- 1Department of Biological Sciences, Microbiology Unit, Faculty of Science, Olusegun Agagu University of Science and Technology, Okitipupa, Nigeria
- 2Food Security and Safety Focus Area, Faculty of Natural and Agricultural Sciences, North-West University, Mahikeng, South Africa
- 3Department of Clinical Biology, School of Medicine and Pharmacy, College of Medicine and Health Sciences, University of Rwanda, Kigali, Rwanda
- 4Department of Biology, University of Waterloo, Waterloo, ON, Canada
Plant responses to abiotic stresses through diverse mechanisms and strategic measures in utilizing nanomaterials have positively impacted crop productivity. Stress can cause membrane depletion, reactive oxygen species formation, cell toxicity and death, and reduction in plant growth. However, nanomaterials can mitigate some of the negative impacts of abiotic stresses and enhance crop yield. Some endophytic microbes can synthesize nanomaterials, which can maintain and enhance plant health and growth via nitrogen fixation, siderophore production, phytohormones synthesis, and enzyme production without any pathological effects. Nanoparticle-synthesizing endophytes also help boost plant biochemical and physiological functions by ameliorating the impact of abiotic stresses. The increase in the use and implementation of nano-growth enhancers from beneficial microbes, such as nano-biofertilizers, nano-pesticides, nano-herbicides, and nano-fungicides are considered safe and eco-friendly in ensuring sustainable agriculture and reduction of agrochemical usage. Promisingly, nanotechnology concepts in agriculture aim to sustain plant health and protect plants from oxidative stresses through the activation of anti-oxidative enzymes. The mechanisms and the use of nanomaterials to relieve abiotic plant stress still require further discussion in the literature. Therefore, this review is focused on endophytic microbes, the induction of abiotic stress tolerance in plants, and the use of nanomaterials to relieve abiotic plant stresses.
Introduction
The environmental problems linked to climate abiotic-induced stresses pose serious threats and ecological pressures on soils and plant health, limiting crop productivity (Varshney et al., 2011). Thus, the need to devise a problem-solving approach to enhance crop yield under stress becomes imperative. The biotic factors, such as bacterial and fungal pathogens, insect and nematode pests as well as abiotic factors such as temperature, salinity, drought, flooding, heavy metals, and pH cause a large number of modifications in plant biochemical and physiological processes (Kumar et al., 2019). The approaches to mitigate these stresses in crops should be targeted to maximally address the food supply and demand of the world population. Over time, the use of chemical fertilizers to improve crop productivity has been employed, but with profound detrimental effects on the ecosystems (Adeleke and Babalola, 2022). Hence, developing modern technology remains important to ensure Sustainable Development Goals (SDGs) without any significant negative impact on the ecosystem.
Nanotechnological approaches have been employed in agriculture, industry, and medicine (Audah, 2019; Elemike et al., 2019; Zulfiqar et al., 2019). Nanoparticles (NPs), are characterized by sizes ranging from 1 to 100 nm in diameter, various physical, chemical features, biochemical activity, and increased reactivity (Dutta and Sugumaran, 2021). Different methods have been employed in the synthesis of NPs, which include inert gas condensation, physical ball milling, biological and chemical processes (Aboyewa et al., 2021). The biological means of synthesizing NPs can be achieved by harnessing some endophytic microbes, such as bacteria and fungi (Ahmad F. et al., 2012; Eid et al., 2021). Some examples of NPs produced by endophytic microbes include titanium, platinum, cadmium, gold, zirconium, selenium, magnetite, usnic acid, gold-silver alloy, uraninite, tellurium, and palladium (Aboyewa et al., 2021). NPs from endophytic fungi have been reported to play an important role in plant disease management due to the presence of NP-assisted genes (Sonawane et al., 2022).
Endophytic microbes are microbes inhabiting the internal tissues of plants, which can be beneficial or pathogenic (Adeleke and Babalola, 2022). The beneficial types help ensure sustainable plant and soil health under a variety of stresses including drought stress (Premachandra et al., 2020). Some of these microbes also possess the ability to synthesize nanomaterials, which can be exploited in maintaining plant health without any pathological effects (Sonawane et al., 2022). Promisingly, NP-synthesizing endophytic microbes can help boost plant physiological functions and can be used as bioinoculants in developing eco-friendly agriculture. Nevertheless, information on the actual mechanisms and the use of nanomaterials to relieve abiotic plant stresses have not been fully discussed in the literature. Depending on the type, application and use, various NPs of carbon-based, metallic and non-metallic and organic polymers have been developed (Kumar et al., 2021; Fadiji et al., 2022b). The use and implementation of known nano-growth enhancers, nano-biofertilizers, nano-pesticides, nano-herbicides, and nano-fungicides are on the increase in modern agricultural systems (Imade et al., 2022; Sonawane et al., 2022). Based on experience to date, this approach is considered safe, eco-friendly for improved soil nutrition and crop yield.
Plants are prone to different environmental stressors, such as ultraviolent light, drought, flooding, salinity, temperature extremes (low or high), and the presence of heavy metals (Chaudhary et al., 2021c). All of these factors can induce oxidative stress causing membrane depletion, reactive oxygen species formation, cell toxicity and death, which cause a reduction in plant growth (Thomas and Puthur, 2017; Hasanuzzaman et al., 2019). Regardless of the nature of the abiotic stress, NPs may be involved in plant cellular metabolism, growth, and stress protection (Ajilogba et al., 2021). Also, some NPs exhibit the ability to modify the expression of genes involved in electron transport, energy transport, cell biosynthesis, and cell organization under stress conditions (Pandey, 2018; Sonawane et al., 2022). Thus, many studies have validated the multifunctional attributes of NPs in crop improvement (Abd-Alla et al., 2019; Chavan and Nadanathangam, 2019; Kibbey and Strevett, 2019).
Notwithstanding the positive attributes of NPs, information on the mechanisms of how NPs alleviate stresses and how endophytic microbes induce plant stress tolerance are still required. Consequently, this review addresses the role of endophytic microbes in inducing abiotic stress tolerance in plants, and the use of nanomaterials to relive abiotic plant stresses.
Microbe-nanomaterial interactions
Biological activities through alterations in the function and structure of bacteria can be unveiled using modern and advanced nano-technological processes (Chaudhary et al., 2021b). Recent methods are been used to assess the surface chemistry, structural form of NPs and their effects on biocidal activities (Noukelag et al., 2022; Rehman et al., 2022). Examples of ecofriendly nano-sized agents include a variety of phyto/zooplankton, fungal spores, bacteria, and other microorganisms. NPs react differently with microbes, which shows that the microbial cell surfaces can differ substantially in their reactivity and attraction (Gangadoo et al., 2022). Silica NPs react effectively with microorganisms of different groups (Wang et al., 2020). For instance, bacteria and microalgae are smaller with less reactive attributes compared to fungal spores. A comprehensive mechanism showing the harmful effect of metallic nano-sized particles on bacteria cells is still required. In addition, there is a need for special attention to the structural alteration of bacterial cells using in vitro studies.
The continuous upsurge in the cases of fungal infections in immunocompromised patients, which require urgent medical treatment has caught the attention of most researchers. Meanwhile, the need for an ecofriendly measure for treating mycoses and identification of the source of infection has prompted researchers toward the use of metallic NPs (Singh et al., 2019; Soliman et al., 2021). Several studies have assessed the antifungal effects of NPs (Khatoon et al., 2018; Ahmadpour et al., 2021; Sadek et al., 2022). A study by Masoumizadeh et al. (2022) reported the effect of AgNPs on fungal pathogens, Candida spp. Also, the findings of Santhoshkumar et al. (2019) on the toxicological ad antidermatophytic activity of AgNPs synthesized using leaf extract of Passiflora caerulea revealed the maximum antifungal activities against dermatophyte, Trichophyton rubrum.
Nanomaterial-microbial compatibility
Bacteria and NPs
Plasmolysis of bacteria is a slaying event that involves the breakdown of cytoplasmic components and morphological reduction of cytoplasm due to the loss of intracellular components and plasma membrane contraction from the cell wall. It has been reported that metallic NPs induce pleiotrophic effect on bacteria cells. Nanomaterials bind with bacteria proteins (thiol moieties) hindering their activities, forming an attachment with the cell membrane and causing cell death. Consequently, altering cell permeability by obstructing the activities of electrons in cells and obstructing respiration (Radzig et al., 2013; Mohanty et al., 2014). ROS generated as a result also inhibits respiratory enzymes. Oxidized DNA precursors result in DNA lesions (Park et al., 2009).
Advances in the cell to the non-cell formation, the reaction against resistant, persistent strains and swarming motility have encouraged researchers about bacterial genes encoding guanine nucleotide exchange factors. NPs enhance the activities and response of genes encoding guanine nucleotide exchange factors, which gives scientists better insights into improved NPs applications as antibacterial agents. Because of these highlighted responses, several studies have been conducted to verify the expression of bacterial genes to nano-sized particles (Khati et al., 2018). Exposure to metallic NPs revealed consistent gene patterns using transcriptional analytical methods such as RT qPCR or microarray. For instance, when E. coli was subjected to AgNPs, it exhibited a distinctive expression in gene functions, such as homeostasis of iron, silver and copper, which regulate the oxidative balance via the use of microarray experiment and its features to metabolize sulfur (Nagy et al., 2011; McQuillan and Shaw, 2014). In other studies, researchers assessed alterations in gene expression of bacteria subjected to the treatment with carbon NPs. A study by Kang et al. (2008) showed the leakage of cellular material, disrupted membrane, reduced viability and metabolism of E. coli when subjected to single-walled nanotubes. In another study by Pelletier et al. (2010) using microarray, E. coli was exposed to cerium oxide NPs, the NPs upregulated several oxidoreductases sowing depletion in iron deficiency, oxidation stress and cellular respiration. Yang et al. (2012b) exposed Pseudomonas aeruginosa to quantum dots and genes controlling metal efflux transporters and oxidative stress were upregulated.
To check the compatibility of nanomaterials and bacterial cells, Dimkpa et al. (2012) showed that NPs could also affect microorganisms and plants by causing modifications in cellular levels of siderophores (pyoverdine) of plant growth-promoting bacterium (Dimkpa et al., 2012).
Fungi and NPs
Almost all NPs are capable of creating holes in the membrane of most fungal cells. The alteration in physiological traits of fungi releases biomolecules resulting in cell death. In a study by Kim et al. (2009), Kim and co. observed the reaction between AgNPS and Candida albicans and discovered membrane depolarization in C. albicans. Pits and pores were formed on the cell wall of the organism. This leads to the release of trehalose and glucose into the prepared suspension. The antifungal activity of AgNPs was also performed on other fungal species such as Saccharomyces cerevisiae, Candida tropicalis, Phomopsis spp., Penicillium expansum, Botrytis cinerea and Trichophyton rubrum (He et al., 2011; Nasrollahi et al., 2011; Mallmann et al., 2015). The effect of Fe3O4NPs was tested against Candida spp and perforation of cell membranes was observed (Prucek et al., 2011). The cell wall and the membrane of Cryptoccocus neoformans were also depleted when C. neoformans was exposed to AgNPs in a study by Ishida et al. (2013).
The use of In silico and mathematical modelling could help provide a better understanding of microbe-nanomaterial compatibility and interactions. Studies to experiment with the interaction between microbes and NPs are extremely important to unveil the details of these interactions. Meanwhile, bioinformatics tools are also needed to ensure statistical analysis and data curation about the future occurrences of microbial interaction with NPs (Singh et al., 2019; Adeleke et al., 2022).
Synergistic relationship: Endophyte-induced NPs
Recent developments required to ensure an ecofriendly interface for nanoscience studies have delivered exciting results by revealing multifaceted metal-based NPs with numerous applications and functions (Baker et al., 2015a; Kumari et al., 2020). Ecofriendly biological resources viz. algae, fungi, bacteria and plants have been adopted to synthesize NPs, with each bio-factory having its pros and cons (Iravani et al., 2014; Rahman et al., 2019). Microorganisms are said to be an attractive option because of their dependable and unlimited metabolite production which are useful as reducing agents. In the case of plants, the disturbing plant diversity/species most times complicate the usage (because of selection problem) (Baker et al., 2015b). Even though microorganisms have been identified as the best option for the ecofriendly synthesis of NPs, the potential of endophytes remains under-explored. Adopting endophytes as reducing agents for the biosynthesis of nanomaterials opens new opportunities for the discovery of novel NPs with various applications (Rahman et al., 2019). Microorganisms (e.g., endophytes) remain the biological agents with harmless, clean and the most commercially available approach for NPs synthesis. That said, limitations faced by often synthesized endophyte NPs affect the stability of NPs because microbes are retarded overtime. With the variation in parameters such as substrate condition, synthesis condition, growth on media, pH, temperature and physicochemical parameters (stability, shape, and size) of NPs, which might change easily (Ovais et al., 2018).
The diverse endophytic microbes from different sources have been used in the synthesis of NPs. These include; the biosynthesis of AgNPs using Bacillus cereus isolated from Adhatoda beddomei and Garcinia xanthocymus as recapping agents to produce AgNPs with antibacterial properties (Sunkar and Nachiyar, 2012a; b). A study by Devi and Joshi (2015) also reported the use of Cryptosporiopsis ericae isolated from Poteotilla fulgens L. in the synthesis of NPs. The synthesized nanomaterial had an absorbance peek of ≈430 nm, spherical, and a diameter ranging between 2 nm and 16 nm. Rahi and Parmar (2014) and Singh et al. (2013) also synthesized AgNPs using Penicillium spp. isolated from the tissue of Curcuma longa and Aloe vera root. The synthesized NPs had a size range between 15 nm and 45 nm with immense antibacterial activity against antibiotic-resistant pathogens. Several studies have used endophytes as an ecofriendly route for the biosynthesis of multifunctional metal-based NPs. These include; Hulikere and Joshi (2019)—Cladosporium cladosporoides, Ramalingmam et al. (2015)—Cochliobolus lunatus, Qian et al. (2013)—Epicoccum nigrum, Yashavantha Rao et al. (2016)—Endophytic bacterium EH419, Neethu et al. (2018)—Penicillium polonicum and Abdel-Aziz et al. (2018)—Aspergillus spp.
Impact of nanomaterials on microbial diversity and soil health
In natural ecosystems, microorganisms drive ecological processes (Chaudhary et al., 2022). These processes include; anaerobic digestion, removal of nutrients in wastewater treatment, and biogeochemical cycling (Ahmed et al., 2012). On a single cell or population of microbes, antimicrobial activities and the potency of nanomaterials have been studied extensively elucidating their effects on the microbial community. As a result, there is an extensive understanding of the pros and cons associated with the ecotoxicity of NPs. Recently, scientists have studied the effect of nanomaterials on the community structure and functions of microorganisms in natural environments, such as water treatment facilities, marine, rivers and soils (Mohanty et al., 2014).
Effect of nanomaterials on microbial diversity
Microbial diversity in the soil plays a crucial role in nutrient cycling, plant diversity and agricultural output (Mohanty et al., 2014). Scientists have shown that important nanomaterials properties viz., aggregation, size, shape and charge, could be influenced by the environment (Lowry et al., 2012; Liu et al., 2014). NPs migrate at different levels in the soil matrix, and as such altering the microbial community structure in the soil. Also, the type of soil affects the impact of NPs. In a study by Frenk et al. (2013), the microbial community in clay and sandy soils were shown to respond differently to magnetite and copper oxide NPs. In a related study by Pawlett et al. (2013), a similar result was obtained from the reaction of microorganisms in the sandy soil to zero-valent iron nanomaterials. Herein, the microbial groups obtained from the combination of sandy soil and FeNPs were more susceptible than microbial communities in clay soil. Also, AgNPs were suspected to affect the community profile of freshwater microbial habitat in a study by Das et al. (2012). Both microbial biofilms and planktonic communities were influenced by nanomaterials as related in the study by Flemming and Wingender (2010) and Ding et al. (2014). Although, planktonic communities most times exhibit low tolerance to antimicrobial agents and toxic environments compared to their biofilm counterparts (Cao et al., 2012). For instance, the exposure of marine biofilm to AgNPs does not affect the community structure, succession and biofilm development of the community (Fabrega et al., 2011). However, apart from the reduction in microbial communities associated with a biofilm, the integrity of cells in a biofilm could be compromised because of its exposure to NPs (Battin et al., 2009).
In an engineered ecosystem using nanomaterials, most researchers adopt microbial communities associated with waste plants as model systems. Often, the impact of microbial communities and their community composition is of utmost interest to researchers. Meanwhile, most studies concentrate on the impact of NPs on commonly studied bioprocesses with the inclusion of methanogenesis, phosphorus and nitrogen removal. Even though most studies have reported the negative of nanomaterials on microbial community structure (Liang et al., 2010; Ahmed and Rodrigues, 2013), some other studies showed that the microbial communities associated with sludge digester were not affected by nanomaterials (Nyberg et al., 2008; Yang et al., 2012a). This discrepancy could be associated with variations in the physical and chemical properties of nanomaterials and their complex reaction with several other materials from either organic or inorganic sources (Mohanty et al., 2014).
Impact of nanomaterials on microbial community functions
The effect of nanomaterials on microbial community functions is another important aspect of NPs-induced community variation yet to be explored. Some studies have further highlighted possible alterations in microbial functions induced by the exposure of the environment to nanomaterials. These include functions associated with nutrient removal and methanogenesis in wastewater treatment plants. In a study by Alvarez and Cervantes (2012), the process of methane production was significantly inhibited by nanomaterials such as Al2O3 and its toxicity was reduced when coated with humic acids. In a similar study, Yang et al. (2012a) reported the effect of AgNPs on methane production in landfill bioreactors at different concentrations.
Several studies viz., Masrahi et al. (2014), Liang et al. (2010), and Li et al. (2014) also reported nitrogen removal processes using nanomaterials such as TiO2 and AgNPs. The negative impact of graphene oxide was also reported on wastewater treatment by removing nitrogen and phosphorus from waste materials (Ahmed and Rodrigues, 2013). To discuss the effect of nanomaterials on microbial diversity, structure and functions, organism-determined toxicity of nanomaterials is required i.e., each microorganism with susceptible nanomaterials because bacteria tolerate nanomaterials differently. For instance, Gram-positive bacteria react positively to single-walled carbon nanotubes by changing their membrane lipid composition. The mechanism of nanomaterials-microbe specificity is widely unknown and there is a need to further investigate the process (Jin et al., 2014; Mohanty et al., 2014).
Mechanisms of mitigating abiotic stress in plants
Abiotic stresses remain one of the most significant factors limiting the growth and yield of plant crops (Yadav, 2017). There is a need for plants to resist stressful edaphic and environmental conditions using either innate or induced biological mechanisms. For induced biological mechanisms to relieve the plant of unwanted stressors, an environmentally friendly method is needed to avoid complications associated with the use of synthetic chemicals. In this regard, the use of endophytic microbes remains one of the more reliable methods to mitigate the effects of abiotic stresses. Microbes are ubiquitous in diverse natural environments and exhibit diverse metabolic responses to manage soil stressors (Meena et al., 2017; Akinola and Babalola, 2020; Akinola et al., 2021b). Due to the proximity between plants and microbes in the soil, the plant microbiome induces local and systemic mechanisms in crop plants to cope with continuous changes to the environment. This synergism (plant-microbe) in the agroecosystem induces complex mechanisms within the plant cellular system (Figure 1). Interestingly, the continuous change in climatic conditions has paved the way for a better understanding of plant cellular complexity; researchers are constantly ruminating on questions associated with the physiological, molecular, and biochemical processes related to plant-microbe interplay (Glick, 2020; Akanmu et al., 2021).
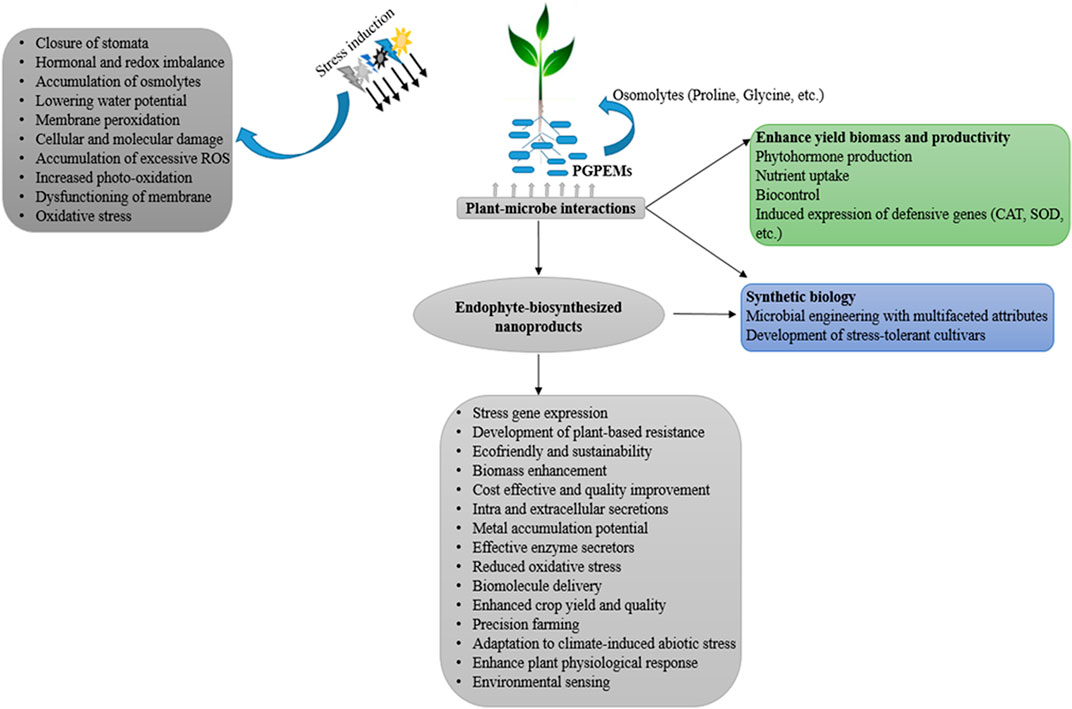
FIGURE 1. Concept of endophyte interactions and biosynthesized nanoproducts to alleviate abiotic stress and enhance crop yield.
Because of the growing concern about climate change, it is important to explicitly explicate the synergy between plant-soil-microbe about protection against abiotic stressors. Understanding the changes in different abiotic stresses induced by either anthropogenic or natural means is crucial to reducing the negative effects of environmental stress as it impacts agricultural productivity (Jalil and Ansari, 2019). A report by the Food and Agriculture Organization (FAO) of the United Nations on the challenges limiting global food productivity argued that one of the major problems faced by the scientific community in the effort to increase crop production is unwanted abiotic stressors (FAO, 2009). As such, there is a need to address the challenges associated with plant growth sustainably. These include eco-friendly technological processes and the efficient use of bioproducts to address the constraints posed by environmental stresses (FAO, 2009).
Several abiotic factors can limit plant growth and development including low or high temperature, heavy metal toxicity, soil alkalinity or acidity, drought, flooding and salinity (Emamverdian et al., 2015; Pasala et al., 2016; Jalil and Ansari, 2019). Abnormal soil acidity can lead to nutrient deficiency in plants thereby reducing essential physiological attributes needed to improve plant growth and development (Jalil and Ansari, 2019; Akinola and Babalola, 2020). Similarly, salt treatment induces toxicity in plant tissues, leading to osmotic imbalance and stress which hinders plant growth. In addition, abiotic stressors increase the production of ROS and induce phytotoxicity by negatively impacting protein structure and functions (Baral and Izaguirre-Mayoral, 2017; Mukhtar et al., 2018; Komaresofla et al., 2019).
Naturally, plant cell organelles viz., chloroplasts, peroxisomes and mitochondria help in producing ROS, with hydrogen peroxide and oxygen radicals being produced in the mitochondria. Hydrogen peroxide and oxygen are produced in the chloroplast (Jalil and Ansari, 2019). Using peroxidase dismutase, the peroxides are transformed into hydrogen peroxide (H2O2). The oxidation process involved in the conversion of xanthine and hypoxanthine to uric acid is achieved in the peroxisomal matrix using xanthine oxidase to generate oxygen radicals (Halliwell and Gutteridge, 2015; Jalil and Ansari, 2019). These radicals destroy cell biomolecules, such as DNA, carbohydrates, lipids, and proteins, resulting in cell death.
Plants can rapidly acclimatize to an abrupt change in the environment, such as unwanted abiotic conditions. A shift in the soil condition alters plant metabolic equilibrium and causes plant cells to modify genetic and metabolic processes (Tuteja and Mahajan, 2007; Tuteja and Sopory, 2008; Simontacchi et al., 2015). Plants then activate defense mechanisms needed to relieve unwanted stress conditions, reprogramming metabolic processes within the plant cell, thus facilitating bio-physicochemical relief of abiotic stress conditions (Massad et al., 2012; Mickelbart et al., 2015; Yolcu et al., 2016).
Due to uncertainty surrounding the mechanism of action of metallic NPs, different hypothetical mechanisms were frequently mentioned in different studies. These include that:
a) NPs aggregate and dissolve the cell membrane, resulting in the alteration of cell permeability and dissolution of the PMF—Proton motive force (McQuillan, 2010; Singh et al., 2019).
b) ROS—Reactive oxygen species that help in the destruction of the cellular structure are produced by metallic NPs and ions (Singh et al., 2019).
c) Absorption of metal ions by cells helps in the degradation of intracellular ATP and the disruption of DNA synthesis (Singh et al., 2019).
Oxidation reactions attributed to metallic ions in cells induce responses such as ROS—due to cell signal differentiation and cell death (Mueller et al., 2005). The integral components of ROS include peroxomonocarbonate (HOOCO2‾), peroxynitrate (O2NOO‾), peroxynitrite (ONOO‾), nitric compounds, hypochlorite and hypochlorous acids, peroxyl (RO2‾), and hydroperoxyl (HO2‾), and other oxygen-related compounds (Wu et al., 2014). With the catalysis of superoxidase dismutase (SOD), oxygen ions have a short lifespan due to instant reduction. NADPH—nicotinamide adenine dinucleotide phosphate oxidase in the mitochondria induces lipid peroxidation of the cell membrane (Singh et al., 2019). SOD initiates the complete conversion of oxygen into hydrogen peroxide. Physiologically, different detoxifying enzymes viz., glutathione peroxidase, catalase, SOD and antioxidants (flavonoids, vitamin E and ascorbic acids) modulate intracellular stages. Meanwhile, ROS activated by NPs of either CuO, ZnO or Ag plays a crucial role in genotoxicity. Oxidative stress degraded genetic materials are associated with different biological mechanisms viz., mutagenesis. Stress activation due to oxidative species results in nanotoxicity and the accumulation of oxidative stress leading to DNA destruction (Fu et al., 2014). The destruction of DNA because of OS; involves the breakage of single and double-stranded sugar bases, the generation of basic sites and DNA-protein crosslinks. Closely, hydroxyl radicals cause rapid damage to cells, whereas, from a distance, less-reactive ROS may interact easily (Fu et al., 2014).
Microbe-induced abiotic stress tolerance
An adaptation used in the process of abiotic stress tolerance is often referred to as induced systemic tolerance/resistance (IST/ISR). The intrinsic genetic and metabolic potential of microbes contribute immensely to the relief of plants from abiotic stresses (Gopalakrishnan et al., 2015). The role of endosphere and rhizosphere inhabitants belonging to different genera viz., Cyanobacteria (Singh et al., 2011), Trichoderma (Pandey et al., 2016; Igiehon and Babalola, 2021), Burkholderia (Naveed et al., 2014a; Naveed et al., 2014b), Methylobacterium (Meena et al., 2012), Bradyrhizobium (Tittabutr et al., 2013), Enterobacter (Sorty et al., 2016), Bacillus (Ashraf et al., 2004; Sorty et al., 2016), Pantoea (Sorty et al., 2016), Rhizobium (Igiehon and Babalola, 2021; Igiehon et al., 2021), Azospirillum (Omar et al., 2009), Azotobacter, and Pseudomonas (Ndeddy Aka and Babalola, 2016) have functional traits useful in improving plant growth under abiotic stresses. The functional role of Trichoderma harzianum in alleviating soil stresses through the upregulation of genes such as malonialdehyde, dehydrin, and aquaporin genes, has been reported by Pandey et al. (2016) on different serotypes of rice. Also, the synthesis of exopolysaccharides, antioxidants, protein defensins and phytohormones may be induced using plant growth-promoting rhizobacteria. Most of these functions are effective against drought and other abiotic stressors (Kaushal and Wani, 2016). Therefore, the effective productivity monitoring parameters, viz., screening, selection and inoculation of stress-mitigating microbes, can be helpful as a viable option to increase crop productivity to solve the problem of a growing world population with insufficient food (Akinola and Babalola, 2021). Trichoderma harzianum inoculation enhances the oil content of Brassica juncea inhibited by salinity stress and improves the plant’s physiological traits, such as reducing sodium ion uptake, enhancing osmolyte synthesis, antioxidant accumulation, and facilitating the uptake of essential plant nutrients (Ahmad et al., 2015). Similarly, ACC deaminase production was shown to be responsible for the upregulation of monodehydroascorbate reductase in B. juncea (Brotman et al., 2013). Also, the addition of Acinetobacter sp. and Pseudomonas sp. have been used to increase the production of ACC deaminase and indole-3-acetic acid (IAA) in oat and barley grown in salinity-stressed soil (Chang et al., 2014). Streptomyces sp. strain PGPA39 has also been used to alleviate salinity stress in tomato plants (Palaniyandi et al., 2014). In Arabidopsis, wheat, and maize plants, Burkholderia sp., has been used to relieve plants and soil of salt and drought stresses (Naveed et al. (2014a); Naveed et al. (2014b); Pinedo et al. (2015).
There are a large number of microorganisms within proximity to plant tissues and across the vicinity of plant roots because the plant root exudates provide diverse metabolites and nutrients which attract beneficial microorganisms. These metabolites are crucial to the microbial presence surrounding and attached to plants (Akinola et al., 2021a; Akinola and Babalola, 2021) with chemoattraction being associated with microbial movement toward these compounds (Meena et al., 2017). While utilizing these plant exudates, plant growth-beneficial microorganisms associated with the plant endosphere induce both direct and indirect mechanisms, such as biocontrol agents, phytostimulation, and biofertilization (Hayat et al., 2010; Akinola and Babalola, 2020).
Indirect mechanisms of plant growth promotion include the production of antimicrobial agents, hydrogen cyanide (HCN), and antibiotics, which exert antagonistic effects against plant pathogens. Direct mechanisms include nitrogen fixation, stimulation of plant hormone synthesis, solubilization of potassium and phosphorus, synthesis of siderophores which facilitate iron uptake, and sequestration of zinc and other micro-and macronutrients from the soil (Meena et al., 2017). In addition, many plant-associated microbes also induce systemic resistance against various phytopathogens triggered by plant secondary metabolites (Meena et al., 2017; Omomowo and Babalola, 2019). Apart from bacteria, mycorrhizal fungi are also good to plant growth promoters. These include both vesicular-arbuscular mycorrhiza (VAM) and other ectomycorrhizal fungi (Akinola and Babalola, 2021). These fungi use their extensive hyphal networking to increase plant nutrient uptake. For instance, in studies by Sun et al. (2010) and Baltruschat et al. (2008), an endophytic fungus—Piriformospora indica was used to improve drought and salinity tolerance in Chinese cabbage and barley, respectively. These processes were achieved by improving both physiological traits and the level of plant antioxidants. At some point, microbes activate systemic or local stress responses in plants under abiotic stress. In other instances, they activate direct responses to support plant growth and development. This complex and multipronged action of soil microbes makes them a vital and viable choice for disease suppression and abiotic stress control in plants (Franken, 2012; Meena et al., 2017).
Several mechanisms have highlighted the enormous benefit of plant-associated microbiomes (Kushwaha et al., 2020; Glick and Gamalero, 2021; Adeleke and Babalola, 2022). The microbes found in the plant root environment typically belong to the genera Pseudomonas, Klebsiella, Aeromonas, Azotobacter, Enterobacter, Bacillus, Azospirillum, and Achromobacter (Ortiz et al., 2015; Kaushal and Wani, 2016; Sorty et al., 2016; Babalola et al., 2021; Fasusi et al., 2021) (Table 1).

TABLE 1. Examples from the recent literature of the effect of plant growth-promoting microbes in the relief of plant stress.
All rhizosphere and endosphere bacteria with the ability to maintain plant growth under different adverse soil conditions are referred to as plant growth-promoting bacteria (PGPB) (Agri et al., 2022). There are other mechanisms plant microbes use to promote plant growth and development. IAA is produced to improve plant root development (Meena et al., 2017) where auxins initiate root growth and cell elongation. However, the high production of auxin may negatively affect root growth (Sorty et al., 2016; Akinola and Babalola, 2020). High auxin secretion also has drawbacks because of the increased ethylene production. In addition, the enzyme ACC deaminase is a key component in lowering the stress ethylene that results from both biotic and abiotic stress (Glick, 2004).
The mechanisms mentioned above have been reported in rhizosphere bacteria and fungi with enhanced phytohormones production for sustainable plant growth (Belimov et al., 2007; Ojuederie et al., 2019; Akinola et al., 2021c). Other studies have employed rhizobiomes to mitigate environmental stresses and improve the growth of crop plants in maize (Rojas-Tapias et al., 2012; Akinola et al., 2021a; Chaudhary A. et al., 2021), rice (Sharma et al., 2013), soybean (Sen and Chandrasekhar, 2014), and barley (Suarez et al., 2015).
The use of nanomaterials to relieve abiotic plant stresses
Plants possess several mechanisms needed to cope with unwanted soil conditions, including heat stress, drought, flooding, salinity, and chilling. Several researchers have studied molecular and cellular plant responses to abiotic stress (Gepstein and Glick, 2013; Ali and Glick, 2019; Santoyo et al., 2021a). Primarily, plants respond to abiotic stress using methods, such as an increase in MAPK (mitogen-activated protein kinase), abscisic acid, ROS, increased intracellular messenger viz., polyphosphate, inositol and raised Ca2+ in the cytoplasm as shown in Figure 2.
Meanwhile, stress relief responses, such as regulation of the expression of specific stress genes, and the proteins involved in the protection from cellular damage are involved in the advanced level of plant response. In addition, secondary metabolites ensure physiological processes to reduce abiotic stress conditions by activating the biosynthesis of polyamines signal transduction, ROS-induced photosystem protection and stabilizing cellular structure (Oh et al., 2009; Jalil and Ansari, 2019).
In mitigating soil stresses, the plant cell wall helps in plant adaptation and guides against stress perception. Induced peroxidases modify plant cell walls, which bring together oxidative stress and ROS when in contact with plant stressors (Rouet et al., 2006; Daudi et al., 2012). When plants encounter oxidative stress, immediate defense responses, such as the regulation of gene expression, enzyme production, phenylpropanoid aggregation, and ROS are produced (Daudi et al., 2012; Jalil and Ansari, 2019).
Although plants develop various mechanisms to initiate responses against adverse conditions. Nevertheless, their responses may differ even among the same plant species. Consequently, augmentation of stress tolerance in plants and identification of tolerant plant material remains conservative and ecofriendly methods towards sustainable agricultural practices and crop production (Akinola et al., 2022; Chaudhary et al., 2022). Nanoscience is an emerging multi-disciplinary area that involved the use of nanomaterials in different fields at the nano-level. The most promising application of nanoscience could be exploited in agroecosystem practices, food processing and packaging materials. In the current scenario, nanomaterials can be used as a tool to effectively promote plant growth and also ameliorate plant stressors (Saxena et al., 2016; Chaudhary et al., 2021b).
A lot has been done on the use of nanotechnological approaches to stress responses (Shabnam et al., 2014; Tripathi et al., 2015; Singh and Lee, 2016). The effect of NPs on sustainable plant growth and development is concentration-dependent, which also increases antioxidant enzyme activity. For example, in a study to assess the effect of TiO2 NPs on onion seedlings, TiO2 NPs increased the activity of the superoxidase dismutase enzyme (Laware and Raskar, 2014). Meanwhile, a drastic change in the physiological traits of the onion plant was noticed with an increased concentration of TiO2 NPs (Laware and Raskar, 2014). Under these conditions, the activities of the catalase and amylase enzymes decreased at lower concentrations of TiO2. In another study by Changmei et al. (2002), SiO2 and TiO2 NPs showed significant positive effects on the growth and sprouting of Glycine max seedlings.
Effect of NPs on heavy metal stressed plants
Contamination of the plant-soil environment by metallic ions is a severe menace to sustainable agricultural practices worldwide. Heavy metal stress increases plant toxicity, thus leading to retarded plant growth (Chibuike and Obiora, 2014; Jalil and Ansari, 2019). This happens due to decreased enzymatic activities induced by a continuous decrease in essential nutrients available in the soil (Sharma et al., 2012). Furthermore, heavy metal ions induce ROS production affecting the plant’s physiological properties; viz., membrane permeability reduction, cell structure deformation, and degradation of available plant cell protein. To relieve the constraints attributed to heavy metal stress, plants induce defense mechanisms including the production of polyphosphates, organic acids, and metal chelates, which all reduce the influx of metal ions and activate the synthesis of antioxidants to lower ROS production. The activation of these defense mechanisms ensures resistance against heavy metal stress. Moreover, the use of synthesized NPs can reduce the burden of phytotoxicity induced by heavy metals on plants (Sharma et al., 2012; Gunjan and Zaidi, 2014; Tripathi et al., 2015).
Because of the small size and surface area of synthesized NPs, they can easily penetrate plant cells and retain a high affinity for metallic ions. In a study by Worms et al. (2012), it was reported that quantum dots (i.e., the nanoparticles of a semiconductor) reduce Pb and Cu accessibility to plant cells. The report of Singh and Lee (2016) showed TiO2 NPs reduce Cd toxicity and improve physiological traits, viz., plant growth, and photosynthetic rate. The study of Li and Huang (2014) with Brassica juncea revealed the effect of hydroxyapatite NPs in the relief of cadmium toxicity. Similarly, synthesized SiNPs helped to reduce chromium toxicity in peas (Tripathi et al., 2015). Shabnam et al. (2014) also discovered that the treatment of cowpea with AuNPs induces a reduction of Au ions to a nontoxic form by phenolic compounds of cowpea seeds (Table 2).
Effect of NPs on heat stress
Exposing a plant to an extreme temperature for a long period results in retarded plant growth and development. Heat stress reduces photosynthetic and chlorophyll content, membrane ion leakage, protein degradation, and lipid depletion. This is because an increase in ROS generation induces oxidative stress (Wahid, 2007; Karuppanapandian et al., 2011; Prasad et al., 2011). Haghighi et al. (2014) reported the effect of low concentrations SeNPs in reducing heat stress by stimulating the photosynthetic ability of plants, increasing hydration, and improving plant growth.
Anti-oxidative properties of the plant have also been improved at low SeNPs levels, while high concentrations of SeNPs induce oxidative stress (Haghighi et al., 2014). Plants induce the production of molecular chaperones and heat shock proteins during heat stress to resist oxidative stress (Hasanuzzaman et al., 2013; Hasanuzzaman et al., 2014).
Furthermore, carbon nanotubes, such as HSP90 have been used to upregulate genes involved in heat shock protein synthesis. A study by Zhao et al. (2012) showed that the exposure of maize to CeO2NPs upregulates HSP70 and generates large amounts of hydrogen peroxide.
Effect of NPs on salinity stress
Salinity is an important abiotic stressor that deteriorates and limits the output of food crops. Owing to the susceptibility of most plants (i.e., lycophyte category) to salt stress, the majority of plant products are negatively affected, thereby reducing their economic value (Munns and Tester, 2008; Akinola and Babalola, 2020). Salinity stress hinders both physiological and biochemical processes associated with the sprouting of the plant.
Salinity causes one or more of the following: specific ionic toxicity, nutritional imbalance, and a reduced osmotic potential (Jalil and Ansari, 2019). In addition, some other critical physiological processes, such as lipid metabolism, protein synthesis, and photosynthesis, are often negatively affected (Parida and Das, 2005). The use of nano-based-fertilization processes proffers solutions to relieve unwanted plant stresses and enhance the efficient use of plant resources. Less than 50% of applied chemical pesticides and fertilizers are used by the plant; the remainder often increases soil toxicity. This problem and many other growth-impeding factors may be effectively resolved using nanoscience (Martínez-Ballesta et al., 2016). For instance, the use of SiNPs and Si-fertilizer has a sustainable effect on the morphological and physiology of basil plants (Ocimum basilicum) under salinity stress. The results of this study suggested that the change in the physiological traits may be a result of tolerance induction in the basil plant, which helps to mitigate the effect of salt stress (Kalteh et al., 2018). Many other studies have shown that SiO2NPs can relieve the effect of salinity stress. For example, Haghighi et al. (2014) and Sabaghnia and Janmohammadi (2015) showed the positive effects of SiNPs on Lens culinaris Medik. Under salinity stress, SiNPs was able to induce a significant increase in the growth of Lens culinaris Medik seedlings and the germination of seeds. Introducing SiNPs not only enhances early seedling growth and seed germination but also improves other growth features associated with the plant under salinity stress. In the same study by Haghighi et al. (2014) on tomatoes, SiO2NPs decreased ionic toxicity of the stressor leading to a substantial increase in the shoot, root fresh and dry weight of tomato plants under salt stress. Gao et al. (2006) showed the effect of SiO2NPs on maize plants after long exposure to salinity stress. Applying SiO2NPs enhanced the sprouting of the plant (Savvas et al., 2009), as shown in Table 2. The mechanism of action of silica nanoparticles reduces the Na+ ion concentration in the plant. As a result, limited Na+ ion is available for absorption by plant tissues. Since salinity stress increases Na+ ion uptake and osmotic potential, the process of contamination is reduced using SiO2NPs because of this mechanism of action (Raven, 1983).
In addition, multi-walled carbon nanotubes (MCN) have been tested against salinity-stressed broccoli plants (Martínez-Ballesta et al. (2016). The MCN-treated plants exhibited increased assimilation of CO2, aquaporin transduction, increased water uptake and modified the broccoli root plasma membrane which increased the sprouting of the plant.
Effect of NPs on chilling stress
Low temperatures can destroy plant cells because of ion leakage and permeability distortion of the plant cell membrane. This chilling stress leads to a reduction in plant growth and germination (Bhattacharya, 2022; Petruccelli et al., 2022) with tolerance to chilling varying between different plant species. The greater the damage to plant membranes, the more deleterious the effect of chilling stress on the plant (Rawat et al., 2020). In addition, photosynthesis and its biochemical components are greatly affected by chilling stress because low temperature damages the photosystems, inhibiting properties associated with light absorption, such as increasing Rubisco degradation, CO2 assimilation, transpiration rate, and reducing the chlorophyll content (Jajoo and Mathur, 2021; Sherin et al., 2022). To ensure the relief of plants from chilling stress, NPs are used to enhance photosystem activities by inhibiting ROS production, increasing the activities of the chloroplast, and improving the production of Rubisco enzymes (Ayyaz et al., 2022; Chandel et al., 2022).
TiO2NPs activate processes needed to enhance the synthesis of chlorophyll and the expression of the Rubisco binding protein gene, improve leaf pigment, antioxidant enzyme synthesis, and decrease the effect of chilling stress by reducing plant cell damage and ion leakage (Asadi and Cheniany, 2022; Sardar et al., 2022; Zare et al., 2022). Low-temperature stress upregulates the expression of MeAPX2 and ZnSOD/MeCu genes, which increases glutathione reductase, dehydroascorbate reductase, and monodehydroascorbate activities that remove ROS. It also helps to reduce oxidative stress (Sonkar et al., 2021). The use of TiO2NPs to reduce chilling stress has restructured plant biochemical physiognomies whenever plant cells are exposed to low-temperature environments (El-Gazzar et al., 2020; Elsheerya et al., 2020; Nasr et al., 2021).
Effect of NPs on drought stress
Soil drought is an abiotic stress limiting crop productivity in arid and semi-arid regions (Gamalero and Glick, 2022). Several studies have highlighted the effects of silicon NPs on drought-induced plant stress. For instance, SiNPs have been used to relieve the impact of drought stress on hawthorns (Crataegus sp.) (Ashkavand et al., 2015). The aforementioned study was conducted using different concentrations of SiNPs, depending on the severity of the stress. Biochemical and physiological responses differ in plant seedlings based on the positive effect of SiNPs on carbohydrate contents, proline, leaf pigments, membrane leakage, water content, malondialdehyde, and photosynthetic parameters (Ashkavand et al., 2015). A study was conducted to test the effect of SiNPs on two different sorghum (Sorghum bicolor (L.) Moench) cultivars with different drought tolerance susceptibility patterns, maintaining the photosynthetic rate and reducing the root-to-shoot ratio. This showed that SiNPs was able to augment plant water uptake efficacy (Hattori et al., 2005). Also, a low concentration of sodium silicate was used to mitigate the effect of drought stress on wheat (Pei et al., 2010). The silicon content of the compound was able to maintain the leaf potential in water absorption, improve the leaf chlorophyll content, and enhance shoot growth. Although the mechanism of action of this compound is yet to be determined, silicon compounds have been reported to be involved in the reduction of plant membrane lipid peroxidation.
In soybean, ZnONPs have been reported to boost the resilience of soybean plants to drought stress (Sedghi et al., 2013). This study revealed that the application of ZnONPs helped in the germination of soybean in a drought-stressed plant; an effect attributed to the role of Zn in the improvement of seed viability and sprouting of plant seeds in Zn deficient areas (Degenhardt and Gimmler, 2000).
Iron is an essential nutrient needed for plant growth and development; an iron-deficient plant shows physiological change, viz., chlorosis and reduced metabolism (Jalil and Ansari, 2019). Micronutrients can be used to relieve the effects of drought stress in some plants. Davar et al. (2014) showed the impact of exogenous FeNPs in the flowering and fruiting stages of a plant under drought stress. In addition, to reduce the adverse effects of drought stress, TiO2NPs have been applied to the leaves of wheat to improve agronomic and physiological features, such as gluten, starch, photosynthetic activities, biomass, harvest index, final yield and plant weight (Jaberzadeh et al., 2013).
Plant microbes in agriculture to address future food scarcity
Some bacteria and fungi can colonize the internal tissues of their host plants without causing any detrimental effects (Adeleke and Babalola, 2021b). Various bacterial and fungal endophytes produce plant growth traits, such as siderophores, nitrogen fixation, phosphate solubilization, antibiotic production and induced systemic resistance to various environmental stresses (Santoyo et al., 2016; Adeleke et al., 2021).
Plant roots absorb water and minerals from the soil, then translocate them to other plant parts (Liu et al., 2021). In addition, the plant produces copious amounts of exudates such as amino acids, organic acids, and sugars into the soil which are utilized by soil microbes and contribute to the microbial biomass in the root environment (Lyu et al., 2021). Also, seeds produce low molecular weight organic exudates into the surrounding soil during germination. Several endophytes have been reported to be present in the endosphere (He et al., 2021).
Endophytic relationships with the host plants can be symbiotic or pathogenic. Often, essential and uncommon organic substances are secreted by endophytes that assist in providing various functions, not only for soil health but also as a solution to plant stress challenges. Endophytes can often protect plants from phytopathogens and abiotic stresses (Table 3).
The impact of abiotic stressors on plant growth and soil health can be major or minor depending on the prevailing environmental conditions (Sachdev et al., 2021). Abiotic stresses negatively affect crop production and microbial diversity (Chouhan et al., 2021). Various mechanisms employed by endophytic microorganisms induce systemic resistance (ISR) or abiotic stress tolerance in plants (Gupta et al., 2021). Therefore, there is a need to restructure modern agricultural systems to include recent developments in endosphere biology (Santoyo et al., 2021b). Some studies have explained the role of endophytic bacteria in agricultural systems under abiotic stresses in combating future food scarcity. For instance, B. amyloliquefaciens RWL-1 producing ABA can enhance rice yield in soil with a high salt concentration (Ganie et al., 2021). This bacterium produces essential amino acids and salicylic acid which assist rice growth in salinity/drought conditions (Thepbandit et al., 2021). The endophytic fungus, Bipolaris sp., produces gibberellins which contribute to the growth of Glycine max (Lubna et al., 2022). Sphingomonas sp. LK11 is an endophyte from leguminous plants that also synthesizes gibberellins which enhance tomato growth and the plant chlorophyll content (Adeleke and Babalola, 2021a).
Various reports revealed the presence of IAA-producing endophytic bacteria (Rashid et al., 2012; Panigrahi et al., 2020; Turbat et al., 2020). Burkholderia kururiensis is an endophyte that stimulates the expression of IAA genes, especially in the roots of transgenic rice, thereby contributing to rice growth (Zhou et al., 2020).
Some endophytes are halotolerant, which can be isolated from the weed Psoralea corylifolia to assess their PGP activity in wheat. The growth of wheat plants can be improved with the aid of an extract from the bacterial isolates during the production of IAA under saline-stress conditions (Amini Hajiabadi et al., 2021). The identification of various strains embedded in weeds revealed various genera including Acinetobacter, Enterobacter, Marinobacterium, Pseudomonas, Rhizobium, and Sinorhizobium (AlSharari et al., 2022). The hormone cytokinin is also produced by some endophytic bacteria, according to Eid et al. (2021). Pseudomonas resinovorans and Paenibacillus polymyxa isolated from Gynura procumbens are good examples of endophytic bacteria producing cytokinin (Eid et al., 2021). From a bacterial culture, the obtained extracts were tested in vitro and inoculated into the cotyledon of cucumber to observe their cytokinin activity.
A strain of Sinorhizobium meliloti engineered to overproduce cytokinin by transferring the Agrobacterium tumefaciens ipt gene into the bacterium, and a strain of Pseudomonas spp., that protected alfalfa plants from drought-stressed conditions (Oleńska et al., 2020), these two bacteria were inoculated together or separately, in the cultivation of sorghum (Sorghum bicolor). The results revealed that the two strains inoculated together reduced the requirement for chemical nitrogen fertilizer to a low level and significantly improved the cultivation of sorghum. The procedure also enhanced the colonization effectiveness of both bacteria in the roots of rice plants (Abbaszadeh-Dahaji et al., 2020). In summary, endophytes can play a major role in modulating phytohormone levels in plants, thereby contributing to plant growth and managing various stress conditions.
Symbiotic nitrogen fixation reduces atmospheric nitrogen by the action of the leguminous plants in association with nitrogen-fixing bacteria, increasing the plant’s nutritional value (Rana et al., 2020). Several years ago, the only known nitrogen-fixing bacteria in legume nodules were rhizobia. However, numerous non-rhizobial bacterial species have been found in legume root nodules. Hanaka et al. (2021) reported how an endophytic bacteria isolated from the soybean, Bacillus mojavensis, exhibited biocontrol activity against the soybean pathogenic fungus, Rhizoctonia solani. These endophytic microorganisms have antagonistic activity against soil-borne pathogens and possess the ability to enhance the growth of soybean plants. The B. mojavensis strain produced ammonia, HCN, and siderophores. It also contributed to chitinase activity and solubilization of phosphate (Hanaka et al., 2021). The inoculation of this endophyte onto soybean seeds can help control various pathogens.
The introduction of nanotechnology in agriculture is a developing sector in agriculture despite several applications, and the true possibilities are yet to be obtained. Nanotechnology constitutes certain substances with unusual features that are revealed either as a result of the quantum confinement effects or the production of certain reactive surfaces that are at a nanoscale (Umapathy et al., 2022). The nanoscale degree when compared with the macroscopic level, reveals the properties of the material that are special as a result of the reduced size, shape of nanomaterials, and greater surface area-to-weight ratio. Nanomaterials or nanoparticles (NPs) have beneficial properties, with high reactivity, modified bioactivity, and surface effects (Bruchiel-Spanier et al., 2022). NPs are produced by either a single element like silver (Au) or gold (Au) or by a mixture of elements, which are observed in those constituting oxides like titanium oxide (TiO2), silicon oxide (SiO2), and zinc oxide (ZnO) (Behl et al., 2022). Gold and silver NPs regarded as inorganic NPs, are relevant as a result of their application. Although, few studies have shown how the NPs are manufactured from endophytes (Fadiji et al., 2022a; Kaur et al., 2022; Saqib et al., 2022), thus suggesting possible means for continuous studies on endophytic nanoparticles.
Various approaches including biological, chemical, and physical approaches are made use of in the production of NP, yet, the process of biosynthesis is environmentally friendly, free of chemical derivatives that are hazardous to humans, animals, and the environment. These chemicals have been used recently to reduce the potential of applying chemicals in the biomedical and food processing industries. Several procedures involving intracellular and extracellular for the biological synthesis of nanomaterials coexist in nature. In this field, the study is still understudied (Qian et al., 2022). The production of environmentally friendly, and non-toxic biological materials for manufacturing NPs would result in supporting the production of natural materials with the aid of living organisms (Fadiji et al., 2022a).
NPs produced from metal-based are manufactured by microorganisms through extracellular and intracellular mechanisms (Franco et al., 2022). The process of electrostatic induction takes place in metallic ions between negative and positive charges intracellularly in the cell wall of the microorganisms, followed by the decrease of the metal ions to their metallic form. Cell disruption is a constitutional prerequisite to acquiring pure NPs (Fadiji et al., 2022a). The biomass extracts from the cell, or culture supernatant when added to the solution of metals produce NPs outside the cell of the microorganisms (extracellularly) (Jadoun et al., 2022). Reductases are produced and liberated into the culture medium by cofactors, with microbial cells that execute this procedure. Endophytic microbes have been suggested as biofactories for the synthesis of metal-based NPs with agricultural and therapeutic applications. These microbes are embedded in plants intercellularly, producing a symbiotic link (Roy et al., 2022). The advantage confers on the plant by the endophytes is to improve health status via various mechanisms, like the release of antimicrobial (antibacterial and antifungal) compounds and the secretion of growth-promoting metabolites (Shahid et al., 2022).
In plant tissues, endophytic microbes accommodating them can produce nanoparticles, which are advantageous to the host plant by promoting plant growth or reducing the prevalence of diseases (Koné et al., 2022). Endophytes can resist metals occurring in the environment to alleviate toxicity and stress in the host plant, as well as improve their beneficial association and adaption over other microbes inhabiting the ecosystem (Mathur and Ulanova, 2022). The potential of endophytes to take away metals can be applied to manufacture metal-based NPs via extracellular and intracellular processes (Table 4). Typical examples were obtained in the production of AgNPs, which have a spherical shape and a mean size of 22 nm–45 nm, which can be manufactured intracellularly employing the supernatant of Ag-resistant Bacillus safensis TEN12 (Ahmed et al., 2020). Zinc oxide (ZnO) NPs sized 2 nm–9 nm, were produced extracellularly by the zinc-tolerant endophyte Curvularia geniculata (Ahmed et al., 2020). Gürsoy et al. (2021) reported how gold nanoparticles (AuNPs) sized 20 nm–40 nm were produced intracellularly in the cell wall and cytoplasm of Chlorella sorokiniana. Cobalt oxide nanoparticles (CoONPs), which are spherical at 20 nm in diameter, were produced extracellularly by the A. nidulans that are CoO-tolerant (Ahmed et al., 2020). Aspergillus nidulans are endophytes, which reveal the potential of the CoO-tolerant produced spherical CoONPs with a diameter of 20 nm through an extracellular tract (Fadiji et al., 2022a). Endophytic microorganisms can produce some biological active materials with a broad gap of structural and biological potential, which can be employed to examine the health and promote plant growth and are significant in improving the sustainability of agriculture (Kumar and Nautiyal, 2022). Fungi and bacteria isolated from parts of plants can be cultured in the laboratory under the most desirable growth conditions to synthesize NPs with the needed characteristics for application in the agricultural sector (Elnahal et al., 2022).
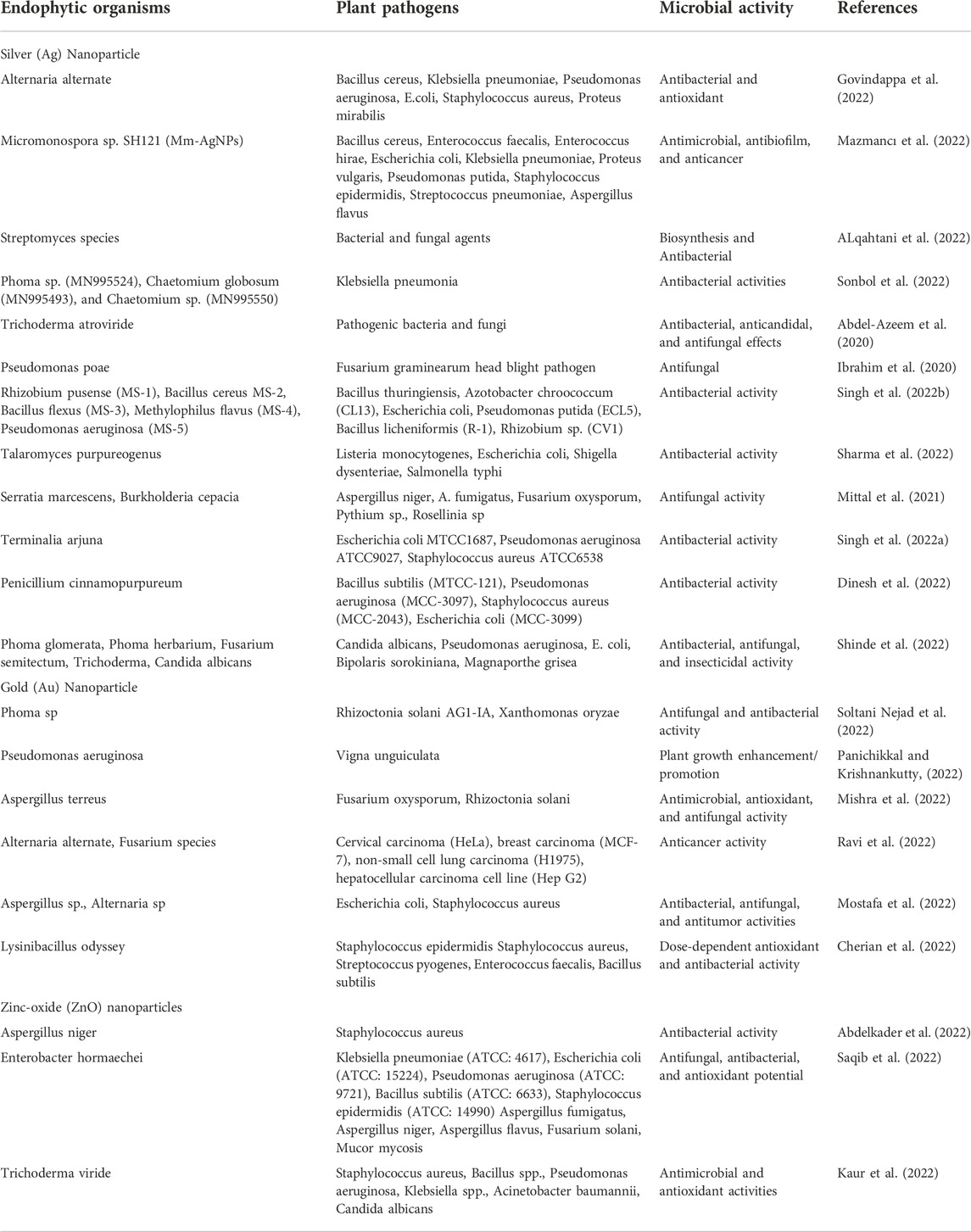
TABLE 4. The biological activities of nanoparticle-synthesizing endophytic microbes against plant pathogens.
Fate of NPs
The use of NPs for agroecosystem practices could be a very complicated matrix and information on the fate of nanomaterials in the soil is inadequate. After applying NPs to the soil, they are absorbed in the plant tissue directly. Such interaction could either increase or decrease the bioavailability and toxicity of NPs depending on the physicochemical properties of the soil. However, the potency of NPs is most dependent on the type of crop and the properties of the soil viz., microbial community, clay content, ionic strength, pH, salinity, organic matter, etc., (Reddy et al., 2016; Thiagarajan and Ramasubbu, 2021).
Effect of microbial community
Plant endophyte and the microbial community helps in the transformation of NPs. They help to recycle nutrients, effectively decomposition of organic compounds and conserve soil quality. The introduction of nanomaterials could affect microbial inhabitation which invariably reduces plant productivity (Jacoby et al., 2017; Chavan et al., 2020). The effect of NPs on the rhizosphere bacteria of butter crunch lettuce was reported by Kibbey and Strevett (2019). NPs and rhizosphere bacteria react together via electrostatic interactions that affect the surface properties of bacteria, which disallow easy attachment to the root surfaces of the plant. They also reported that fluctuations in soil mineral nutrients (P, Fe, Mn, etc.) were a result of spiked biosolid amendment of soil with NPs, which affects soil microbial load. Withal, NPs were also reported to have affected the sequestration of plant nutrients and other agroecosystem processes as reported by Bellani et al. (2020).
At times, NPs may also inhibit the colonization of plant growth promoters when combined with other nutrients. This deleterious effect was reported by Liu et al. (2020). Here, the negative effect associated with the combination of TiO2NPs with Cu2 (OH)2 CO3 was reported. The synergy between TiO2NPs and Cu2 (OH)2 CO3 boosted photocatalytic disinfection processes that disabled the effect of microorganisms such as Fusarium graminearum and E. coli within a short time of application (Liu et al., 2020).
Effect of clay content
Another important component that determines the fate of NPs is the clay content of the soil. The high the clay content of the soil, the decreased mobility of NPs because both the physical straining and electrostatic interactions would be increased. However, the soil retention capacity depends mainly on the clay to NPs ratio. The higher the ratio, the better the soil retention capacity (Shah et al., 2016). Metals are easily retained in the soil when there is an increase in the clay content. And as such reduces the uptake of metallic ions by the plant. This simply indicates the low toxicity of metallic NPs (Larue et al., 2018; Thiagarajan and Ramasubbu, 2021).
Effect of natural organic matter
NOM influences the stability and aggregation of NPs within the soil. NOM includes mobile and reactive organic fractions viz. hydrocarbons, amino acids, hydrophilic acids, fatty acids, fulvic, and humic acids. NOM is produced through the disintegration of animal and plant remains in the soil. Sludge-amended soil and NOM have been reported to cover 10.9% and 8.9% of soil, respectively. Meanwhile, the physicochemical properties of NPs and features of proximal soil are usually influenced after the adsorption of NOM (Bakshi et al., 2019). A recent study by Zhang et al. (2020) showed the stability of TiO2NPs when introduced to a paddy field with high organic matter. In another study that demonstrated the effect of a high concentration of NOM on the bioaccumulation of NPs, the ability of NOM to retrain NPs could be attributed to the change in the surface area of soil as a result of an increase in the concentration of NOM. The interaction of NPs with NOM alters the binding property of the soil to improve the steric repulsion between nanomaterials by aggregating and retaining them in the soil. Asides, the reaction of NPs and NOM depends greatly on their particle sizes through hydrophobic interaction (Lee et al., 2011).
Conclusion
Globally, crop improvement and productivity are faced with diverse abiotic stress challenges. To avert this problem, the use of nanomaterials from endophytic microbes has the potential to mitigate the effects of abiotic stresses affecting plants by stimulating plant defense mechanisms. Beneficial endophytic microbes as bioinoculants can be effectively harnessed for various ecological purposes such as abiotic stress reduction, nutrient absorption, enhancing photosynthesis, increasing plant growth parameters, and obviating agrochemical use. Additionally, the nanomaterial synthesizing endophytic microbes promise to improve crop productivity sustainably.
Author contributions
All authors listed have made a substantial, direct, and intellectual contribution to the work and approved it for publication.
Funding
This research was funded by the National Research Foundation of South Africa (UID: 123634; 132595).
Acknowledgments
National Research Foundation of South Africa and The World Academy of Science (NRF-TWAS) African Renaissance is acknowledged for a Doctoral scholarship stipend to BA (UID: 116100). SA and AA are grateful to North-West University, South Africa, for the postgraduate bursary. OB recognizes NRF for grants (UID: 123634; 132595) that support work in her research group.
Conflict of interest
The authors declare that the research was conducted in the absence of any commercial or financial relationships that could be construed as a potential conflict of interest.
Publisher’s note
All claims expressed in this article are solely those of the authors and do not necessarily represent those of their affiliated organizations, or those of the publisher, the editors and the reviewers. Any product that may be evaluated in this article, or claim that may be made by its manufacturer, is not guaranteed or endorsed by the publisher.
References
Abbaszadeh-Dahaji, P., Masalehi, F., and Akhgar, A. (2020). Improved growth and nutrition of sorghum (Sorghum bicolor) plants in a low-fertility calcareous soil treated with plant growth–promoting rhizobacteria and Fe-edta. J. Soil Sci. Plant Nutr. 20, 31–42. doi:10.1007/s42729-019-00098-9
Abd-Alla, M. H., Nafady, N. A., Bashandy, S. R., and Hassan, A. A. (2019). Mitigation of effect of salt stress on the nodulation, nitrogen fixation and growth of chickpea (Cicer arietinum L.) by triple microbial inoculation. Rhizosphere 10, 100148. doi:10.1016/j.rhisph.2019.100148
Abdel-Azeem, A., Nada, A., O’donovan, A., Kumar Thakur, V., and Elkelish, A. (2020). Mycogenic silver nanoparticles from endophytic Trichoderma atroviride with antimicrobial activity. J. Renew. Mat. 8, 171–185. doi:10.32604/jrm.2020.08960
Abdel-Aziz, S. M., Prasad, R., Hamed, A. A., and Abdelraof, M. (2018). “Fungal nanoparticles: A novel tool for a green biotechnology?,” in Fungal nanobionics: Principles and applications (Springer), 61–87.
Abdelkader, D. H., Negm, W. A., Elekhnawy, E., Eliwa, D., Aldosari, B. N., and Almurshedi, A. S. (2022). Zinc oxide nanoparticles as potential delivery carrier: Green synthesis by Aspergillus niger endophytic fungus, characterization, and in vitro/in vivo antibacterial activity. Pharm. (Basel). 15, 1057. doi:10.3390/ph15091057
Aboyewa, J. A., Sibuyi, N. R., Meyer, M., and Oguntibeju, O. O. (2021). Green synthesis of metallic nanoparticles using some selected medicinal plants from southern Africa and their biological applications. Plants 10, 1929. doi:10.3390/plants10091929
Acuña, J. J., Campos, M., De La Luz Mora, M., Jaisi, D. P., and Jorquera, M. A. (2019). ACCD-producing rhizobacteria from an Andean Altiplano native plant (Parastrephia quadrangularis) and their potential to alleviate salt stress in wheat seedlings. Appl. Soil Ecol. 136, 184–190. doi:10.1016/j.apsoil.2019.01.005
Adeleke, B. S., Ayilara, M. S., Akinola, S. A., and Babalola, O. O. (2022). Biocontrol mechanisms of endophytic fungi. Egypt. J. Biol. Pest Control 32, 46–17. doi:10.1186/s41938-022-00547-1
Adeleke, B. S., and Babalola, O. O. (2021a). Biotechnological overview of agriculturally important endophytic fungi. Hortic. Environ. Biotechnol. 62, 507–520. doi:10.1007/s13580-021-00334-1
Adeleke, B. S., Babalola, O. O., and Glick, B. R. (2021). Plant growth-promoting root-colonizing bacterial endophytes. Rhizosphere 20, 100433. doi:10.1016/j.rhisph.2021.100433
Adeleke, B. S., and Babalola, O. O. (2022). Meta-omics of endophytic microbes in agricultural biotechnology. Biocatal. Agric. Biotechnol. 42, 102332. doi:10.1016/j.bcab.2022.102332
Adeleke, B. S., and Babalola, O. O. (2021b). Pharmacological potential of fungal endophytes associated with medicinal plants: A review. J. Fungi (Basel). 7, 147. doi:10.3390/jof7020147
Agri, U., Chaudhary, P., Sharma, A., and Kukreti, B. (2022). Physiological response of maize plants and its rhizospheric microbiome under the influence of potential bioinoculants and nanochitosan. Plant Soil 474, 451–468. doi:10.1007/s11104-022-05351-2
Ahmad, F., Siddiqui, M. A., Babalola, O. O., and Wu, H.-F. (2012a). Biofunctionalization of nanoparticle assisted mass spectrometry as biosensors for rapid detection of plant associated bacteria. Biosens. Bioelectron. X. 35, 235–242. doi:10.1016/j.bios.2012.02.055
Ahmad, M., Zahir, Z. A., Asghar, H. N., and Arshad, M. J. a. O. M. (2012b). The combined application of rhizobial strains and plant growth promoting rhizobacteria improves growth and productivity of mung bean (Vigna radiata L.) under salt-stressed conditions. Ann. Microbiol. 62, 1321–1330. doi:10.1007/s13213-011-0380-9
Ahmad, M., Zahir, Z. A., Nazli, F., Akram, F., Arshad, M., and Khalid, M. J. B. J. O. M. (2013). Effectiveness of halo-tolerant, auxin producing Pseudomonas and Rhizobium strains to improve osmotic stress tolerance in mung bean (Vigna radiata L.). Braz. J. Microbiol. 44, 1341–1348. doi:10.1590/s1517-83822013000400045
Ahmad, P., Hashem, A., Abd-Allah, E. F., Alqarawi, A., John, R., Egamberdieva, D., et al. (2015). Role of Trichoderma harzianum in mitigating NaCl stress in Indian mustard (Brassica juncea L) through antioxidative defense system. Front. Plant Sci. 6, 868. doi:10.3389/fpls.2015.00868
Ahmadpour, K. S., Salari, S., and Ghasemi, N. A. P. (2021). Comparison of antifungal and cytotoxicity activities of titanium dioxide and zinc oxide nanoparticles with amphotericin B against different Candida species: In vitro evaluation. J. Clin. Lab. Anal. 35, 23577. doi:10.1002/jcla.23577
Ahmed, B., Cao, B., Mishra, B., Boyanov, M. I., Kemner, K. M., Fredrickson, J. K., et al. (2012). Immobilization of U (VI) from oxic groundwater by Hanford 300 Area sediments and effects of Columbia River water. Water Res. 46, 3989–3998. doi:10.1016/j.watres.2012.05.027
Ahmed, F., and Rodrigues, D. F. (2013). Investigation of acute effects of graphene oxide on wastewater microbial community: A case study. J. Hazard. Mat. 256, 33–39. doi:10.1016/j.jhazmat.2013.03.064
Ahmed, T., Noman, M., Manzoor, N., Shahid, M., Abdullah, M., Ali, L., et al. (2021). Nanoparticle-based amelioration of drought stress and cadmium toxicity in rice via triggering the stress responsive genetic mechanisms and nutrient acquisition. Ecotoxicol. Environ. Saf. 209, 111829. doi:10.1016/j.ecoenv.2020.111829
Ahmed, T., Shahid, M., Noman, M., Bilal Khan Niazi, M., Zubair, M., Almatroudi, A., et al. (2020). Bioprospecting a native silver-resistant Bacillus safensis strain for green synthesis and subsequent antibacterial and anticancer activities of silver nanoparticles. J. Adv. Res. 24, 475–483. doi:10.1016/j.jare.2020.05.011
Ajilogba, C. F., Babalola, O. O., and Nikoro, D. (2021). “Nanotechnology as vehicle for biocontrol of plant diseases in crop production,” in Food security and safety: Africa’s Perspective. Editor O. O. Babalola (Hardcover: eBook), 709–724. 978-3-030-50672-8978-3-030-50671-1.
Akanmu, A. O., Babalola, O. O., Venturi, V., Ayilara, M. S., Adeleke, B. S., Amoo, A. E., et al. (2021). Plant disease management: Leveraging on the plant-microbe-soil interface in the biorational use of organic amendments. Front. Plant Sci. 12, 700507. doi:10.3389/fpls.2021.700507
Akinola, S. A., Adeyemo, R. O., and Adebayo, I. A. (2022). “Overview and emergence of nanobiotechnology in plants,” in Diverse applications of nanotechnology in the biological sciences (Apple Academic Press), 173–197.
Akinola, S. A., Ayangbenro, A. S., and Babalola, O. O. (2021c). Metagenomic insight into the community structure of maize-rhizosphere bacteria as predicted by different environmental factors and their functioning within plant proximity. Microorganisms 9, 1419. doi:10.3390/microorganisms9071419
Akinola, S. A., Ayangbenro, A. S., and Babalola, O. O. (2021a). The diverse functional genes of maize rhizosphere microbiota assessed using shotgun metagenomics. J. Sci. Food Agric. 101, 3193–3201. doi:10.1002/jsfa.10948
Akinola, S. A., Ayangbenro, A. S., and Babalola, O. O. (2021b). The immense functional attributes of maize rhizosphere microbiome: A shotgun sequencing approach. Agriculture 11, 118. doi:10.3390/agriculture11020118
Akinola, S. A., and Babalola, O. O. (2021). The fungal and archaeal community within plant rhizosphere: A review on their contribution to crop safety. J. Plant Nutr. 44, 600–618. doi:10.1080/01904167.2020.1845376
Akinola, S. A., and Babalola, O. O. (2020). The importance of adverse soil microbiomes in the light of omics: Implications for food safety. Plant Soil Environ. 66, 421–430. doi:10.17221/118/2020-pse
Ali, S., and Glick, B. R. (2019). “Plant–bacterial interactions in management of plant growth under abiotic stresses,” in New and future developments in microbial biotechnology and bioengineering (Elsevier), 21–45.
Alqahtani, M. S., Hozzein, W. N., and Alharbi, S. A. (2022). Biosynthesis and antibacterial activity of silver nanoparticles using medicinal plants associated endophytic bacteria from in Riyadh Region Saudi Arabia. Sch. Acad. J. Biosci. 4, 68–76. doi:10.36347/sajb.2022.v10i04.001
Alsharari, S. S., Galal, F. H., and Seufi, A. M. (2022). Composition and diversity of the culturable endophytic community of six stress-tolerant dessert plants grown in stressful soil in a hot dry desert region. J. Fungi (Basel). 8, 241. doi:10.3390/jof8030241
Álvarez, C., Navarro, J. A., Molina-Heredia, F. P., and Mariscal, V. (2020). Endophytic colonization of rice (Oryza sativa L.) by the symbiotic strain Nostoc punctiforme PCC 73102. Mol. Plant. Microbe. Interact. 33, 1040–1045. doi:10.1094/mpmi-01-20-0015-sc
Alvarez, L. H., and Cervantes, F. J. (2012). Assessing the impact of alumina nanoparticles in an anaerobic consortium: Methanogenic and humus reducing activity. Appl. Microbiol. Biotechnol. 95, 1323–1331. doi:10.1007/s00253-011-3759-4
Amini Hajiabadi, A., Mosleh Arani, A., Ghasemi, S., Rad, M. H., Etesami, H., Shabazi Manshadi, S., et al. (2021). Mining the rhizosphere of halophytic rangeland plants for halotolerant bacteria to improve growth and yield of salinity-stressed wheat. Plant Physiol. biochem. 163, 139–153. doi:10.1016/j.plaphy.2021.03.059
Anam, G. B., Reddy, M. S., and Ahn, Y.-H. (2019). Characterization of Trichoderma asperellum RM-28 for its sodic/saline-alkali tolerance and plant growth promoting activities to alleviate toxicity of red mud. Sci. Total Environ. 662, 462–469. doi:10.1016/j.scitotenv.2019.01.279
Andreolli, M., Zapparoli, G., Lampis, S., Santi, C., Angelini, E., and Bertazzon, N. (2021). In vivo endophytic, rhizospheric and epiphytic colonization of Vitis vinifera by the plant-growth promoting and antifungal strain Pseudomonas protegens MP12. Microorganisms 9, 234. doi:10.3390/microorganisms9020234
Ansari, F. A., Ahmad, I., and Pichtel, J. (2019). Growth stimulation and alleviation of salinity stress to wheat by the biofilm forming Bacillus pumilus strain FAB10. Appl. Soil Ecol. 143, 45–54. doi:10.1016/j.apsoil.2019.05.023
Armada, E., Azcón, R., López-Castillo, O. M., Calvo-Polanco, M., and Ruiz-Lozano, J. M. (2015a). Autochthonous arbuscular mycorrhizal fungi and Bacillus thuringiensis from a degraded Mediterranean area can be used to improve physiological traits and performance of a plant of agronomic interest under drought conditions. Plant Physiol. biochem. 90, 64–74. doi:10.1016/j.plaphy.2015.03.004
Armada, E., Barea, J.-M., Castillo, P., Roldán, A., and Azcón, R. J. a. S. E. (2015b). Characterization and management of autochthonous bacterial strains from semiarid soils of Spain and their interactions with fermented agrowastes to improve drought tolerance in native shrub species. Appl. Soil Ecol. 96, 306–318. doi:10.1016/j.apsoil.2015.08.008
Asadi, A., and Cheniany, M. (2022). Enhancing effect of titanium dioxide nanoparticles on growth, phenolic metabolites production and antioxidant potential of Ziziphora clinopodioides Lam. Russ. J. Plant Physiol. 69, 74. doi:10.1134/s1021443722040021
Ashkavand, P., Tabari, M., Zarafshar, M., Tomaskova, I., and Struve, D. (2015). Effect of SiO2 nanoparticles on drought resistance in hawthorn seedlings. For. Res. Pap. 76, 350–359. doi:10.1515/frp-2015-0034
Ashraf, M., Hasnain, S., Berge, O., and Mahmood, T. (2004). Inoculating wheat seedlings with exopolysaccharide-producing bacteria restricts sodium uptake and stimulates plant growth under salt stress. Biol. Fertil. Soils 40, 157–162. doi:10.1007/s00374-004-0766-y
Audah, K. A. (2019). Nanotechnology: Applications in energy, drug and food. Springer, 249–265.Drug discovery: A biodiversity perspective
Ayyaz, A., Fang, R., Ma, J., Hannan, F., Huang, Q., Athar, H., et al. (2022). Calcium nanoparticles (Ca-NPs) improve drought stress tolerance in Brassica napus by modulating the photosystem II, nutrient acquisition and antioxidant performance. NanoImpact 28, 100423. doi:10.1016/j.impact.2022.100423
Azimi, R., Borzelabad, M. J., Feizi, H., and Azimi, A. (2014). Interaction of SiO2 nanoparticles with seed prechilling on germination and early seedling growth of tall wheatgrass (Agropyron elongatum L.). Pol. J. Chem. Technol. 16, 25–29. doi:10.2478/pjct-2014-0045
Babalola, O. O., Fasusi, O. A., Amoo, A. E., and Ayangbenro, A. S. (2021). Complete genome sequence of a plant growth-promoting rhizobacterium, Bacillus sp. strain OA1, isolated from soybeans. Biocatal. Agric. Biotechnol. 36, 102121. doi:10.1016/j.bcab.2021.102121
Baker, S., Kavitha, K. S., Yashavantha Rao, H. C., Rakshith, D., Harini, B. P., Kumar, K., et al. (2015a). Bacterial endo-symbiont inhabiting Tridax procumbens L. and their antimicrobial potential. Chin. J. Biol. 2015, 1–6. doi:10.1155/2015/309267
Baker, S., Kumar, K. M., Santosh, P., Rakshith, D., and Satish, S. (2015b). Extracellular synthesis of silver nanoparticles by novel Pseudomonas veronii AS41G inhabiting Annona squamosa L. and their bactericidal activity. Spectrochimica Acta Part A Mol. Biomol. Spectrosc. 136, 1434–1440. doi:10.1016/j.saa.2014.10.033
Bakshi, M., Liné, C., Bedolla, D. E., Stein, R. J., Kaegi, R., Sarret, G., et al. (2019). Assessing the impacts of sewage sludge amendment containing nano-TiO2 on tomato plants: A life cycle study. J. Hazard. Mat. 369, 191–198. doi:10.1016/j.jhazmat.2019.02.036
Bal, H. B., Nayak, L., Das, S., and Adhya, T. K. (2013). Isolation of ACC deaminase producing PGPR from rice rhizosphere and evaluating their plant growth promoting activity under salt stress. Plant Soil 366, 93–105. doi:10.1007/s11104-012-1402-5
Baltruschat, H., Fodor, J., Harrach, B. D., Niemczyk, E., Barna, B., Gullner, G., et al. (2008). Salt tolerance of barley induced by the root endophyte Piriformospora indica is associated with a strong increase in antioxidants. New Phytol. 180, 501–510. doi:10.1111/j.1469-8137.2008.02583.x
Baral, B., and Izaguirre-Mayoral, M. L. (2017). “Purine-derived ureides under drought and salinity,” in Advances in agronomy (Elsevier), 167–204.
Battin, T. J., Kammer, F. V., Weilhartner, A., Ottofuelling, S., and Hofmann, T. (2009). Nanostructured TiO2: Transport behavior and effects on aquatic microbial communities under environmental conditions. Environ. Sci. Technol. 43, 8098–8104. doi:10.1021/es9017046
Behl, T., Kaur, I., Sehgal, A., Singh, S., Sharma, N., Bhatia, S., et al. (2022). The dichotomy of nanotechnology as the cutting edge of agriculture: Nano-farming as an asset versus nanotoxicity. Chemosphere 288, 132533. doi:10.1016/j.chemosphere.2021.132533
Belimov, A., Dodd, I., Safronova, V., Hontzeas, N., and Davies, W. (2007). Pseudomonas brassicacearum strain Am3 containing 1-aminocyclopropane-1-carboxylate deaminase can show both pathogenic and growth-promoting properties in its interaction with tomato. J. Exp. Bot. 58, 1485–1495. doi:10.1093/jxb/erm010
Bellani, L., Siracusa, G., Giorgetti, L., Di Gregorio, S., Castiglione, M. R., Spanò, C., et al. (2020). TiO2 nanoparticles in a biosolid-amended soil and their implication in soil nutrients, microorganisms and Pisum sativum nutrition. Ecotoxicol. Environ. Saf. 190, 110095. doi:10.1016/j.ecoenv.2019.110095
Bhattacharya, A. (2022). “Low temperature stress and plant-water relationship: A review,” in Physiological processes in plants under low temperature stress (Singapore: Springer), 107–197.
Brotman, Y., Landau, U., Cuadros-Inostroza, Á., Takayuki, T., Fernie, A. R., Chet, I., et al. (2013). Trichoderma-plant root colonization: Escaping early plant defense responses and activation of the antioxidant machinery for saline stress tolerance. PLoS Pathog. 9, e1003221. doi:10.1371/journal.ppat.1003221
Bruchiel-Spanier, N., Betsis, S., Naim, G., and Mandler, D. (2022). Electrochemical and electrophoretic coatings of medical implants by nanomaterials. J. Solid State Electrochem. 26, 1871–1896. doi:10.1007/s10008-022-05235-6
Cao, B., Majors, P. D., Ahmed, B., Renslow, R. S., Silvia, C. P., Shi, L., et al. (2012). Biofilm shows spatially stratified metabolic responses to contaminant exposure. Environ. Microbiol. 14, 2901–2910. doi:10.1111/j.1462-2920.2012.02850.x
Chandel, S. S., Shree, B., Mondal, K., and Mali, S. (2022). “Mechanism of nanoparticles-mediated alleviating biotic and abiotic stresses in agricultural crops: Recent advances and future perspectives,” in The Role of nanoparticles in plant nutrition under soil pollution. Sustainable plant nutrition in a changing world. Editors V. D. Rajput, K. K. Verma, N. Sharma, and T. Minkina (Cham: Springer), 117–139.
Chandra, D., Srivastava, R., Glick, B. R., and Sharma, A. K. J. P. (2018). Drought-tolerant Pseudomonas spp. improve the growth performance of finger millet (Eleusine coracana (L.) Gaertn.) under non-stressed and drought-stressed conditions. Pedosphere 28, 227–240. doi:10.1016/s1002-0160(18)60013-x
Chang, P., Gerhardt, K. E., Huang, X.-D., Yu, X.-M., Glick, B. R., Gerwing, P. D., et al. (2014). Plant growth-promoting bacteria facilitate the growth of barley and oats in salt-impacted soil: Implications for phytoremediation of saline soils. Int. J. Phytoremediation 16, 1133–1147. doi:10.1080/15226514.2013.821447
Changmei, L., Chaoying, Z., Junqiang, W., Guorong, W., and Mingxuan, T. (2002). Research of the effect of nanometer materials on germination and growth enhancement of Glycine max and its mechanism. Soybean Sci. 21, 168–171.
Chaudhary, A., Chaudhary, P., Upadhyay, A., Kumar, A., and Singh, A. (2021a). Effect of Gypsum on plant growth promoting rhizobacteria. Environ. Ecol. 39, 1248–1256.
Chaudhary, P., Chaudhary, A., Bhatt, P., Kumar, G., Khatoon, H., Rani, A., et al. (2022). Assessment of soil health indicators under the influence of nanocompounds and Bacillus spp. in field condition. Front. Environ. Sci. 9, 769871. doi:10.3389/fenvs.2021.769871
Chaudhary, P., Chaudhary, A., Khati, P., and Kumar, G. (2021b). Nanotechnology and its role in improvement of crop production for sustainable agriculture. Krishi Sci. 2, 22–24.
Chaudhary, P., Khati, P., Chaudhary, A., Gangola, S., Kumar, R., and Sharma, A. (2021c). Bioinoculation using indigenous Bacillus spp. improves growth and yield of Zea mays under the influence of nanozeolite. 3 Biotech. 11, 11. doi:10.1007/s13205-020-02561-2
Chavan, S., and Nadanathangam, V. (2019). Effects of nanoparticles on plant growth-promoting bacteria in Indian agricultural soil. Agron. (Basel). 9, 140. doi:10.3390/agronomy9030140
Chavan, S., Sarangdhar, V., and Nadanathangam, V. (2020). Toxicological effects of TiO2 nanoparticles on plant growth promoting soil bacteria. Emerg. Contam. 6, 87–92. doi:10.1016/j.emcon.2020.01.003
Cherian, T., Maity, D., Rajendra Kumar, R. T., Balasubramani, G., Ragavendran, C., Yalla, S., et al. (2022). Green chemistry based gold nanoparticles synthesis using the marine bacterium Lysinibacillus odysseyi PBCW2 and their multitudinous activities. Nanomaterials 12, 2940. doi:10.3390/nano12172940
Chibuike, G. U., and Obiora, S. C. (2014). Heavy metal polluted soils: Effect on plants and bioremediation methods. Appl. Environ. Soil Sci. 2014, 1–12. doi:10.1155/2014/752708
Chouhan, G. K., Verma, J. P., Jaiswal, D. K., Mukherjee, A., Singh, S., De Araujo Pereira, A. P., et al. (2021). Phytomicrobiome for promoting sustainable agriculture and food security: Opportunities, challenges, and solutions. Microbiol. Res. 248, 126763. doi:10.1016/j.micres.2021.126763
Damodaran, T., Sharma, D., Mishra, V., Jha, S., Kannan, R., Sah, V., et al. (2013). Isolation of salt tolerant endophyticand rhizospheric bacteria by natural selection and screening for promising plant growth promoting rhizobacteria [PGPR] and growth vigour in tomato under sodic soil. Afr. J. Microbiol. Res. 7, 5082–5089.
Das, P., Williams, C. J., Fulthorpe, R. R., Hoque, M. E., Metcalfe, C. D., and Xenopoulos, M. A. (2012). Changes in bacterial community structure after exposure to silver nanoparticles in natural waters. Environ. Sci. Technol. 46, 9120–9128. doi:10.1021/es3019918
Daudi, A., Cheng, Z., O’brien, J. A., Mammarella, N., Khan, S., Ausubel, F. M., et al. (2012). The apoplastic oxidative burst peroxidase in Arabidopsis is a major component of pattern-triggered immunity. Plant Cell 24, 275–287. doi:10.1105/tpc.111.093039
Davar, Z. F., Roozbahani, A., and Hosnamidi, A. (2014). Evaluation the effect of water stress and foliar application of Fe nanoparticles on yield, yield components and oil percentage of safflower (Carthamus tinctorious L.) Int. J. Adv. Biol. Biomed. Res. 2, 1150–1159.
Degenhardt, B., and Gimmler, H. (2000). Cell wall adaptations to multiple environmental stresses in maize roots. J. Exp. Bot. 51, 595–603. doi:10.1093/jexbot/51.344.595
Devi, L. S., and Joshi, S. (2015). Ultrastructures of silver nanoparticles biosynthesized using endophytic fungi. J. Microsc. Ultrastruct. 3, 29–37. doi:10.1016/j.jmau.2014.10.004
Dimkpa, C. O., Mclean, J. E., Britt, D. W., and Anderson, A. J. (2012). CuO and ZnO nanoparticles differently affect the secretion of fluorescent siderophores in the beneficial root colonizer, Pseudomonas chlororaphis O6. Nanotoxicology 6, 635–642. doi:10.3109/17435390.2011.598246
Dinesh, B., Monisha, N., Shalini, H. R., Prathap, G. K., Poyya, J., Shantaram, M., et al. (2022). Antibacterial activity of silver nanoparticles synthesized using endophytic fungus - Penicillium cinnamopurpureum. Spectrosc. Lett. 55, 20–34. doi:10.1080/00387010.2021.2010764
Ding, Y., Peng, N., Du, Y., Ji, L., and Cao, B. (2014). Disruption of putrescine biosynthesis in Shewanella oneidensis enhances biofilm cohesiveness and performance in Cr (VI) immobilization. Appl. Environ. Microbiol. 80, 1498–1506. doi:10.1128/aem.03461-13
Dutta, G., and Sugumaran, A. (2021). Bioengineered zinc oxide nanoparticles: Chemical, green, biological fabrication methods and its potential biomedical applications. J. Drug Deliv. Sci. Technol. 66, 102853. doi:10.1016/j.jddst.2021.102853
Egamberdieva, D. J. (2009). Alleviation of salt stress by plant growth regulators and IAA producing bacteria in wheat. Acta Physiol. Plant. 31, 861–864. doi:10.1007/s11738-009-0297-0
Eid, A. M., Fouda, A., Abdel-Rahman, M. A., Salem, S. S., Elsaied, A., Oelmüller, R., et al. (2021). Harnessing bacterial endophytes for promotion of plant growth and biotechnological applications: An overview. Plants (Basel). 10, 935. doi:10.3390/plants10050935
Eida, A. A., Alzubaidy, H. S., De Zélicourt, A., Synek, L., Alsharif, W., Lafi, F. F., et al. (2019). Phylogenetically diverse endophytic bacteria from desert plants induce transcriptional changes of tissue-specific ion transporters and salinity stress in Arabidopsis thaliana. Plant Sci. 280, 228–240. doi:10.1016/j.plantsci.2018.12.002
El-Gazzar, N., Almaary, K., Ismail, A., and Polizzi, G. (2020). Influence of Funneliformismosseae enhanced with titanium dioxide nanoparticles (TiO2NPs) on Phaseolus vulgaris L. under salinity stress. PLoS One 15, e0243491. doi:10.1371/journal.pone.0243491
Elemike, E. E., Uzoh, I. M., Onwudiwe, D. C., and Babalola, O. O. (2019). The role of nanotechnology in the fortification of plant nutrients and improvement of crop production. Appl. Sci. (Basel). 9, 499. doi:10.3390/app9030499
Elnahal, A. S. M., El-Saadony, M. T., Saad, A. M., Desoky, E.-S. M., El-Tahan, A. M., Rady, M. M., et al. (2022). The use of microbial inoculants for biological control, plant growth promotion, and sustainable agriculture: A review. Eur. J. Plant Pathol. 162, 759–792. doi:10.1007/s10658-021-02393-7
Elsheerya, N. I., Sunoja, V. S. J., Wena, Y., Zhua, J. J., Muralidharana, G., and Cao, K. F. (2020). Foliar application of nanoparticles mitigates the chilling effect on photosynthesis and photoprotection in sugarcane. Plant Physiol. biochem. 149, 50–60. doi:10.1016/j.plaphy.2020.01.035
Emamverdian, A., Ding, Y., Mokhberdoran, F., and Xie, Y. (2015). Heavy metal stress and some mechanisms of plant defense response. Sci. World J. 2015, 1–18. doi:10.1155/2015/756120
Fabrega, J., Zhang, R., Renshaw, J. C., Liu, W.-T., and Lead, J. R. (2011). Impact of silver nanoparticles on natural marine biofilm bacteria. Chemosphere 85, 961–966. doi:10.1016/j.chemosphere.2011.06.066
Fadiji, A. E., Mortimer, P. E., Xu, J., Ebenso, E. E., and Babalola, O. O. (2022a). Biosynthesis of nanoparticles using endophytes: A novel approach for enhancing plant growth and sustainable agriculture. Sustainability 14, 10839. doi:10.3390/su141710839
Fadiji, A. E., Onwudiwe, D. C., Mthiyane, D. M. N. B., and Oluranti, O. (2022b). Harnessing the known and unknown impact of nanotechnology on enhancing food security and reducing postharvest losses: Constraints and future prospects. Agron. (Basel). 12, 1657. doi:10.3390/agronomy12071657
Faiz, S., Yasin, N. A., Khan, W. U., Shah, A. A., Akram, W., Ahmad, A., et al. (2022). Role of magnesium oxide nanoparticles in the mitigation of lead-induced stress in daucus carota: Modulation in polyamines and antioxidant enzymes. Int. J. Phytoremediation 24, 364–372. doi:10.1080/15226514.2021.1949263
Fan, P., Chen, D., He, Y., Zhou, Q., Tian, Y., and Gao, L. J. I. J. O. P. (2016). Alleviating salt stress in tomato seedlings using Arthrobacter and Bacillus megaterium isolated from the rhizosphere of wild plants grown on saline–alkaline lands. Int. J. Phytoremediation 18, 1113–1121. doi:10.1080/15226514.2016.1183583
FAO (2009). Food Agriculture Organization of the United Nations. High level expert forum—how to feed the world in 2050. Italy: Office of the Director, Agricultural Development Economics Division Rome.
Fasusi, O. A., Amoo, A. E., and Babalola, O. O. (2021). Characterization of plant growth-promoting rhizobacterial isolates associated with food plants in South Africa. Antonie Leeuwenhoek 114, 1683–1708. doi:10.1007/s10482-021-01633-4
Flemming, H., and Wingender, J. (2010). The biofilm matrix. Nat. Rev. Microbiol. 8, 623–633. doi:10.1038/nrmicro2415
Franco, D., Calabrese, G., Guglielmino, S. P. P., and Conoci, S. (2022). Metal-based nanoparticles: Antibacterial mechanisms and biomedical application. Microorganisms 10, 1778. doi:10.3390/microorganisms10091778
Franken, P. (2012). The plant strengthening root endophyte Piriformospora indica: Potential application and the biology behind. Appl. Microbiol. Biotechnol. 96, 1455–1464. doi:10.1007/s00253-012-4506-1
Frenk, S., Ben-Moshe, T., Dror, I., Berkowitz, B., and Minz, D. (2013). Effect of metal oxide nanoparticles on microbial community structure and function in two different soil types. PloS One 8, e84441. doi:10.1371/journal.pone.0084441
Fu, P. P., Xia, Q., Hwang, H.-M., Ray, P. C., and Yu, H. (2014). Mechanisms of nanotoxicity: Generation of reactive oxygen species. J. Food Drug Anal. 22, 64–75. doi:10.1016/j.jfda.2014.01.005
Gamalero, E., and Glick, B. R. (2022). Recent advances in bacterial amelioration of plant drought and salt stress. Biology 11, 437. doi:10.3390/biology11030437
Gangadoo, S., Xu, C., Cozzolino, D., Latham, K., Della Gaspera, E., Chapman, J., et al. (2022). Probing nanoscale interactions of antimicrobial zinc oxide quantum dots on bacterial and fungal cell surfaces. Adv. Mat. Interfaces 9, 2101484. doi:10.1002/admi.202101484
Ganie, S. A., Bhat, J. A., and Devoto, A. (2021). The influence of endophytes on rice fitness under environmental stresses. Plant Mol. Biol. 109, 447–467. doi:10.1007/s11103-021-01219-8
Gao, X., Zou, C., Wang, L., and Zhang, F. (2006). Silicon decreases transpiration rate and conductance from stomata of maize plants. J. Plant Nutr. 29, 1637–1647. doi:10.1080/01904160600851494
Gepstein, S., and Glick, B. R. (2013). Strategies to ameliorate abiotic stress-induced plant senescence. Plant Mol. Biol. 82, 623–633. doi:10.1007/s11103-013-0038-z
Giraldo, J. P., Landry, M. P., Faltermeier, S. M., Mcnicholas, T. P., Iverson, N. M., Boghossian, A. A., et al. (2014). Plant nanobionics approach to augment photosynthesis and biochemical sensing. Nat. Mat. 13, 400–408. doi:10.1038/nmat3890
Glick, B. R. (2004). Bacterial ACC deaminase and the alleviation of plant stress. Adv. Appl. Microbiol. 56, 291–312. doi:10.1016/S0065-2164(04)56009-4
Glick, B. R. (2020). Beneficial plant-bacterial interactions. 2nd Edition. Heidelberg: Springer, 383.
Glick, B. R., and Gamalero, E. (2021). Recent developments in the study of plant microbiomes. Microorganisms 9, 1533. doi:10.3390/microorganisms9071533
Gopalakrishnan, S., Sathya, A., Vijayabharathi, R., Varshney, R. K., Gowda, C., and Krishnamurthy, L. (2015). Plant growth promoting rhizobia: Challenges and opportunities. 3 Biotech. 5, 355–377. doi:10.1007/s13205-014-0241-x
Gorai, P. S., Ghosh, R., Mandal, S., Ghosh, S., Chatterjee, S., Gond, S. K., et al. (2021). Bacillus siamensis CNE6- a multifaceted plant growth promoting endophyte of Cicer arietinum L. having broad spectrum antifungal activities and host colonizing potential. Microbiol. Res. 252, 126859. doi:10.1016/j.micres.2021.126859
Govindappa, M., Manasa, D., Vinaykiya, V., Dutta, S., Pawar, R., and Raghavendra, V. B. (2022). Screening of antibacterial and antioxidant activity of biogenically synthesized silver nanoparticles from alternaria alternata, endophytic fungus of Dendrophthoe falcata-a Parasitic Plant. Bionanoscience 12, 128–141. doi:10.1007/s12668-021-00932-4
Gunjan, B., and Zaidi, M. (2014). Impact of gold nanoparticles on physiological and biochemical characteristics of Brassica juncea. J. Plant Biochem. Physiol. 2, 1000133.
Gupta, S., Schillaci, M., Walker, R., Smith, P. M. C., Watt, M., and Roessner, U. (2021). Alleviation of salinity stress in plants by endophytic plant-fungal symbiosis: Current knowledge, perspectives and future directions. Plant Soil 461, 219–244. doi:10.1007/s11104-020-04618-w
Gürsoy, N., Yilmaz Öztürk, B., and Dağ, İ. (2021). Synthesis of intracellular and extracellular gold nanoparticles with a green machine and its antifungal activity. Turk. J. Biol. 45, 196–213. doi:10.3906/biy-2010-64
Haghighi, M., Abolghasemi, R., and Da Silva, J. a. T. (2014). Low and high temperature stress affect the growth characteristics of tomato in hydroponic culture with Se and nano-Se amendment. Sci. Hortic. 178, 231–240. doi:10.1016/j.scienta.2014.09.006
Halliwell, B., and Gutteridge, J. M. (2015). Free radicals in biology and medicine. USA: Oxford University Press.
Hanaka, A., Ozimek, E., Reszczyńska, E., Jaroszuk-Ściseł, J., and Stolarz, M. (2021). Plant tolerance to drought stress in the presence of supporting bacteria and fungi: An efficient strategy in horticulture. Horticulturae 7, 390. doi:10.3390/horticulturae7100390
Hasanpour, H., Maali-Amir, R., and Zeinali, H. (2015). Effect of TiO2 nanoparticles on metabolic limitations to photosynthesis under cold in chickpea. Russ. J. Plant Physiol. 62, 779–787. doi:10.1134/s1021443715060096
Hasanuzzaman, M., Bhuyan, M., Anee, T. I., Parvin, K., Nahar, K., Mahmud, J. A., et al. (2019). Regulation of ascorbate-glutathione pathway in mitigating oxidative damage in plants under abiotic stress. Antioxidants 8, 384. doi:10.3390/antiox8090384
Hasanuzzaman, M., Nahar, K., and Fujita, M. (2013). “Extreme temperature responses, oxidative stress and antioxidant defense in plants,” in Abiotic stress-plant responses and applications in agriculture. Editors K. Vahdat, and C. Leslie (Rijeka: InTech), 169–205. doi:10.5772/54833
Hasanuzzaman, M., Nahar, K., and Fujita, M. (2014). “Silicon and selenium: Two vital trace elements that confer abiotic stress tolerance to plants,” in Emerging technologies and management of crop stress tolerance (Elsevier), 377–422.
Hattori, T., Inanaga, S., Araki, H., An, P., Morita, S., Luxová, M., et al. (2005). Application of silicon enhanced drought tolerance in Sorghum bicolor. Physiol. Plant. 123, 459–466. doi:10.1111/j.1399-3054.2005.00481.x
Hayat, R., Ali, S., Amara, U., Khalid, R., and Ahmed, I. (2010). Soil beneficial bacteria and their role in plant growth promotion: A review. Ann. Microbiol. 60, 579–598. doi:10.1007/s13213-010-0117-1
He, C., Zeng, Q., Chen, Y., Chen, C., Wang, W., Hou, J., et al. (2021). Colonization by dark septate endophytes improves the growth and rhizosphere soil microbiome of licorice plants under different water treatments. Appl. Soil Ecol. 166, 103993. doi:10.1016/j.apsoil.2021.103993
He, L., Liu, Y., Mustapha, A., and Lin, M. (2011). Antifungal activity of zinc oxide nanoparticles against Botrytis cinerea and Penicillium expansum. Microbiol. Res. 166, 207–215. doi:10.1016/j.micres.2010.03.003
Hmaeid, N., Wali, M., Mahmoud, O. M.-B., Pueyo, J. J., Ghnaya, T., and Abdelly, C. (2019). Efficient rhizobacteria promote growth and alleviate NaCl-induced stress in the plant species Sulla carnosa. Appl. Soil Ecol. 133, 104–113. doi:10.1016/j.apsoil.2018.09.011
Hulikere, M. M., and Joshi, C. G. (2019). Characterization, antioxidant and antimicrobial activity of silver nanoparticles synthesized using marine endophytic fungus-Cladosporium cladosporioides. Process Biochem. 82, 199–204. doi:10.1016/j.procbio.2019.04.011
Hwang, H. H., Chien, P. R., Huang, F. C., Hung, S. H., Kuo, C. H., Deng, W. L., et al. (2021). A plant endophytic bacterium, Burkholderia seminalis strain 869T2, promotes plant growth in Arabidopsis, Pak Choi, Chinese Amaranth, Lettuces, and other vegetables. Microorganisms 9, 1703. doi:10.3390/microorganisms9081703
Ibrahim, E., Zhang, M., Zhang, Y., Hossain, A., Qiu, W., Chen, Y., et al. (2020). Green-synthesization of silver nanoparticles using endophytic bacteria isolated from garlic and its antifungal activity against Wheat Fusarium head blight pathogen Fusarium graminearum. Nanomaterials 10, 219. doi:10.3390/nano10020219
Igiehon, N. O., Babalola, O. O., Cheseto, X., and Torto, B. (2021). Effects of rhizobia and arbuscular mycorrhizal fungi on yield, size distribution and fatty acid of soybean seeds grown under drought stress. Microbiol. Res. 242, 126640. doi:10.1016/j.micres.2020.126640
Igiehon, O. N., and Babalola, O. O. (2021). Rhizobium and mycorrhizal fungal species improved soybean yield under drought stress conditions. Curr. Microbiol. 78, 1615–1627. doi:10.1007/s00284-021-02432-w
Imade, E. E., Ajiboye, T. O., Fadiji, A. E., Onwudiwe, D. C., and Babalola, O. O. (2022). Green synthesis of zinc oxide nanoparticles using plantain peel extracts and the evaluation of their antibacterial activity. Sci. Afr. 16, e01152. doi:10.1016/j.sciaf.2022.e01152
Iravani, S., Korbekandi, H., Mirmohammadi, S. V., and Zolfaghari, B. (2014). Synthesis of silver nanoparticles: Chemical, physical and biological methods. Res. Pharm. Sci. 9, 385–406.
Ishida, K., Cipriano, T. F., Rocha, G. M., Weissmüller, G., Gomes, F., Miranda, K., et al. (2013). Silver nanoparticle production by the fungus Fusarium oxysporum: Nanoparticle characterisation and analysis of antifungal activity against pathogenic yeasts. Mem. Inst. Oswaldo Cruz 109, 220–228. doi:10.1590/0074-0276130269
Jaberzadeh, A., Moaveni, P., Moghadam, H. R. T., and Zahedi, H. (2013). Influence of bulk and nanoparticles titanium foliar application on some agronomic traits, seed gluten and starch contents of wheat subjected to water deficit stress. Not. Bot. Horti Agrobot. Cluj. Napoca. 41, 201–207. doi:10.15835/nbha4119093
Jacoby, R., Peukert, M., Succurro, A., Koprivova, A., and Kopriva, S. (2017). The role of soil microorganisms in plant mineral nutrition—Current knowledge and future directions. Front. Plant Sci. 8, 1617. doi:10.3389/fpls.2017.01617
Jadoun, S., Chauhan, N. P. S., Zarrintaj, P., Barani, M., Varma, R. S., Chinnam, S., et al. (2022). Synthesis of nanoparticles using microorganisms and their applications: A review. Environ. Chem. Lett. 20, 3153–3197. doi:10.1007/s10311-022-01444-7
Jajoo, A., and Mathur, S. (2021). Role of arbuscular mycorrhizal fungi as an underground saviuor for protecting plants from abiotic stresses. Physiol. Mol. Biol. Plants 27, 2589–2603. doi:10.1007/s12298-021-01091-2
Jalil, S. U., and Ansari, M. I. (2019). Nanoparticles and abiotic stress tolerance in plants: Synthesis, action, and signaling mechanisms. Plant Signal. Mol. 2019, 549–561.
Jha, Y., and Subramanian, R. B. (2014). PGPR regulate caspase-like activity, programmed cell death, and antioxidant enzyme activity in paddy under salinity. Physiol. Mol. Biol. Plants 20, 201–207. doi:10.1007/s12298-014-0224-8
Jin, L., Son, Y., Deforest, J. L., Kang, Y. J., Kim, W., and Chung, H. (2014). Single-walled carbon nanotubes alter soil microbial community composition. Sci. Total Environ. 466, 533–538. doi:10.1016/j.scitotenv.2013.07.035
Kalteh, M., Alipour, Z. T., Ashraf, S., Marashi Aliabadi, M., and Falah Nosratabadi, A. (2018). Effect of silica nanoparticles on basil (Ocimum basilicum) under salinity stress. J. Chem. Health Risks. 4, 49–55.
Kang, S., Herzberg, M., Rodrigues, D. F., and Elimelech, M. (2008). Antibacterial effects of carbon nanotubes: Size does matter. Langmuir 24, 6409–6413. doi:10.1021/la800951v
Karuppanapandian, T., Wang, H. W., Prabakaran, N., Jeyalakshmi, K., Kwon, M., Manoharan, K., et al. (2011). 2, 4-dichlorophenoxyacetic acid-induced leaf senescence in mung bean (Vigna radiata L. Wilczek) and senescence inhibition by co-treatment with silver nanoparticles. Plant Physiol. biochem. 49, 168–177. doi:10.1016/j.plaphy.2010.11.007
Kaur, T., Bala, M., Kumar, G., and Vyas, A. (2022). Biosynthesis of zinc oxide nanoparticles via endophyte Trichoderma viride and evaluation of their antimicrobial and antioxidant properties. Arch. Microbiol. 204, 620. doi:10.1007/s00203-022-03218-9
Kaushal, M., and Wani, S. P. (2016). Plant-growth-promoting rhizobacteria: Drought stress alleviators to ameliorate crop production in drylands. Ann. Microbiol. 66, 35–42. doi:10.1007/s13213-015-1112-3
Khalid, M., Bilal, M., Hassani, D., Iqbal, H., Wang, H., and Huang, D. J. B. S. (2017). Mitigation of salt stress in white clover (Trifolium repens) by Azospirillum brasilense and its inoculation effect. Bot. Stud. 58, 5. doi:10.1186/s40529-016-0160-8
Khan, A., Zhao, X. Q., Javed, M. T., Khan, K. S., Bano, A., Shen, R. F., et al. (2016). Bacillus pumilus enhances tolerance in rice (Oryza sativa L.) to combined stresses of NaCl and high boron due to limited uptake of Na+. Environ. Exp. Bot. 124, 120–129. doi:10.1016/j.envexpbot.2015.12.011
Khati, P., Pankaj, P., Nisha, B., Kumar, R., and Sharma, A. (2018). Effect of nanozeolite and plant growth promoting rhizobacteria on maize. 3 Biotech. 8, 141. doi:10.1007/s13205-018-1142-1
Khatoon, U. T., Rao, G. N., Mohan, M. K., Ramanaviciene, A., and Ramanavicius, A. (2018). Comparative study of antifungal activity of silver and gold nanoparticles synthesized by facile chemical approach. J. Environ. Chem. Eng. 6, 5837–5844. doi:10.1016/j.jece.2018.08.009
Kibbey, T. C., and Strevett, K. A. (2019). The effect of nanoparticles on soil and rhizosphere bacteria and plant growth in lettuce seedlings. Chemosphere 221, 703–707. doi:10.1016/j.chemosphere.2019.01.091
Kim, K.-J., Sung, W. S., Suh, B. K., Moon, S.-K., Choi, J.-S., Kim, J. G., et al. (2009). Antifungal activity and mode of action of silver nano-particles on Candida albicans. Biometals 22, 235–242. doi:10.1007/s10534-008-9159-2
Kohan-Baghkheirati, E., and Geisler-Lee, J. (2015). Gene expression, protein function and pathways of Arabidopsis thaliana responding to silver nanoparticles in comparison to silver ions, cold, salt, drought, and heat. Nanomaterials 5, 436–467. doi:10.3390/nano5020436
Komaresofla, B. R., Alikhani, H. A., Etesami, H., and Khoshkholgh-Sima, N. A. (2019). Improved growth and salinity tolerance of the halophyte Salicornia sp. by co–inoculation with endophytic and rhizosphere bacteria. Appl. Soil Ecol. 138, 160–170. doi:10.1016/j.apsoil.2019.02.022
Koné, Y., Alves, E., Silveira, P. R., Ferreira, A. N., De Medeiros, F. H. V., and Cruz-Magalhães, V. (2022). Control of blast disease caused by Pyricularia oryzae with Epicoccum nigrum and microscopic studies of their interaction with rice plants under greenhouse conditions. Biol. Control 167, 104840. doi:10.1016/j.biocontrol.2022.104840
Kumar, A., Patel, J. S., Meena, V. S., and Srivastava, R. (2019). Recent advances of PGPR based approaches for stress tolerance in plants for sustainable agriculture. Biocatal. Agric. Biotechnol. 20, 101271. doi:10.1016/j.bcab.2019.101271
Kumar, R., Sahoo, S., Joanni, E., Singh, R. K., Tan, W. K., Kar, K. K., et al. (2021). Recent progress on carbon-based composite materials for microwave electromagnetic interference shielding. Carbon 177, 304–331. doi:10.1016/j.carbon.2021.02.091
Kumar, V., and Nautiyal, C. S. (2022). Plant abiotic and biotic stress alleviation: From an endophytic microbial perspective. Curr. Microbiol. 79, 311. doi:10.1007/s00284-022-03012-2
Kumari, S., Sharma, A., Chaudhary, P., and Khati, P. (2020). Management of plant vigor and soil health using two agriusable nanocompounds and plant growth promotory rhizobacteria in Fenugreek. 3 Biotech. 10, 461. doi:10.1007/s13205-020-02448-2
Kushwaha, P., Kashyap, P. L., Bhardwaj, A. K., Kuppusamy, P., Srivastava, A. K., and Tiwari, R. K. (2020). Bacterial endophyte mediated plant tolerance to salinity: Growth responses and mechanisms of action. World J. Microbiol. Biotechnol. 36, 26. doi:10.1007/s11274-020-2804-9
Larue, C., Baratange, C., Vantelon, D., Khodja, H., Surblé, S., Elger, A., et al. (2018). Influence of soil type on TiO2 nanoparticle fate in an agro-ecosystem. Sci. Total Environ. 630, 609–617. doi:10.1016/j.scitotenv.2018.02.264
Laware, S., and Raskar, S. (2014). Effect of titanium dioxide nanoparticles on hydrolytic and antioxidant enzymes during seed germination in onion. Int. J. Curr. Microbiol. App. Sci. 3, 749–760.
Lee, S., Kim, K., Shon, H., Kim, S. D., and Cho, J. (2011). Biotoxicity of nanoparticles: Effect of natural organic matter. J. Nanopart. Res. 13, 3051–3061. doi:10.1007/s11051-010-0204-z
Lee, Y., Krishnamoorthy, R., Selvakumar, G., Kim, K., and Sa, T. (2015). Alleviation of salt stress in maize plant by co-inoculation of arbuscular mycorrhizal fungi and Methylobacterium oryzae CBMB20. J. Korean Soc. Appl. Biol. Chem. 58, 533–540. doi:10.1007/s13765-015-0072-4
Li, D., Cui, F., Zhao, Z., Liu, D., Xu, Y., Li, H., et al. (2014). The impact of titanium dioxide nanoparticles on biological nitrogen removal from wastewater and bacterial community shifts in activated sludge. Biodegradation 25, 167–177. doi:10.1007/s10532-013-9648-z
Li, J., Zhang, P., Ye, J., Zhang, G., and Cai, Y. (2019). Simultaneous in-situ remediation and fertilization of Cd-contaminated weak-alkaline farmland for wheat production. J. Environ. Manage. 250, 109528. doi:10.1016/j.jenvman.2019.109528
Li, Z., and Huang, J. (2014). Effects of nanoparticle hydroxyapatite on growth and antioxidant system in pakchoi (Brassica chinensis L.) from cadmium-contaminated soil. J. Nanomater. 2014, 470962.
Liang, Z., Das, A., and Hu, Z. (2010). Bacterial response to a shock load of nanosilver in an activated sludge treatment system. Water Res. 44, 5432–5438. doi:10.1016/j.watres.2010.06.060
Liu, B., Mu, L., Zhang, J., Han, X., and Shi, H. (2020). TiO2/Cu2 (OH)2 CO3 nanocomposite as efficient antimicrobials for inactivation of crop pathogens in agriculture. Mater. Sci. Eng. C 107, 110344. doi:10.1016/j.msec.2019.110344
Liu, H. Q., Lu, X. B., Li, Z. H., Tian, C. Y., and Song, J. (2021). The role of root-associated microbes in growth stimulation of plants under saline conditions. Land Degrad. Dev. 32, 3471–3486. doi:10.1002/ldr.3955
Liu, X., Jin, X., Cao, B., and Tang, C. Y. (2014). Bactericidal activity of silver nanoparticles in environmentally relevant freshwater matrices: Influences of organic matter and chelating agent. J. Environ. Chem. Eng. 2, 525–531. doi:10.1016/j.jece.2013.10.008
Lowry, G. V., Gregory, K. B., Apte, S. C., and Lead, J. R. (2012). Transformations of nanomaterials in the environment. Environ. Sci. Technol. 7, 6893–6899.
Lubna, , Khan, M. A., Asaf, S., Jan, R., Waqas, M., Kim, K.-M., et al. (2022). Endophytic fungus Bipolaris sp. CSL-1 induces salt tolerance in Glycine max. L via modulating its endogenous hormones, antioxidative system and gene expression. J. Plant Interact. 17, 319–332. doi:10.1080/17429145.2022.2036836
Lyu, D., Msimbira, L. A., Nazari, M., Antar, M., Pagé, A., Shah, A., et al. (2021). The coevolution of plants and microbes underpins sustainable agriculture. Microorganisms 9, 1036. doi:10.3390/microorganisms9051036
Mahmood, A., Amaya, R., Turgay, O. C., Yaprak, A. E., Taniguchi, T., and Kataoka, R. (2019). High salt tolerant plant growth promoting rhizobacteria from the common ice-plant Mesembryanthemum crystallinum L. Rhizosphere 9, 10–17. doi:10.1016/j.rhisph.2018.10.004
Mahmood, S., Daur, I., Al-Solaimani, S. G., Ahmad, S., Madkour, M. H., Yasir, M., et al. (2016). Plant growth promoting rhizobacteria and silicon synergistically enhance salinity tolerance of mung bean. Front. Plant Sci. 7, 876. doi:10.3389/fpls.2016.00876
Mallmann, E. J. J., Cunha, F. A., Castro, B. N., Maciel, A. M., Menezes, E. A., and Fechine, P. B. A. (2015). Antifungal activity of silver nanoparticles obtained by green synthesis. Rev. Inst. Med. Trop. S. Paulo 57, 165–167. doi:10.1590/s0036-46652015000200011
Martínez-Ballesta, M., Zapata, L., Chalbi, N., and Carvajal, M. (2016). Multiwalled carbon nanotubes enter broccoli cells enhancing growth and water uptake of plants exposed to salinity. J. Nanobiotechnol. 14, 42–14. doi:10.1186/s12951-016-0199-4
Masoumizadeh, M., Madani, M., Fatahian, S., and Shakib, P. (2022). Effect of silver nanoparticles (AgNPs) on Candida albicans, Candida dubliniensis and Candida guilliermondii. Curr. Drug Ther. 17, 50–55. doi:10.2174/1574885517666220221093456
Masrahi, A., Vandevoort, A. R., and Arai, Y. (2014). Effects of silver nanoparticle on soil-nitrification processes. Arch. Environ. Contam. Toxicol. 66, 504–513. doi:10.1007/s00244-013-9994-1
Massad, T. J., Dyer, L. A., and Vega C, G. (2012). Costs of defense and a test of the carbon-nutrient balance and growth-differentiation balance hypotheses for two co-occurring classes of plant defense. PLoS One 7, e47554. doi:10.1371/journal.pone.0047554
Mathur, V., and Ulanova, D. (2022). Microbial metabolites beneficial to plant hosts across ecosystems. Microb. Ecol. doi:10.1007/s00248-022-02073-x
Mazmancı, B., Könen Adıgüzel, S., Sadak, Y. S., Yetkin, D., Ay, H., and Adıgüzel, A. O. (2022). Antimicrobial, antibiofilm, and anticancer potential of silver nanoparticles synthesized using pigment-producing Micromonospora sp. SH121. Prep. Biochem. Biotechnol., 1–13. doi:10.1080/10826068.2022.2101001
Mcquillan, J. (2010). Bacterial nanoparticle interactions. PhD thesis. Exeter: University of Exeter, 211.
Mcquillan, J. S., and Shaw, A. M. (2014). Differential gene regulation in the Ag nanoparticle and Ag+-induced silver stress response in Escherichia coli: A full transcriptomic profile. Nanotoxicology 8, 177–184. doi:10.3109/17435390.2013.870243
Meena, K. K., Kumar, M., Kalyuzhnaya, M. G., Yandigeri, M. S., Singh, D. P., Saxena, A. K., et al. (2012). Epiphytic pink-pigmented methylotrophic bacteria enhance germination and seedling growth of wheat (Triticum aestivum) by producing phytohormone. Ant. Van Leeuwenhoek 101, 777–786. doi:10.1007/s10482-011-9692-9
Meena, K. K., Sorty, A. M., Bitla, U. M., Choudhary, K., Gupta, P., Pareek, A., et al. (2017). Abiotic stress responses and microbe-mediated mitigation in plants: The omics strategies. Front. Plant Sci. 8, 172. doi:10.3389/fpls.2017.00172
Mickelbart, M. V., Hasegawa, P. M., and Bailey-Serres, J. (2015). Genetic mechanisms of abiotic stress tolerance that translate to crop yield stability. Nat. Rev. Genet. 16, 237–251. doi:10.1038/nrg3901
Mishra, R. C., Kalra, R., Dilawari, R., Goel, M., and Barrow, C. J. (2022). Bio-synthesis of Aspergillus terreus mediated gold nanoparticle: Antimicrobial, antioxidant, antifungal and in vitro cytotoxicity studies. Materials 15, 3877. doi:10.3390/ma15113877
Mittal, D., Kumar, A., Balasubramaniam, B., Thakur, R., Singh Siwal, S., Saini, V., et al. (2021). Synthesis of Biogenic silver nanoparticles using plant growth-promoting bacteria: Potential use as biocontrol agent against phytopathogens. Biomat. Polym. Horiz. 1, 22–31. doi:10.37819/bph.001.01.0130
Mohammadi, R., Maali-Amiri, R., and Abbasi, A. (2013). Effect of TiO2 nanoparticles on chickpea response to cold stress. Biol. Trace Elem. Res. 152, 403–410. doi:10.1007/s12011-013-9631-x
Mohanty, A., Wu, Y., and Cao, B. (2014). Impacts of engineered nanomaterials on microbial community structure and function in natural and engineered ecosystems. Appl. Microbiol. Biotechnol. 98, 8457–8468. doi:10.1007/s00253-014-6000-4
Moreira, H., Pereira, S. I., Marques, A. P., Rangel, A. O., Castro, P. M. J. E. S., and Research, P. (2016). Mine land valorization through energy maize production enhanced by the application of plant growth-promoting rhizobacteria and arbuscular mycorrhizal fungi. Environ. Sci. Pollut. Res. 23, 6940–6950. doi:10.1007/s11356-015-5914-4
Moreno-Galván, A. E., Cortés-Patiño, S., Romero-Perdomo, F., Uribe-Vélez, D., Bashan, Y., and Bonilla, R. R. (2020). Proline accumulation and glutathione reductase activity induced by drought-tolerant rhizobacteria as potential mechanisms to alleviate drought stress in Guinea grass. Appl. Soil Ecol. 147, 103367. doi:10.1016/j.apsoil.2019.103367
Mostafa, E. M., Abdelgawad, M. A., Musa, A., Alotaibi, N. H., Elkomy, M. H., Ghoneim, M. M., et al. (2022). Chitosan silver and gold nanoparticle formation using endophytic fungi as powerful antimicrobial and anti-biofilm potentialities. Antibiotics 11, 668. doi:10.3390/antibiotics11050668
Mowafy, A. M., Fawzy, M. M., Gebreil, A., and Elsayed, A. (2021). Endophytic Bacillus, Enterobacter, and Klebsiella enhance the growth and yield of maize. Acta Agric. Scand. Sect. B —. Soil & Plant Sci. 71, 237–246. doi:10.1080/09064710.2021.1880621
Mueller, C. F., Laude, K., Mcnally, J. S., and Harrison, D. G. (2005). Redox mechanisms in blood vessels. Arterioscler. Thromb. Vasc. Biol. 25, 274–278. doi:10.1161/01.atv.0000149143.04821.eb
Mukhtar, S., Mirza, B. S., Mehnaz, S., Mirza, M. S., Mclean, J., and Malik, K. A. (2018). Impact of soil salinity on the microbial structure of halophyte rhizosphere microbiome. World J. Microbiol. Biotechnol. 34, 136. doi:10.1007/s11274-018-2509-5
Munns, R., and Tester, M. (2008). Mechanisms of salinity tolerance. Annu. Rev. Plant Biol. 59, 651–681. doi:10.1146/annurev.arplant.59.032607.092911
Nadeem, S. M., Zahir, Z. A., Naveed, M., and Nawaz, S. J. a. O. M. (2013). Mitigation of salinity-induced negative impact on the growth and yield of wheat by plant growth-promoting rhizobacteria in naturally saline conditions. Ann. Microbiol. 63, 225–232. doi:10.1007/s13213-012-0465-0
Nagy, A., Harrison, A., Sabbani, S., Munson, R. S., Dutta, P. K., and Waldman, W. J. (2011). Silver nanoparticles embedded in zeolite membranes: Release of silver ions and mechanism of antibacterial action. Int. J. Nanomedicine 6, 1833–1852. doi:10.2147/ijn.s24019
Nasr, F., Pateiro, M., Rabiei, V., Razavi, F., Formaneck, S., Gohari, G., et al. (2021). Chitosan-phenylalanine nanoparticles (Cs-Phe Nps) extend the postharvest life of Persimmon (Diospyros kaki) fruits under chilling stress. Coatings 11, 819. doi:10.3390/coatings11070819
Nasrollahi, A., Pourshamsian, K., and Mansourkiaee, P. (2011). Antifungal activity of silver nanoparticles on some of fungi. Int. J. Nano Dimens. 1, 233–239.
Nautiyal, C. S., Srivastava, S., Chauhan, P. S., Seem, K., Mishra, A., and Sopory, S. K. (2013). Plant growth-promoting bacteria Bacillus amyloliquefaciens NBRISN13 modulates gene expression profile of leaf and rhizosphere community in rice during salt stress. Plant Physiol. biochem. 66, 1–9. doi:10.1016/j.plaphy.2013.01.020
Naveed, M., Hussain, M. B., Zahir, Z. A., Mitter, B., and Sessitsch, A. (2014a). Drought stress amelioration in wheat through inoculation with Burkholderia phytofirmans strain PsJN. Plant Growth Regul. 73, 121–131. doi:10.1007/s10725-013-9874-8
Naveed, M., Mitter, B., Reichenauer, T. G., Wieczorek, K., and Sessitsch, A. (2014b). Increased drought stress resilience of maize through endophytic colonization by Burkholderia phytofirmans PsJN and Enterobacter sp. FD17. Environ. Exp. Bot. 97, 30–39. doi:10.1016/j.envexpbot.2013.09.014
Ndeddy Aka, R. J., and Babalola, O. O. (2016). Effect of bacterial inoculation of strains of Pseudomonas aeruginosa, Alcaligenes feacalis and Bacillus subtilis on germination, growth and heavy metal (Cd, Cr, and Ni) uptake of Brassica juncea. Int. J. Phytoremediation 18, 200–209. doi:10.1080/15226514.2015.1073671
Neethu, S., Midhun, S. J., Sunil, M., Soumya, S., Radhakrishnan, E., and Jyothis, M. (2018). Efficient visible light induced synthesis of silver nanoparticles by Penicillium polonicum ARA 10 isolated from Chetomorpha antennina and its antibacterial efficacy against Salmonella enterica serovar Typhimurium. J. Photochem. Photobiol. B Biol. 180, 175–185. doi:10.1016/j.jphotobiol.2018.02.005
Noukelag, S. K., Mewa-Ngongang, M., Ngqoloda, S., Kotsedi, L., Razanamahandry, L. C., Ntwampe, S. K. O., et al. (2022). Influence of synthesis method on structural, morphological, magnetic, and antimicrobial properties of Fe-Ag nanoparticles. J. Inorg. Organomet. Polym. Mat. doi:10.1007/s10904-022-02493-9
Nyberg, L., Turco, R. F., and Nies, L. (2008). Assessing the impact of nanomaterials on anaerobic microbial communities. Environ. Sci. Technol. 42, 1938–1943. doi:10.1021/es072018g
Oh, M.-M., Trick, H. N., and Rajashekar, C. (2009). Secondary metabolism and antioxidants are involved in environmental adaptation and stress tolerance in lettuce. J. Plant Physiol. 166, 180–191. doi:10.1016/j.jplph.2008.04.015
Ojuederie, O. B., Olanrewaju, O. S., and Babalola, O. O. (2019). Plant growth promoting rhizobacterial mitigation of drought stress in crop plants: Implications for sustainable agriculture. Agron. (Basel). 9, 712. doi:10.3390/agronomy9110712
Oleńska, E., Małek, W., Wójcik, M., Swiecicka, I., Thijs, S., and Vangronsveld, J. (2020). Beneficial features of plant growth-promoting rhizobacteria for improving plant growth and health in challenging conditions: A methodical review. Sci. Total Environ. 743, 140682. doi:10.1016/j.scitotenv.2020.140682
Omar, M., Osman, M., Kasim, W., and El-Daim, A. (2009). “Improvement of salt tolerance mechanisms of barley cultivated under salt stress using Azospirillum brasilense,” in Salinity and water stress (Springer), 133–147.
Omomowo, O. I., and Babalola, O. O. (2019). Bacterial and fungal endophytes: Tiny giants with immense beneficial potential for plant growth and sustainable agricultural productivity. Microorganisms 7, 481. doi:10.3390/microorganisms7110481
Ortiz, N., Armada, E., Duque, E., Roldán, A., and Azcón, R. (2015). Contribution of arbuscular mycorrhizal fungi and/or bacteria to enhancing plant drought tolerance under natural soil conditions: Effectiveness of autochthonous or allochthonous strains. J. Plant Physiol. 174, 87–96. doi:10.1016/j.jplph.2014.08.019
Ovais, M., Khalil, A. T., Islam, N. U., Ahmad, I., Ayaz, M., Saravanan, M., et al. (2018). Role of plant phytochemicals and microbial enzymes in biosynthesis of metallic nanoparticles. Appl. Microbiol. Biotechnol. 102, 6799–6814. doi:10.1007/s00253-018-9146-7
Palaniyandi, S., Damodharan, K., Yang, S., and Suh, J. (2014). Streptomyces sp. strain PGPA39 alleviates salt stress and promotes growth of ‘Micro Tom’tomato plants. J. Appl. Microbiol. 117, 766–773. doi:10.1111/jam.12563
Pandey, G. (2018). Challenges and future prospects of agri-nanotechnology for sustainable agriculture in India. Environ. Technol. Innov. 11, 299–307. doi:10.1016/j.eti.2018.06.012
Pandey, V., Ansari, M. W., Tula, S., Yadav, S., Sahoo, R. K., Shukla, N., et al. (2016). Dose-dependent response of Trichoderma harzianum in improving drought tolerance in rice genotypes. Planta 243, 1251–1264. doi:10.1007/s00425-016-2482-x
Panichikkal, J., and Krishnankutty, R. E. (2022). Chitosan and gold nanoparticles supplementation for augmentation of indole-3-acetic acid production by rhizospheric Pseudomonas aeruginosa and plant growth enhancement. Curr. Microbiol. 79, 185. doi:10.1007/s00284-022-02850-4
Panigrahi, S., Mohanty, S., and Rath, C. C. (2020). Characterization of endophytic bacteria Enterobacter cloacae MG00145 isolated from Ocimum sanctum with indole acetic acid (IAA) production and plant growth promoting capabilities against selected crops. S. Afr. J. Bot. 134, 17–26. doi:10.1016/j.sajb.2019.09.017
Parida, A., and Das, A. (2005). Salt tolerance and salinity effects on plants: A review. Ecotoxicol. Environ. Saf. 60, 324–349. doi:10.1016/j.ecoenv.2004.06.010
Park, H.-J., Kim, J. Y., Kim, J., Lee, J.-H., Hahn, J.-S., Gu, M. B., et al. (2009). Silver-ion-mediated reactive oxygen species generation affecting bactericidal activity. Water Res. 43, 1027–1032. doi:10.1016/j.watres.2008.12.002
Pasala, R. K., Khan, M. I. R., Minhas, P. S., Sultana, R., Per, T., Deokate, P., et al. (2016). Can plant bioregulators minimize the crop productivity losses caused by drought, heat stress and salinity? An integrated review. J. Appl. Bot. Food. 89, 113–125.
Pawlett, M., Ritz, K., Dorey, R. A., Rocks, S., Ramsden, J., and Harris, J. A. (2013). The impact of zero-valent iron nanoparticles upon soil microbial communities is context dependent. Environ. Sci. Pollut. Res. 20, 1041–1049. doi:10.1007/s11356-012-1196-2
Pei, Z., Ming, D., Liu, D., Wan, G., Geng, X., Gong, H., et al. (2010). Silicon improves the tolerance to water-deficit stress induced by polyethylene glycol in wheat (Triticum aestivum L.) seedlings. J. Plant Growth Regul. 29, 106–115. doi:10.1007/s00344-009-9120-9
Pelletier, D. A., Suresh, A. K., Holton, G. A., Mckeown, C. K., Wang, W., Gu, B., et al. (2010). Effects of engineered cerium oxide nanoparticles on bacterial growth and viability. Appl. Environ. Microbiol. 76, 7981–7989. doi:10.1128/aem.00650-10
Petruccelli, R., Bartolini, G., Ganino, T., Zelasco, S., Lombardo, L., Perri, E., et al. (2022). Cold stress, freezing adaptation, varietal susceptibility of Olea europaea L.: A review. Plants (Basel). 11, 1367. doi:10.3390/plants11101367
Pinedo, I., Ledger, T., Greve, M., and Poupin, M. J. (2015). Burkholderia phytofirmans PsJN induces long-term metabolic and transcriptional changes involved in Arabidopsis thaliana salt tolerance. Front. Plant Sci. 6, 466. doi:10.3389/fpls.2015.00466
Prasad, P., Pisipati, S., Momčilović, I., and Ristic, Z. (2011). Independent and combined effects of high temperature and drought stress during grain filling on plant yield and chloroplast EF-Tu expression in spring wheat. J. Agron. Crop Sci. 197, 430–441. doi:10.1111/j.1439-037x.2011.00477.x
Premachandra, D., Hudek, L., Enez, A., Ballard, R., Barnett, S., Franco, C. M., et al. (2020). Assessment of the capacity of beneficial bacterial inoculants to enhance canola (Brassica napus L.) growth under low water activity. Agron. (Basel). 10, 1449. doi:10.3390/agronomy10091449
Prucek, R., Tuček, J., Kilianová, M., Panáček, A., Kvítek, L., Filip, J., et al. (2011). The targeted antibacterial and antifungal properties of magnetic nanocomposite of iron oxide and silver nanoparticles. Biomaterials 32, 4704–4713. doi:10.1016/j.biomaterials.2011.03.039
Qian, D., Liu, H., Hu, F., Song, S., and Chen, Y. (2022). Extracellular electron transfer-dependent Cr(VI)/sulfate reduction mediated by iron sulfide nanoparticles. J. Biosci. Bioeng. 134, 153–161. doi:10.1016/j.jbiosc.2022.05.005
Qian, Y., Yu, H., He, D., Yang, H., Wang, W., Wan, X., et al. (2013). Biosynthesis of silver nanoparticles by the endophytic fungus Epicoccum nigrum and their activity against pathogenic fungi. Bioprocess Biosyst. Eng. 36, 1613–1619. doi:10.1007/s00449-013-0937-z
Radzig, M., Nadtochenko, V., Koksharova, O., Kiwi, J., Lipasova, V., and Khmel, I. (2013). Antibacterial effects of silver nanoparticles on gram-negative bacteria: Influence on the growth and biofilms formation, mechanisms of action. Colloids Surfaces B Biointerfaces 102, 300–306. doi:10.1016/j.colsurfb.2012.07.039
Rahi, D. K., and Parmar, A. S. (2014). Mycosynthesis of silver nanoparticles by an endophytic Penicillium species of Aloe vera root, evaluation of their antibacterial and antibiotic enhancing activity. Int. J. Nanomater. Biostructures. 6, 160–166.
Rahman, S., Rahman, L., Khalil, A. T., Ali, N., Zia, D., Ali, M., et al. (2019). Endophyte-mediated synthesis of silver nanoparticles and their biological applications. Appl. Microbiol. Biotechnol. 103, 2551–2569. doi:10.1007/s00253-019-09661-x
Ramadoss, D., Lakkineni, V. K., Bose, P., Ali, S., and Annapurna, K. J. S. (2013). Mitigation of salt stress in wheat seedlings by halotolerant bacteria isolated from saline habitats. SpringerPlus 2, 6. doi:10.1186/2193-1801-2-6
Ramalingmam, P., Muthukrishnan, S., and Thangaraj, P. (2015). Biosynthesis of silver nanoparticles using an endophytic fungus, Curvularia lunata and its antimicrobial potential. J. Nanosci. Nanoeng. 1, 241–247.
Rana, K. L., Kour, D., Kaur, T., Devi, R., Yadav, A. N., Yadav, N., et al. (2020). Endophytic microbes: Biodiversity, plant growth-promoting mechanisms and potential applications for agricultural sustainability. Antonie Leeuwenhoek 113, 1075–1107. doi:10.1007/s10482-020-01429-y
Rani, A., Souche, Y. S., and Goel, R. (2009). Comparative assessment of in situ bioremediation potential of cadmium resistant acidophilic Pseudomonas putida 62BN and alkalophilic Pseudomonas monteilli 97AN strains on soybean. Int. Biodeterior. Biodegrad. 63, 62–66. doi:10.1016/j.ibiod.2008.07.002
Rashid, S., Charles, T. C., and Glick, B. R. (2012). Isolation and characterization of new plant growth-promoting bacterial endophytes. Appl. Soil Ecol. 61, 217–224. doi:10.1016/j.apsoil.2011.09.011
Raven, J. A. (1983). The transport and function of silicon in plants. Biol. Rev. 58, 179–207. doi:10.1111/j.1469-185x.1983.tb00385.x
Ravi, P., Somu, P., Acharya, D., Gomez, L. A., Thathapudi, J. J., Ramachandra, Y. L., et al. (2022). Isolation and phytochemical screening of endophytic fungi isolated from medicinal plant Mappia foetida and evaluation of its in vitro cytotoxicity in cancer. Appl. Biochem. Biotechnol. 194, 4570–4586. doi:10.1007/s12010-022-03929-1
Rawat, N., Sneh, L., and Singla-Pareek, A. P. (2020). Membrane dynamics during individual and combined abiotic stresses in plants and tools to study the same. Physiol. Plant. 171, 653–676.
Reddy, P. V. L., Hernandez-Viezcas, J., Peralta-Videa, J., and Gardea-Torresdey, J. (2016). Lessons learned: Are engineered nanomaterials toxic to terrestrial plants? Sci. Total Environ. 568, 470–479. doi:10.1016/j.scitotenv.2016.06.042
Rehman, H., Ali, W., Khan, N. Z., Aasim, M., Khan, T., and Khan, A. L. (2022). Delphinium uncinatum mediated biosynthesis of zinc oxide nanoparticles and in-vitro evaluation of their antioxidant, cytotoxic, antimicrobial, anti-diabetic, anti-inflammatory, and anti-aging activities. Saudi J. Biol. Sci. 30, 103485. doi:10.1016/j.sjbs.2022.103485
Rojas-Tapias, D., Moreno-Galván, A., Pardo-Díaz, S., Obando, M., Rivera, D., and Bonilla, R. (2012). Effect of inoculation with plant growth-promoting bacteria (PGPB) on amelioration of saline stress in maize (Zea mays). Appl. Soil Ecol. 61, 264–272. doi:10.1016/j.apsoil.2012.01.006
Rouet, M., Mathieu, Y., Barbier-Brygoo, H., and Laurière, C. (2006). Characterization of active oxygen-producing proteins in response to hypo-osmolarity in tobacco and arabidopsis cell suspensions: Identification of a cell wall peroxidase. J. Exp. Bot. 57, 1323–1332. doi:10.1093/jxb/erj107
Roy, A., Pandit, C., Gacem, A., Alqahtani, M. S., Bilal, M., Islam, S., et al. (2022). Biologically derived gold nanoparticles and their applications. Bioinorg. Chem. Appl. 2022, 1–13. doi:10.1155/2022/8184217
Sabaghnia, N., and Janmohammadi, M. (2015). “Effect of nano-silicon particles application on salinity tolerance in early growth of some lentil genotypes,” in Annales universitatis mariae curie-sklodowska, sectio C–biologia, 39.
Sachdev, S., Ansari, S. A., Ansari, M. I., Fujita, M., and Hasanuzzaman, M. (2021). Abiotic stress and reactive oxygen species: Generation, signaling, and defense mechanisms. Antioxidants 10, 277. doi:10.3390/antiox10020277
Sadeghi, A., Karimi, E., Dahaji, P. A., Javid, M. G., Dalvand, Y., and Askari, H. (2018). Plant growth promoting activity of an auxin and siderophore producing isolate of Streptomyces under saline soil conditions. World J. Microbiol. Biotechnol. 34, 16. doi:10.1007/s11274-017-2393-4
Sadek, M. E., Shabana, Y. M., Sayed-Ahmed, K., and Abou-Tabl, A. H. (2022). Antifungal activities of sulfur and copper nanoparticles against cucumber postharvest diseases caused by Botrytis cinerea and Sclerotinia sclerotiorum. J. Fungi (Basel). 8, 412. doi:10.3390/jof8040412
Sahu, P. K., Thomas, P., Singh, S., and Gupta, A. (2020). Taxonomic and functional diversity of cultivable endophytes with respect to the fitness of cultivars against Ralstonia solanacearum. J. Plant Dis. Prot. (2006). 127, 667–676. doi:10.1007/s41348-020-00320-2
Saluja, B., Sharma, V. J. S., and Journal, S. (2014). Cadmium resistance mechanism in acidophilic and alkalophilic bacterial isolates and their application in bioremediation of metal-contaminated soil. Soil Sediment Contam. Int. J. 23, 1–17. doi:10.1080/15320383.2013.772094
Santhoshkumar, J., Sowmya, B., Kumar, S. V., and Rajeshkumar, S. (2019). Toxicology evaluation and antidermatophytic activity of silver nanoparticles synthesized using leaf extract of Passiflora caerulea. S. Afr. J. Chem. Eng. 29, 17–23. doi:10.1016/j.sajce.2019.04.001
Santoyo, G., Gamalero, E., and Glick, B. R. (2021a). Mycorrhizal-bacterial amelioration of plant abiotic and biotic stress. Front. Sustain. Food Syst. 5, 672881. doi:10.3389/fsufs.2021.672881
Santoyo, G., Guzmán-Guzmán, P., Parra-Cota, F. I., Santos-Villalobos, S. D. L., Orozco-Mosqueda, M. D. C., and Glick, B. R. (2021b). Plant growth stimulation by microbial consortia. Agron. (Basel). 11, 219. doi:10.3390/agronomy11020219
Santoyo, G., Moreno-Hagelsieb, G., Del Carmen Orozco-Mosqueda, M., and Glick, B. R. (2016). Plant growth-promoting bacterial endophytes. Microbiol. Res. 183, 92–99. doi:10.1016/j.micres.2015.11.008
Saqib, S., Nazeer, A., Ali, M., Zaman, W., Younas, M., Shahzad, A., et al. (2022). Catalytic potential of endophytes facilitates synthesis of biometallic zinc oxide nanoparticles for agricultural application. BioMetals 35, 967–985. doi:10.1007/s10534-022-00417-1
Sardar, R., Ahmed, S., and Yasin, N. A. (2022). Titanium dioxide nanoparticles mitigate cadmium toxicity in Coriandrum sativum L. through modulating antioxidant system, stress markers and reducing cadmium uptake. Environ. Pollut. 292, 118373. doi:10.1016/j.envpol.2021.118373
Savvas, D., Giotis, D., Chatzieustratiou, E., Bakea, M., and Patakioutas, G. (2009). Silicon supply in soilless cultivations of zucchini alleviates stress induced by salinity and powdery mildew infections. Environ. Exp. Bot. 65, 11–17. doi:10.1016/j.envexpbot.2008.07.004
Saxena, R., Tomar, R. S., and Kumar, M. (2016). Exploring nanobiotechnology to mitigate abiotic stress in crop plants. J. Pharm. Sci. Res. 8, 974.
Sedghi, M., Hadi, M., and Toluie, S. G. (2013). Effect of nano zinc oxide on the germination parameters of soybean seeds under drought stress. Ser. Biol. 16, 73.
Sen, S., and Chandrasekhar, C. (2014). Effect of PGPR on growth promotion of rice (Oryza sativa L.) under salt stress. Asian J. Plant Sci. Res. 4, 62–67.
Shabnam, N., Pardha-Saradhi, P., and Sharmila, P. (2014). Phenolics impart Au3+-stress tolerance to cowpea by generating nanoparticles. PLoS One 9, e85242. doi:10.1371/journal.pone.0085242
Shah, V., Luxton, T. P., Walker, V. K., Brumfield, T., Yost, J., Shah, S., et al. (2016). Fate and impact of zero-valent copper nanoparticles on geographically-distinct soils. Sci. Total Environ. 573, 661–670. doi:10.1016/j.scitotenv.2016.08.114
Shahid, M., Zeyad, M. T., Syed, A., Singh, U. B., Mohamed, A., Bahkali, A. H., et al. (2022). Stress-tolerant endophytic isolate Priestia aryabhattai BPR-9 modulates physio-biochemical mechanisms in wheat (Triticum aestivum L.) for enhanced salt tolerance. Int. J. Environ. Res. Public Health 19, 10883. doi:10.3390/ijerph191710883
Sharma, A., Sagar, A., Rana, J., and Rani, R. (2022). Green synthesis of silver nanoparticles and its antibacterial activity using fungus Talaromyces purpureogenus isolated from Taxus baccata Linn. Micro Nano Syst. Lett. 10, 2–12. doi:10.1186/s40486-022-00144-9
Sharma, A., Shankhdhar, D., and Shankhdhar, S. (2013). Enhancing grain iron content of rice by the application of plant growth promoting rhizobacteria. Plant Soil Environ. 59, 89–94. doi:10.17221/683/2012-pse
Sharma, P., Jha, A. B., Dubey, R. S., and Pessarakli, M. (2012). Reactive oxygen species, oxidative damage, and antioxidative defense mechanism in plants under stressful conditions. J. Bot. 2012, 1–26. doi:10.1155/2012/217037
Sheikhalipour, M., Esmaielpour, B., Behnamian, M., Gohari, G., Giglou, M. T., Vachova, P., et al. (2021). Chitosan–selenium nanoparticle (Cs–Se NP) foliar spray alleviates salt stress in bitter melon. Nanomaterials 11, 684. doi:10.3390/nano11030684
Sherin, G., Raj-Aswathi, K. P., and Puthur, J. T. (2022). Photosynthetic functions in plants subjected to stresses are positively influenced by priming. Plant Stress 4, 100079. doi:10.1016/j.stress.2022.100079
Shilev, S., Sancho, E. D., and Benlloch-González, M. J. (2012). Rhizospheric bacteria alleviate salt-produced stress in sunflower. J. Environ. Manage. 95, S37–S41. doi:10.1016/j.jenvman.2010.07.019
Shinde, B. H., Inamdar, S. N., Nalawade, S. A., and Chaudhari, S. B. (2022). A systematic review on antifungal and insecticidal applications of biosynthesized metal nanoparticles. Mater. Today Proc. doi:10.1016/j.matpr.2022.09.548
Shirinbayan, S., Khosravi, H., and Malakouti, M. J. (2019). Alleviation of drought stress in maize (Zea mays) by inoculation with Azotobacter strains isolated from semi-arid regions. Appl. Soil Ecol. 133, 138–145. doi:10.1016/j.apsoil.2018.09.015
Shukla, P. S., Agarwal, P. K., and Jha, B. J. J. O. P. G. R. (2012). Improved salinity tolerance of Arachis hypogaea (L.) by the interaction of halotolerant plant-growth-promoting rhizobacteria. J. Plant Growth Regul. 31, 195–206. doi:10.1007/s00344-011-9231-y
Silambarasan, S., Logeswari, P., Valentine, A., and Cornejo, P. (2019). Role of Curtobacterium herbarum strain CAH5 on aluminum bioaccumulation and enhancement of Lactuca sativa growth under aluminum and drought stresses. Ecotoxicol. Environ. Saf. 183, 109573. doi:10.1016/j.ecoenv.2019.109573
Silambarasan, S., and Vangnai, A. S. (2017). Plant-growth promoting Candida sp. AVGB4 with capability of 4-nitroaniline biodegradation under drought stress. Ecotoxicol. Environ. Saf. 139, 472–480. doi:10.1016/j.ecoenv.2017.02.018
Simontacchi, M., Galatro, A., Ramos-Artuso, F., and Santa-María, G. E. (2015). Plant survival in a changing environment: The role of nitric oxide in plant responses to abiotic stress. Front. Plant Sci. 6, 977. doi:10.3389/fpls.2015.00977
Singh, D. P., Prabha, R., Yandigeri, M. S., and Arora, D. K. (2011). Cyanobacteria-mediated phenylpropanoids and phytohormones in rice (Oryza sativa) enhance plant growth and stress tolerance. Ant. Van Leeuwenhoek 100, 557–568. doi:10.1007/s10482-011-9611-0
Singh, D., Rathod, V., Ninganagouda, S., Herimath, J., and Kulkarni, P. (2013). Biosynthesis of silver nanoparticle by endophytic fungi Pencillium sp. isolated from Curcuma longa (turmeric) and its antibacterial activity against pathogenic gram negative bacteria. J. Pharm. Res. 7, 448–453. doi:10.1016/j.jopr.2013.06.003
Singh, J., and Lee, B.-K. (2016). Influence of nano-TiO2 particles on the bioaccumulation of Cd in soybean plants (Glycine max): A possible mechanism for the removal of Cd from the contaminated soil. J. Environ. Manage. 170, 88–96. doi:10.1016/j.jenvman.2016.01.015
Singh, J., Perumal, V., Singh, U., Tripathi, D. K., and Sharma, S. (2022a). Green synthesis of silver nanoparticles from bark extract of terminalia arjuna and their application as next generation antibacterial agents. Curr. Nanosci. 18, 743–757. doi:10.2174/1573413718666220221102909
Singh, J., Vishwakarma, K., Ramawat, N., Rai, P., Singh, V. K., Mishra, R. K., et al. (2019). Nanomaterials and microbes’ interactions: A contemporary overview. 3 Biotech. 9, 68–14. doi:10.1007/s13205-019-1576-0
Singh, M., Qureshi, K. A., Jaremko, M., Rajput, M., Singh, S. K., KaushalendraPandey, K. D., et al. (2022b). Bioprospects of endophytic bacteria in plant growth promotion and ag-nanoparticle biosynthesis. Plants (Basel). 11, 1787. doi:10.3390/plants11141787
Soliman, A. M., Abdel-Latif, W., Shehata, I. H., Fouda, A., Abdo, A. M., and Ahmed, Y. M. (2021). Green approach to overcome the resistance pattern of Candida spp. using biosynthesized silver nanoparticles fabricated by Penicillium chrysogenum F9. Biol. Trace Elem. Res. 199, 800–811. doi:10.1007/s12011-020-02188-7
Soltani Nejad, M., Samandari Najafabadi, N., Aghighi, S., Pakina, E., and Zargar, M. (2022). Evaluation of Phoma sp. biomass as an endophytic fungus for synthesis of extracellular gold nanoparticles with antibacterial and antifungal properties. Molecules 27, 1181. doi:10.3390/molecules27041181
Sonawane, H., Shelke, D., Chambhare, M., Dixit, N., Math, S., Sen, S., et al. (2022). Fungi-derived agriculturally important nanoparticles and their application in crop stress management–Prospects and environmental risks. Environ. Res. 212, 113543. doi:10.1016/j.envres.2022.113543
Sonbol, H., Mohammed, A., and Korany, S. M. (2022). Soil fungi as biomediator in silver nanoparticles formation and antimicrobial efficacy. Int. J. Nanomedicine 17, 2843–2863. doi:10.2147/ijn.s356724
Sonkar, S., Pandey, B., Rathore, S. S., Sharma, L., and Singh, A. K. (2021). “Applications of nanobiotechnology in overcoming temperature stress,” in Nanobiotechnology. Editors J. M. Al-Khayri, M. I. Ansari, and A. K. Singh (Cham: Springer), 417–435.
Sorty, A. M., Meena, K. K., Choudhary, K., Bitla, U. M., Minhas, P., and Krishnani, K. (2016). Effect of plant growth promoting bacteria associated with halophytic weed (Psoralea corylifolia L) on germination and seedling growth of wheat under saline conditions. Appl. Biochem. Biotechnol. 180, 872–882. doi:10.1007/s12010-016-2139-z
Suarez, C., Cardinale, M., Ratering, S., Steffens, D., Jung, S., Montoya, A. M. Z., et al. (2015). Plant growth-promoting effects of Hartmannibacter diazotrophicus on summer barley (Hordeum vulgare L.) under salt stress. Appl. Soil Ecol. 95, 23–30. doi:10.1016/j.apsoil.2015.04.017
Sun, C., Johnson, J. M., Cai, D., Sherameti, I., Oelmüller, R., and Lou, B. (2010). Piriformospora indica confers drought tolerance in Chinese cabbage leaves by stimulating antioxidant enzymes, the expression of drought-related genes and the plastid-localized CAS protein. J. Plant Physiol. 167, 1009–1017. doi:10.1016/j.jplph.2010.02.013
Sunkar, S., and Nachiyar, C. V. (2012a). Biogenesis of antibacterial silver nanoparticles using the endophytic bacterium Bacillus cereus isolated from Garcinia xanthochymus. Asian pac. J. Trop. Biomed. 2, 953–959. doi:10.1016/s2221-1691(13)60006-4
Sunkar, S., and Nachiyar, C. V. (2012b). Microbial synthesis and characterization of silver nanoparticles using the endophytic bacterium Bacillus cereus: A novel source in the benign synthesis. Glob. J. Med. Res. 12, 43–50.
Teheran-Sierra, L. G., Funnicelli, M. I. G., De Carvalho, L. a. L., Ferro, M. I. T., Soares, M. A., and Pinheiro, D. G. (2021). Bacterial communities associated with sugarcane under different agricultural management exhibit a diversity of plant growth-promoting traits and evidence of synergistic effect. Microbiol. Res. 247, 126729. doi:10.1016/j.micres.2021.126729
Thepbandit, W., Papathoti, N. K., Daddam, J. R., Thumanu, K., Siriwong, S., Thanh, T. L., et al. (2021). Identification of salicylic acid mechanism against leaf blight disease in Oryza sativa by SR-FTIR microspectroscopic and docking studies. Pathogens 10, 652. doi:10.3390/pathogens10060652
Thiagarajan, V., and Ramasubbu, S. (2021). Fate and behaviour of TiO2 nanoparticles in the Soil: Their impact on staple food crops. Water Air Soil Pollut. 232, 274–318. doi:10.1007/s11270-021-05219-8
Thomas, T. T, D., and Puthur, J. T. (2017). UV radiation priming: A means of amplifying the inherent potential for abiotic stress tolerance in crop plants. Environ. Exp. Bot. 138, 57–66. doi:10.1016/j.envexpbot.2017.03.003
Tittabutr, P., Piromyou, P., Longtonglang, A., Noisa-Ngiam, R., Boonkerd, N., and Teaumroong, N. (2013). Alleviation of the effect of environmental stresses using co-inoculation of mungbean by Bradyrhizobium and rhizobacteria containing stress-induced ACC deaminase enzyme. Soil Sci. Plant Nutr. 59, 559–571. doi:10.1080/00380768.2013.804391
Tiwari, S., Lata, C., Chauhan, P. S., and Nautiyal, C. S. (2016). Pseudomonas putida attunes morphophysiological, biochemical and molecular responses in Cicer arietinum L. during drought stress and recovery. Plant Physiol. biochem. 99, 108–117. doi:10.1016/j.plaphy.2015.11.001
Tripathi, D. K., Singh, V. P., Prasad, S. M., Chauhan, D. K., and Dubey, N. K. (2015). Silicon nanoparticles (SiNp) alleviate chromium (VI) phytotoxicity in Pisum sativum (L.) seedlings. Plant Physiol. biochem. 96, 189–198. doi:10.1016/j.plaphy.2015.07.026
Turbat, A., Rakk, D., Vigneshwari, A., Kocsubé, S., Thu, H., Szepesi, Á., et al. (2020). Characterization of the plant growth-promoting activities of endophytic fungi isolated from Sophora flavescens. Microorganisms 8, 683. doi:10.3390/microorganisms8050683
Tuteja, N., and Mahajan, S. (2007). Calcium signaling network in plants: An overview. Plant Signal. Behav. 2, 79–85. doi:10.4161/psb.2.2.4176
Tuteja, N., and Sopory, S. K. (2008). Chemical signaling under abiotic stress environment in plants. Plant Signal. Behav. 3, 525–536. doi:10.4161/psb.3.8.6186
Umapathy, V. R., Natarajan, P. M., Sumathijones, C., Swamikannu, B., Johnson, W. M. S., Alagarsamy, V., et al. (2022). Current trends and future perspectives on dental nanomaterials – an overview of nanotechnology strategies in dentistry. J. King Saud Univ. - Sci. 34, 102231. doi:10.1016/j.jksus.2022.102231
Upadhyay, S., Singh, J., and Singh, D. P. (2011). Exopolysaccharide-producing plant growth-promoting rhizobacteria under salinity condition. Pedosphere 21, 214–222. doi:10.1016/s1002-0160(11)60120-3
Van Nguyen, D., Nguyen, H. M., Le, N. T., Nguyen, K. H., Nguyen, H. T., Le, H. M., et al. (2022). Copper nanoparticle application enhances plant growth and grain yield in maize under drought stress conditions. J. Plant Growth Regul. 41, 364–375. doi:10.1007/s00344-021-10301-w
Varshney, R. K., Bansal, K. C., Aggarwal, P. K., Datta, S. K., and Craufurd, P. Q. (2011). Agricultural biotechnology for crop improvement in a variable climate: Hope or hype? Trends Plant Sci. 16, 363–371. doi:10.1016/j.tplants.2011.03.004
Vimal, S. R., Patel, V. K., and Singh, J. S. (2019). Plant growth promoting Curtobacterium albidum strain SRV4: An agriculturally important microbe to alleviate salinity stress in paddy plants. Ecol. Indic. 105, 553–562. doi:10.1016/j.ecolind.2018.05.014
Vurukonda, S. S. K. P., Vardharajula, S., Shrivastava, M., and Skz, A. J. R. (2016). Multifunctional Pseudomonas putida strain FBKV2 from arid rhizosphere soil and its growth promotional effects on maize under drought stress. Rhizosphere 1, 4–13. doi:10.1016/j.rhisph.2016.07.005
Wahid, A. (2007). Physiological implications of metabolite biosynthesis for net assimilation and heat-stress tolerance of sugarcane (Saccharum officinarum) sprouts. J. Plant Res. 120, 219–228. doi:10.1007/s10265-006-0040-5
Wang, C. Y., Makvandi, P., Zare, E. N., Tay, F. R., and Niu, L. N. (2020). Advances in antimicrobial organic and inorganic nanocompounds in biomedicine. Adv. Ther. (Weinh). 3, 2000024. doi:10.1002/adtp.202000024
Worms, I. A., Boltzman, J., Garcia, M., and Slaveykova, V. I. (2012). Cell-wall-dependent effect of carboxyl-CdSe/ZnS quantum dots on lead and copper availability to green microalgae. Environ. Pollut. 167, 27–33. doi:10.1016/j.envpol.2012.03.030
Wu, H., Yin, J.-J., Wamer, W. G., Zeng, M., and Lo, Y. M. (2014). Reactive oxygen species-related activities of nano-iron metal and nano-iron oxides. J. Food Drug Anal. 22, 86–94. doi:10.1016/j.jfda.2014.01.007
Wu, Z., Yue, H., Lu, J., and Li, C. J. (2012). Characterization of rhizobacterial strain Rs-2 with ACC deaminase activity and its performance in promoting cotton growth under salinity stress. World J. Microbiol. Biotechnol. 28, 2383–2393. doi:10.1007/s11274-012-1047-9
Xu, H., Lu, Y., and Tong, S. (2018). Effects of arbuscular mycorrhizal fungi on photosynthesis and chlorophyll fluorescence of maize seedlings under salt stress. Emir. J. Food Agric. 30, 199–204. doi:10.9755/ejfa.2018.v30.i3.1642
Yadav, A. N. (2017). Agriculturally important microbiomes: Biodiversity and multifarious PGP attributes for amelioration of diverse abiotic stresses in crops for sustainable agriculture. Biomed. J. Sci. Tech. Res. 1, 861–864. doi:10.26717/bjstr.2017.01.000321
Yang, Y., Chen, Q., Wall, J. D., and Hu, Z. (2012a). Potential nanosilver impact on anaerobic digestion at moderate silver concentrations. Water Res. 46, 1176–1184. doi:10.1016/j.watres.2011.12.024
Yang, Y., Mathieu, J. M., Chattopadhyay, S., Miller, J. T., Wu, T., Shibata, T., et al. (2012b). Defense mechanisms of Pseudomonas aeruginosa PAO1 against quantum dots and their released heavy metals. ACS Nano 6, 6091–6098. doi:10.1021/nn3011619
Yashavantha Rao, H. C., Nagendra-Prasad, M. N., Prasad, A., Harini, B. P., Azmath, P., Rakshith, D., et al. (2016). Biomimetic synthesis of silver nanoparticles using endosymbiotic bacterium inhabiting Euphorbia hirta L. and their bactericidal potential. Scientifica 2016, 9020239. doi:10.1155/2016/9020239
Yolcu, S., Ozdemir, F., Güler, A., and Bor, M. (2016). Histone acetylation influences the transcriptional activation of POX in Beta vulgaris L. and Beta maritima L. under salt stress. Plant Physiol. biochem. 100, 37–46. doi:10.1016/j.plaphy.2015.12.019
Younesi, O., and Moradi, A. J. A. (2014). Effects of plant growth-promoting rhizobacterium (PGPR) and arbuscular mycorrhizal fungus (AMF) on antioxidant enzyme activities in salt-stressed bean (Phaseolus vulgaris L.) Agriculture 60, 10–21. doi:10.2478/agri-2014-0002
Zare, A. S., Ganjeali, A., Kakhki, M. R. V., Cheniany, M., and Mashreghi, M. (2022). Plant elicitation and TiO2 nanoparticles application as an effective strategy for improving the growth, biochemical properties, and essential oil of peppermint. Physiol. Mol. Biol. Plants 28, 1391–1406.
Zhang, J., Wang, P., Tian, H., Jiang, H., Wang, Y., and Yan, C. J. S. (2018). Identification of interior salt-tolerant bacteria from ice plant Mesembryanthemum crystallinum and evaluation of their promoting effects. Symbiosis 76, 243–252. doi:10.1007/s13199-018-0551-6
Zhang, R., Tu, C., Zhang, H., and Luo, Y. (2020). Stability and transport of titanium dioxide nanoparticles in three variable-charge soils. J. Soils Sediments 20, 1395–1403. doi:10.1007/s11368-019-02509-x
Zhao, L., Peng, B., Hernandez-Viezcas, J. A., Rico, C., Sun, Y., Peralta-Videa, J. R., et al. (2012). Stress response and tolerance of Zea mays to CeO2 nanoparticles: Cross talk among H2O2, heat shock protein, and lipid peroxidation. ACS Nano 6, 9615–9622. doi:10.1021/nn302975u
Zhou, H., Wu, H., Zhang, F., Su, Y., Guan, W., Xie, Y., et al. (2021). Molecular basis of cerium oxide nanoparticle enhancement of rice salt tolerance and yield. Environ. Sci. Nano 8, 3294–3311. doi:10.1039/d1en00390a
Zhou, J., Li, P., Meng, D., Gu, Y., Zheng, Z., Yin, H., et al. (2020). Isolation, characterization and inoculation of Cd tolerant rice endophytes and their impacts on rice under Cd contaminated environment. Environ. Pollut. 260, 113990. doi:10.1016/j.envpol.2020.113990
Keywords: environmental stressors, food security, plant health management, soil-plant microbe interactions, sustainable development goal 2, zero hunger
Citation: Adeleke BS, Akinola SA, Adedayo AA, Glick BR and Babalola OO (2022) Synergistic relationship of endophyte-nanomaterials to alleviate abiotic stress in plants. Front. Environ. Sci. 10:1015897. doi: 10.3389/fenvs.2022.1015897
Received: 10 August 2022; Accepted: 14 November 2022;
Published: 24 November 2022.
Edited by:
Parul Chaudhary, G. B. Pant University of Agriculture and Technology, IndiaReviewed by:
Priyanka Khati, Indian Council of Agricultural Research (ICAR), IndiaGovind Kumar, Central Institute for Subtropical Horticulture (ICAR), India
Copyright © 2022 Adeleke, Akinola, Adedayo, Glick and Babalola. This is an open-access article distributed under the terms of the Creative Commons Attribution License (CC BY). The use, distribution or reproduction in other forums is permitted, provided the original author(s) and the copyright owner(s) are credited and that the original publication in this journal is cited, in accordance with accepted academic practice. No use, distribution or reproduction is permitted which does not comply with these terms.
*Correspondence: Olubukola Oluranti Babalola, b2x1YnVrb2xhLmJhYmFsb2xhQG53dS5hYy56YQ==