- 1Nevada Business Unit, The Nature Conservancy, Reno, NV, United States
- 2Oregon Business Unit, The Nature Conservancy, Bend, OR, United States
Increased groundwater demand is causing aquifer declines that impact viability of groundwater-dependent ecosystems (GDEs) like springs and phreatophyte communities. To understand which springs and phreatophyte communities may be stressed by groundwater level declines in Oregon and Nevada, we assessed groundwater level trends in nearby monitoring wells. Very few springs and phreatophyte communities were near monitoring wells with adequate data. Less than 1% of >50,000 springs in Nevada and Oregon were within 800 m of analyzed wells, and only 52 springs were near a shallow (<30 m below ground surface) well. Among springs near analyzed wells, 56% in Nevada and 29% in Oregon were near wells with declining groundwater level trends, and percentages were similar among springs that were within 800 m of analyzed shallow wells. Less than 22% of all phreatophyte communities in Nevada and Oregon were near analyzed wells, and only 9.6% were within 800 m of a shallow well. Of phreatophyte communities near analyzed wells, 48% and 57% were near wells with declining trends in Nevada and Oregon, respectively. Differences among GDE types could reflect more groundwater development where phreatophytes exist. Differences between states in proportion of springs near wells with declining trends could be due to more surface water capture in Oregon or increased pressure for groundwater development in Nevada. State-specific policies and administration of groundwater rights and monitoring affect data availability and trends observed in the two states. More groundwater level data are essential for understanding impacts of groundwater withdrawals to GDEs.
1 Introduction
Groundwater is increasingly relied upon by humans as surface water supplies become more limited due to overuse and climate change (Grantham and Viers 2014; de Graaf et al., 2019). These limitations are especially evident in semi-arid and arid regions where 39% of total global groundwater withdrawals occur, but only 2% of total global groundwater recharge occurs (Wada et al., 2010). Groundwater overuse has led to groundwater depletion and water table declines in the United States and across the world (Konikow 2015; Perrone and Jasechko 2017). Water table declines can reduce the ability of plants and wildlife to access groundwater, affecting their long-term ecosystem viability and the important services they provide to human communities such as drinking water, agriculture, water quality improvements, and recreation (Brown et al., 2011). Groundwater-dependent ecosystems (GDEs) like springs and phreatophyte communities rely on groundwater for their structure, composition, and function (Kløve et al., 2011) and can act as ecological refugia due to their climate-buffering capacity (Cartwright et al., 2020). Despite their climate resilience, aquifers can be threatened by groundwater overuse (Taylor et al., 2013). The impact of reduced groundwater availability can be especially profound for nature in arid regions, where groundwater is essential as an often-perennial source of water for wildlife for large distances around desert springs, rivers and streams (Hjort et al., 2015), and rare and sensitive species adapted to these isolated water bodies may not be able to persist elsewhere (Wolaver et al., 2020).
An indicator of reduced access to groundwater for both people and nature is declining groundwater levels in monitoring wells. Perrone and Jasechko (2017) found that 3.3% of the wells they analyzed in 2013–2015 in the western US were dry, and well depths have been deepened over time across much of the United States (Perrone and Jasechko 2019), implying that declining groundwater levels are affecting human access to water. Studies have also shown that floodplain and riparian vegetation are increasingly stressed as groundwater levels fall (Stromberg et al., 1992; González et al., 2012; Kath et al., 2014), and very small declines in groundwater levels can alter streamflow (de Graaf et al., 2019). Phreatophyte communities have been directly affected by groundwater level declines due to groundwater abstraction (e.g., Cooper et al., 2006; Patten et al., 2008) if the shallow groundwater table declines below the root zone more quickly than plant growth can respond (Naumburg et al., 2005). Groundwater levels may decline as a response to short- or long-term climate changes, increased groundwater extraction due to land use changes, increases to consumptive use, cumulative and chronic effects of pumping, and other reasons. Regardless of the cause of declines, hydrologic alteration associated with groundwater decline can affect GDEs (Kath et al., 2018). However, the distribution and abundance of GDEs like springs and phreatophytes that are potentially affected by groundwater declines is poorly understood.
In this article, we present an assessment of groundwater level trends over the past 2 decades (2002–2021) in Oregon and Nevada, two states in the western United States that have increasing pressures on limited water supplies. We relate groundwater level declines to the presence and abundance of springs and phreatophyte communities near monitoring wells to better understand how many of these GDEs may be threatened by hydrologic alteration. The two states combined have more than 50,000 springs and millions of hectares of phreatophyte communities that are dependent on groundwater. We examine the relation between declining groundwater trends and these GDEs that are essential to people, plants and wildlife.
2 Methods
Oregon and Nevada are climatologically and geologically diverse. Precipitation is highly seasonal in Oregon, with less than 10% occurring during summer months (Western Regional Climate Center 2013). Annual precipitation in Oregon ranges from less than 20 cm (8 inches) per year in the arid eastern side of the state to almost 500 cm (200 inches) per year in the western side (30-year normal; Oregon State University 2014). Aquifers in Oregon are primarily unconsolidated deposits or basalt rock ranging from Miocene to Pliocene and younger (Whitehead 1994). Undifferentiated and consolidated sedimentary aquifers can be found in coastal Oregon (Whitehead 1994). Nevada averages about 23 cm (9 inches) of precipitation across the state. Aquifers in the state are basin-fill, carbonate-rock, volcanic-rock, and volcanic- and sedimentary-rock aquifers, with most major groundwater development in basin-fill aquifers (Nevada Division of Water Planning 1999).
For this work, we used mapped data on GDEs in each state (Figure 1) as well as available groundwater level data from federal and state databases. Indicators of GDEs in Nevada were mapped in 2019 and included springs as point data and phreatophyte communities as polygons (Saito et al., 2020). As described in Saito et al. (2020), springs data for Nevada were obtained from the Springs Stewardship Institute. Nevada phreatophyte data were obtained from remotely sensed coverages provided by The Nature Conservancy for over 1.6 million ha (over four million ac) in Nevada; LANDFIRE (2014); and phreatophyte boundary polygons mapped by Minor et al. (2019). Spring locations in Oregon were mapped as a compilation of the National Hydrography Dataset (U.S. Geological Survey, 2019), the GTILO-2 dataset (Department of Geology and Mineral Industries (DOGAMI)), and two field surveys (Freed et al., 2019; Freed 2021), while phreatophyte communities in Oregon were mapped using field observations, landform classification, compound topographic index, and soil drainage data (Garcia et al., 2021a).
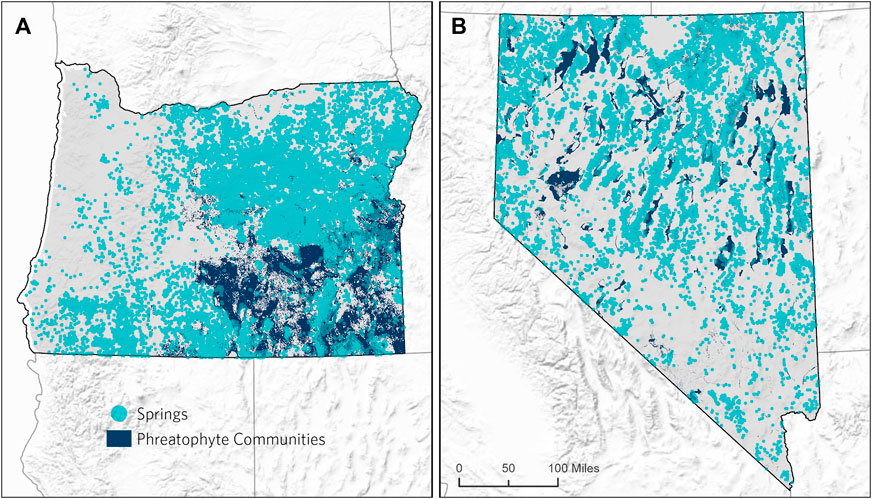
FIGURE 1. Statewide distribution of groundwater-dependent ecosystems assessed in this study in (A) Oregon (n = 29,379 springs and n = 682,136 ha phreatophyte communities) and (B) Nevada (n = 25,194 springs and n = 2,171,800 ha phreatophyte communities).
Groundwater data recorded between 2002 and 2021 were obtained from the US Geological Survey’s (USGS) National Water Information System (NWIS; https://waterdata.usgs.gov/nwis), the Nevada Division of Water Resources’ WellNet database (http://water.nv.gov/WaterLevelData.aspx), and the Oregon Water Resources Department Groundwater Information System (GWIS) database (https://apps.wrd.state.or.us/apps/gw/gw_info/gw_info_report/Default.aspx). If the same data were in more than one database, data from the database with the most recent data were used. USGS data were used when the same data were present in both USGS and state databases. Attribute data collected for all wells included water level measurements, dates of measurement, well depth, and monitoring well location. Monitoring well depths in Oregon range from 0.51 m (1.7 feet) below ground surface to more than 610 m (2000 feet) below ground surface. In Nevada well depths range from 1.77 m (5.8 feet) below ground surface to more than 2,130 m (7,000 feet) below ground surface.
Monitoring wells with pre-irrigation (February 1 to April 1) water level measurements among at least 5 years between 2002 and 2021 were included for analysis. When wells had multiple pre-irrigation water level measurements in the same year, the shallowest water level was used. Water level data were restricted to measurements between February and April to identify annual high groundwater levels while limiting the effects of pumping, which should result in near-static conditions for most wells. The irrigation season commonly begins by April in Oregon (Zach Freed, unpublished data) and Nevada (Wyatt Fereday, personal communication).
We analyzed trends for groundwater levels in wells that had at least 5 years of data with the Modified Mann-Kendall approach using the ‘modifiedmk’ package in R (Patakamuri and O’Brien 2021). Positive groundwater level trends that were significantly different than zero at p ≤ 0.05 indicated net recharge and increasing ecological groundwater availability, whereas negative trends that were significantly different than zero at p ≤ 0.05 indicated net groundwater loss and decreasing ecological groundwater availability.
Monitoring well data were considered to be representative of GDE aquifer conditions if the well was within 800 m (0.5 miles) of the spring or phreatophyte. The 800 m (0.5 mile) threshold was chosen based on prior publications (e.g., Garcia et al., 2021b) but does not necessarily mean that a monitoring well is representative of groundwater conditions for a given GDE. A total of 5,675 wells met criteria for analysis. We further distinguished shallow (within 30 m of ground surface; Rohde et al., 2021) monitoring wells that were more likely to be drawing water from shallow unconfined aquifers. Thus, two sets of monitoring wells were analyzed: all monitoring wells (n = 5,675) and shallow monitoring wells only (n = 464). Mapped spring and phreatophyte communities were associated with nearby monitoring wells and their trends using a spatial join (ESRI 2022). The number of springs and area (ha) of phreatophyte communities associated with monitoring wells that had declining groundwater level trends were summed.
3 Results
Combined among both Nevada and Oregon, there were 54,573 mapped springs of which 434 (0.8%) were within 800 m (0.5 mile) of analyzed wells. There are 2,853,936 ha of mapped phreatophytes total, and 627,724 ha (22%) were within 800 m (0.5 mile) of analyzed wells.
In Nevada, 33.0% of wells (620 out of 1,879) analyzed across the state had significantly falling trends in groundwater level, 14.4% had significantly rising trends, and the remainder did not have significant trends between 2002 and 2021 (Figure 2). Only 197 of 25,194 springs in Nevada were within 800 m (0.5 mile) of analyzed wells (Table 1). Of those 197 springs, 56.3% were near wells with declining trends. More than 518,000 ha of phreatophyte communities in Nevada were within 800 m (0.5 mile) of analyzed wells (Table 1), and 47.6% of that area was near wells with significantly declining trends.
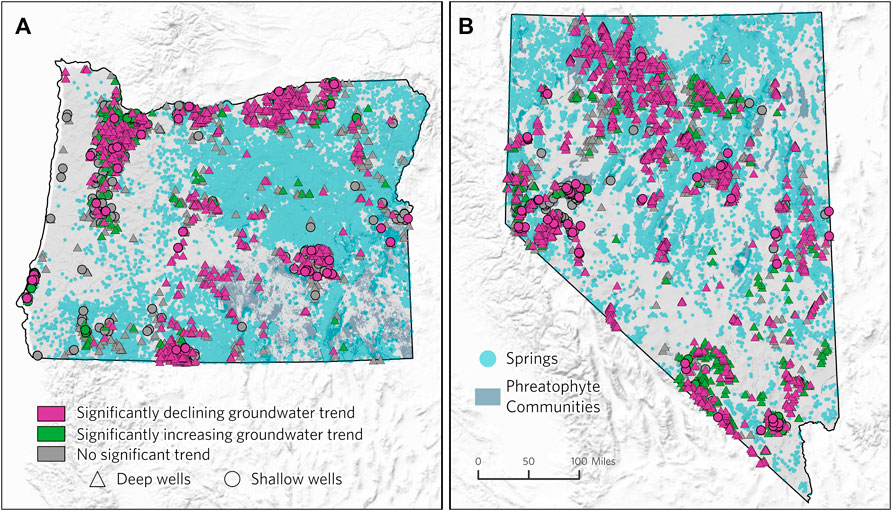
FIGURE 2. Map of statistically-significant groundwater trends among (A) 3,796 state and federal monitoring wells in Oregon and (B) 1,879 state and federal monitoring wells in Nevada. Statistical significance of Sen’s slope trends is assessed using a Modified Mann-Kendall test with p ≤ 0.05. Springs and phreatophyte communities are shown for context.
In Oregon, 27.2% of wells (1,032 out of 3,796) analyzed across the state had significantly falling trends in groundwater level, 9.2% were significantly rising, and 63.6% did not have significant trends between 2002 and 2021 (Figure 2). Only 237 of 29,379 springs in Oregon were within 800 m (0.5 mile) of analyzed wells (Table 1). Of those 237 springs, 29.1% were near wells with a declining trend. About 109,000 ha of phreatophyte communities in Oregon were within 800 m (0.5 mile) of analyzed wells (Table 1), and 57.0% of that area was near wells with significantly declining trends.
Nevada had 175 shallow monitoring wells, and 38 (27.7%) had significantly falling trends, 13 (7.4%) had significantly rising trends, and 124 (70.9%) did not have a significant trend. There were 28 springs in Nevada within 800 m (0.5 mile) of analyzed shallow wells, of which 57% (16 springs) were near shallow wells with a declining trend (Table 1). There were 250,334 ha of phreatophyte communities near analyzed shallow wells in Nevada, and 26% of them (64,427 ha) were near shallow wells with a declining trend. Among the 289 shallow monitoring wells in Oregon, 40 (13.8%) had significantly falling groundwater level trends, 29 (10.0%) had significantly rising trends, and the remaining 220 (76.1%) did not have a significant trend. In Oregon, eight of 24 springs (33%) within 800 m (0.5 mile) of analyzed shallow wells were near shallow wells with a declining trend. Only 23,761 ha of phreatophytes in Oregon were near analyzed shallow wells, and of those, 3,667 ha (15%) were near shallow wells with a declining trend. Nevada had more springs and phreatophyte community areas near shallow wells than Oregon, as well as slightly higher percentages of those GDEs near shallow wells with significantly falling trends, but there were relatively few shallow monitoring wells near springs or phreatophyte communities.
4 Discussion
Groundwater-dependent ecosystems are key components of ecological resilience to climate change but they are vulnerable to local and regional groundwater withdrawal. This analysis demonstrates that large proportions of springs and phreatophytes are in close proximity to groundwater declines and may be at risk of hydrologic alteration. Combined for both Oregon and Nevada, our results show that 42% of springs (n = 180 springs) and 49% of phreatophyte community area (n = 309,327 ha) within 800 m of a monitoring well were near wells with a declining groundwater level trend between 2002 and 2021.
Because it is possible in both states that wells may be present in shallow unconfined aquifers and deeper confined aquifers, we also considered only shallow wells in Oregon and Nevada and found that 46% of springs (n = 24 springs) and 25% of phreatophyte community area (n = 68,094 ha) that were within 800 m of a shallow well were near wells with a declining groundwater level trend. Groundwater level responses to pumping in confined aquifers is different than for unconfined aquifers (Alley et al., 1999), and springs can be fed from shallow aquifers or deeper confined aquifers (Cantonati et al., 2020). For springs near wells analyzed for our assessment, a much higher proportion of springs in Nevada (56%; n = 111) were near wells with groundwater decline than in Oregon (29%; n = 69). This pattern was consistent when considering only shallow wells: 57% (n = 16) of Nevada springs and 33% (n = 8) of Oregon springs were near shallow wells with groundwater decline. Oregon’s greater abundance of surface water sources and wetter climate may allow groundwater development near springs to be offset by surface water capture, keeping groundwater tables at higher levels near springs (Bredehoeft 2002). Additionally, Nevada’s drier climate could result in increased pressure for groundwater development compared to Oregon, leading to more widespread impacts to GDEs like springs.
In both states, the proportion of phreatophyte communities near analyzed shallow wells that were near wells with declining trends was less (26%, n = 64,427 ha for Nevada; 15%, n = 3,667 ha for Oregon) than when considering all analyzed wells with declining trends (48%, n = 247,191 ha for Nevada; 57%, 62,136 ha for Oregon). Phreatophyte viability can be affected when groundwater levels drop below the root zone (Naumberg et al., 2005) regardless of the groundwater source, so the higher percentages for all analyzed wells are concerning. Eamus et al. (2015) suggest that ecosystem structure and function of groundwater-dependent ecosystems can be affected when groundwater levels fall beyond about 7–10 m depth.
These results are broadly consistent with the 44% of all GDEs in California that were associated with significant groundwater declines between 1985 and 2019 (Rohde et al., 2021). That assessment used a random forest model to estimate groundwater levels wherever GDEs were located, whereas our assessment was dependent on only considering areas where adequate well data were available. Additionally, Rohde et al. (2021) used average annual measurements rather than pre-irrigation annual maximum groundwater levels. The use of pre-irrigation annual maximum groundwater levels allows the construction of interannual trends between consistent local maxima (following Garcia et al., 2021b), reducing the impact of seasonal drawdowns due to irrigation which vary based on the amount and timing of pumping and the hydrogeologic characteristics of the aquifer.
Groundwater level declines stress all GDEs, but the specific impacts vary by GDE type. With loss of groundwater discharge, spring flow may transition from perennial to intermittent before entirely drying, causing them to act as ecological traps rather than safe climate refugia (Cartwright et al., 2020). As shallow groundwater levels decline, groundwater that could have otherwise discharged to a spring may instead remain in the aquifer, offsetting rates of aquifer decline by up to the full amount of spring discharge (Bredehoeft 2002). Phreatophyte communities experience plant stress and mortality when the water table decreases (Naumberg et al., 2005). Loss of access to groundwater can lead to transitions of phreatophyte communities to ones with less ecological value. For example, black greasewood is the largest groundwater-dependent ecosystem by area in Nevada and is a good indicator of the presence of shallow groundwater (Lopes et al., 2006), and this phreatophyte is naturally fire-resistant but can transition to weedy, fire-prone vegetation if access to groundwater is lost (Provencher et al., 2020).
In Oregon and Nevada, 1,652 (29%) of the 5,675 analyzed monitoring wells were experiencing groundwater level declines from 2002 to 2021 (Figure 2), suggesting aquifer declines among both states. Long-term groundwater level trends are an indicator of sustainability and reflect changes in aquifer storage, which can occur as a result of decreased recharge and increased extraction, or both (Currell 2016). Groundwater is over-appropriated compared to available groundwater in aquifers of both states (e.g., Garcia et al., 2022; Saito et al., 2022), and pressure to develop groundwater will increase as crop evapotranspiration rates require more water to sustain current yields (Huntington et al., 2015; Albano et al., 2022). Projected increases to frequency and extent of drought (Ahmadalipour et al., 2016; Saito et al., 2022) could lead to more groundwater development as surface water availability becomes unreliable. As demand for groundwater withdrawal intensifies, future climate conditions will also affect groundwater recharge. Climate warming in the western United States is anticipated to shift winter precipitation from snow to rain, reducing the volume and persistence of snowpack (Knowles et al., 2006). This shift is expected to reduce high-elevation groundwater recharge (Meixner et al., 2016), creating further discrepancy between groundwater availability and demand. Springs and phreatophytes with shorter or shallower flow systems, such as those in low-permeability terranes, are likely more vulnerable to changes in recharge (Freed et al., 2019) because they lack the buffering capacity of greater aquifer storage in longer flow systems (Waibel et al., 2013).
More than a quarter of all analyzed monitoring wells in Oregon and Nevada were significantly declining between 2002 and 2021, indicating that more effective management is needed to ensure long-term sustainability in both states to conserve GDEs and protect groundwater resources for human uses. The management of groundwater is different in each state. Under Oregon water law, state agencies are required to maintain “reasonably stable” groundwater levels and aquifers must be protected from overdraft leading to “excessively declining” groundwater levels (Young 1991). Although “reasonably stable” is not well-defined, “excessive declines” are defined in rule § OAR 690-008-0001. One definition of “excessive declines” is any aquifer with an average downward trend of three feet (0.91 m) or more per year for at least 10 years, a criterion met by 108 monitoring wells assessed over 20 years in this study (Supplementary Table S1). Groundwater in Nevada is administered in 256 hydrographic areas with assigned “perennial yields” that indicate the amount of usable water that can be withdrawn and consumed without depleting the source (Nevada Division of Water Planning 1999). Most of these perennial yields were estimated in the 1950s–1970s and many were based on groundwater discharge using estimates of evaporation from playas and evapotranspiration of phreatophytic vegetation (Bredehoeft 2002). Thus, if groundwater use was at the perennial yield, GDEs could be progressively eliminated as water stored in the aquifer reaches a new equilibrium. Currently, about 50% of hydrographic areas in Nevada are over-appropriated, and almost 20% have more water withdrawn than the perennial yield (Sullivan 2022). In 2017, the Nevada Legislature declared that it is the policy of the state to administer groundwater and surface water together (§NRS 533.024) which should enable more consideration of groundwater withdrawals and GDE impacts, but a recent ruling on the Lower White River Flow System indicates the Nevada State Engineer may not have adequate authority to apply conjunctive management (LVVWD and SNWA v. Wilson 2022).
We found that existing monitoring wells are not equally present among GDE types: wells with a 20-year period of record were rarely co-located within 800 m of springs but were found within 800 m of a relatively large proportion of phreatophyte communities, and very few wells with adequate data near springs and phreatophyte communities were shallow (less than 30 m deep). Monitoring wells in Oregon and Nevada are much more abundant in valley-bottom areas where both groundwater extraction (Aldous and Gannett 2021) and phreatophyte communities (Garcia et al., 2021a) tend to be more common. It is likely that groundwater development is more prevalent where surface water resources like spring flow are unavailable. This disparity in available monitoring data between GDE types in extant wells emphasizes the need for additional investment from local, state and federal agencies to more effectively monitor groundwater levels near springs to understand groundwater availability and GDE sustainability.
Groundwater can provide ecological stability to groundwater dependent ecosystems under climate variability by providing fairly constant aquatic environments, enabling the evolution of assemblages of endemic taxa (Cantonati et al., 2020), but excessive groundwater withdrawals can dry springs and lead to the elimination of springs-dependent taxa (Williams and Sada 2021). Monitoring at appropriate temporal and spatial scales can provide early warning for impacts and can help resource managers make appropriate decisions to maintain sustainable yield, learn from management actions, and mitigate risk to GDEs (Saito et al., 2021). This study demonstrates that groundwater levels between 2002 and 2021 were frequently declining in Oregon and Nevada, and substantial proportions of springs and phreatophytes near monitoring wells could be impacted by the declines. Long-term continuous monitoring of spring discharge and phreatophyte community condition are needed to understand how their condition and ecosystem function is affected by groundwater level declines. Future research is needed to assess the impacts of groundwater level declines on springs, phreatophyte communities, and other GDE types such as groundwater-dependent rivers, lakes, and wetlands. Meanwhile, state agencies and resource managers must manage groundwater sustainably in a changing climate by reducing long-term overextraction of groundwater.
Data availability statement
The original contributions presented in the study are included in the article/Supplementary Material further inquiries can be directed to the corresponding author.
Author contributions
LS, SB, ZF, and MS contributed to the study methodology. LS and ZF conceptualized the study and wrote the original draft. SB provided analysis of NV data. ZF provided analysis of OR data. SB and MS contributed to the study’s data curation and map development. All authors have read and agreed to the published version of the manuscript.
Funding
The LS and SB was provided by Bureau of Land Management Cooperative Agreement L17AC00150. Funding for Freed and Schindel was provided by the Portland General Electric Salmon Habitat Support Fund grant 2021-07.
Acknowledgments
The authors thank Claire Ruffing and Shonene Scott from The Nature Conservancy in Oregon and Kevin Badik and Louis Provencher from The Nature Conservancy in Nevada for advising on well trend analysis and GDE locations. The authors thank two reviewers and the handling editor for their helpful comments to improve the manuscript.
Conflict of interest
The authors declare that the research was conducted in the absence of any commercial or financial relationships that could be construed as a potential conflict of interest.
Publisher’s note
All claims expressed in this article are solely those of the authors and do not necessarily represent those of their affiliated organizations, or those of the publisher, the editors and the reviewers. Any product that may be evaluated in this article, or claim that may be made by its manufacturer, is not guaranteed or endorsed by the publisher.
Supplementary material
The Supplementary Material for this article can be found online at: https://www.frontiersin.org/articles/10.3389/fenvs.2022.1007114/full#supplementary-material
References
Ahmadalipour, A., Moradkhani, H., and Svoboda, M. (2016). Centennial drought outlook over the CONUS using NASA-NEX downscaled climate ensemble. Int. J. Climatol. 37, 2477–2491. doi:10.1002/joc.4859
Albano, C. M., Abatzoglou, J. T., McEvoy, D. J., Huntington, J. L., Morton, C. O., Dettinger, M. D., et al. (2022). A multidataset assessment of climatic drivers and uncertainties of recent trends in evaporative demand across the continental United States. J. Hydrometeorol. 23, 505–519. doi:10.1175/JHM-D-21-0163.1
Aldous, A. R., and Gannett, M. W. (2021). Groundwater, biodiversity, and the role of flow system scale. Ecohydrology 14, e2342. doi:10.1002/eco.2342
Alley, W. M., Reilly, T. E., and Franke, O. L. (1999). Sustainability of ground-water resources. U.S. Geological Survey Circular 1186. Denver: U.S. Geological Survey. Available at https://pubs.usgs.gov/circ/circ1186/pdf/circ1186.pdf (accessed 9 10, 2022).
Bredehoeft, J. D. (2002). The water budget myth revisited: Why hydrogeologists model. Ground Water 49 (4), 340–345. doi:10.1111/j.1745-6584.2002.tb02511.x
Brown, J., Bach, L., Aldous, A., Wyers, A., and DeGagné, J. (2011). Groundwater-dependent ecosystems in Oregon: An assessment of their distribution and associated threats. Front. Ecol. Environ. 9, 97–102. doi:10.1890/090108
Cantonati, M., Poikane, S., Pringle, C. M., Stevens, L. E., Turak, E., Heino, J., et al. (2020). Characteristics, main impacts, and stewardship of natural and artificial freshwater environments: Consequences for biodiversity conservation. Water 12, 260. doi:10.3390/w12010260
Cartwright, J. M., Dwire, K. A., Freed, Z., Hammer, S. J., McLaughlin, B., Misztal, L. W., et al. (2020). Oases of the future? Springs as potential hydrologic refugia in drying climates. Front. Ecol. Environ. 18 (5), 245–253. doi:10.1002/fee.2191
Cooper, D. J., Sanderson, J. S., Stannard, D. I., and Groeneveld, D. P. (2006). Effects of long-term water table drawdown on evapotranspiration and vegetation in an arid region phreatophyte community. J. Hydrology 325, 21–34. doi:10.1016/j.jhydrol.2005.09.035
Currell, M. J. (2016). Drawdown “triggers”: A misguided strategy for protecting groundwater-fed streams and springs. Groundwater 54, 619–622. doi:10.1111/gwat.12425
de Graaf, I. E. M., Gleeson, T., van Beek, L. P. H., Sutanudjaja, E. H., and Bierkens, M. F. P. (2019). Environmental flow limits to global groundwater pumping. Nature 574, 90–94. doi:10.1038/s41586-019-1594-4
Department of Geology and Mineral Industries (DOGAMI) (2013). Geothermal information layer for Oregon (GTILO) – release 2. Salem, Oregon: Oregon Department of Geology and Mineral Industries. https://www.oregongeology.org/gtilo/index.htm (Accessed June 15, 2021).
Eamus, D., Zolfaghar, S., Villalobos-Vega, R., Cleverly, J., and Huete, A. (2015). Groundwater-dependent ecosystems: Recent insights from satellite and field-based studies. Hydrol. Earth Syst. Sci. 19, 4229–4256. doi:10.5194/hess-19-4229-2015
ESRI Inc (2022). ArcGIS pro. https://www.esri.com/en-us/arcgis/products/arcgis-pro/overview.
Freed, T. Z., Aldous, A., and Gannett, M. W. (2019). Landscape controls on the distribution and ecohydrology of central Oregon springs. Ecohydrology 12, e2065. doi:10.1002/eco.2065
Freed, Z. (2021). “Summary of groundwater-dependent ecosystems in the harney basin,” in The Harney County community-based water plan (Burns, OR), 42–47.
Garcia, C. A., Corson-Dosch, N. T., Beamer, J. P., Gingerich, S. B., Grondin, G. H., Overstreet, B. T., et al. (2022). Hydrologic budget of the Harney Basin groundwater system, southeastern Oregon. Reston, Virginia: U.S. Geological Survey Scientific Investigations, 144. Report 2021-5128. doi:10.3133/sir20215128
Garcia, C. A., Haynes, J. V., Herrera, N. B., and Gingerich, S. B. (2021b). Historical trends in shallow and deep groundwater levels by well and 8-digit hydrologic units (HUC8) (1990-2019, 2005-2019, and 2015-2019), GridMET precipitation and air temperature by HUC8 (1990-2019), and snow-water equivalent by station and HUC8 (1955-2016), statewide summaries, time-series graphs, and groundwater-well proximity to streams and cultivated crops, Oregon. Reston, VA: U.S. Geological Survey data release. doi:10.5066/P901GNIX
Garcia, C. A., Haynes, J. V., Herrera, N. B., and Gingerich, S. B. (2021a). Select phreatophytic shrub and grass species of Oregon, the estimated distribution of phreatophytic shrubland and grassland across Oregon, and field observations used to constrain mapped species distributions. U.S. Geol. Surv. data release. doi:10.5066/P901GNIX
González, E., González-Sanchis, M., Comín, F. A., and Muller, E. (2012). Hydrologic thresholds for riparian forest conservation in a regulated large Mediterranean river. River Res. applic. 28, 71–80. doi:10.1002/rra.1436
Grantham, T. E., and Viers, J. H. (2014). 100 years of California’s water rights system: Patterns, trends and uncertainty. Environ. Res. Lett. 9, 084012. doi:10.1088/1748-9326/9/8/084012
Hjort, J., Gordon, J. E., Gray, M., and Hunter, M. L. (2015). Why geodiversity matters in valuing nature’s stage. Conserv. Biol. 29, 630–639. doi:10.1111/cobi.12510
Huntington, J. L., Gangopadhyay, S., Spears, M., Allen, R., King, D., Morton, C., et al. (2015). West-wide climate risk assessments: Irrigation demand and reservoir evaporation projections: Washington DC, U.S. Bureau of Reclamation. Technical memorandum 68-68210-2014-01. 196p.
Kath, J., Reardon-Smith, K., Le Brocque, A. F., Dyer, F. J., Dafny, E., Fritz, L., et al. (2014). Groundwater decline and tree change in floodplain landscapes: Identifying non-linear threshold responses in canopy condition. Glob. Ecol. Conservation 2, 148–160. doi:10.1016/j.gecco.2014.09.002
Kløve, B., Pertti, A., Bertrand, G., Boukalova, Z., Widerlund, A., Goldscheider, N., et al. (2011). Groundwater dependent ecosystems. Part I: Hydroecological status and trends. Environ. Sci. Policy 14 (7), 770–781. doi:10.1016/j.envsci.2011.04.002
Knowles, N., Dettinger, M. D., and Cayan, D. R. (2006). Trends in snowfall versus rainfall in the Western United States. J. Clim. 19, 4545–4559. doi:10.1175/JCLI3850.1
Konikow, L. F. (2015). Long-term groundwater depletion in the United States. Groundwater 53, 2–9. doi:10.1111/gwat.12306
LANDFIRE (2014). Biophysical settings, LANDFIRE 1.4.0. Rapid City, SD: U.S. Department of Interior Geological Survey. www.landfire.gov (accessed 91722.
Lopes, T. J., Buto, S. G., Smith, J. L., and Welborn, T. L. (2006). Water-table levels and gradients: Carson City: U.S., Nevada 1947-2004. Scientific investigations report 2006-5100. Geological Survey. Available at https://pubs.usgs.gov/sir/2006/5100/pdf/SIR2006_5100.pdf (accessed 17 922.
Meixner, T., Manning, A. H., Stonestrom, D. A., Allen, D. M., Ajami, H., Blasch, K. W., et al. (2016). Implications of projected climate change for groundwater recharge in the Western United States. J. Hydrology 534, 124–138. doi:10.1016/j.jhydrol.2015.12.027
Minor, B. A., Huntington, J. L., and Bromley, M. (2019). Potential groundwater discharge boundaries and evapotranspiration units in Nevada, vers. 1.0. Reno: Desert Research Institute. Available at https://www.conservationgateway.org/ConservationByGeography/NorthAmerica/UnitedStates/nevada/water/Pages/database-collaboration.aspx (accessed 91722.
Naumburg, E., Mata-Gonzalez, R., Hunter, R. G., McLendon, T., and Martin, D. W. (2005). Phreatophytic vegetation and groundwater fluctuations: A review of current research and application of ecosystem response modeling with an emphasis on great basin vegetation. Environ. Manag. 35 (6), 726–740. doi:10.1007/s00267-004-0194-7
Nevada Division of Water Planning, (1999). Nevada state water plan. Carson City, Nevada: Nevada Department of Conservation and Natural Resources. Available at http://water.nv.gov/waterplandetail.aspx.accessed 7/23/22)
Oregon State University, (2014). PRISM climate group data. https://prism.oregonstate.edu (accessed September 19, 2022).
Patakamuri, S. K., and O’Brien, N. (2021). modifiedmk: Modified versions of Mann Kendall and Spearman’s Rho trend tests. R package version 1.6.0. https://CRAN.R-project.org/package=modifiedmk.
Patten, D. T., Rouse, L., and Stromberg, J. C. (2008). Isolated spring wetlands in the great basin and mojave deserts, USA: Potential response of vegetation to groundwater withdrawal. Environ. Manag. 41 (3), 398–413. doi:10.1007/s00267-007-9035-9
Perrone, D., and Jasechko, S. (2019). Deeper well drilling an unsustainable stopgap to groundwater depletion. Nat. Sustain. 2, 773–782. doi:10.1038/s41893-019-0325-z
Perrone, D., and Jasechko, S. (2017). Dry groundwater wells in the Western United States. Environ. Res. Lett. 12, 104002. doi:10.1088/1748-9326/aa8ac0
Provencher, L., Saito, L., Badik, K., and Byer, S. (2020). All systems are equal: In defense of undervalued ecosystems. Rangelands 42 (5), 159–167. doi:10.1016/j.rala.2020.07.002
Rohde, M. M., Biswas, T., Housman, I. W., Campbell, L. S., Klausmeyer, K. R., and Howard, J. K. (2021). A machine learning approach to predict groundwater levels in California reveals ecosystems at risk. Front. Earth Sci. 9, 784499. doi:10.3389/feart.2021.784499
Saito, L., Byer, S., Badik, K., McGwire, K., Provencher, L., and Minor, B. (2020). J. Nevada water resour. Assoc. Winter 2020, 48–72. Available at http://www.nvwra.org/journal (accessed 10 06, 22).Mapping indicators of groundwater dependent ecosystems in Nevada: Important resources for a water-limited state
Saito, L., Byer, S., Badik, K., Provencher, L., and McEvoy, D. (2022). Stressor and threat assessment of Nevada groundwater dependent ecosystems. Reno: The Nature Conservancy. Available at https://www.conservationgateway.org/ConservationByGeography/NorthAmerica/UnitedStates/nevada/water/Pages/database-collaboration.aspx (accessed 72322.
Saito, L., Christian, B., Diffley, J., Richter, H., Rohde, M. M., and Morrison, S. A. (2021). Managing groundwater to ensure ecosystem function. Groundwater 59, 322–333. doi:10.1111/gwat.13089
SNWA, V., and Wilson, L. (2022 Vegas Valley water district and southern Nevada water authority v. Wilson Clark county district court case. No. A-20-816761-C
Stromberg, J. C., Tress, J. A., Wilkins, S. D., and Clark, S. D. (1992). Response of velvet mesquite to groundwater decline. J. Arid Environ. 23, 45–58. doi:10.1016/s0140-1963(18)30540-8
Sullivan, A. (2022). Statewide water resource management issues. Presentation to joint interim standing committee on natural resources. Available at https://www.leg.state.nv.us/App/InterimCommittee/REL/Document/27034 (accessed 72322.
Taylor, R. G., Scanlon, B., Doll, P., Rodell, M., van Beek, R., Wada, Y., et al. (2013). Groundwater and climate change. Nat. Clim. Change 3 (4), 322–329. doi:10.1038/nclimate1744
U.S. Geological Survey (2019). National Hydrography dataset plus version 2. https://www.usgs.gov/national-hydrography/access-national-hydrography-products (Accessed April 21, 2022).
Wada, Y., van Beek, L. P. H., van Kempen, C. M., Reckman, J. W. T. M., Vasak, S., and Bierkens, M. F. P. (2010). Global depletion of groundwater resources. Geophys. Res. Lett. 37, L20402. doi:10.1029/2010GL044571
Waibel, M. S., Gannett, M. W., Chang, H., and Hulbe, C. L. (2013). Spatial variability of the response to climate change in regional groundwater systems – examples from simulations in the Deschutes Basin, Oregon. J. Hydrology 486, 187–201. doi:10.1016/j.jhydrol.2013.01.019
Western Regional Climate Center (2013). Cooperative climatological data summaries. https://wrcc.dri.edu/Climate/narrative_or.php (accessed September 19, 2022).
Whitehead, R. A. (1994). Ground water atlas of the United States. Section 7: Idaho, Oregon. Washington: U.S. Geological Survey. https://pubs.usgs.gov/ha/ha730/ch_h/.
Williams, J. E., and Sada, D. W. (2021). in Ghost of our making: Extinct aquatic species of the north American desert region” in standing between life and extinction: Ethics and ecology of conserving aquatic species in north American deserts. Editors D. Probst, J. E. Williams, K. Bestgen, and C. Hoagstrom (Chicago IL: University of Chicago Press), 89–105.
Wolaver, B. D., Priestley, S. C., Crossey, L. J., Karlstrom, K. E., and Love, A. J. (2020). Elucidating sources to aridland dalhousie springs in the great artesian basin (Australia) to inform conservation. Hydrogeol. J. 28, 279–296. doi:10.1007/s10040-019-02072-2
Young, W. H. (1991). Water planning: The Oregon approach innovation in western water law and management. Available at: https://scholar.law.colorado.edu/innovation-in-western-water-law-and-management/5.
Keywords: groundwater level trends, groundwater-dependent ecosystems, stressors, groundwater pumping, springs, phreatophytes
Citation: Saito L, Freed Z, Byer S and Schindel M (2022) The vulnerability of springs and phreatophyte communities to groundwater level declines in Oregon and Nevada, 2002–2021. Front. Environ. Sci. 10:1007114. doi: 10.3389/fenvs.2022.1007114
Received: 29 July 2022; Accepted: 05 December 2022;
Published: 15 December 2022.
Edited by:
Kirsten Work, Stetson University, United StatesReviewed by:
María Trinidad Torres-Garcia, Swedish University of Agricultural Sciences, SwedenAlex K. Manda, East Carolina University, United States
Copyright © 2022 Saito, Freed, Byer and Schindel. This is an open-access article distributed under the terms of the Creative Commons Attribution License (CC BY). The use, distribution or reproduction in other forums is permitted, provided the original author(s) and the copyright owner(s) are credited and that the original publication in this journal is cited, in accordance with accepted academic practice. No use, distribution or reproduction is permitted which does not comply with these terms.
*Correspondence: Laurel Saito, bGF1cmVsLnNhaXRvQHRuYy5vcmc=
†These authors have contributed equally to this work and share first authorship