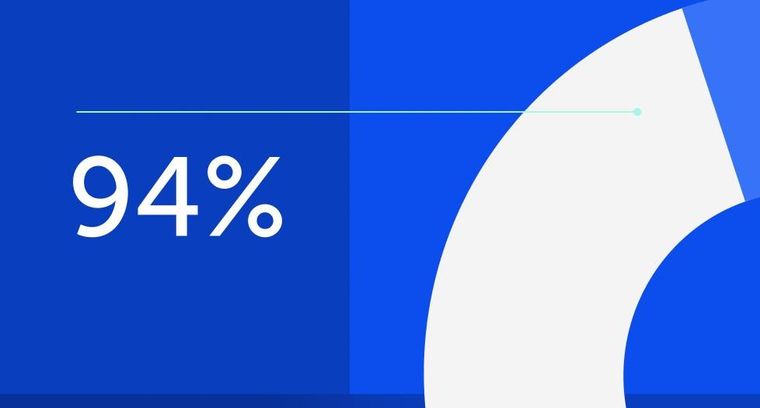
94% of researchers rate our articles as excellent or good
Learn more about the work of our research integrity team to safeguard the quality of each article we publish.
Find out more
ORIGINAL RESEARCH article
Front. Environ. Sci., 11 October 2022
Sec. Toxicology, Pollution and the Environment
Volume 10 - 2022 | https://doi.org/10.3389/fenvs.2022.1011820
Soil pollution with heavy metals has become a global issue because of anthropogenic activities causing gradual loss of soil nutrients and fertility, thus, reducing agricultural production. Biochar is recommended as an organic and environment-friendly option to address the issue of nutrient deficiency and heavy metal pollution. The present study was performed with biochar derived from Cannabis sativa to surplus soil nutrient pool and heavy metal immobilization. The characterization through scanning electron microscopy (SEM) revealed that biochar was brittle, porous, alkaline, and labile in nature. The elemental composition of biochar was carbon (75.3%), oxygen (19.2%), calcium (3.9%), potassium (1.5%), and chlorine (0.08%) determined by energy dispersive X-ray spectroscopy. Fourier transform infrared (FTIR) spectroscopy revealed the occurrence of carbonyl group, phenols, and alcohols in biochar derived from Cannabis sativa. The soil was spiked with lead and cadmium salt solution (25 ppm and 250) and incubated for 30 days. It was found that biochar amendments (1% = BC1 and 5% = BC5) significantly (p < 0.05) increased the soil physicochemical properties such as pH, electrical conductivity (EC), oxidizable organic carbon (OC), total organic carbon (TOC), and organic matter (OM). In the case of BC5, OC increased by 189.86%, TOC increased by 189.13%, and OM increased by 188.68%, as compared to the control. Similarly, soil available nitrogen (AN) and soil available phosphorous improved by 233.3% and 101.79%, respectively, compared to control. On the other hand, BC1 showed a significant reduction (p < 0.05) in lead and cadmium concentrations by 45.74% and 56.58%, respectively, in comparison to BC5 and control. In conclusion, we suggest that Cannabis sativa biochar may serve as an effective treatment for enhancing soil fertility and remediation of soil polluted with heavy metals.
Soil is not only a substrate for plant growth but also the ultimate sink for organic and inorganic pollutants such as pesticides and heavy metals (Qayyum et al., 2017; Tu et al., 2020). As a consequence of human activities such as mining, oil drilling, waste disposal, industrial operations, and pesticide and metal processing, heavy metal pollution has become a global challenge for sustainable agriculture in recent years (Abbas et al., 2017a; Shen et al., 2018; Juraszek and Piasecka, 2020). Once heavy metals enter the soil environment through irrigation with wastewater or land application of contaminated biosolids, they can induce irreversible damage to plant growth and enter the human body through ingestion of edible plants parts, causing inactivation of enzymes and destruction of proteins (Hamzenejad Taghlidabad and Sepehr, 2018). In addition to the tremendous amount of heavy metal pollution, soils all over the world are being overexploited for food production, raising concerns for the agricultural ecosystem.
The extensive use of inorganic fertilizers to replenish soil nutrients is disregarded as a cause of heavy metal accumulation in agricultural soil. Continuous cropping practices lead to gradual loss of soil fertility that will promote the widespread use of inorganic fertilizers (Sandhu et al., 2017) that contain essential nutrients along with trace quantities of heavy metals (Iheanacho et al., 2017). Therefore, the inevitable use of chemical fertilizers is now recognized as an unsustainable agricultural strategy because it is causing rapid soil organic matter mineralization and addition of heavy metals, posing a threat to the soil environment (Naeem et al., 2018) (Barrow, 2012). Ultimately, there is a need to reduce the risk of heavy metals by limiting the bioavailability in the food chain via metal absorption and their accumulation in the sink (Juraszek and Piasecka, 2020).
Organic fertilizers, including compost and manure, were created as alternatives to chemical fertilizers; nevertheless, despite their eco-friendliness, they include pathogens, pharmaceuticals, and organic waste and have the potential to contaminate streams and groundwater with nutrients (Barrow, 2012; Al-Wabel et al., 2017). Due to their organic nature, pharmaceuticals and organic waste were readily decomposed by soil microbes, whereas heavy metal removal requires costly and risky physical and chemical processes such as precipitation, ion exchange, adsorption, and electro-coagulation. Adsorption is a fast and cost-effective method for recovering heavy metal polluted soils (Park et al., 2015).
Considering the situation, a more resistant organic amendment called “agrichar” or “biochar” that may survive in soils for thousands of years (Naeem et al., 2018) has received particular attention for treating soil nutrient deficit and metal pollution via adsorption (Cao et al., 2011; Ahmad et al., 2017; Li F. et al., 2021). Biochar is an organic substance that is typically manufactured from a range of biomass, such as wood, branches, leaves, agricultural waste (Han et al., 2013), shells, husk, and animal and poultry waste (Yang et al., 2017; Hamzenejad Taghlidabad and Sepehr, 2018). The biomass feedstock can be pyrolyzed at a high temperature with limited oxygen. The resulting material (biochar) is highly porous and stabilized and can be utilized as an adsorbent due to the large surface area, microporosity, functional groups, and hydrophobicity (Omidi et al., 2020). Biochar is regarded as a soil conditioner because it influences soil properties such as high water holding and cation exchange capacity, which helps in retaining nutrients and microbial activity in the soil (Fellet et al., 2011; Wu et al., 2017; Rizwan et al., 2018).
The characteristics and efficiency of biochar vary depending on the feedstock and pyrolysis temperature (Tu et al., 2020; Manori et al., 2021). The pyrolysis temperature is an important factor in determining the adsorption capacity of heavy metals in each feedstock (Ding et al., 2014; Lian et al., 2020). For instance, biochar produced at higher temperatures performed better for metal immobilization than those produced at lower temperatures (Paz-Ferreiro et al., 2014; Shu et al., 2016; Kavitha et al., 2018; Wei et al., 2019). It was also reported that oxygen-containing functional groups tend to be formed at lower pyrolysis temperatures, while aromatic structures and alkaline minerals are always formed at higher temperatures (Yang et al., 2019). Because of its porous structure, biochar is perfect for adsorption and has been effectively used to stabilize different pollutants in soil (Gholizadeh and Hu, 2021).
Cannabis is an annual flowering herb that grows rapidly in large areas; it is extensively cultivated and used in central Asia. Cannabis is a moderately common plant that grows abundantly in northern Pakistan. Although the cultivation, possession, and distribution of cannabis are prohibited in Pakistan by the National Act of 1997, however, this plant offers a broad variety of potential uses and grows abundantly in the wild. Cannabis has hitherto been utilized exclusively for medical research, but there are several opportunities to use it as an energy crop, an absorbent, insulation, pyrolysis material, and activated biomass, all of which improve the soil systems. Recognizing the diverse application and the potential for turning a wild-grown plant into a useful product, Pakistan’s Federal Government allowed the legalization of Cannabis production, primarily for scientific, technological, and industrial research purposes. In this study, Cannabis sativa was used to prepare biochar, and this activated product was used as soil conditional and metal adsorbent in contaminated soil.
Cannabis sativa, commonly known as industrial hemp, was collected from the roadside of Islamabad. The plant biomass, including the stems and branch of C. staiva, was collected, air-dried to remove moisture, chopped into 2–3-inch pieces, and further dried in an oven at 105°C for 1 h to remove any remaining moisture (Qadeer et al., 2014). Healthy dried stems were used for the biochar preparation.
The oven-dried Cannabis sativa biomass was pyrolyzed at 450°C for 20 min in a muffle furnace (Qadeer et al., 2014). After 20 min of pyrolysis, the material was allowed to cool at room temperature. The obtained black and brittle biochar was weighed and placed in an airtight plastic jar with silica gel (for moisture absorption) till further use. The biochar was ground to powder using a pestle and mortar and sieved to 0.5-mm for characterization and even distribution in the soil (Xu et al., 2013).
The prepared biochar was analyzed for physico-chemical parameters. pH and EC of the prepared biochar were measured using calibrated multi-parameter PCSTestr™ 35. Powdered biochar solution was prepared by using deionized water in a 1:20 solid:deionized water ratio (Hamdani et al., 2017). Conversion efficiency or yield of biochar was determined using the following formula:
Weights were recorded prior to and after pyrolysis for calculating the yield (Hamdani et al., 2017).
To calculate the bulk density, the biochar sample was placed in an oven at 105°C for 24 h. Afterward, it was noted that the weight of the oven-dried biochar occupied 50 ml cylinder volume, and it was divided by the volume of the cylinder used (Qadeer et al., 2014; Khater, 2015). Bulk density was calculated using the formula given by Dawood et al. (2017):
The moisture content of the biochar was calculated by drying the biochar in a muffle furnace for 10 min at 750°C. It was then taken out of the furnace and allowed to cool down at room temperature, after which it was weighed (ASTM, 2007). The dried biochar sample was then further placed in an oven at 105°C for 2 h. Afterward, it was allowed to cool down to room temperature and weighed again (Batool et al., 2015). The moisture content was calculated using the formula given in ASTM D1762-84:
The volatile content of the biochar was determined by subjecting the dried biochar to 550°C in a muffle furnace for 3 h (Waqas et al., 2018). The volatile content was calculated using the formula given in ASTM (2007):
To determine the ash content, the biochar sample was weighed and placed in a crucible uncovered. It was then heated to 800°C for 4 h in a muffle furnace (Batool et al., 2015). After that, it was removed from the furnace, cooled to room temperature, and weighed again. The ash content was calculated according to the following formula (Waqas et al., 2018):
Fixed carbon was calculated by the difference in mass using the following formula (Lam et al., 2018):
FC (%) = 100—Ash content (%)—Moisture content (%)—Volatile content (%)
Scanning electron microscopy with energy dispersive X-ray spectroscopy (SEM-EDX) was used to determine the surface morphology (porosity, pore size, and pore shape) and elemental composition of the prepared biochar (Xu et al., 2013; Waqas et al., 2018). Tescan Vega 3 scanning electron microscope with Oxford EDX detector at the National University of Science and Technology (CASEN-NUST) was used to perform SEM-EDX.
In order to determine the surface functional groups, Fourier transform infrared spectroscopy (FTIR) was employed (Xu et al., 2013; Waqas et al., 2018). About 0.7–1 mg of powdered biochar and 100 mg of spectroscopic grade potassium bromide (KBr) were mixed and pressed in a hydraulic press to form a pellet (Pourret and Houben, 2018). A Perkin Elmer FTIR spectrophotometer at the National University of Science and Technology (SCME-NUST) was used to analyze the prepared KBr and biochar pellets, scanning in the range of 400–4,000 cm−1, with an average of 32 scans and a resolution of 4 cm−1 (Waqas et al., 2018). FTIR spectrum obtained was interpreted using already reported data in previous literature (Mohanty et al., 2013).
Soil was collected from a private agricultural farmland area near Chak Shahzad in Islamabad; the cultivator does not apply inorganic fertilizer to the fields. Soil was collected from the top/surface 0–20 cm, air-dried, grounded, and passed through a 2-mm sieve to homogenize the soil sample (Abbas et al., 2017b). To determine the metal (Pb & Cd) background concentration, 5 g of air-dried soil was acid digested (HNO3: HCl; 1:3) to be analyzed through inductively coupled plasma optical emission spectrometry (ICP-OES).
The air-dried soil was spiked with Pb(NO3)2 and Cd(NO3)2 solutions (Bashir et al., 2018; Mehmood et al., 2018), at concentrations of 25 ppm and 250 ppm, respectively, which were nearly four times the average concentrations of Pb and Cd in soils of Islamabad (Faiz et al., 2009). After spiking, the soil was incubated at room temperature (22 ± 2°C) and aged for 15 days to allow adequate exposure of soil particles to metal contamination; the soil pack was occasionally mixed to homogenize the bulk soil (Khalkhaliani et al., 2006). Following this period, the soil was divided equally into three portions (in triplicates) for different treatments/experimental set-up.
A pot experiment was conducted to evaluate the effects of biochar addition on the soil nutrient pool and soil metal immobilization. Incubated soil was shifted into pots with a capacity of 2.5 kg, and all pots were grouped in different treatments of soil biochar combination and control soil. The prepared biochar was added to the pots with spiked soil separately, at two different concentrations, i.e., 1% and 5% (w/w). The pots without any biochar addition were used as the control (Table 1). All the pots were arranged in a complete randomized design and placed in a laboratory at room temperature (22 ± 2°C).
After biochar addition, the field capacity of the soil was calculated, and the overall set-up was maintained at 70% field capacity gravimetrically. The pots were covered with plastic food wrap to avoid loss of moisture; however, small holes were made in them to allow an exchange of gases (Mehmood et al., 2018). The entire experimental set-up was kept at room temperature for a period of 1 month, and all the treatments, along with the control, were set in triplicates (Qadeer et al., 2014; Bashir et al., 2018; Mehmood et al., 2018). Soil samples from each treatment were collected individually and periodically, i.e., at the beginning of the experimental set-up (time zero) and after an interval of every 10 days, for a period of 1 month. Each time, the samples were taken directly from every treatment and control pots and analyzed in triplicates. None of the samples was used as a composite sample.
pH and EC of the biochar amended soil samples and of the control were measured using calibrated multi-parameter PCSTestr™ 35. In a solution of soil:deionized water, at the ratio of 1:5 (Bashir et al., 2018).
Walkley and Black’s (1934) titration method was employed to calculate the organic matter (OM) (Qadeer et al., 2014), total organic carbon (TOC), and oxidizable organic carbon (OC) (Choudhary et al., 2018) present in the soil samples. OC, TOC, and OM were calculated using the following formulae:
where
M = molarity of FAS solution–about 0.5 M.
VBlank = volume of FAS solution required to titrate the blank (ml).
VSample = volume of FAS solution required to titrate the sample (ml).
0.3 = 3 × 10−3 × 100, where 3 is the equivalent weight of C.
Soil available nitrogen (AN) was analyzed using the method described by Arif et al. (2018). In total, 5 g of soil sample and 25 ml of K2SO4 were added to a 50 ml centrifuge tube and shaken at 150 rpm for a period of 60 min. A total of 0.5 ml of the extract was taken and mixed with 1 ml of salicylic acid. The mixture was allowed to stand for 10 min, after which 10 ml of 0.02 M NaOH was added and incubated at 25°C for 1 h. The absorbance was measured at 410 nm using an O.R.I. UV4000-Visible spectrophotometer (Germany).
For measuring soil available phosphorous, 3 g of air-dried soil was mixed with 60 ml 0.5 M NaHCO3 in a 150 ml flask and shaken at 150 rpm for 30 min. The mixture was filtered, and the filtrate was analyzed for soil available phosphorous using an ascorbate-molybdate reagent on a UV Visible spectrophotometer at 880 nm (Arif et al., 2018).
Soil samples were acid digested with aqua regia (HNO3: HCl; 1:3), and digestate was used for heavy metals (Pb and Cd) analysis through ICP-OES using the method described by O’Shea et al. (2018).
For statistical analysis, Microsoft Excel 2013 was used for the computation of mean and standard deviation (Batool et al., 2015), and the mean values of all treatments were compared through ANOVA and LSD using the “R” program.
The Freundlich adsorption isotherm was used to check the efficiency of adsorption at different biochar concentrations, considering the constant ambient temperature on a specified dose, the response was monitored against fluctuating pH, and the modified mechanism of the isotherm calculation was
where dy is the metal removed from the soil, dx is the concentration of the biochar applied, and pH is the pH of the soil corresponding to the removal of the metal from the soil. Taking the log of the equation,
log K is the coefficient of the adsorption based on the isotherm, where
The r physicochemical and proximate analyses of Cannabis sativa biochar are presented in Table 2, indicating ash content of around 3.45% with electrical conductivity (EC) of 110.5 μS/cm. The results also indicated a bulk density of 0.163 g/cm3 that can improve soil aeration, and the conversion efficiency was 37.94%. Cannabis sativa prepared biochar had a fixed carbon (or residual matter) concentration of 53.64%, while it had a volatile content (mobile matter) of 37.91%.
The surface profile of the prepared biochar indicated through SEM was rough, cracked, and porous. The biochar had sufficient longitudinal pore structures with sizes ranging from 0.70 μm to 3.33 μm. The elemental composition of the prepared biochar was determined using energy dispersive X-ray spectroscopy (EDX) by observing peaks of carbon ©, oxygen (O), potassium (K), calcium (Ca), and chlorine (Cl). The energy dispersive X-ray spectroscopy spectrum of Cannabis sativa biochar is presented in Figure 1, while their percentages along with other identified elements are given in Table 3. The carbon percentage was highest (75.3%), followed by oxygen (19.2%), calcium (3.9%), potassium (1.5%), and chlorine (0.08%), which indicated that the biochar was carbonaceous in nature. Apart from the EDX analysis, an inductively coupled plasma optical emission spectrometry (ICP-OES) analysis of Cannabis sativa biochar revealed the absence of lead and cadmium in the biochar; however, phosphorus was detected. Fourier transform infrared (FTIR) spectroscopy analysis was performed to investigate the surface functional groups in the prepared biochar presented in Figure 2, while the identified surface functional groups are presented in Table 4.
In both cases, with the addition of biochar at 1% (BC1) and 5% (BC5), an increase in pH was recorded. Notably, only a slight change in pH was observed in the control treatment over the first 10-day interval (7.71–7.79); however, it remained constant after that throughout the experimental period. In the case of BC1, pH increased from 7.83 to 7.98, showing a 2% increase; whereas, in the case of BC5, pH increased from 7.81 to 7.94, showing a 1.6% increase over the successive 30-day period of incubation. (Figure 3). Considering the EC of the soil, it was found that the addition of BC1 and BC5 caused an increase in EC by 27.08% and 23.51% by the end of incubation.
FIGURE 3. (A) pH trend of control and biochar amended soils over 1 month period (B) EC trend of control and biochar amended soils over 1 month period.
As expected, the addition of 5% biochar considerably increased OC from 1.53 to 2% and TO from 2.04 to 2.66%, whereas OM increased from 3.52 to 4.59% (Figures 4A–C respectively). Meanwhile, the application of biochar at 1% increased OC from 1.51 to 1.74%, TOC increased from 2.01 to 2.32%, and OM increased from 3.5 to 4%. In contrast to control, BC5 exhibited 189.86% increase in OC, 189.13% increase in TOC, and 188.68% increase in OM, while BC1 showed 152.17% increase in OC and TOC and 151.57% increase in OM.
FIGURE 4. (A) Organic carbon (OC), (B) Total Organic Carbon (TOC), (C) Organic Matter (OM) trend of control and biochar amended soils over 1 month period.
Biochar-treated soils presented a notable improvement in soil available nitrogen fraction (Figure 5A). At 1% biochar (w/w), AN increased from 0.14 ppm to 0.52 ppm, showing a 271.4% increase, whereas the addition of 5% biochar (w/w) increased the AN from 0.16 ppm to 0.6 ppm, indicating a 275% increase. However, as compared to the control, BC1 showed 188.8% increase in AN, while BC5 showed 233.3% increase in AN. SAP increased from 5.28 ppm to 13.56 ppm over 30 days experimental period in BC5, showing a 156.82% increase, whereas SAP increased from 5.23 ppm to 10.08 ppm in BC1 treatment over the experimental time frame, indicating a 92.73% increase in concentration (Figure 6). As compared to the control, BC1 showed a 50% increase in SAP, whereas a 101.79% improvement was observed for BC5.
FIGURE 5. (A) Soil available nitrogen (SAN) (B) Soil available phosphorous (SAP) trend of control and biochar amended soils over 1 month period.
FIGURE 6. (A) Lead (Pb) concentration (B) Cadmium (Cd) trend of control and biochar amended soils over 1 month period.
Concentrations of lead (Pb) in control, BC1, and BC5 are presented in Figure 6A. During the experimental period, Pb concentration in control was observed to increase with time ranging from 3.97 ppm to 5.64 ppm, indicating a 42.07% increase. However, comparatively, both biochar treatments showed reductions in Pb concentrations. In BC1, lead concentration (ppm) the lead concentration decreased as 3.91 > 3.57 > 3.26 > 3.06. In comparison with the control, BC1 showed a reduction in Pb concentration by 0.06 (1.51%), 0.75 (17.36%), 1.66 (33.74%), and 2.58 (45.74%) ppm at 0- , 10-, 20-, and 30-day intervals, respectively. In BC5 treatment, lead concentration (ppm) decreased over the entire time of 30 days in the following order: 3.87 > 3.63 > 3.58 > 3.43, whereas Pb concentration decreased by 0.24 ppm (6.2%), 0.05 ppm (1.38%), and 0.15 ppm (4.19%) during the first, second, and third intervals, respectively. In comparison with the control, BC5 showed a reduction in Pb concentration by 0.1 (2.52%), 0.69 (15.97%), 1.34 (27.24%), and 2.21 (39.18%) ppm at 0- , 10-, 20-, and 30-day intervals, respectively.
Similarly, both biochar amendments effectively reduced the Cd concentration in the soil as compared to the control (Figure 6B). Over the 1-month experimental duration, Cd concentration in control was observed to increase with time ranging from 0.55 ppm to 0.76 ppm, showing a 38.18% increase in concentration. However, in BC1, cadmium concentration (ppm) decreased in the following order: 0.52 > 0.46 > 0.38 > 0.33. Cadmium concentrations decreased by 0.06 ppm (11.54%), 0.08 ppm (17.39%), and 0.05 ppm (13.16%) during the first, second, and third intervals, respectively. As compared to control, BC1 showed a reduction in Cd concentration by 0.03 ppm (5.45%), 0.15 ppm (24.59%), 0.31 ppm (44.93%), and 0.43 ppm (56.58%) at 0-, 10-, 20-, and 30-day intervals, respectively. In case of BC5, Cd concentration (ppm) decreased over the entire 30 days pot experiment in the following order: 0.54 > 0.50 > 0.47 > 0.42. Cadmium concentration decreased by 0.04 ppm (7.41%), 0.03 ppm (6%), and 0.05 ppm (10.64%) at the first, second, and third intervals, respectively. In comparison with the control, BC5 also showed a reduction in Cd concentration by 0.01 ppm (1.82%), 0.11 ppm (18.03%), 0.22 ppm (31.88%), and 0.29 ppm (38.16%) at 0-, 10-, 20-t, and 30- day, respectively.
The adsorption isotherms were obtained for a constant ambient temperature of 24°C (±2) and concentration of the biochar applied, considering the pH as fluctuating and controlling factor. It was found that at 1% biochar application, the intercept value for cadmium was negative, indicating the low adsorption at the lower pH of cadmium. Overall, the intercept values for lead and cadmium were 1.9006 and 0.012, respectively, keeping the constant concentration of biochar at1%. Interestingly, at a 5% biochar application rate, the intercept values were 1.02 and 0.052 for lead and cadmium, respectively. The linear fitted slope indicated a constant distribution of adsorbent over the pH change (Figure 7).
FIGURE 7. Adsorption isotherm for heavy metal removal from the solid phase i:e soil using biochar as adsorbent [(A): 1% BC and Lead; (B): 1% BC and Cadmium; (C): 5% biochar and lead and (D): 5% biochar and cadmium].
Cannabis sativa (industrial hemp) is an invasive plant species found abundantly in Islamabad (Khan et al., 2010). The plant biomass pyrolyzed at 450°C showed a high pH value of 9.22, indicating its alkaline nature. These findings were in line with previous studies stating that an increase in temperature results in a higher pH of biochar (Al-Wabel et al., 2017; Poo et al., 2018). A temperature range of 200°C to 300°C causes cellulosic materials to decompose, resulting in alcoholic and phenolic substances (-OH containing substances) that impart alkalinity to biochar. Moreover, at temperatures above 300°C, alkaline minerals begin to disengage from the organic matrix, resulting in increased pH (Poo et al., 2018; Waqas et al., 2018). Identical to Cannabis sativa biochar, alkaline biochar was also obtained as a result of using woody materials, Lantana camara and Cladophora patentiramea (algal specie) (Bird et al., 2011; Qadeer et al., 2014; Rees et al., 2014). Biochar derived from Conocarpus tree species waste at 400°C ± 10°C was also reported to have a pH of 9.85 (Al-Wabel et al., 2015).
The ash content was subjected to pyrolysis temperature because an increase in temperature resulted in higher ash content since the minerals and combustion residues of organic matter become concentrated at high temperatures (Ahmad et al., 2012). High ash content in biochar is generally undesirable at higher temperatures, and metal volatilization can result in serious issues of air pollution. Moreover, it also hampers the development of aromatic carbon structures (Enders et al., 2012). Electrical conductivity is based on the ash content of biochar, such that higher ash content can impart a higher EC of biochar (Ahmad et al., 2017). Most of the volatiles are removed because of pyrolysis, leaving behind exchangeable cations such as Ca+2 and Na+, which concentrate and, thereby, increase the pH and EC of biochar (Waqas et al., 2018). Ahmad et al. (2017) reported an EC of 150 μS/cm for biochar produced at 300°C from peanut shells. A very low EC of 0.09 μS/cm was obtained by Pourret and Houben (2018) for a sewage sludge biochar prepared at 500°C.
Low bulk density is desirable for plant growth, with the ideal bulk density being <1.6 g/cm3. The addition of biochar lowers the bulk density of the soil and, thus, improves soil aeration (Reverchon et al., 2014; Tan et al., 2018). Low bulk density can enhance soil quality as it aerates the soil, making it more effective for holding water, which, in turn, promotes better growth and development of the plant root system (Hossain et al., 2010). As bulk density is inversely proportional to organic matter and porosity (Khater, 2015; Juma et al., 2018), the obtained low bulk density of Cannabis sativa biochar (0.163 g/cm3) indicated high porosity and organic matter in biochar. This porous nature is further confirmed by SEM of the biochar indicating up to 3.33 µm pore length (Figure 8).
FIGURE 8. Scanning Electron Microscopy (SEM) images of Cannabis sativa biochar at different magnifications.
The obtained conversion efficiency of 37.94% is more than usually expected (∼35%) during slow pyrolysis (Sohi et al., 2010; Ahmad et al., 2014). Since the conversion efficiency of biochar is inversely proportional to the temperature, thus, the current noticeable conversion efficiency can be explained by the low pyrolysis temperature (Sohi et al., 2010; Hernandez-Mena et al., 2014; Poo et al., 2018). The maximum biomass loss occurs when the hemicelluloses degrade at a temperature range of 250°C to 350°C. Cellulosic components of the biomass begin to decompose at temperatures ranging from 325°C to 400°C, while lignin structures start to degrade at temperatures ranging from 300°C to 550°C (Poo et al., 2018). This indicates that most of the compounds retained in the biomass could be related to lignin-related compounds, followed by cellulosic compounds. The same can be related to the functional group analysis of the biochar that indicated aliphatic and aromatic stretching of the biochar (Table 4). However, in addition to pyrolysis temperature, the type of feedstock utilized for biochar production has an impact on biochar properties, including its yield (Uchimiya et al., 2010; Spokas et al., 2012; Ahmad et al., 2017; Sandhu et al., 2017; Grutzmacher et al., 2018). It was also found that temperatures higher than 450°C can lead to the degradation of lignin (Li Y. et al., 2021). Therefore, it is critical to control the process conditions like temperature and residence time to get an efficient biochar product (Ahmad et al., 2017).
Fixed carbon is a representative of the completely carbonized organic matter within the biochar, presenting its stability in the soil as it is subject to slow microbial and chemical decomposition, whereas volatile content provides a carbon source for the soil microflora and improves soil health (Ahmad et al., 2014; Juraszek and Piasecka, 2020; Medyńska-Juraszek and Ćwieląg-Piasecka, 2020). Volatile content is generally believed to reflect the stability of biochar, such that higher volatile content indicates low stability and lower volatile content points to the high stability of biochar (Waqas et al., 2018). This implies that Cannabis sativa biochar is more labile and, therefore, it contributes to enhancing the soil organic pool and plays an effective role in improving soil fertility. The higher fixed carbon, as compared to volatile carbon, can be recognized as a sustainable carbon pool in the soil. This is in contradiction to some previous studies where it was found that biochar with low fixed carbon can serve as a better source for increasing soil nutrient pool (Chaturvedi et al., 2021). The contradictory results can be explained by the fact that fixed carbon may not be readily available for increasing the soil carbon content. But it is a consistent source of slow carbon release in the soil. In a previous study, biochar was prepared from agricultural green waste at 450°C and reported a fixed carbon content of 14.1% and a volatile content of 7.6% (Waqas et al., 2018), providing more of a stable biochar, effective for carbon sequestration and mitigating climate change. Such differences in the physicochemical properties of biochar produced at the same pyrolysis temperature, i.e., 450°C, can be explained by the usage of different feedstocks and residence times (Sandhu et al., 2017; Grutzmacher et al., 2018). The volatile content of biochar is also indicative of nitrogen availability and, hence, plant growth (Enders et al., 2012).
Low-temperature pyrolysis (450°C) causes the thermal breakdown of hemicelluloses and cellulosic plant materials, thereby giving rise to large pore spaces that can serve as a habitat for soil microflora, which ultimately improves soil health (Pattnaik et al., 2018). This further explains the low bulk density of the prepared biochar. Hernandez-Mena et al. (2014) suggested that the formation of large pores is due to the vascular bundles of the raw plant material. Moreover, the longitudinal pore shape is the result of the distortion of the original porous structure of the plant during pyrolysis (Hardie et al., 2014), which helps vapors that were produced during pyrolysis to escape (Hernandez-Mena et al., 2014). This porous structure of biochar helps in retaining water and nutrients that can be beneficial for soil quality and soil microflora (Hamdani et al., 2017).
The elemental composition of biochar was greatly influenced by the composition of the feedstock from which it was derived and the pyrolysis temperature during its production (Enders et al., 2012). A previous study also reported carbon and oxygen percentages of 77.81 and 8.76%, respectively, for biochar produced at 450°C (Waqas et al., 2018). Biochar rich in carbon can play an important role in carbon sequestration and contaminant adsorption from soil (Hernandez-Mena et al., 2014). Surface functional groups play an important role in binding the metal cations; hence, heavy metals and other nutrient cations such as K+ and Mg+2 remain adsorbed to the biochar surface. This ensures heavy metal immobilization and reduced nutrient leaching in soil, both of which are desirable scenarios (Paz-Ferreiro et al., 2014; Bashir et al., 2018). However, this also comes with a critical point of fixing soil micronutrients inside the soil (Mikula et al., 2020).
The band at 3,422.13 cm-1 corresponded to O-H and H-bonded stretching of phenols and alcohols along with organic acids (Poo et al., 2018; Pourret and Houben, 2018). These may have appeared due to moisture within the samples (Chia et al., 2012). A peak at 2,921.32 cm-1 represented the aliphatic C-H stretching, indicating the presence of hemicellulose and cellulose and N-H stretching in the carbonyl group (Begum et al., 2016; Waqas et al., 2018). The peak observed at 1,613.48 cm-1 indicated the C=C and C=O bond in aromatic structures, imparting aromaticity to the biochar (Ahmad et al., 2012; Begum et al., 2016; Çaglar et al., 2018). The band at 1,430.98 cm-1 presented the C=O and N-H stretching in carbonyl and amine functional groups, respectively (Pattnaik et al., 2018), whereas the peak at 1,312.32 cm-1 indicated the presence of hemicelluloses through C-O stretching in esters and ethers (Armynah et al., 2018) and deformed O-H vibrations of alcohols and phenols (Angın, 2013).
The addition of biochar caused an increase in the pH that can be attributed to the alkalinity of biochar. Higher pyrolysis temperatures generally yield high pH biochar with more basic surface functional groups and reduced acidic groups, promoting an overall liming effect (Al-Wabel et al., 2017). Biochar was reported to carry basic cations such as Ca+, Mg+, Na+, and K+ that convert into hydroxides, oxides, and carbonates, resulting in increased alkalinity of the soil and, hence, its pH (Mehmood et al., 2018). Biochars were generally characterized by having a porous nature and large surface area. These attributes of biochar were the main reason for imparting a high cation exchange capacity (CEC) to biochar, which, in turn, resulted in higher soil pH. About 68% of the changes in soil pH following biochar addition was attributable to the property of high cation exchange capacity (Nigussie et al., 2012). Similarly, the increase of EC in the control can be attributed to native soil organic matter mineralization (Al-Wabel et al., 2017). The addition of biochar at BC1 and BC5 increased EC due to the ash inclusions that were present within the biochar, which contains soluble salts (Figure 4B). These salts imparted a higher soil EC when biochar was decomposed or oxidized (Al-Wabel et al., 2017; Ghorbani and Amirahmadi, 2018). In addition, hydrophobic compounds also leach from the biochar to alter the soil EC (Loper et al., 2010). Furthermore, increased soil EC can be linked with the ionic content of biochar (Ahmad et al., 2017). Previously, consistent findings were reported by Mehmood et al. (2018) over a period of 60 days. Similarly, many other researchers have reported an increase in soil pH (Hossain et al., 2010; Qadeer et al., 2014; Rees et al., 2014; Bashir et al., 2018; Rizwan et al., 2018) and soil EC as compared to the control on the addition of biochar to soil (Hossain et al., 2010; Rizwan et al., 2018).
The application of biochar primarily increased the organic matter, which improved soil organic carbon (Hamdani et al., 2017). The results of the current study are compatible with previous work and showed a rise in all types of carbon content (Qadeer et al., 2014; Manore et al., 2021). Oxidizable organic carbon, total organic carbon, and organic matter are interconnected, which implies that a change in one induces a change in the others. As a result, the application of biochar at 1% and 5% can be justified by the fact that biochar produced at low pyrolysis temperatures had a substantial volatile carbon content, (which can be characterized by high volatile carbon in the current study) which, being mobile in nature, replenishes soil carbon and serves as a source of energy for soil micro flora (Jindo et al., 2014). Therefore, since the fraction of volatile carbon is homogenous in biochar, increasing the concentration of biochar is a factor of high organic matter and oxidizable carbon in the soil. Biochar, being highly carbonaceous in nature, was mineralized by soil microbes over time, and this led to a 0.3–0.8% loss of total assimilated carbon from biochar within almost initial 2 months of biochar application. Biochar contains a labile carbon fraction that can leach easily and replenish soil carbon content, allowing soil biota to flourish and improve soil fertility. The decrease in organic carbon in control treatment may be attributed to the progressive decomposition of the already present organic matter in the soil (Al-Wabel et al., 2017). In addition, biochar application increased soil pH levels, causing the release of labile carbon fraction from biochar into the soil and, thus, increasing organic carbon lability (Li et al., 2018). Increased pH also induces chemical hydrolysis of already present soil organic matter (Ahmad et al., 2014), thereby, increasing the available carbon content in the soil. In addition, native soil carbon readily decomposed because of applying biochar (positive priming effect) because it enhanced the soil microbial community (Ahmad et al., 2014; Luo et al., 2018). Previously, many studies have documented that biochar application increased soil organic carbon (Bashir et al., 2018; Tan et al., 2018; Zheng et al., 2018).
Biochar can provide nutrients to the soil directly because it contains nitrogen, phosphorous, potassium, magnesium, and calcium (Nelson et al., 2011). Similar results of increased AN in response to biochar addition were reported by other studies (Ghorbani and Amirahmadi, 2018; Tan et al., 2018; Zheng et al., 2018). Progressive mineralization of biochar in soil releases inherent nutrients into the soil (Jain et al., 2018; Zheng et al., 2018). Moreover, enhanced pH due to alkaline minerals in biochar promotes the formation of NH3 (Liu et al., 2018), which takes part in the nitrification process because biochar addition stimulates the activity of nitrifying bacteria (Song et al., 2014), which increases AN. The positive priming effect of biochar, i.e., accelerating the decomposition of already present organic matter in the soil, can also be the cause of enhanced AN (Ahmad et al., 2014). In addition to increasing AN content, biochar can effectively adsorb nitrate and ammonium ions through surface functional groups; mainly the hydroxyl and carboxyl groups, thereby retaining their availability in soil over a longer period (Tan et al., 2018; Zheng et al., 2018). Phosphorous is a vital component of plant growth, and its availability can be affected by biochar (Reverchon et al., 2014). Increased SAP could be the result of the direct addition of biochar itself to the soil because of its inherent available nutrients, such as phosphorous and potassium (Qadeer et al., 2014; Zheng et al., 2018). The increase in SAP over the experimental period supports the finding that with an increase in biochar application rates, SAP was increased. Gradual increment in SAP over the 1-month period can also be attributed to the phosphorous compounds present in the ash content of biochar, as reported previously (Chen et al., 2019).
The use of biochar to stabilize heavy metals was a proven approach, and it was considered a low-cost and efficient solution to the heavy metal contamination problem (Poo et al., 2018; Lian et al., 2020). The decrease in metal concentration was observed in the current work. Park et al. (2011) reported similar results of reduced soil Pb and Cd concentrations upon applying chicken manure and green waste–derived biochar. Many other studies also reported reduced Cd concentrations in soils after biochar application (Abbas et al., 2017a; Rizwan et al., 2018; Van Poucke et al., 2018). Additionally, Fellet et al. (2011), Park et al. (2013), Lu et al. (2017), and Lian et al. (2020) also observed a reduction in heavy metals (Pb and Cd) concentration in soils treated with biochar. Biochar treatment of 1% (w/w) proved to be more effective in immobilizing lead as compared to the 5% biochar treatment (w/w), but overall, both biochar amendments were successful compared to the control in reducing the soil lead concentrations. In addition to the biodegradable fraction, much of the biochar was composed of the recalcitrant carbon fraction that ensures its sustenance in the soil for thousands of years. Furthermore, it is renowned for its ability to adsorb heavy metals, rendering them immobile (Al-Wabel et al., 2017; Shen et al., 2018; Lian et al., 2020; Tu et al., 2020). This can be achieved either through the formation of surface complexes (with surface functional groups) and/or precipitates (in the form of carbonates and phosphates) or through electrostatic attraction (Shen et al., 2018). Wang and Liu (2017) also reported the phenomenon of cation exchange (in which biochar-associated nutrient cations such as Na+, Mg+2, and Ca+2 were exchanged with soil heavy metal cations such as Pb+2 and Cd+2) and physical sorption to be involved in the immobilization of heavy metals. As for the current study, the reduction in the heavy metals (Pb and Cd) concentration could be attributed to the formation of Pb and Cd carbonates and phosphates (Rees et al., 2014; Mehmood et al., 2018). The ash content of biochar was accompanied by many different compounds, including phosphates, carbonates, and sulphates. These compounds play an important role in stabilizing the heavy metal cations in soil by precipitation of these contaminants (Paz-Ferreiro et al., 2014) in the form of insoluble metal carbonates (Al-Wabel et al., 2017). Moreover, increased pH due to the biochar addition could also be the cause of metal immobilization facilitated by hydrolysis. Adsorption is also an explanation for the metal stabilization scenario because biochar, being negatively charged, attracts the positively charged heavy metal cations (Mehmood et al., 2018). Various functional groups identified on biochar surface through SEM analysis also support the possibility of surface complexation of metals with the organic functional groups, rendering the heavy metals immobile in the form of organo-metal complexes (Al-Wabel et al., 2017; Mehmood et al., 2018; Qi et al., 2018). It was also found that the addition of biochar has a significant effect in increasing the soil microbial community that can aid in the stabilization and passivation of the metals through the inorganic ion transport mechanism (Lan et al., 2021).
The current study sheds light on the usage of organic value-added products to enhance soil fertility in addition to being an environmentally benign choice for remediation. Additionally, it offers a fresh perspective because biochar may be combined with various substrates and nanomaterials to increase its effectiveness and longevity in a system. In other words, biochar can be used as a growth medium for advantageous rhizobacteria, which, in turn, promote agriculture and soil health. It provides multiple ways to improve agriculture and full the food demand in a sustainable way.
The original contributions presented in the study are included in the article/Supplementary Material; further inquiries can be directed to the corresponding author.
AS and QM contributed to the conception and design of the study. ZA and Waqar-Un-Nisa executed the laboratory work and prepared the first draft of the manuscript. AS and SQ refined sections of the manuscript. LR provided a brief review and performed proofreading. All authors contributed to manuscript revision, read, and approved the submitted version.
The authors declare that the research was conducted in the absence of any commercial or financial relationships that could be construed as a potential conflict of interest.
All claims expressed in this article are solely those of the authors and do not necessarily represent those of their affiliated organizations, or those of the publisher, the editors, and the reviewers. Any product that may be evaluated in this article, or claim that may be made by its manufacturer, is not guaranteed or endorsed by the publisher.
Abbas, T., Rizwan, M., Ali, S., Adrees, M., Zia-ur-Rehman, M., Qayyum, M. F., et al. (2017b). Effect of biochar on alleviation of cadmium toxicity in wheat (Triticumaestivum L.) grown on Cd-contaminated saline soil. Environ. Sci. Poll. Res. 25 (26), 25668–25680.
Abbas, T., Rizwan, M., Ali, S., Zia-ur-Rehman, M., Qayyum, M. F., Abbas, F., et al. (2017a). Effect of biochar on cadmium bioavailability and uptake in wheat (Triticumaestivum L.) grown in a soil with aged contamination. Ecotoxicol. Environ. Saf. 140, 37–47. doi:10.1016/j.ecoenv.2017.02.028
Ahmad, M., Lee, S. S., Dou, X., Mohan, D., Sung, J. K., Yang, J. E., et al. (2012). Effects of pyrolysis temperature on soybean stover-and peanut shell-derived biochar properties and TCE adsorption in water. Bioresour. Technol. 118, 536–544. doi:10.1016/j.biortech.2012.05.042
Ahmad, M., Lee, S. S., Lee, S. E., Al-Wabel, M. I., Tsang, D. C., and Ok, Y. S. (2017). Biochar-induced changes in soil properties affected immobilization/mobilization of metals/metalloids in contaminated soils. J. Soils Sediments 17 (3), 717–730. doi:10.1007/s11368-015-1339-4
Ahmad, M., Rajapaksha, A. U., Lim, J. E., Zhang, M., Bolan, N., Mohan, D., et al. (2014). Biochar as a sorbent for contaminant management in soil and water: A review. Chemosphere 99, 19–33. doi:10.1016/j.chemosphere.2013.10.071
Al-Wabel, M. I., Usman, A. R. A., Al-Farraj, A. S., Ok, Y. S., Abduljabbar, A., Al-Faraj, A. I., et al. (2017). Date palm waste biochars alter a soil respiration, microbial biomass carbon, and heavy metal mobility in contaminated mined soil. Environ. Geochem. health, 41(4):1705–1722. doi:10.1007/s10653-017-9955-0
Al-Wabel, M. I., Usman, A. R., El-Naggar, A. H., Aly, A. A., Ibrahim, H. M., Elmaghraby, S., et al. (2015). Conocarpusbiochar as a soil amendment for reducing heavy metal availability and uptake by maize plants. Saudi J. Biol. Sci. 22 (4), 503–511. doi:10.1016/j.sjbs.2014.12.003
Angın, D. (2013). Effect of pyrolysis temperature and heating rate on biochar obtained from pyrolysis of safflower seed press cake. Bioresour. Technol. 128, 593–597. doi:10.1016/j.biortech.2012.10.150
Arif, M. S., Riaz, M., Shahzad, S. M., Yasmeen, T., Ashraf, M., Siddique, M., et al. (2018). Fresh and composted industrial sludge restore soil functions in surface soil of degraded agricultural land. Sci. Total Environ. 619, 517–527. doi:10.1016/j.scitotenv.2017.11.143
Armynah, B., Djafar, Z., Piarah, W. H., and Tahir, D. (2018). March.Analysis of chemical and physical properties of biochar from rice husk biomass. J. Phys. Conf. Ser. 979 (1), 012038.
ASTM D1762-84 ASTM International (2007). Standard test method for chemical analysis of wood charcoal. Pennsylvania: ASTM International. doi:10.1520/D1762-84R07
Barrow, C. J. (2012). Biochar: Potential for countering land degradation and for improving agriculture. Appl. Geogr. 34, 21–28. doi:10.1016/j.apgeog.2011.09.008
Bashir, S., Hussain, Q., Akmal, M., Riaz, M., Hu, H., Ijaz, S. S., et al. (2018). Sugarcane bagasse-derived biochar reduces the cadmium and chromium bioavailability to mash bean and enhances the microbial activity in contaminated soil. J. Soils Sediments 18 (3), 874–886. doi:10.1007/s11368-017-1796-z
Batool, A., Taj, S., Rashid, A., Khalid, A., Qadeer, S., Saleem, A. R., et al. (2015). Potential of soil amendments (Biochar and Gypsum) in increasing water use efficiency of Abelmoschus esculentus L. Moench. Front. Plant Sci. 6, 733. doi:10.3389/fpls.2015.00733
Begum, K. T. M., Alhaji, N. M. I., Ayeshamariam, A., Vidhya, V. S., and Jayachandran, M. (2016). Removal of Ni (II) ions on to Polymer Loaded Sawdust (PLSD)–Batch Adsorption studies. Fluid Mech. Open Acc. 3 (138), 2. doi:10.4172/2476-2296.1000138
Bird, M. I., Wurster, C. M., de Paula Silva, P. H., Bass, A. M., and De Nys, R. (2011). Algal biochar – production and properties. Bioresour. Technol. 102 (2), 1886–1891. doi:10.1016/j.biortech.2010.07.106
Çaglar, E., Donar, Y. O., Sinag, A., Birogul, İ., Bilge, S., Aydincak, K., et al. (2018). Adsorption of anionic and cationic dyes on biochars, produced by hydrothermal carbonization of waste biomass: Effect of surface functionalization and ionic strength. Turk. J. Chem. 42 (1), 86–99. doi:10.3906/kim-1704-12
Cao, X., Ma, L., Liang, Y., Gao, B., and Harris, W. (2011). Simultaneous immobilization of lead and atrazine in contaminated soils using dairy-manure biochar. Environ. Sci. Technol. 45 (11), 4884–4889. doi:10.1021/es103752u
Chaturvedi, S., Singh, S. V., Dhyani, V. C., Govindaraju, K., Vinu, R., and Mandal, S. (2021). Characterization, bioenergy value, and thermal stability of biochars derived from diverse agriculture and forestry lignocellulosic wastes. Biomass Convers. Biorefinery.
Chen, W., Meng, J., Han, X., Lan, Y., and Zhang, W. (2019). Past, present, and future of biochar. Biochar 1 (1), 75–87. doi:10.1007/s42773-019-00008-3
Chia, C. H., Gong, B., Joseph, S. D., Marjo, C. E., Munroe, P., and Rich, A. M. (2012). Imaging of mineral-enriched biochar by FTIR, Raman and SEM–EDX. Vib. Spectrosc. 62, 248–257. doi:10.1016/j.vibspec.2012.06.006
Choudhary, M., Datta, A., Jat, H. S., Yadav, A. K., Gathala, M. K., Sapkota, T. B., et al. (2018). Changes in soil biology under conservation agriculture based sustainable intensification of cereal systems in Indo-Gangetic Plains. Geoderma 313, 193–204. doi:10.1016/j.geoderma.2017.10.041
Dawood, S., Sen, T. K., and Phan, C. (2017). Synthesis and characterization of slow pyrolysis pine cone bio-char in the removal of organic and inorganic pollutants from aqueous solution by adsorption: Kinetic, equilibrium, mechanism and thermodynamic. Bioresour. Technol. 246, 76–81. doi:10.1016/j.biortech.2017.07.019
Ding, W., Dong, X., Ime, I. M., Gao, B., and Ma, L. Q. (2014). Pyrolytic temperatures impact lead sorption mechanisms by bagasse biochars. Chemosphere 105, 68–74. doi:10.1016/j.chemosphere.2013.12.042
Enders, A., Hanley, K., Whitman, T., Joseph, S., and Lehmann, J. (2012). Characterization of biochars to evaluate recalcitrance and agronomic performance. Bioresour. Technol. 114, 644–653. doi:10.1016/j.biortech.2012.03.022
Faiz, Y., Tufail, M., Javed, M. T., Chaudhry, M. M., and Naila-Siddique, (2009). Road dust pollution of Cd, Cu, Ni, Pb and Zn along Islamabad Expressway, Pakistan. Microchem. J. 92 (2), 186–192. doi:10.1016/j.microc.2009.03.009
Fellet, G., Marchiol, L., DelleVedove, G., and Peressotti, A. (2011). Application of biochar on mine tailings: Effects and perspectives for land reclamation. Chemosphere 83 (9), 1262–1267. doi:10.1016/j.chemosphere.2011.03.053
Gholizadeh, M., and Hu, X. (2021). Removal of heavy metals from soil with biochar composite: A critical review of the mechanism. J. Environ. Chem. Eng. 9 (5), 105830. doi:10.1016/j.jece.2021.105830
Ghorbani, M., and Amirahmadi, E. (2018). Effect of rice husk Biochar (RHB) on some of chemical properties of an acidic soil and the absorption of some nutrients. J. Appl. Sci. Environ. Manag. 22 (3), 313–317. doi:10.4314/jasem.v22i3.4
Grutzmacher, P., Puga, A. P., Bibar, M. P. S., Coscione, A. R., Packer, A. P., and de Andrade, C. A. (2018). Carbon stability and mitigation of fertilizer induced N 2 O emissions in soil amended with biochar. Sci. Total Environ. 625, 1459–1466. doi:10.1016/j.scitotenv.2017.12.196
Hamdani, S. A. F., Aon, M., Ali, L., Aslam, Z., Khalid, M., and Naveed, M. (2017). Application of dalbergia sissoo biochar enhanced wheat growth, yield and nutrient recovery under reduced fertilizer doses in calcareous soil. Pak. J. Agric. Sci. 54 (1), 107–115. doi:10.21162/pakjas/17.5102
Hamzenejad Taghlidabad, R., and Sepehr, E. (2018). Heavy metals immobilization in contaminated soil by grape-pruning-residue biochar. Arch. Agron. Soil Sci. 64 (8), 1041–1052. doi:10.1080/03650340.2017.1407872
Han, X., Liang, C. F., Li, T. Q., Wang, K., Huang, H. G., and Yang, X. E. (2013). Simultaneous removal of cadmium and sulfamethoxazole from aqueous solution by rice straw biochar. J. Zhejiang Univ. Sci. B 14 (7), 640–649. doi:10.1631/jzus.b1200353
Hardie, M., Clothier, B., Bound, S., Oliver, G., and Close, D. (2014). Does biochar influence soil physical properties and soil water availability? Plant Soil 376 (1-2), 347–361. doi:10.1007/s11104-013-1980-x
Hernandez-Mena, L. E., Pécoraa, A. A., and Beraldob, A. L. (2014). Slow pyrolysis of bamboo biomass: Analysis of biochar properties. Chem. Eng. 37.
Hossain, M. K., Strezov, V., Chan, K. Y., and Nelson, P. F. (2010). Agronomic properties of wastewater sludge biochar and bioavailability of metals in production of cherry tomato (Lycopersiconesculentum). Chemosphere 78 (9), 1167–1171. doi:10.1016/j.chemosphere.2010.01.009
Iheanacho, S. C., Nworu, S. A., Ogueji, E. O., Nnatuanya, I., Mbah, C. E., Anosike, F., et al. (2017). Comparative assessment of proximate content and organoleptic quality of African catfish (Clarias gariepinus) processed by smoking and solar drying methods. Afr. J. Agric. Res. 12 (38), 2824–2829. doi:10.5897/ajar2017.12599
Jain, M. S., Jambhulkar, R., and Kalamdhad, A. S. (2018). Biochar amendment for batch composting of nitrogen rich organic waste: Effect on degradation kinetics, composting physics and nutritional properties. Bioresour. Technol. 253, 204–213. doi:10.1016/j.biortech.2018.01.038
Jindo, K., Mizumoto, H., Sawada, Y., Sanchez-Monedero, M. A., and Sonoki, T. (2014). Physical and chemical characterization of biochars derived from different agricultural residues. Biogeosciences 11 (23), 6613–6621. doi:10.5194/bg-11-6613-2014
Juma, K. N., Nakhone, L., Musandu, A. A. O., Nyalala, S., and Ogendo, J. O. (2018). Availability of nitrogen, microbial respiration and bulk density as influenced by Faecal matter Fertiliser in Acrisol, Andosol and Planosol. J. Soil Sci. Plant Health 2 (1), 15–25.
Juraszek, A. M., Piasecka, I. Ć., Guan, F., Liu, Y., Sun, Y., and Luo, Y. (2020). Biochar and bacteria inoculated biochar enhanced Cd and Cu immobilization and enzymatic activity in a polluted soil. Environ. Int. 137, 105576. doi:10.1016/j.envint.2020.105576
Kavitha, B., Reddy, P. V. L., Kim, B., Lee, S. S., Pandey, S. K., and Kim, K. H. (2018). Benefits and limitations of biochar amendment in agricultural soils: A review. J. Environ. Manage. 227, 146–154. doi:10.1016/j.jenvman.2018.08.082
Khalkhaliani, Z. N., Mesdaghinia, A. R., Mahvi, A., Nouri, J., and Vaezi, F. (2006). An experimental study of heavy metal extraction, using various concentration of EDTA in a sandy loam soils. Pak. J. Biol. Sci. 9 (5), 837–842. doi:10.3923/pjbs.2006.837.842
Khater, E. (2015). Some physical and chemical properties of compost. Intl J. Waste Res. 5 (01). doi:10.4172/2252-5211.1000172
Lam, S. S., Liew, R. K., Cheng, C. K., Rasit, N., Ooi, C. K., Ma, N. L., et al. (2018). Pyrolysis production of fruit peel biochar for potential use in treatment of palm oil mill effluent. J. Environ. Manage. 213, 400–408. doi:10.1016/j.jenvman.2018.02.092
Lan, J., Zhang, S., Dong, Y., Li, J., Li, S., Feng, L., et al. (2021). Stabilization and passivation of multiple heavy metals in soil facilitating by pinecone-based biochar: Mechanisms and microbial community evolution. J. Hazard. Mat. 420, 126588. doi:10.1016/j.jhazmat.2021.126588
Li, F., Zhao, H., Shao, R., Zhang, X., and Yu, H. (2021). Enhanced Fenton reaction for xenobiotic compounds and lignin degradation fueled by quinone redox cycling by lytic polysaccharide monooxygenases. J. Agric. Food Chem. 69 (25), 7104–7114. doi:10.1021/acs.jafc.1c01684
Li, G., Khan, S., Ibrahim, M., Sun, T. R., Tang, J. F., Cotner, J. B., et al. (2018). Biochars induced modification of dissolved organic matter (DOM) in soil and its impact on mobility and bioaccumulation of arsenic and cadmium. J. Hazard. Mat. 348, 100–108. doi:10.1016/j.jhazmat.2018.01.031
Li, Y., Yu, H., Liu, L., and Yu, H. (2021). Application of co-pyrolysis biochar for the adsorption and immobilization of heavy metals in contaminated environmental substrates. J. Hazard. Mater. 420, 126655. doi:10.1016/j.jhazmat.2021.126655
Lian, W., Yang, L., Joseph, S., Shi, W., Bian, R., Zheng, J., et al. (2020). Utilization of biochar produced from invasive plant species to efficiently adsorb Cd (II) and Pb (II). Bioresour. Technol. 317, 124011. doi:10.1016/j.biortech.2020.124011
Liu, Q., Zhang, Y., Liu, B., Amonette, J. E., Lin, Z., Liu, G., et al. (2018). How does biochar influence soil N cycle? A meta-analysis. Plant Soil 426, 211–225. doi:10.1007/s11104-018-3619-4
Loper, S., Shober, A. L., Wiese, C., Denny, G. C., Stanley, C. D., and Gilman, E. F. (2010). Organic soil amendment and tillage affect soil quality and plant performance in simulated residential landscapes. HortScience 45 (10), 1522–1528. doi:10.21273/hortsci.45.10.1522
Lu, K., Yang, X., Gielen, G., Bolan, N., Ok, Y. S., Niazi, N. K., et al. (2017). Effect of bamboo and rice straw biochars on the mobility and redistribution of heavy metals (Cd, Cu, Pb and Zn) in contaminated soil. J. Environ. Manage. 186, 285–292. doi:10.1016/j.jenvman.2016.05.068
Luo, Y., Lin, Q., Durenkamp, M., and Kuzyakov, Y. (2018). Does repeated biochar incorporation induce further soil priming effect? J. Soils Sediments 18 (1), 128–135. doi:10.1007/s11368-017-1705-5
Manori, S., Shah, V., Soni, V., Dutta, K., and Daverey, A. (2021). Phytoremediation of cadmium-contaminated soil by Bidens pilosa L. Impact of pine needle biochar amendment. Environ. Sci. Pollut. Res. 28 (42), 58872–58884. doi:10.1007/s11356-021-12953-3
Medyńska-Juraszek, A., and Ćwieląg-Piasecka, I. (2020). Effect of biochar application on heavy metal mobility in soils impacted by copper smelting processes. Pol. J. Environ. Stud. 29, 1749–1757. doi:10.15244/pjoes/108928
Mehmood, S., Rizwan, M., Bashir, S., Ditta, A., Aziz, O., Yong, L. Z., et al. (2018). Comparative effects of biochar, slag and ferrous–Mn ore on lead and cadmium immobilization in soil. Bull. Environ. Contam. Toxicol. 100 (2), 286–292. doi:10.1007/s00128-017-2222-3
Mikula, K., Izydorczyk, G., Skrzypczak, D., Mironiuk, M., Moustakas, K., Krowiak, A. W., et al. (2020). Controlled release micronutrient fertilizers for precision agriculture – Areview. Sci. total Environ. 712, 136365. doi:10.1016/j.scitotenv.2019.136365
Mohanty, P., Nanda, S., Pant, K. K., Naik, S., Kozinski, J. A., and Dalai, A. K. (2013). Evaluation of the physiochemical development of biochars obtained from pyrolysis of wheat straw, timothy grass and pinewood: Effects of heating rate. J. Anal. Appl. Pyrolysis 104, 485–493. doi:10.1016/j.jaap.2013.05.022
Monroe, P. H. M., Barreto-Garcia, P. A. B., Barros, W. T., de Oliveira, F. G. R. B., and Pereira, M. G. (2021). Physical protection of soil organic carbon through aggregates in different land use systems in the semi-arid region of Brazil. J. Arid. Environ. 186, 104427. doi:10.1016/j.jaridenv.2020.104427
Naeem, M. A., Khalid, M., Aon, M., Abbas, G., Amjad, M., Murtaza, B., et al. (2018). Combined application of biochar with compost and fertilizer improves soil properties and grain yield of maize. J. Plant Nutr. 41 (1), 112–122. doi:10.1080/01904167.2017.1381734
Nelson, N. O., Agudelo, S. C., Yuan, W., and Gan, J. (2011). Nitrogen and phosphorus availability in biochar-amended soils. Soil Sci. 176 (5), 218–226. doi:10.1097/ss.0b013e3182171eac
Nigussie, A., Kissi, E., Misganaw, M., and Ambaw, G. (2012). Effect of biochar application on soil properties and nutrient uptake of lettuces (Lactuca sativa) grown in chromium polluted soils. American-Eurasian J. Agri. Environ. Sci. 12 (3), 369–376.
O'Shea, F. T., Cundy, A. B., and Spencer, K. L. (2018). The contaminant legacy from historic coastal landfills and their potential as sources of diffuse pollution. Mar. Pollut. Bull. 128, 446–455. doi:10.1016/j.marpolbul.2017.12.047
Omidi, A. H., Cheraghi, M., Lorestani, B., Sobhanardakani, S., and Jafari, A. (2020). The biochars prepared from cinnamon and cannabis as nature-friendly adsorbents for removal of Cd (II) ions from aqueous solutions. SN Appl. Sci. 2 (7), 1163–1213. doi:10.1007/s42452-020-2954-2
Park, J. H., Choppala, G. K., Bolan, N. S., Chung, J. W., and Chuasavathi, T. (2011). Biochar reduces the bioavailability and phytotoxicity of heavy metals. Plant Soil 348 (1-2), 439–451. doi:10.1007/s11104-011-0948-y
Park, J. H., Choppala, G., Lee, S. J., Bolan, N., Chung, J. W., and Edraki, M. (2013). Comparative sorption of Pb and Cd by biochars and its implication for metal immobilization in soils. Water Air Soil Pollut. 224 (12), 1711. doi:10.1007/s11270-013-1711-1
Park, J. H., Ok, Y. S., Kim, S. H., Cho, J. S., Heo, J. S., Delaune, R. D., et al. (2015). Evaluation of phosphorus adsorption capacity of sesame straw biochar on aqueous solution: Influence of activation methods and pyrolysis temperatures. Environ. Geochem. Health 37 (6), 969–983. doi:10.1007/s10653-015-9709-9
Pattnaik, D., Kumar, S., Bhuyan, S. K., and Mishra, S. C. (2018). March. Effect of carbonization temperatures on biochar formation of bamboo leaves IOP Conference Series: Materials Science and Engineering. IOP Conf. Ser. Mat. Sci. Eng. 338 (1), 012054. doi:10.1088/1757-899x/338/1/012054
Paz-Ferreiro, J., Lu, H., Fu, S., Méndez, A., and Gascó, G. (2014). Use of phytoremediation and biochar to remediate heavy metal polluted soils: A review. Solid earth. 5 (1), 65–75. doi:10.5194/se-5-65-2014
Poo, K. M., Son, E. B., Chang, J. S., Ren, X., Choi, Y. J., and Chae, K. J. (2018). Biochars derived from wasted marine macro-algae (Saccharina japonica and Sargassumfusiforme) and their potential for heavy metal removal in aqueous solution. J. Environ. Manage. 206, 364–372. doi:10.1016/j.jenvman.2017.10.056
Pourret, O., and Houben, D. (2018). Characterization of metal binding sites onto biochar using rare Earth elements as a fingerprint. Heliyon 4 (2), e00543. doi:10.1016/j.heliyon.2018.e00543
Qadeer, S., Batool, A., Rashid, A., Khalid, A., Samad, N., and Ghufran, M. A. (2014). Effectiveness of biochar in soil conditioning under simulated ecological conditions. Soil & Environ. 33 (2).
Qayyum, M. F., Rehman, M. Z., Ali, S., Rizwan, M., Naeem, A., Maqsood, M. A., et al. (2017). Residual effects of monoammonium phosphate, gypsum and elemental sulfur on cadmium phytoavailability and translocation from soil to wheat in an effluent irrigated field. Chemosphere 174, 515–523. doi:10.1016/j.chemosphere.2017.02.006
Qi, F., Lamb, D., Naidu, R., Bolan, N. S., Yan, Y., Ok, Y. S., et al. (2018). Cadmium solubility and bioavailability in soils amended with acidic and neutral biochar. Sci. Total Environ. 610, 1457–1466. doi:10.1016/j.scitotenv.2017.08.228
Rees, F., Simonnot, M. O., and Morel, J. L. (2014). Short‐term effects of biochar on soil heavy metal mobility are controlled by intra‐particle diffusion and soil pH increase. Eur. J. Soil Sci. 65 (1), 149–161. doi:10.1111/ejss.12107
Reverchon, F., Flicker, R. C., Yang, H., Yan, G., Xu, Z., Chen, C., et al. (2014). Changes in δ 15 N in a soil–plant system under different biocharfeedstocks and application rates. Biol. Fertil. Soils 50 (2), 275–283. doi:10.1007/s00374-013-0850-2
Rizwan, M., Ali, S., Abbas, T., Adrees, M., Zia-ur-Rehman, M., Ibrahim, M., et al. (2018). Residual effects of biochar on growth, photosynthesis and cadmium uptake in rice (Oryza sativa L.) under Cd stress with different water conditions. J. Environ. Manage. 206, 676–683. doi:10.1016/j.jenvman.2017.10.035
Sandhu, S. S., Ussiri, D. A., Kumar, S., Chintala, R., Papiernik, S. K., Malo, D. D., et al. (2017). Analyzing the impacts of three types of biochar on soil carbon fractions and physiochemical properties in a corn-soybean rotation. Chemosphere 184, 473–481. doi:10.1016/j.chemosphere.2017.05.165
Shen, Z., Hou, D., Zhao, B., Xu, W., Ok, Y. S., Bolan, N. S., et al. (2018). Stability of heavy metals in soil washing residue with and without biochar addition under accelerated ageing. Sci. Total Environ. 619, 185–193. doi:10.1016/j.scitotenv.2017.11.038
Shu, R., Dang, F., and Zhong, H. (2016). Effects of incorporating differently-treated rice straw on phytoavailability of methylmercury in soil. Chemosphere 145, 457–463. doi:10.1016/j.chemosphere.2015.11.037
Sohi, S. P., Krull, E., Lopez-Capel, E., and Bol, R. (2010). A review of biochar and its use and function in soil. Adv. Agron. 105, 47–82.
Song, Y., Zhang, X., Ma, B., Chang, S. X., and Gong, J. (2014). Biochar addition affected the dynamics of ammonia oxidizers and nitrification in microcosms of a coastal alkaline soil. Biol. Fertil. Soils 50 (2), 321–332. doi:10.1007/s00374-013-0857-8
Spokas, K. A., Cantrell, K. B., Novak, J. M., Archer, D. W., Ippolito, J. A., Collins, H. P., et al. (2012). Biochar: A synthesis of its agronomic impact beyond carbon sequestration. J. Environ. Qual. 41 (4), 973–989. doi:10.2134/jeq2011.0069
Tan, G., Wang, H., Xu, N., Liu, H., and Zhai, L. (2018). Biochar amendment with fertilizers increases peanut N uptake, alleviates soil N 2 O emissions without affecting NH 3 volatilization in field experiments. Environ. Sci. Poll. Res., 25, 8817–8826.
Tu, C., Wei, J., Guan, F., Liu, Y., Sun, Y., and Luo, Y. (2020). Biochar and bacteria inoculated biochar enhanced Cd and Cu immobilization and enzymatic activity in a polluted soil. Environ. Int. 137, 105576. doi:10.1016/j.envint.2020.105576
Uchimiya, M., Lima, I. M., Thomas Klasson, K., Chang, S., Wartelle, L. H., and Rodgers, J. E. (2010). Immobilization of heavy metal ions (CuII, CdII, NiII, and PbII) by broiler litter-derived biochars in water and soil. J. Agric. Food Chem. 58 (9), 5538–5544. doi:10.1021/jf9044217
Van Poucke, R., Ainsworth, J., Maeseele, M., Ok, Y. S., Meers, E., and Tack, F. M. G. (2018). Chemical stabilization of Cd-contaminated soil using biochar. Appl. Geochem. 88, 122–130. doi:10.1016/j.apgeochem.2017.09.001
Walkley, A., and Black, I. A. (1934). An examination of the Degtjareff method for determining soil organic matter, and a proposed modification of the chromic acid titration method. Soil Sci. 37 (1), 29–38. doi:10.1097/00010694-193401000-00003
Wang, Y., and Liu, R. (2017). Comparison of characteristics of twenty-one types of biochar and their ability to remove multi-heavy metals and methylene blue in solution. Fuel Process. Technol. 160, 55–63. doi:10.1016/j.fuproc.2017.02.019
Waqas, M., Nizami, A. S., Aburiazaiza, A. S., Barakat, M. A., Ismail, I. M. I., and Rashid, M. I. (2018). Optimization of food waste compost with the use of biochar. J. Environ. Manage. 216, 70–81. doi:10.1016/j.jenvman.2017.06.015
Wei, S., Zhu, M., Fan, X., Song, J., Li, K., Jia, W., et al. (2019). Influence of pyrolysis temperature and feedstock on carbon fractions of biochar produced from pyrolysis of rice straw, pine wood, pig manure and sewage sludge. Chemosphere 218, 624–631. doi:10.1016/j.chemosphere.2018.11.177
Wu, H., Lai, C., Zeng, G., Liang, J., Chen, J., Xu, J., et al. (2017). The interactions of composting and biochar and their implications for soil amendment and pollution remediation: A review. Crit. Rev. Biotechnol. 37 (6), 754–764. doi:10.1080/07388551.2016.1232696
Xu, X., Cao, X., Zhao, L., Wang, H., Yu, H., and Gao, B. (2013). Removal of Cu, Zn, and Cd from aqueous solutions by the dairy manure-derived biochar. Environ. Sci. Pollut. Res. 20 (1), 358–368. doi:10.1007/s11356-012-0873-5
Yang, W., Wang, Z., Song, S., Han, J., Chen, H., Wang, X., et al. (2019). Adsorption of copper (II) and lead (II) from seawater using hydrothermal biochar derived from Enteromorpha. Mar. Pollut. Bull. 149, 110586. doi:10.1016/j.marpolbul.2019.110586
Yang, X., Han, F., Xu, C., Jiang, S., Huang, L., Liu, L., et al. (2017). Effects of preparation methods on the morphology and properties of nanocellulose (NC) extracted from corn husk. Industrial Crops Prod. 109, 241–247. doi:10.1016/j.indcrop.2017.08.032
Keywords: biochar, pyrolysis, cannabis sativa, heavy metals, total carbon, soil nitrogen
Citation: Anjum Z, Min Q, Riaz L, Waqar-Un-Nisa , Qadeer S and Saleem AR (2022) Employment of Cannabis sativa biochar to improve soil nutrient pool and metal immobilization. Front. Environ. Sci. 10:1011820. doi: 10.3389/fenvs.2022.1011820
Received: 04 August 2022; Accepted: 21 September 2022;
Published: 11 October 2022.
Edited by:
Muhammad Azeem, Ningbo Urban Environment Observation and Station, Institute of Urban Environment (CAS), ChinaReviewed by:
Muhammad Rahil Afzal, Jiangsu University, ChinaCopyright © 2022 Anjum, Min, Riaz, Waqar-Un-Nisa, Qadeer and Saleem. This is an open-access article distributed under the terms of the Creative Commons Attribution License (CC BY). The use, distribution or reproduction in other forums is permitted, provided the original author(s) and the copyright owner(s) are credited and that the original publication in this journal is cited, in accordance with accepted academic practice. No use, distribution or reproduction is permitted which does not comply with these terms.
*Correspondence: Aansa Rukya Saleem, cnVrYXl5YTU4M0BnbWFpbC5jb20=
†These authors have contributed equally to this work
Disclaimer: All claims expressed in this article are solely those of the authors and do not necessarily represent those of their affiliated organizations, or those of the publisher, the editors and the reviewers. Any product that may be evaluated in this article or claim that may be made by its manufacturer is not guaranteed or endorsed by the publisher.
Research integrity at Frontiers
Learn more about the work of our research integrity team to safeguard the quality of each article we publish.