely distributed loess soil that has a deep profile of more than 50 m. It has better geological conditions for the storage and migration of groundwater. The climate is semiarid and humid. The annual average temperature in Xianyang is 13.3°C, the rainfall is 580.2 mm, and the evaporation is 990 mm. The precipitation is uneven throughout the year, and more than 80% of rainfall occurs from May to October. This study involves a CRIS, and the study area is shown in Figure 1.
The CRIS was monitored to investigate the influence of stormwater concentration infiltration on the groundwater table and quality from October 2017 to December 2019. It was installed in July 2017 to collect and treat stormwater runoff from 1,100 m2 of concrete roof, road, and lawn. The CRIS is circular (diameter = 2.5 m, height = 3 m; area = 4.91 m2) and includes two parts. The upper part, made of reinforced concrete, is a percolation tank, and the other part is the double glass–steel pipe installed at the bottom of the tank for water draining (Figure 2). The inner diameter of the pipe is 600 mm, and the length is 6 m. The height of the pipes inserted in the medium sand of the stratum is 0.5 m, but it is more than 1 m beyond the highest groundwater table. The effective thickness of the filler layer is 2.4 m. The main filler of the CRIS is medium-coarse sand with 5% (mass ratio) zeolite, and the particle size of the sand varies from 0.10 mm to 1.0 mm. The size of the zeolite is 1–3 mm. The percolation tank area of the CRIS is 4.91 m2, and the confluence area is 1,100 m2 (950 m2 concrete impervious surface and 150 m2 lawn). The confluence ratio is 220: 1 (confluence ratio = the confluence area/tank area). A horizontal water sample collection pipe is set at the lower one-third of the filler layer, and a Φ350 PVC pipe is inserted vertically in the middle of the filler to collect the outflow (Figures 2A–C). The diagram of the CRIS and groundwater monitoring well is shown in Figure 3.
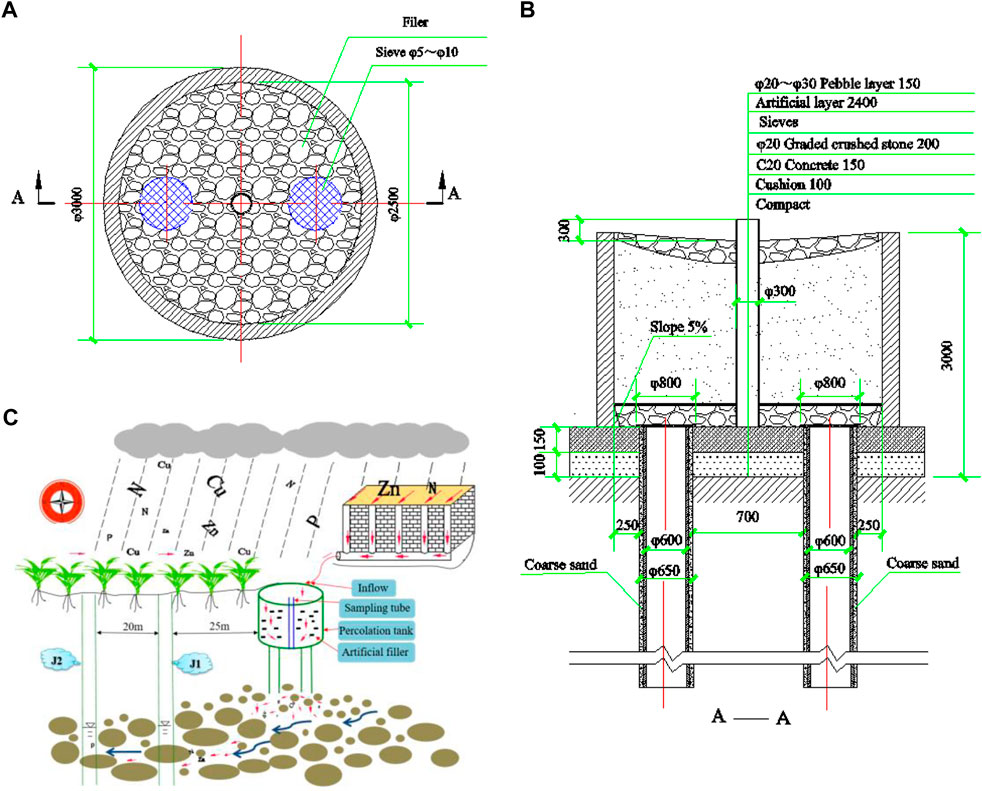
FIGURE 2. CRIs structure and flowchart. Note: (A) the bottom structure of the percolation tank; (B) CRIs structure; (C) flowchart.
Monitoring and analysis methodology
The groundwater flow in the study area is from east to west. A monitoring well (J1) is drilled 25 m away from the CRIS, and a background well (J2) is drilled 45 m away from the CRIS (Figures 2C, 3) to study the effluence of stormwater concentration infiltration on the groundwater table and quality in the CRIS. The groundwater table online monitor is installed in J1, and the data are recorded every 5 min. The water samples in J1 are collected manually to test the concentration of chemical oxygen demand (COD), N, P, and the heavy metals. The groundwater depth and samples in J2 are measured manually, and they are collected 2–3 times a month. A total of 56 groundwater table values and water samples are collected in J2 from October 2017 to December 2019. The rainfall infiltration recharge is calculated by using Eq. 1.
where V-Recharge volume, m3.
α-Precipitation infiltration recharge coefficient, the rainfall volume varies from 348.0–726.2 mm, and α = 0.15–0.24 (Shu, et al., 2008). The rainfall volume remains between 503.6 and 788.4 mm during the monitoring period, and α is 0.15 in this article.
P-Rainfall volume, mm.
F-The confluence area, km2.
Pollutant reduction can be evaluated in terms of the concentration removal rate (Rc), which can be expressed as follows:
The rainfall volume within 24 h is used as the criterion to distinguish rainfall types. A volume of more than 50 mm is defined as rainstorm, whereas a volume between 25 and 50 mm is defined as heavy rain. A volume between 10 and 25 mm is defined as moderate rain, whereas a volume less than 10 mm is defined as light rain. Rainfall parameters for the storm events are shown in Table 1. The “Standard for Groundwater Quality” (GB/T14848-2017) in China is shown in Table 2.
Sample collection analysis
The concentrations of COD, N, and P in water were tested and analyzed using standard analysis methods. The COD was measured by potassium dichromate rapid digestion spectrophotometry (DR5000). The H4-N and NO3-N in water were measured by continuous flowing analysis (SKALAR, Holland), and the TN and TP were measured by using an ultraviolet spectrophotometer (DR5000). The SRP samples were first filtered through a 0.22 μm membrane filter and analyzed by using methods identical to TP analysis, except without the digestion process for SRP. TSS was processed gravimetrically by using the Standard Method 2540 D.
Results
Rainfall runoff reduction rate
A total of 86 rainfall events are monitored from October 2017 to December 2019. The characteristics of rainfall are shown in Table 1, and the infiltration volume and the runoff reduction rate are shown in Figure 4. Most of the rainfall events occurred from April to September every year. Three rainstorm events and 19 heavy rains are observed, and the remaining are all moderate or light rain. The rainfall volume of the rainstorm in 2017 is 54.5 mm, and the maximum rainfall intensity within 60 min reaches 45.8 mm. No rainstorm occurs in 2018. Two rainstorm events occur in 2019, the rainfall volume varies from 59.8 mm to 66.4 mm, and the maximum rain intensity within 60 min ranges from 35.9 mm to 45.7 mm. No overflow occurs during the monitoring period, and the runoff reduction rate and the flood peak reduction rate of CRIS all reach 100% (Figure 4). This condition indicates that the rainfall runoff reduction effect is good although the CRIS has a large confluence area. The main reason is that the filler in CRIS is medium-coarse sand, the permeability coefficient is about 2.38*10–3 m/s, and it has a good water-conducting effect on rainfall runoff. Relevant studies have shown that the reduction of rainfall runoff mainly depends on the moisture content of the filler (Crespo et al., 2011). The moisture content of the CRIS is consistently small, and the permeability is good. Therefore, the CRIS has better reduction effect on rainfall runoff.
Pollutants’ purification effect of the CRIS
A total of 21 rainfall events are monitored from October 2017 to September 2019. The inflow and outflow water samples are collected to study the pollutants’ purification effect by the CRIS. The COD, TSS, N, and P removal rates are calculated by using Eq. 2, and the effect of runoff pollutant removal rate is shown in Table 3. The removal rate of pollutants gradually increased with the monitoring time. The purification effect is poor for the first three rainfall events from October to November 2017, and the removal rates are all negative except for COD, NH4-N, and TSS. This finding indicates that the pollutants in the CRIS fillers are leached with the infiltration of water. However, the mean removal rates of all the pollutants are positive in 2018, the purification effect is extremely good for NH4-N and TSS, and the removal rates reach 80.45% and 76.85%, respectively. The removal rates of TN and TP are 70.49% and 64.75%, respectively. This finding is mainly because the CRIS is completed in August 2017 in an unstable operation stage. The CRIS mainly receives roof rainfall runoff with good water quality, and the dissolved pollutants in the CRIS are leached because of erosion by rainwater infiltration, so the removal rate of pollutants is mostly negative. However, the CRIS gradually reaches a stable operation stage for the purification of rainfall runoff pollutants. The better removal purification of NH4+-N mainly depends on the zeolite. The natural zeolite is rich in Al3+, and Al3+ was hydrolyzed to form an Al(OH)3 colloid, which plays an important role in the adsorption of NH4+ in a water body. Studies show that zeolite has a good adsorption effect on NH4-N (Zheng et al., 2008). The removal rates of NH4-N and TSS reach 85.64% and 80.16% in 2019, respectively, and they are 72.48% and 70.40% for the TN and TP. The purification effect of NO3−-N, and SRP are poor during the monitoring period, and the removal rates remain at −40.15%–26.79% and −35.42%–32.48%.
Influence of stormwater concentration infiltration on groundwater table
The groundwater table depth is affected by rainfall infiltration recharge, and the groundwater in this study area is mainly recharged by rainfall runoff through the CRIS. The whole process of rainfall entering the soil and then reaching the groundwater is called rainfall infiltration recharge (Grismer et al., 2015). However, rainfall infiltration is different from the recharge, and part of the water is intercepted by soil or vegetation roots when the rainfall reaches the ground. Thus, the amount of available water reaching the groundwater is reduced.
Figure 5 shows the relationship among the daily average groundwater table depth, rainfall volume, and rainfall infiltration recharge in J1. The groundwater depth in the study area is greatly affected by the concentration infiltration recharge of the CRIS. The groundwater depth exhibits a minor change in the rainy season (March–September) and is in the period of pollutant purification stability. It fluctuates greatly in the nonrainy season (October–February of the following year). The change process of groundwater depth is inconsistent with the rainfall recharge. It indicates that the groundwater recharge by rainfall infiltration in the rainy season has obvious hysteresis. The response of J1, 25 m far away from CRIS, to the concentration infiltration recharge is delayed, and the lag time is about 3–4 months. The stormwater concentration infiltration in the CRIS causes the groundwater table to rise year by year, and the water depth becomes remarkably shallow. The variation range of the depth is increased within 0.5–1.5 m. The groundwater depth of J2 is deeper than that of J1, the variation of J2 is small during the monitoring period, and the range remains between 0 and 0.47 m. Hocking and Kelly (2016) studied the groundwater recharge and time lag measurement through Vertosols using impulse response functions. They found that the long-term groundwater level trend rose 1 m over the simulation period. Conclusions from the relevant research show that the influence scope on the groundwater table is greater than 25 m if the CRIS is taken as the concentrated infiltration point, but the impact is small when the distance exceeds 45 m.
Influence of stormwater concentration infiltration on groundwater quality
A total of 64 and 65 groundwater samples in J1 and J2 are collected from July 2017 to December 2019, respectively. As shown in Table 4, the pollutants’ concentration in J1 and J2 is less than those of the inflow during the monitoring period. However, the pollutant concentration is larger in J1 than those in J2. The pollutants’ concentration in J1 reaches the maximum value in the early operation stage of the CRIS from July to December 2017. It increases slightly at the stable operation stage from 2018 to 2019, and the mean values of COD, TP, TN, NH4+-N, NO3−-N, Cu, Zn, and Cd in 2019 increased by 5.32%, 8.00%, 8.25%, 3.13%, 9.49%, 17.39%, 19.67%, and 15.38% respectively. The increased proportion of COD, N, and P is less than 10% and is less than 20% for heavy metals (the background value is small). The NH4+-N, NO3−-N, Cu, and Zn concentrations at the stable operation stage reach the class II or III standard values specified in the “Standard for Groundwater Quality” (GB/T 14848-2017, Table 2). The COD, N, P, and heavy metals exhibit a minor change in J2 over the monitoring time. Thus, the influence scope of the concentrated infiltration on groundwater quality is between 25 and 45 m.
Discussion
The CRIS is widely used in rapid rainwater treatment facilities. The confluence area of the CRIS is large. Relevant studies have shown that the confluence ratio of CRIS can reach 50: 1–250: 1 (Zhao et al., 2019). This condition is because the CRIS can absorb rainfall runoff 50–250 times of its own area, and most of the runoff volume infiltrates to recharge the groundwater. Thus, the CRIS needs to treat roof runoff with good water quality and cannot be used to treat road runoff for preventing groundwater pollution (Ma et al., 2018).
The purification effect of pollutants by the CRIS mainly depends on the filler. The CRIS is filled with medium or coarse sand, and the pollutant adsorption materials such as zeolite, granite, and muscovite are added to improve the purification effect. Hydraulic retention time (HRT) is an important factor affecting the pollutants’ purification effect in the CRIS(Aris et al., 2016). However, the duration of HRT should not be extremely long, otherwise, it will affect the water infiltration rate of the CRIS. Zhao et al. (2019) showed that the water infiltration rate of the CRIS should be larger than 2.6*10–4 m/s. The water infiltration rate is 2.38*10–3 m/s in this study, which can meet the requirements of rapid water infiltration. However, the purification effect on pollutants mainly depends on the filtration, adsorption, and ion exchange of the fillers in the CRIS. If the water infiltration rate of the CRIS is large, then the purification effect on pollutants is poor (Zhao et al., 2011). Compared with bioretention facilities, the purification effect of the CRIS on pollutants is relatively poor mainly because the organic content of the fillers in bioretention facilities is large, which provides favorable living conditions for microorganisms. Microorganisms play an important role in degrading pollutants in bioretention facilities after rainfall (Li and Davis 2008). The fillers of the CRIS are mainly medium-coarse sand with low organic matter content, which is unsuitable for the survival of microorganisms, so the degradation possibility of pollutants by microorganisms in the CRIS is small (Zuo et al., 2019). The removal rate of NO3−-N and SRP is small in this study. The CRIS mainly receives roof runoff with good water quality and has a high water infiltration rate. Under the scouring effect of rainfall runoff, the soluble nitrate and phosphate are prone to leaching (Li and Davis, 2015). This condition is due to the negative charge of NO3−-N and the filler. NO3−-N is extremely active in the filler, so it is easy to migrate downward with water infiltration (Blecken et al., 2010). The CRIS is filled with mixed fillers of medium-coarse sand and zeolite, and they have a low content of iron and aluminum salts, so they are less effective for P removal (Liu and Davis, 2014). Kamali et al. (2017) found that the dissolved phosphate in the groundwater has a higher concentration than the stormwater influent, indicating that it is produced or added in the infiltration bed. Contaminants, such as nitrates and pathogens, are incompletely removed before the runoff enters the underground watercourse (Gallagher, 2017).
The groundwater recharge by rainfall infiltration is affected by the groundwater table depth. Relevant studies have shown that when the groundwater depth exceeds 25 m, the effect of groundwater recharge by rainfall infiltration is weak (Xia et al., 2016). This condition is because when the rainfall has potential energy in the soil, it can flow and penetrate, and the infiltration depth is limited, rather than endless. Specifically, the driving potential energy will be exhausted when the infiltration reaches a certain depth, and the water will not penetrate downward due to the resistance of the soil. The groundwater depth in the study area is about 12 m. The stormwater concentration infiltration by the CRIS can recharge the groundwater and increase the water depth. The increased range of groundwater depth varies from 0.5 m to 1.5 m. The researchers found that groundwater at a depth of 1 m below the water table consists mostly of stormwater, and that stormwater does not penetrate to depths greater than 3 m below the water table (Watson et al., 2018). Boisson et al. (2014) found that the groundwater table in the irrigation area increases by an average of 2 m every year when surface water was used for irrigation. Some scholars asserted that the groundwater recharge by irrigation water is negatively correlated with the groundwater depth (Massuel et al., 2014).
The response of groundwater table to rainfall runoff recharge has a hysteresis due to the impact of the groundwater flow field (Hocking and Kelly 2016), and the lag time is about 3–4 months. Liu (2021) showed that with the increase in the groundwater depth, the lag duration time of infiltration recharge is more obvious. When the groundwater depth reaches 8 m, the lag time of the peak value of irrigation infiltration recharge may reach more than 2–3 months. However, the analysis of hydrograph response times with depth to water table (KCB, 2010) showed no clear correlation. The influence scope of the concentrated infiltration on groundwater table and quality is between 25 and 45 m. The impact of stormwater concentration infiltration on groundwater quality is small. The increased proportion of COD, N, and P in J1 is less than 10% from 2018 to 2019 and less than 20% for heavy metals. The NH4+-N, NO3−-N, Cu, and Zn concentrations at the stable operation stage reach the class II or III standard values specified in the “Standard for Groundwater Quality” (GB/T 14848-2017, Table 2). This conclusion is consistent with Eshtawi et al. (2016). They declared that no evidence points to a high risk of groundwater contamination, but the quality of surface soils decreases because of the long-term infiltration of polluted stormwater runoff.
Conclusion
The CRIS is an important facility for groundwater recharge and has good applicability for the treatment of roof rainfall runoff. In the early operation stage of the CRIS, the pollutants’ concentration in groundwater is relatively large. The pollutants’ concentration in groundwater increases slightly over the monitoring time after the CRIS enters a stable operation stage, but the increased proportion of COD, N, and P is less than 10% and less than 20% for heavy metals. The stormwater concentration infiltration of the CRIS has minimal impact on groundwater quality. However, it can recharge groundwater and increase the groundwater table depth. The groundwater table has an obvious response to the rainfall runoff infiltration recharge. The increase in groundwater depth ranges from 0.5 m to 1.5 m. However, the response of the groundwater table to rainfall runoff recharge in the monitoring well, 25 m far away from the CRIS, has obvious hysteresis and the lag time is about 3–4 months. The influence scope of the stormwater concentration infiltration on the groundwater table and quality in the CRIS is larger than 25 m, whereas its impact is small when the distance exceeds 45 m.
Data availability statement
The original contributions presented in the study are included in the article/Supplementary Material, further inquiries can be directed to the corresponding author.
Author contributions
CG and JL conceived the experiments and analyzed the results. YS and WG conducted the experiments. ZM and SY reviewed the manuscript. All authors contributed to the article and approved the submitted version.
Funding
This research was financially supported by the Key Research and Development Program of Shaanxi (2022ZDLSF06-04), the Technology Innovation Center for Land Engineering and Human Settlements, Shaanxi Provincial Land Engineering Construction Group Co., Ltd., and Xi’an Jiaotong University (2021WHZ0090), the project of Shaanxi Provence Land Engineering Construction Group (DJNY 2022-26).
Conflict of interest
CG, YS, WG, ZM, and SY are employed by Shaanxi Provincial Land Engineering Construction Group Co., Ltd. The authors declare that this study received funding from Shaanxi Provincial Land Engineering Construction Group Co. The funder had the following involvement in the study: Influence of Constructed Rapid Infiltration System on Groundwater Recharge and Quality.
The remaining authors declare that the research was conducted in the absence of any commercial or financial relationships that could be construed as a potential conflict of interest.
Publisher’s note
All claims expressed in this article are solely those of the authors and do not necessarily represent those of their affiliated organizations, or those of the publisher, the editors and the reviewers. Any product that may be evaluated in this article, or claim that may be made by its manufacturer, is not guaranteed or endorsed by the publisher.
References
Al-Yamani, W., Green, S., Pangilinan, R., Dixon, S., Shahid, S. A., Kemp, P., et al. (2019). Water use of Al Samr (Acacia tortilis) forests irrigated with saline groundwater and treated sewage effluent in the hyper-arid deserts of Abu Dhabi. Agric. Water Manag. 216, 361–364. doi:10.1016/j.agwat.2019.01.024
Aris, M. A. M., Chelliapan, S., Din, M. F. M., Anwar, A. N., Shahperi, R., and Selvam, S. B. (2016). Effect of effluent circulation and hydraulic retention time (HRT) on the performance of a modified anaerobic baffled reactor (MABR) during start-up period. Desalination Water Treat. 57 (40), 18597–18605. doi:10.1080/19443994.2015.1094676
Bach, P. M., Mccarthy, D. T., and Deletic, A. (2010). Redefining the stormwater first flush phenomenon. Water Res. 44, 2487–2498. doi:10.1016/j.watres.2010.01.022
Blecken, G. T., Zinger, Y., Deletic, A., Fletcher, T. D., Hedstrom, A., and Viklander, M. (2010). Laboratory study on stormwater biofiltration nutrient and sediment removal in cold temperatures. J. Hydrology 394 (3-4), 507–514. doi:10.1016/j.jhydrol.2010.10.010
Boisson, A., Baisset, M., Alazard, M., Perrin, J., Villesseche, D., Dewandel, B., et al. (2014). Comparison of surface and groundwater balance approaches in the evaluation of managed aquifer recharge structures: Case of a percolation tank in a crystalline aquifer in India. J. Hydrology 519, 1620–1633. doi:10.1016/j.jhydrol.2014.09.022
Brown, R. A., Line, D. E., and Hunt, W. F. (2012). LID treatment train: Pervious concrete with subsurface storage in series with bioretention and care with seasonal high water tables. J. Environ. Eng. New. York. 138 (6), 689–697. doi:10.1061/(ASCE)EE.1943-7870.0000506
Crespo, P. J., Feyen, P., Buytaert, W., Bücker, A., Breuer, L., Frede, H. G., et al. (2011). Identifying controls of the rainfall–runoff response of small catchments in the tropical Andes (Ecuador). J. Hydrology 407, 164–174. doi:10.1016/j.jhydrol.2011.07.021
Duan, J. M., and Su, B. (2014). Removal characteristics of Cd(II) from acidic aqueous solution by modified steel-making slag. Chem. Eng. J. 246, 160–167. doi:10.1016/j.cej.2014.02.056
Eisapour, A. H., Eisapour, M., Sardari, P. T., and Walker, G. S. (2010). An innovative multi-zone configuration to enhance the charging process of magnesium based metal hydride hydrogen storage tank. J. Energy Storage 36, 102443–102450. doi:10.1016/j.est.2021.102443
Eshtawi, T., Evers, M., Tischbein, B., and Diekkrüger, B. (2016). Integrated hydrologic modeling as a key for sustainable urban water resources planning. Water Res. 101, 411–428. doi:10.1016/j.watres.2016.05.061
Grismer, M. E., Bachman, S., and Powers, T. (2015). A comparison of groundwater recharge estimation methods in a semi-arid, coastal avocado and citrus orchard (Ventura County, California). Hydrol. Process. 14 (14), 2527–2543. doi:10.1002/1099-1085(20001015)14:14<2527:aid-hyp112>3.0.co;2-t
Gallagher, K. C. (2017). Stormwater Infiltration and Groundwater Integrity:An Analysis of BMP Siting Tools and Groundwater Vulnerability. University of South Florida, Florida, 3-16.
Guo, C., Li, J. K., Li, H. E., and Li, Y. J. (2019). Influences of stormwater concentration infiltration on soil nitrogen, phosphorus, TOC and their relations with enzyme activity in rain garden. Chemosphere 233 (207), 207–215. doi:10.1016/j.chemosphere.2019.05.236
Hocking, M., and Kelly, B. F. J. (2016). Groundwater recharge and time lag measurement through Vertosols using impulse response functions. J. Hydrology 535, 22–35. doi:10.1016/j.jhydrol.2016.01.042
Kamali, M., Delkash, M., and Tajrishy, M. (2017). Evaluation of permeable pavement responses to urban surface runoff. J. Environ. Manage. 187, 43–53. doi:10.1016/j.jenvman.2016.11.027
Kang, A. (2011). The treatment of high ammonia nitrogen domestic sewage by three-step series constructed rapid infiltration system. Beijing: China University of Geosciences, 38–56. doi:10.1631/jzus.A1010009
KCB (2010). Central Condamine alluvium, stage II-Conceptual hydrogeology study. Queensland: Prepared by Klohn Crippen Berger for the Department of Environment and Resource Management.
Lee, B. S., Hong, S. W., Kang, H. J., Lee, J. S., and Nam, K. P. (2012). Groundwater recharge and discharge in the urban-rural composite area. J. Soil Groundw. Environ. 17 (2), 37–46. doi:10.7857/JSGE.2012.17.2.037
Li, H., and Davis, & A. P. (2008). Urban particle capture in bioretention media. II: Theory and model development. J. Environ. Eng. New. York. 134134 (6), 4196–4432. doi:10.1061/(asce)0733-9372(2008)134:6(419)
Li, L. Q., and Davis, A. P. (2015). Urban storm-water runoff nitrogen composition and fate in bioretention systems. Environ. Sci. Technol. 48 (6), 3403–3410. doi:10.1021/es4055302
Liu, J. Y., and Davis, A. P. (2014). Phosphorus speciation and treatment using enhanced phosphorus removal bioretention. Environ. Sci. Technol. 48 (1), 607–614. doi:10.1021/es404022b
Liu, X., Wang, X., Bai, M., and Shaw, J. J. (2021). Decrease in carabid beetles in grasslands of northwestern China: Further evidence of insect biodiversity loss. Insects 13, 35–43. in Chinese. doi:10.3390/insects13010035
Ma, Y., Hao, S., Zhao, H., Fang, J., Zhao, J., and Li, X. (2018). Pollutant transport analysis and source apportionment of the entire non-point source pollution process in separate sewer systems. Chemosphere 211, 557–565. doi:10.1016/j.chemosphere.2018.07.184
Massuel, S., Perrin, J., Mascre, C., Mohamed, W., Boisson, A., and Ahmed, S. (2014). Managed aquifer recharge in South India: What to expect from small percolation tanks in hard rock. J. Hydrology 512, 157–167. doi:10.1016/j.jhydrol.2014.02.062
Mensah, J. K., Ofosu, E. A., Yidana, S. M., Akpoti, K., and Kabo-bah, A. T. (2022). Integrated modeling of hydrological processes and groundwater recharge based on land use land cover, and climate changes: A systematic review. Environ. Adv. 8, 100224–110240. doi:10.1016/j.envadv.2022.100224
Nguyen, T. T., Ngo, H. H., Guo, W. S., Wang, X. C., Ren, N. Q., Li, G. B., et al. (2019). Implementation of a specific urban water management-Sponge City. Sci. Total Environ. 652, 147–162. doi:10.1016/j.scitotenv.2018.10.168
Shao, W. W., Zhang, H. X., Liu, J. H., Yang, G. Y., Chen, X. D., Yang, Z. Y., et al. (2016). Data integration and its application in the sponge city construction of China. Procedia Eng. 154, 779–786. doi:10.1016/j.proeng.2016.07.583
Shu, L. C., Tao, Y. F., and Liu, P. G. (2008). Reliability calculation method for groundwater recharge in consideration of uncertainty of hydrogeological parameters. SHUILI XUEBAO 39 (3), 346–350. in Chinese. doi:10.3321/j.issn:0559-9350.2008.03.014
Su, C. Y., Zhu, X. W., Shi, X. W., Xie, Y., Fang, Y. T., Zhou, X. B., et al. (2020). Removal efficiency and pathways of phosphorus from wastewater in a modified constructed rapid infiltration system. J. Clean. Prod. 267, 122063–122588. doi:10.1016/j.jclepro.2020.122063
Trowsdale, S. A., and Simcock, R. (2011). Urban stormwater treatment using bioretention. J. Hydrol. X. 397 (3-4), 167–174. doi:10.1016/j.jhydrol.2010.11.023
Vystavna, Y., Schmidt, S. I., Diadin, D., Rossi, P. M., Vadillo, I., Erostate, M., et al. (2019). Multi-tracing of recharge seasonality and contamination in groundwater: A tool for urban water resource management. Water Res. 161, 413–422. doi:10.1016/j.watres.2019.06.028
Watson, A., Miller, J., Fleischer, M., and Clercq, D. W. (2018). Estimation of groundwater recharge via percolation outputs from a rainfall/runoff model for the Verlorenvlei estuarine system, west coast, South Africa. J. Hydrology 558, 238–254. doi:10.1016/j.jhydrol.2018.01.028
Xia, J., Zhao, X., Chen, Y., Ying, F., and Zhao, Z. (2016). Responses of water and salt parameters to groundwater levels for soil columns planted with tamarix chinensis. Plos One 11 (1), 0145828. doi:10.1371/journal.pone.0145828
Yang, L., Kong, F. L., Xi, M., Li, X., and Wang, S. (2017). Environmental economic value calculation and sustainability assessment for constructed rapid infiltration system based on emergy analysis. J. Clean. Prod. 167, 582–588. doi:10.1016/j.jclepro.2017.08.172
Zhai, Y., Zhao, X., Teng, Y., Li, X., Zhang, J. J., Wu, J., et al. (2017). Groundwater nitrate pollution and human health risk assessment by using HHRA model in an agricultural area, NE China. Ecotoxicol. Environ. Saf. 137, 130–142. doi:10.1016/j.ecoenv.2016.11.010
Zhao, F., Zhang, Y., Huang, M. S., Wu, X. H., Zhang, Y. F., and He, Y. (2011). Study on the purification effects of aquatic plant floating-beds for urban polluted water. J. East China Normal Univ. Nat. Sci. 6, 63–70. in Chinese. doi:10.1007/s11783-010-0264-4
Zhao, R. S., Li, J. K., Guo, C., Li, F., and Li, H. E. (2019). Filler improvement and purification effects of constructed rapid infiltration facility. Environ. Sci. Pollut. Res. 26 (32), 33654–33669. doi:10.1007/s11356-019-06462-7
Zheng, H., Han, L. J., Ma, H. W., Zheng, Y., Zhang, H. M., Liu, D. H., et al. (2008). Adsorption characteristics of ammonium ion by zeolite 13X. J. Hazard. Mater. 158 (2-3), 577–584. doi:10.1016/j.jhazmat.2008.01.115
Zhou, X. L., Huang, K. Y., and Wang, J. H. (2017). Numerical simulation of groundwater flow and land deformation due to groundwater pumping in cross-anisotropic layered aquifer system. J. Hydro-environment Res. 14, 19–33. doi:10.1016/j.jher.2016.08.001
Keywords: concentration infiltration, groundwater table, groundwater quality, recharge, constructed rapid infiltration system
Citation: Guo C, Li J, Sun Y, Gao W, Mao Z and Ye S (2022) Influence of constructed rapid infiltration system on groundwater recharge and quality. Front. Environ. Sci. 10:1022970. doi: 10.3389/fenvs.2022.1022970
Received: 24 August 2022; Accepted: 25 November 2022;
Published: 12 December 2022.
Edited by:
Xiaohong Chen, Sun Yat-sen University, ChinaReviewed by:
An Liu, Shenzhen University, ChinaChidozie Charles Nnaji, University of Nigeria, Nsukka, Nigeria
Copyright © 2022 Guo, Li, Sun, Gao, Mao and Ye. This is an open-access article distributed under the terms of the Creative Commons Attribution License (CC BY). The use, distribution or reproduction in other forums is permitted, provided the original author(s) and the copyright owner(s) are credited and that the original publication in this journal is cited, in accordance with accepted academic practice. No use, distribution or reproduction is permitted which does not comply with these terms.
*Correspondence: Jiake Li, eGF1dF9samtAMTYzLmNvbQ==