- 1Laboratory of Stream Ecology, Department of Plant Biology and Ecology, University of the Basque Country (UPV/EHU), Bilbao, Spain
- 2Department of Evolutionary Biology, Ecology and Environmental Sciences, Water Research Institute (IdRA), University of Barcelona (UB), Barcelona, Spain
Rivers are severely affected by human activities and many are simultaneously impacted by multiple stressors. Water diversion for hydropower generation affects ecosystem functioning of the bypassed reaches, which can alternate between periods with natural discharge and others with reduced flow that increase the surface of dry riverbeds. In parallel, urban pollution contributes a complex mixture of nutrients, organic matter, heavy metals, pesticides, and drugs, thus becoming an important stressor in rivers. However, there is little information on the interaction between both stressors on ecosystem functioning and, particularly, on organic matter processing, a key process linked to the input of energy to food webs. To assess the impact of water diversion and urban pollution on organic matter processing, we selected four rivers in a pollution gradient with a similar diversion scheme and compared reaches upstream and downstream from the diversion weirs. We measured leaf-litter decomposition and carbon dioxide (CO2) fluxes in both the wet channel and the dry riverbed. Water diversion and pollution in the wet channel did not affect CO2 fluxes but reduced microbial decomposition, whereas in the dry riverbed, their interaction reduced total and microbial decomposition and CO2 fluxes. Thus, both stressors affected organic matter processing stronger in dry riverbeds than in the wet channel. These results show that dry riverbeds must be taken into account to assess and manage the impacts of human activities on river ecosystems.
Introduction
The increase of human population and its demands for water and energy impact biodiversity and ecosystems worldwide (Crist et al., 2017), streams and rivers being among the most affected ecosystems (Vörösmarty et al., 2010). European rivers are severely affected by human activities (Tockner et al., 2009), and almost half of them are simultaneously impacted by multiple stressors, such as hydromorphological alterations and pollution (Schinegger et al., 2012).
One of the most prevalent hydromorphological alterations is caused by water regulation and abstraction. Nowadays, 12%–16% of global food production and 19% of the world’s electricity depend on river water (Albert et al., 2021), and the dams and weirs built for these purposes fragment the world river network (Grill et al., 2019) and impact their biodiversity (Vörösmarty et al., 2010), as well as their contribution to global biogeochemical cycles (Syvitski et al., 2005). Large reservoirs cause special concern, as they exert strong impacts on hydrology, channel form, water quality, and biodiversity (Poff and Hart, 2002). Although the environmental impact of individual weirs and small dams is likely smaller, their extremely high numbers probably result in a very significant cumulative impact, as they account for over 91% of the barriers in streams and rivers worldwide (Belletti et al., 2020).
One particular type of hydromorphological alteration is caused by diversion hydropower schemes, which divert from the river part of the discharge, usually in a weir or low dam, and transfer it through an artificial canal to a hydropower station where it goes through the turbines before being reverted to the river (Couto and Olden, 2018). The bypassed section of the river, the reach between the diversion dam and the reversion point below the hydropower station, is thus subject to artificially low discharge when the hydropower scheme is operating, which typically leads to an enhanced areal cover of the dry channel (Arroita et al., 2017). Hydropower schemes have a maximum operating capacity that puts a ceiling to the amount of water diverted. Therefore, the proportion of discharge diverted tends to be low in high-flow periods, increases as the hydrographs recede, and can swiftly fall to zero when hydropower schemes close to ensure environmental flows. Therefore, bypassed reaches can alternate between periods with natural discharge and others with various degrees of diversion. Water diversion affects river biota and processes. It reduces biofilm biomass and activity (Arroita et al., 2017), affects the storage of organic matter (OM) (Death et al., 2009; Arroita et al., 2015; Riis et al., 2017), reduces leaf-litter decomposition (Schlief and Mutz, 2009; Martínez et al., 2017), and modifies invertebrate (Dewson et al., 2007; Walters, 2011; González et al., 2018; González and Elosegi, 2021) and fish communities (Anderson et al., 2015; Benejam et al., 2016). These impacts probably are stronger during base flows, when a larger fraction of the water is diverted, but legacy effects from diversion periods can also affect the river during shutdown periods (Arroita et al., 2018). Therefore, it is important to study the effect of hydropower during both active and inactive periods.
In parallel, human activities also increase the concentration of nutrients and other pollutants in the environment, thus degrading water quality and ecosystem status around the world (Hering et al., 2015). Especially worrying is the increase in urban pollution, a direct consequence of the rapid growth of urban areas through the world (Jones and O’Neill, 2016). Urban pollution usually consists of a complex mixture of pollutants that include nutrients and OM (Carey and Migliaccio, 2009), heavy metals (Deycard et al., 2014), pesticides, personal care products, and drugs (Kuzmanović et al., 2015; Osorio et al., 2016; Mandaric et al., 2018), among others. Depending on its composition, on the level of dilution in the receiving waters, and on the variable studied, urban pollution can have contrasting effects, from increases in biofilm biomass (Ribot et al., 2015; Pereda et al., 2020), ecosystem metabolism (Gücker et al., 2006; Aristi et al., 2015), and leaf litter decomposition (Englert et al., 2013; Ferreira et al., 2014), to reduced invertebrate diversity (Mor et al., 2019) and nutrient uptake efficiency (Martí et al., 2010). Although urban pollution is likely to interact with water diversion, most experimental evidence has been gathered from mesocosm experiments (Matthaei et al., 2010; Bækkelie et al., 2017; Arias-Font et al., 2021), real river studies being scarce, especially those dealing with ecosystem functioning.
In forested rivers, litter breakdown and mineralization are important processes linked to the energy inputs to food webs and to the emission of carbon dioxide (CO2) to the atmosphere (Wardle et al., 2004; Marx et al., 2017; Marks, 2019). Although most studies on river ecology focus on the wet channel (Attermeyer et al., 2021), dry riverbeds can have an important contribution to organic matter processing and CO2 emissions (Datry et al., 2018; Boodoo et al., 2019; Keller et al., 2020). Because water diversion increases the proportion of dry riverbeds, it is important to study ecosystem processes in these as well. The aim of this study was to analyze the interactive effects of water diversion and pollution on river carbon processing, including the role of wet and dry channel. To this end, we studied reaches upstream and downstream from diversion schemes in four rivers across a pollution gradient, from clean water to moderate pollution. We predicted that:
1) Water diversion would reduce OM stock and decomposition in the wet channel because of degraded environmental conditions. In the dry channel, there would not be an effect on the OM stock, but decomposition would decrease because of reduced drying–rewetting cycles. The CO2 efflux would respond as decomposition, as both processes are strongly linked to carbon mineralization.
2) Moderate pollution would promote OM decomposition and CO2 efflux, especially in the wet channel, which is in continuous contact with the pollutants. This increase in decomposition would in turn reduce the OM stock in the wet channel. Conversely, in the dry channel, we would expect no changes in OM stock and enhancement of OM decomposition and CO2 efflux.
3) Water diversion and pollution would interact in an antagonistic way in both wet and dry channel. Consequently, OM decomposition and CO2 efflux rates in bypassed reaches of polluted rivers would be closer to that of control reaches in clean rivers. In addition, the OM stock would be reduced in the wet channel but not in the dry channel.
Materials and Methods
Study Sites and Sampling Design
We selected four rivers (Urumea, Leitzaran, Kadagua, and Deba) in the Basque Country (northern Iberian Peninsula) (Supplementary Figure S1), a mountainous, industrial region with wet, temperate climate (Table 1). The catchments drained by these rivers differ in the intensity of human activities and in the proportion of urban land use (0.1%–4.6%; Table 1). As a result, water quality ranges from good to acceptable (Table 1 and Supplementary Table S1). A complex cocktail of pollutants, including nutrients, metals, and organic contaminants is detected in the worst situations (URA, 2016). Although rainfall tends to be higher in the area from winter to spring, precipitation is highly fluctuating, which can result in both flooding and base flow discharge at any season of the year (Elosegi et al., 2006).
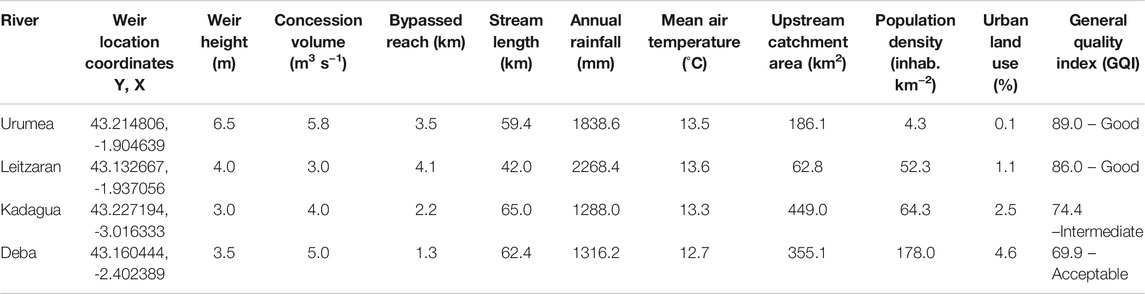
TABLE 1. Main characteristics of the studied rivers and the water diversion schemes within. The total annual rainfall and mean annual air temperature are the average of the 2 years of the study (www.euskalmet.euskadi.eus). Weir height, concession volume, and bypassed reach were obtained from the Cantabrian Hydrographic Confederation and the Institute of the Cultural Heritage of Spain (CHC, 2013; Pérez-Marrero, 2017). Population density was obtained from the National Statistics Institute (www.ine.es). The General Quality Index (GQI), based on water chemistry, was obtained from the Basque Water Agency (www.uragentzia.euskadi.eus).
The four rivers are affected by diversion schemes of similar characteristics consisting of a low weir that diverts water through a canal to a hydropower plant, strongly reducing the discharge in the bypassed section. Each hydropower plant is regulated by a specific water allowance, but generally, environmental flows are set at 10% of the monthly mean discharge (BOE, 2016). Therefore, hydropower plants typically operate in periods of several months (active period), are punctuated by day-to-month-long periods of no diversion during base flows (inactive period), although these periods do not necessarily coincide for all rivers. In this study, we sampled the four systems when the diversion was active but also when it was inactive, to detect potential legacy effects. We sampled two 100-m-long reaches per river: one upstream from the stagnant area created by the weirs (Control), and another one below the weirs (Regulated) (Figure 1). We sampled both the wet and the dry channel in each reach and measured variables linked to the structure and functioning of the river ecosystem. Structural variables included the dimension of the wet and dry channels, water quality, and stocks of OM. Among functional variables, those that reflect biologically mediated processes occurring within the stream channel (von Schiller et al., 2017), we measured leaf litter decomposition and CO2 fluxes.
Water Quality
Water temperature (°C), pH, electrical conductivity (EC, µS cm−1), dissolved oxygen (DO) concentration (mg L−1), and saturation (%) were measured with hand-held probes (WTW Multi 350i and 340i SET, WTW, Weilheim, Germany; YSI ProODO; YSI Incorporated, Yellow Springs, OH, USA). Water samples were taken, filtered in situ (0.7 µm pore size fiber glass filters, Whatman GF/F; Whatman International Ltd., Kent, United Kingdom) and frozen until analysis. We determined the soluble reactive phosphorus (SRP) concentration (mg P L−1) with the molybdate method (Murphy and Riley, 1962), and ammonium (N-NH4+, mg N L−1) with the salicylate method (Reardon et al., 1966), on a Shimadzu UV-1800 UV–vis Spectrophotometer (Shimadzu Corporation, Kyoto, Japan). The concentration of total dissolved nitrogen (TDN, mg N L−1) and dissolved organic carbon (DOC, mg L−1) were determined by catalytic oxidation on a Shimadzu TOC-LCSH analyzer coupled to a TNM-L unit (Shimadzu Corporation, Kyoto, Japan). The concentrations (mg L−1) of nitrate (N-NO3-), sulfate (SO42-) and chloride (Cl−), were measured by capillary electrophoresis (Agilent CE; Agilent Technologies, Santa Clara, CA, USA) (USEPA, 2000).
Hydrology
Mean discharge (m3 s−1) and velocity (m s−1) of whole-reach were estimated from time vs. conductivity curves obtained from pulse additions of NaCl (Martí and Sabater, 2009) at each reach (see below). Additionally, to obtain continuous discharge data, we placed absolute pressure loggers (Solinst Levelogger Edge 3001; Solinst Canada Ltd., Georgetown, ON, Canada) and atmospheric pressure loggers (Solinst Barologger Edge 3001; Solinst Canada Ltd.) at each of the eight reaches to record data every 15 min from June 2017 to September 2018. Absolute pressure was corrected by the atmospheric pressure, and water level regressed against discharge registered at nearby gauging stations to calculate continuous discharge at each reach. Additionally, we measured the width of wet and dry channel at 10 equidistant transects along each reach.
OM Standing Stocks
In each reach, nine samples of coarse benthic OM (CBOM) were randomly taken in the wet channel, and nine more in the dry channel. In the wet channel, CBOM was collected with a Surber sampler (0.09 m2, 0.5 mm mesh), it was sieved (8 mm), and the retained material was stored in zip bags. In the dry riverbed, a Surber frame (0.09 m2) net was used to delimit the area, and all litter found on top of the sediments was collected and stored in zip bags. Then, in the laboratory, the samples were dried (72 h, 70°C), weighed, combusted (5 h, 500°C), and re-weighed to determine the ash-free dry mass (AFDM, g m−2). To estimate fine benthic OM (FBOM, g m−2), nine samples were randomly collected in the wet channel per reach with a plastic sampling corer (81.7 cm2 surface). The corer was forced to the substratum, the volume of sediment inside was measured, benthos was stirred by hand, and a sample was taken and stored in a cool box. In the laboratory, samples were filtered through pre-weighed glass fiber filters (0.7 μm pore size), which were then treated as the CBOM samples. In the dry channel, five sediment samples per reach were collected and, in the laboratory, were dried to determine the water content (%) and then combusted to quantify the sediment OM content (SOM, %), as for the CBOM samples described previously.
Leaf Litter Decomposition
We measured the total and microbial leaf litter decomposition of black alder [Alnus glutinosa (L.) Gaertn.], both in wet and dry channel. Fresh leaves were collected the previous autumn, dried at ambient temperature, and stored in a dark and dry place until the experiment. Then, leaves were enclosed in bags of coarse (5 mm, 4 ± 0.05 g) and fine mesh (100 μm, 3 ± 0.05 g) and five bags per mesh type were secured per habitat (wet vs. dry channel) and reach by means of metal bars randomly anchored along the reach. Leaching was estimated from five bags of each mesh size that were kept in tap water for 24 h in the laboratory and the mass loss measured as for the rest of the bags from both habitats. Water and air temperature were recorded by means of Smart Button temperature loggers (ACR Systems, Surrey, BC, Canada) deployed with the bags. After 3 weeks of incubation, bags were collected and transported to the laboratory in a cool box. Once there, litter was cleaned with tap water to remove invertebrates and mineral particles, and then dried (72 h, 70°C), weighed, combusted (5 h, 500°C), and weighed again to obtain AFDM. Decomposition rates were calculated following Petersen and Cummins (1974) and expressed per degree day (d d−1). The breakdown rate measured in coarse mesh bags was considered total; that in fine mesh bags, microbial (Bärlocher et al., 2020). In addition, fragmentation by detritivorous macroinvertebrates was also calculated following Lecerf (2017).
CO2 Fluxes
CO2 fluxes were measured in both the wet and dry channel. In the wet channel, the partial pressure of CO2 in the atmosphere and water (pCO2,a and pCO2,w, respectively) was measured per triplicate with an infrared gas analyzer (EGM-5; PP-Systems, Amesbury, MA, USA). To measure pCO2,w, a membrane contactor (MiniModule, 3M, Germany) was coupled to the gas analyzer. We took a 10-L sample of water in a container, the water was circulated through the contactor at 300 ml min−1 by gravity, and the equilibrated gas was continuously recirculated into the gas analyzer (Teodoru et al., 2011). According to the manufacturer, the accuracy of the infrared gas analyzer was within 1% over the calibrated range. Then, we estimated the CO2 flux between surface water and atmosphere (FCO2,w, mmol m−2 d−1) applying Fick’s First Law of gas diffusion (Eq. 1):
where kCO2 is the specific gas transfer velocity for CO2 (m d−1), Kh is Henry’s constant (mmol μatm−1 m−3) adjusted for salinity and temperature (Weiss, 1974; Millero, 1995), and pCO2,w and pCO2,a are the partial pressures of CO2 in the water and atmosphere (µatm). To estimate the kCO2, we used the night-time drop in dissolved oxygen concentration (Hornberger and Kelly, 1972), as measured from optical dissolved oxygen sensors (YSI 6150 connected to YSI 600 OMS; YSI Inc., Yellow Springs, OH, USA) deployed for 24 h in each reach. We standardized the oxygen reaeration coefficient for depth to calculate the mean gas transfer velocity of oxygen (kO2, m d−1). Finally, we determined kCO2 by means of Eq. 2:
where kCO2 is the mean gas transfer velocity of CO2 (m d−1), ScCO2 and ScO2 are the Schmidt numbers of CO2 and O2 at a given water temperature (Wanninkhof, 1992), and n corresponds to the surface water motion, that was set to 1/2 according to the turbulence environment of running waters (Bade, 2009).
In the dry channel, we measured CO2 fluxes from five randomly selected sites per reach with the closed dynamic chamber method (Livingston and Hutchinson, 1995). We used an opaque chamber (SRC-2, PP-Systems) connected to the infrared gas analyzer and measured the gas concentration every 4.8 s. CO2 flux measurements lasted until a change of at least 10 µatm was reached, with a duration of 120–300 s. From the rate of change of CO2 inside the chamber, we estimated the CO2 flux between dry riverbed and atmosphere (FCO2,d, mmol m−2 d−1) by means of Eq. 3:
where dpCO2/dt is the slope of the gas accumulation in the chamber along time (µatm s−1), V is the chamber volume (1.171 dm3), R is the ideal gas constant (L atm K−1 mol−1), T is the air temperature (Kelvin), and S is the chamber surface (0.78 dm2).
At each site, we also measured the substrate temperature with a portable soil probe and collected sediment samples (upper 5 cm), which were stored in a cool box and transported to the laboratory, where we determined water content and OM content as described above.
Data Analysis
We statistically tested the variation of ecosystem structure and functioning variables using linear models. In these models, we firstly tested for differences between Control and Regulated reaches, which would result from the direct effect of the barrier on the response variables measured. Secondly, we also tested for the short-term effects of the diversion (when it was Active) against the long-term effects of it (when it was Inactive). The interaction of these two sources of variation would show, for instance, if the differences between Control and Regulated sites were only evident when the diversion was active, or, on the contrary, differences were apparent any time. Thirdly, we tested for the implications of the pollution levels of the water on the measured variables. Thus, linear models built included period (comparison between Active and Inactive periods; factor), reach (comparison between Control and Regulated reaches; factor), pollution (measured through the General Quality Index, GQI; covariate), and second-order interactions as sources of variation. We tested the triple interaction between reach, pollution, and period, but since it was not significant or marginally significant for most of the variables, we decided to remove it from all analyses. Season (Spring/Autumn; factor) was included as a block factor. For water quality variables, linear models considering river, reach, and their interaction were built. As respiration and CO2 fluxes of the dry channel can depend on SOM and water content (Marcé et al., 2019; Keller et al., 2020), we tested relationship among these variables by means of Pearson correlation tests. The significance of each source of variation was tested by means of ANOVA. The behavior of residuals was checked by means of diagnostic plots to assure linearity, normality, homoscedasticity, and absence of outliers. When necessary, log-transformation of the data was enough to meet these requirements for linear models. All analyses were performed using R software, v. 3.4.0 (R Core Team, 2019).
Results
Water Quality
Water quality did not change between Control and Regulated reaches (Table 2), and differences among rivers were not statistically significant for temperature, DO concentration, and saturation and NO3− concentration (12.2°C –21.3°C, 8.6–12.7 mg O2 L−1, 95%–122% and 0.1–2.0 mg N L−1). In contrast, there were significant differences among rivers, but not among reaches, for pH (7.4–8.5), EC (64–640 μS cm−1), NH4+ (0.0–0.1 mg N L−1), TDN (0.8–2.6 mg N L−1), SRP (0.01–0.08 mg P L−1), DOC (1.5–8.4 mg L−1), Cl− (1.8–16.1 mg L−1), and SO4−2 (1.0–31.8 mg L−1) (Table 2 and Supplementary Table S2). The interaction between river and reach, i.e., within-river variation, was also non-significant for all variables measured (Table 2).
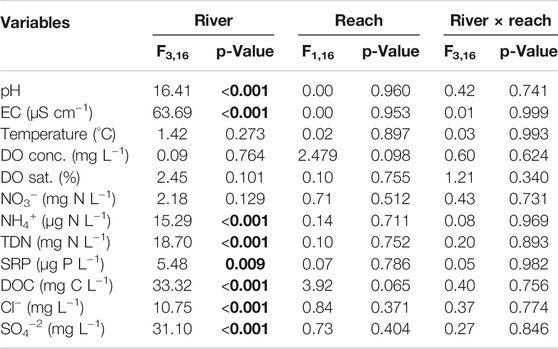
TABLE 2. Results of linear models testing for the effect of river, reach, and their interaction on water quality variables (n = 24). p-Values were obtained by two-way ANOVA test. EC = Electrical conductivity, DO = dissolved oxygen, NO3− = nitrates, NH4+ = ammonium, TDN = total dissolved nitrogen, SRP = soluble reactive phosphorus, DOC = dissolved organic carbon, Cl− = chloride and SO4−2 = sulfate. F-values are shown in the table and the degrees of freedom indicated at the top of the table are the same for all the tests. Significant p-values are shown in bold.
Hydrology
Water discharge in control reaches was 61.0% ± 14.7% higher during the active than during inactive periods (Supplementary Table S2). During the active period sampling, the percentage of water diverted ranged from 40% (Kadagua) to 90% (Leitzaran). Discharge and water velocity did not differ between reaches or periods (Table 3 and Supplementary Tables S2, S5). Nevertheless, the percentage of width of wet channel was on average 17.9% smaller in the regulated reaches during the active period (Table 3 and Supplementary Tables S2, S5).
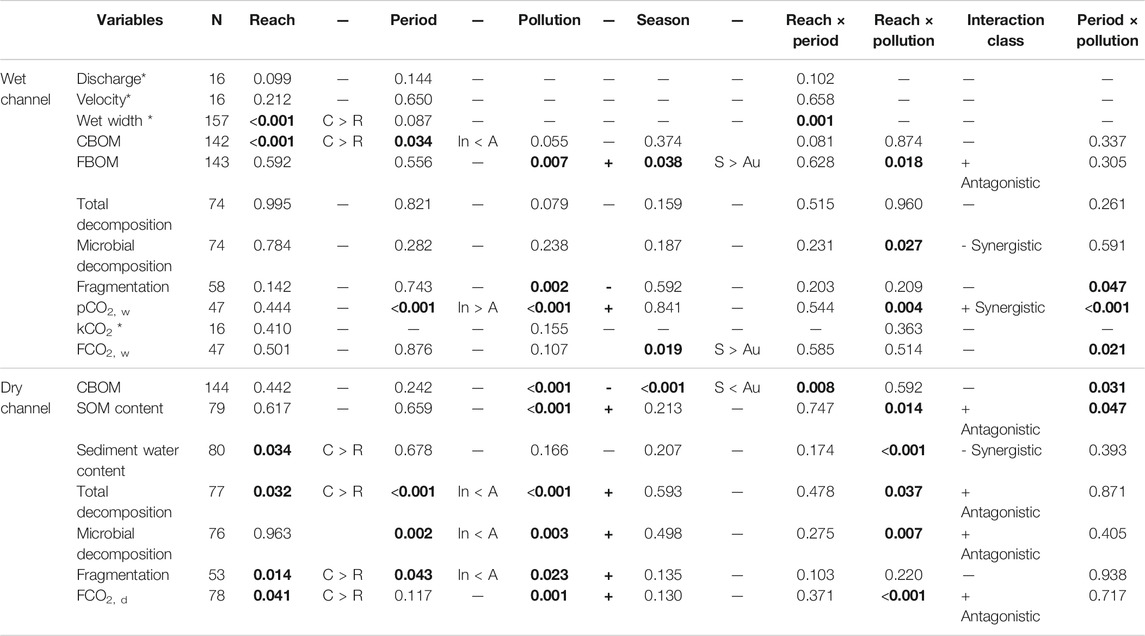
TABLE 3. Results of linear models testing for the effects of reach, status, pollution and season on variables measured in the wet and the dry channel. CBOM = coarse benthic organic matter, FBOM = fine benthic organic matter, pCO2,w = CO2 partial pressure in water, kCO2 = CO2 reaeration coefficient, FCO2,w = CO2 flux between water and atmosphere, FCO2,d = CO2 flux between dry riverbed and atmosphere, SOM = sediment organic matter. p-Values were obtained by ANOVA. Significant p-values are shown in bold. Number of data used (N) is shown. Sign of the coefficients and comparisons between levels of main significant effects are given (C: control; R: Regulated; In: Inactive; A: active; +: increase; -: decrease; S: Spring; Au: Autumn). Interaction class corresponds to reach and pollution interaction and it was classified following Piggott et al. (2015). * Given the limited replication a simpler model was used for the statistical analysis.
OM Standing Stock
The stock of CBOM in the wet channel ranged from 0.0 to 236.9 g m−2 and was not significantly affected by pollution (Table 3; Supplementary Tables S3, S5; Figure 2A). It was lower in regulated than in control reaches and higher in the period of active diversion. In dry channels, CBOM ranged from 0.0 to 7548.0 g m−2 and decreased with pollution (Table 3; Supplementary Tables S4, S5). Although reach and period caused no significant differences, their interaction did, with CBOM being lower in regulated reaches when the diversion was active (Table 3; Supplementary Tables S4, S5; Figure 2B). Moreover, the period × pollution interaction was also significant, indicating a reduction of CBOM with pollution when the diversion period was active (Table 3; Supplementary Tables S4, S5; Figure 2B).
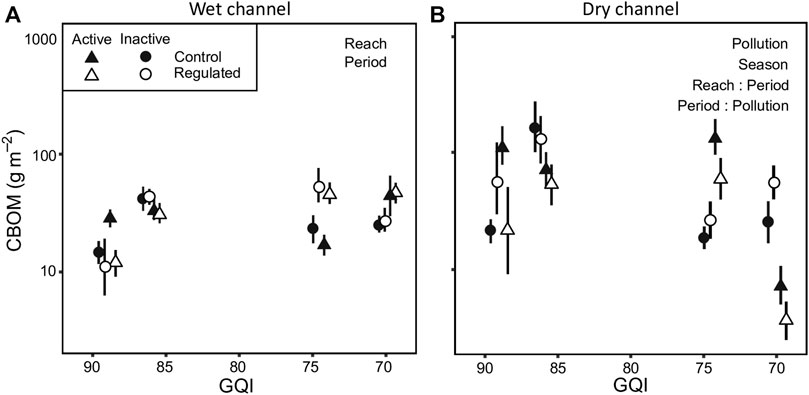
FIGURE 2. Stock of coarse benthic organic matter (CBOM) in (A) wet and (B) dry channel. Values are means and error bars show standard errors. The text in the background indicates significant single-factor effects or interactions. GQI is the General Quality Index and the scale is inverted to make easier the interpretation of the figure, ranging from good (low pollution) to acceptable quality level (moderate pollution). GQI values have been jittered to avoid overlapping points.
The stock of FBOM in the wet channel ranged from 0.4 to 300.1 g m−2 and increased with pollution (Table 3; Supplementary Tables S3, S5, Supplementary Figure S2). Reach and period did not affect FBOM stocks. The interaction between reach and pollution was significant, with FBOM differences being higher in regulated reaches of the most polluted rivers. In the dry channel, the SOM content ranged from 1.8% to 10.8% and increased with pollution (Table 3; Supplementary Tables S4, S5, Supplementary Figure S3A). Reach or period did not affect SOM content. The interaction between reach and pollution was significant, showing a lower SOM content only in the polluted and regulated reaches (Table 3; Supplementary Tables S4, S5, Supplementary Figure S3A). The interaction between period and pollution was also significant; thus, differences in SOM content between periods became larger with increasing pollution, being the lowest OM content during the active period (Table 3; Supplementary Tables S4, S5, Supplementary Figure S3A). However, water content ranged from 2.5% to 44.8% and was not affected by pollution (Table 3; Supplementary Tables S4, S5, Supplementary Figure S3A). Water content was significantly lower in regulated than in control reaches. The interaction of reach and pollution was also significant, with regulated reaches in polluted rivers showing the lowest water content (Table 3; Supplementary Tables S4, S5, Supplementary Figure S3B).
Leaf Litter Decomposition
In the wet channel, total decomposition ranged from 0.0005 to 0.0043 d d−1, microbial decomposition from 0.0005 to 0.0024 d d−1, and fragmentation from 0.0000 to 0.0021 d d−1. Total decomposition was unaffected by the investigated factors (Table 3; Supplementary Tables S3, S5; Figure 3A). Microbial decomposition was not affected by single stressors but was interactively affected by the reach × pollution interaction, being slower in regulated reaches of the most polluted rivers (Table 3; Supplementary Tables S3, S5; Figure 3C). Fragmentation decreased significantly with pollution (Table 3; Supplementary Tables S3, S5; Figure 3E). Moreover, the period × pollution interaction was significant, with fragmentation being lowest during inactive periods in the most polluted rivers (Table 3; Supplementary Tables S3, S5; Figure 3E).
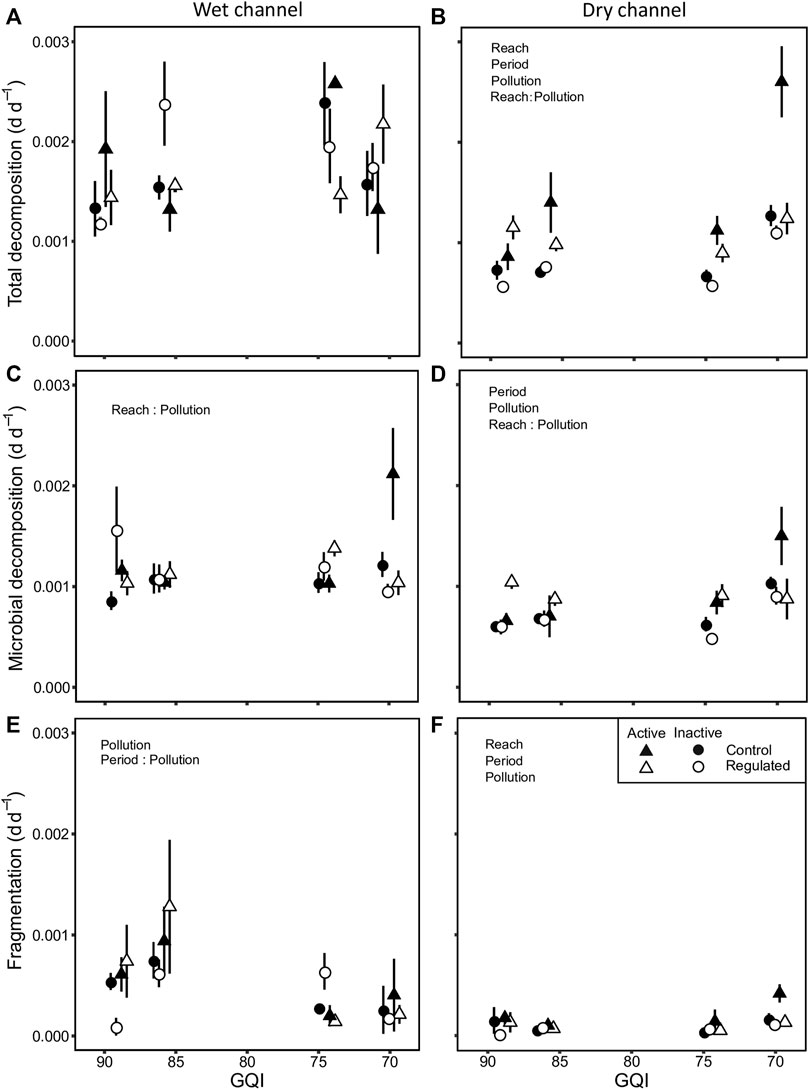
FIGURE 3. Leaf litter decomposition in the wet (left side) and dry channel (right side): (A, B) total decomposition, (C, D) microbial decomposition, and (E, F) fragmentation. Values are mean and error bars show standard error. The text in the background indicates significant single-factor effects or interactions. GQI is the General Quality Index and the scale is inverted to make easier the interpretation of the figure, ranging from good (low pollution) to acceptable quality level (moderate pollution). GQI values have been jittered to avoid overlapping points.
In the dry channel, total decomposition ranged from 0.0005 to 0.003 d d−1, microbial decomposition from 0.000 to 0.002 d d−1, and fragmentation from 0.0000 to 0.0006 d d−1. The three processing rates increased significantly with pollution. Total and microbial decomposition and fragmentation rates were also significantly lower when diversions were inactive compared to when they were active (Table 3; Supplementary Tables S4, S5; Figures 3B,D,F). However, total decomposition and fragmentation were significantly lower in regulated reaches (Table 3; Supplementary Tables S4, S5; Figures 3B,F). The reach × pollution interaction was significant for total and microbial decomposition, with differences between reaches being higher in most polluted rivers (Table 3; Supplementary Tables S4, S5; Figures 3B,D). Total and microbial decomposition was lower in regulated reaches but the reduction was not below the control of the cleanest river.
CO2 Flux
In the wet channel, pCO2, w ranged from 369 to 1175 µatm and increased with pollution (Table 3; Supplementary Tables S3, S5, Supplementary Figure S4). When the diversions were active, pCO2, w was significantly lower compared to when it was inactive; however, there were no differences between reaches. The reach × pollution interaction was significant, showing lower pCO2,w in regulated reaches of the most polluted rivers. The period × pollution interaction also was significant, with pCO2,w decreasing more clearly when the diversions were active in the most polluted sites. The reaeration coefficient kCO2 ranged from 6.0 to 44.2 m d−1 and neither reach, pollution nor their interaction showed any significant effect (Table 3; Supplementary Tables S3, S5). In the wet channel, FCO2, w ranged from −6.7 to 430.3 mmol m−2 d−1 and was not affected by reach, period, or pollution (Table 3; Supplementary Tables S3, S5; Figure 4A). The reach × pollution interaction was not significant, but that between period and pollution was, with pollution increasing differences in FCO2, w between periods (Table 3; Supplementary Tables S3, S5; Figure 4A). In the dry channel, FCO2,d ranged from 28.4 to 986.6 mmol m−2 d−1 (Table 3; Supplementary Tables S4, S5; Figure 4B) and was significantly related to SOM and water content (log transformed data; Pearson r = 0.363, p < 0.001 and Pearson r = 0.288, p = 0.010, respectively). FCO2,d increased with pollution (Table 3; Supplementary Tables S4, S5; Figure 4B). Period did not affect it significantly, but in regulated reaches FCO2,d was significantly lower. The reach × pollution interaction was also significant, with FCO2,d decreasing only in regulated reaches of polluted rivers (Table 3; Supplementary Tables S4, S5; Figure 4B).
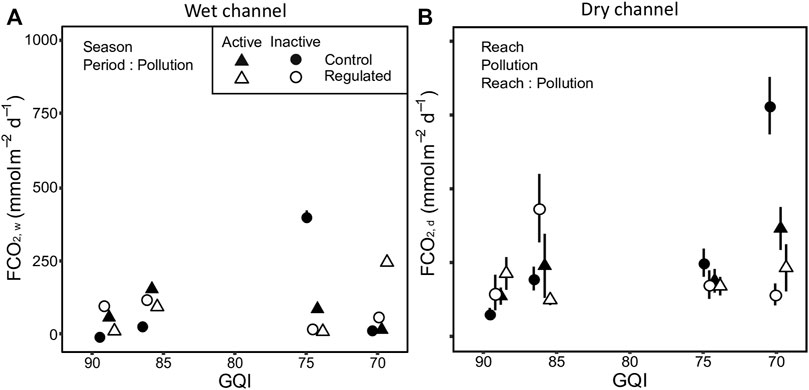
FIGURE 4. CO2 efflux (FCO2) in (A) wet and (B) dry channel. Values are mean and error bars show standard error. The text in the background indicates significant single-factor effects or interactions. GQI is the General Quality Index and the scale is inverted to make easier the interpretation of the figure, ranging from good (low pollution) to acceptable quality level (moderate pollution). GQI values have been jittered to avoid overlapping points.
Discussion
This study adds to the existing knowledge on the effects of water diversion by small hydropower schemes. It does so by focusing mainly on river ecosystem functioning, a relatively little studied component of river integrity (von Schiller et al., 2017), and looking at the interaction between water diversion and pollution, one of the most common stressors in world rivers (Hering et al., 2015). Our approach is also seldom used, as we compared upstream control with downstream regulated reaches, both in periods of active and inactive diversion, thus yielding a comprehensive picture of the overall effects of hydropower schemes, which usually alternate between active and inactive periods. Our results show that water diversion and pollution have interactive effects on river OM processing, dry channels being more reactive to the interaction than wet channels. Overall, water diversion did not affect leaf litter decomposition and CO2 fluxes in the wet channel, but in the dry channel, decomposition and CO2 emissions were lower with water diversion. On the other hand, pollution reduced leaf litter fragmentation but did not affect the CO2 flux in the wet channel, whereas in the dry channel it promoted both decomposition and CO2 emissions. Both stressors interacted antagonistically for microbial decomposition in the wet channel, and for total and microbial decomposition and CO2 fluxes in the dry channel.
Diversion
We hypothesized water diversion to reduce leaf litter decomposition and OM standing stocks in the wet channel, but results only confirmed this for CBOM. This reduction of CBOM might be a consequence of its retention in the dam (Schmutz and Moog, 2018), of its transport through the diversion canal (Arroita et al., 2015), or probably both, to counteract the effect of increased retention in the bypassed reach (Arroita et al., 2017). For the dry channel, we hypothesized a decrease of leaf litter decomposition and no effects on OM stocks with water diversion, but again, results only confirmed this partially. The content of CBOM and SOM were not affected by diversion, but total decomposition and fragmentation (but not microbial decomposition) were significantly reduced. In terrestrial habitats, leaf litter decomposition tends to be lower than in the moist sediments from dry beds, and much lower than in running waters (Lake, 2003; Abril et al., 2016). In our case, diversion caused the contraction of the wet channel by almost 20% in regulated reaches and reduced rewetting cycles and the water content of sediments in the dry channel, conditions that lead to slow decomposition in riparian and terrestrial areas (Tiegs et al., 2019). Microbial decomposition did not differ between reaches, maybe because the fine mesh used maintained a level of moisture in the bags high enough to keep an active microbial community (Romaní et al., 2017).
Regarding the CO2 flux, we predicted a reduction by water diversion in both the wet and the dry channel, but results only confirmed our prediction for the dry channel. Water diversion caused a decrease in FCO2,d in regulated reaches, probably because of the reduction of water content in sediments, which restricts microbial activity and the subsequent release of CO2 (Arce et al., 2019; Marcé et al., 2019). In this sense, dry sediments in regulated reaches would function in a way more similar to that of Mediterranean parafluvial areas (Almagro et al., 2009).
Pollution
Although we expected pollution to reduce the stock of OM in the wet channel and no effects in the dry channel, we found no effect in the wet channel and reduced CBOM stocks in the dry channel. The explanation for this unexpected result could be reduced riparian forests in the most urbanized basins (Pennington et al., 2010), which would limit OM inputs to dry bars in our most altered rivers, whereas urban wastewater would increase FBOM in the wet channel (Kelso and Baker, 2020). Unexpectedly, SOM content increased with pollution, thus suggesting this OM was at least in part derived from FBOM transported by flow and deposited on the dry channel during water level fluctuations. More detailed characterization of the SOM would be needed to elucidate its origin.
On the other hand, pollution did not affect total and microbial decomposition in the wet channel but reduced fragmentation. The response of decomposition to pollution is complex and often hump-shaped because of the differential sensitivity of microbes and detritivorous (Woodward et al., 2012). Detritivorous macroinvertebrates, the main responsible organisms for leaf litter fragmentation (Graça, 2001; Hieber and Gessner, 2002; Lecerf, 2017), tend to decrease at moderate levels of pollution, as occurs in our streams (de Guzman et al., in prep). Woodward and collaborators (2012) reported peak invertebrate-mediated breakdown (similar to our fragmentation rate) at 0.02 mg SRP L−1 and 5.6 mg DIN L−1. Although in our rivers the range of DIN concentration was below the maximum for invertebrate breakdown, the SRP concentration of most polluted rivers was above the maximum concentration promoting a lower fragmentation (Supplementary Table S3). Therefore, probably SRP concentration in our rivers was high and damaging for fragmentation rates. Moreover, several studies have shown the detrimental effect of other pollutants also present in our rivers (e.g., heavy metals, biocides) on shredders and litter decomposition (Carlisle and Clements, 2005; Alonso and Camargo, 2006; Baldy et al., 2007; Brosed et al., 2016). In the dry channel, results confirmed our hypothesis and decomposition increased with pollution. Despite the temporal rewetting cycles of leaf litter on the dry channel, the moderate nutrient load of water promoted decomposition at all levels (i.e., total, microbial and fragmentation). Moderate nutrient enrichment stimulates fungal activity and, in consequence, palatability for invertebrate leaf consumption when litter is submerged (Gulis and Suberkropp, 2003; Dunck et al., 2015).
We also predicted an increase of CO2 efflux with pollution in both habitats, but results only confirmed it partially. In the wet channel, FCO2,w was not affected by pollution. Higher nutrient and OM loads, as those detected across our pollution gradient, can increase river ecosystem respiration, thereby increasing pCO2,w (Prasad et al., 2013; Borges et al., 2015; Hotchkiss et al., 2015). Although there is a dependency between pCO2,w and FCO2,w, the two processes have different drivers (Liu and Raymond, 2018), as the latter is also affected by the reaeration coefficient (kCO2). There was a decrease in kCO2 along the pollution gradient that compensated the increase in pCO2,w, resulting in no effect on FCO2,w. In the dry channel, as we expected, the CO2 efflux increased with pollution. Microbial activity in sediment can be promoted by DOC and TN previously deposited from water (Gómez-Gener et al., 2016), and the water content on sediment during rewetting cycles would facilitate the microbial uptake of these compounds, thus increasing microbial respiration and CO2 fluxes (Xu et al., 2004; Luo and Zhou, 2010; Gómez-Gener et al., 2015).
Diversion–Pollution Interaction
Although we expected the interaction between both stressors to reduce OM stock in the wet channel and to have no effect in the dry channel, results proved otherwise. The CBOM stock did not respond to the interaction in any habitat, but FBOM increased in the wet channel, whereas SOM decreased. The increase in FBOM seems to be a consequence of enhanced deposition under reduced flows (Riis et al., 2017), which would be more noticeable in the polluted rivers, where suspended solids are more abundant (URA, 2016) (Supplementary Table S1). The decrease in SOM content is harder to explain but may be related to lower frequency of rewetting the dry sediments.
Regarding decomposition, in both habitats we expected an antagonistic effect between the reduction caused by diversion and the promotion caused by pollution, which would result in decomposition rates closer to those of control reaches in clean rivers. In the wet channel, results did not confirm our hypothesis since total decomposition and fragmentation did not respond, whereas microbial decomposition in the presence of both stressors was lower than control values. Because of wet channel contraction in regulated reaches, water depth also could be smaller resulting in an increase in the boundary layer thickness surrounding the microbial community growing on leaf litter (Bishop et al., 1997). In consequence, in spite of the presence of nutrients from moderate pollution of water, the exchange of nutrients and oxygen with the water column would be limited and microbial decomposition would be reduced (Medeiros et al., 2009; Bruder et al., 2016). In the dry channel, we also expected an antagonistic effect, partially confirmed by our results. Although the fragmentation rate did not respond, total and microbial decomposition were reduced, the interaction being closer to control reaches in cleaner rivers. Despite pollution stimulated decomposition, the contact with water is one of the most important factors that influences leaf litter decomposition (Northington and Webster, 2017).
For CO2 fluxes, we also expected an antagonistic response to the interaction in wet and dry channels. Whereas FCO2,w in the wet channel did not respond to the interaction, pCO2,w was lower in polluted and regulated reaches than in control reaches. This reduction in pCO2,w might be a consequence of algal growth under moderate nutrient load, good solar irradiation, and low water velocity (Marx et al., 2017). Alternatively, it could be due to reduced hydrological connectivity between wet and dry channels, as soil respiration and weathering processes contribute to aquatic pCO2,w (Striegl and Michmerhuizen, 1998; Riveros-Iregui and McGlynn, 2009). In the dry channel, we also hypothesized an antagonistic response of FCO2,d to the interaction of both stressors, a prediction that was confirmed by our results. FCO2,d was lower in regulated than in control reaches in polluted rivers and closer to control reaches of cleaner rivers. The low water and OM content in sediment could lead to low microbial activity, which in turn causes low CO2 efflux.
Conclusion and Implications
Our results show that the interaction between water diversion and pollution can have important consequences on in-stream OM processing. These two stressors interacted antagonistically and their effects were more pronounced in the dry channel than in the wet channel. The observed changes in particulate OM decomposition both in the wet and the dry channel, as a result of water diversion and pollution, may cause profound shifts in the trophic structure and energetic balance of the ecosystem, as allochthonous OM is the main energy source supporting food webs in forested rivers (Marks, 2019). On the other hand, the two investigated stressors had significant effects on CO2 emissions in the dry channel, emphasizing the role of dry riverbeds in gaseous carbon exchange along river networks (Marcé et al., 2019). Overall, our study reinforces the idea that not considering dry channels as an active part of rivers could lead to an underestimation of the effect of diversion and urban pollution on these ecosystems.
Data Availability Statement
The original contributions presented in the study are included in the article/Supplementary Material, further inquiries can be directed to the corresponding author.
Author Contributions
AE, AL, and DS contributed to conception and design of the study. AP, IG, DS, AL, and AE participated in the field work. AP organized the database. AP and AL performed the statistical analysis. AP wrote the draft of the manuscript. All authors contributed to manuscript revision, read, and approved the submitted version.
Funding
This research was funded by the Spanish Department of Economy, Industry and Competitiveness through the project GL 2016-77487-R (DIVERSION), the European Social Fund, the Basque Government (Consolidated Research Group IT951-16), and the Biscay Province Council (61/2015). AP and IG carried out this work, thanks to a pre-doctoral grant by the Spanish Department of Economy, Industry, and Competitiveness (BES-2017-081959) and a pre-doctoral grant of the Basque Government, respectively. DS is a Serra Húnter Fellow.
Conflict of Interest
The authors declare that the research was conducted in the absence of any commercial or financial relationships that could be construed as a potential conflict of interest.
Publisher’s Note
All claims expressed in this article are solely those of the authors and do not necessarily represent those of their affiliated organizations, or those of the publisher, the editors, and the reviewers. Any product that may be evaluated in this article, or claim that may be made by its manufacturer, is not guaranteed or endorsed by the publisher.
Acknowledgments
The authors thank Miren Atristain, Olatz Pereda, and Libe Solagaistua for assistance in field samplings, and to SCAB from SGIKER (UPV/EHU/ERDF, EU) for technical and human support provided.
Supplementary Material
The Supplementary Material for this article can be found online at: https://www.frontiersin.org/articles/10.3389/fenvs.2021.817665/full#supplementary-material
References
Abril, M., Muñoz, I., and Menéndez, M. (2016). Heterogeneity in Leaf Litter Decomposition in a Temporary Mediterranean Stream during Flow Fragmentation. Sci. Total Environ. 553, 330–339. doi:10.1016/j.scitotenv.2016.02.082
Albert, J. S., Destouni, G., Duke-Sylvester, S. M., Magurran, A. E., Oberdorff, T., Reis, R. E., et al. (2021). Scientists' Warning to Humanity on the Freshwater Biodiversity Crisis. Ambio 50, 85–94. doi:10.1007/s13280-020-01318-8
Almagro, M., López, J., Querejeta, J. I., and Martínez-Mena, M. (2009). Temperature Dependence of Soil CO2 Efflux Is Strongly Modulated by Seasonal Patterns of Moisture Availability in a Mediterranean Ecosystem. Soil Biol. Biochem. 41, 594–605. doi:10.1016/j.soilbio.2008.12.021
Alonso, A., and Camargo, J. A. (2006). Toxicity of Nitrite to Three Species of Freshwater Invertebrates. Environ. Toxicol. 21, 90–94. doi:10.1002/tox.20155
Anderson, D., Moggridge, H., Warren, P., and Shucksmith, J. (2015). The Impacts of 'run-Of-River' Hydropower on the Physical and Ecological Condition of Rivers. Water Environ. J. 29, 268–276. doi:10.1111/wej.12101
Arce, M. I., Mendoza-Lera, C., Almagro, M., Catalán, N., Romaní, A. M., Martí, E., et al. (2019). A Conceptual Framework for Understanding the Biogeochemistry of Dry Riverbeds through the Lens of Soil Science. Earth-Science Rev. 188, 441–453. doi:10.1016/j.earscirev.2018.12.001
Arias Font, R., Khamis, K., Milner, A. M., Sambrook Smith, G. H., and Ledger, M. E. (2021). Low Flow and Heatwaves Alter Ecosystem Functioning in a Stream Mesocosm Experiment. Sci. Total Environ. 777, 146067. doi:10.1016/j.scitotenv.2021.146067
Aristi, I., Schiller, D., Arroita, M., Barceló, D., Ponsatí, L., García‐Galán, M. J., et al. (2015). Mixed Effects of Effluents from a Wastewater Treatment Plant on River Ecosystem Metabolism: Subsidy or Stress. Freshw. Biol. 60, 1398–1410. doi:10.1111/fwb.12576
Arroita, M., Aristi, I., Díez, J., Martinez, M., Oyarzun, G., and Elosegi, A. (2015). Impact of Water Abstraction on Storage and Breakdown of Coarse Organic Matter in Mountain Streams. Sci. Total Environ. 503-504, 233–240. doi:10.1016/j.scitotenv.2014.06.124
Arroita, M., Flores, L., Larrañaga, A., Chauvet, E., and Elosegi, A. (2018). Hydrological Contingency: Drying History Affects Aquatic Microbial Decomposition. Aquat. Sci. 80, 0. doi:10.1007/s00027-018-0582-3
Arroita, M., Flores, L., Larrañaga, A., Martínez, A., Martínez-Santos, M., Pereda, O., et al. (2017). Water Abstraction Impacts Stream Ecosystem Functioning via Wetted-Channel Contraction. Freshw. Biol. 62, 243–257. doi:10.1111/fwb.12864
Attermeyer, K., Casas-Ruiz, J. P., Fuss, T., Pastor, A., Cauvy-Fraunié, S., Sheath, D., et al. (2021). Carbon Dioxide Fluxes Increase from Day to Night across European Streams. Commun. Earth Environ. 2, 118. doi:10.1038/s43247-021-00192-w
Bade, D. L. (2009). “Gas Exchange at the Air-Water Interface,” in Encycl. Inl. Waters. Editor E. L. Gene (Oxford Acad. Press.), 70–78. doi:10.1016/b978-012370626-3.00213-1
Bækkelie, K. A. E., Schneider, S. C., Hagman, C. H. C., and Petrin, Z. (2017). Effects of Flow Events and Nutrient Addition on Stream Periphyton and Macroinvertebrates: an Experimental Study Using Flumes. Knowl. Manag. Aquat. Ecosyst. 47.
Baldy, V., Gobert, V., Guerold, F., Chauvet, E., Lambrigot, D., and Charcosset, J.-Y. (2007). Leaf Litter Breakdown Budgets in Streams of Various Trophic Status: Effects of Dissolved Inorganic Nutrients on Microorganisms and Invertebrates. Freshw. Biol 52, 1322–1335. doi:10.1111/j.1365-2427.2007.01768.x
Bärlocher, F., Gessner, M. O., and Garca, M. O. S. (2020). Methods to Study Litter Decomposition. Springer.
Belletti, B., Garcia de Leaniz, C., Jones, J., Bizzi, S., Börger, L., Segura, G., et al. (2020). More Than One Million Barriers Fragment Europe's Rivers. Nature 588, 436–441. doi:10.1038/s41586-020-3005-2
Benejam, L., Saura-Mas, S., Bardina, M., Solà, C., Munné, A., and García-Berthou, E. (2016). Ecological Impacts of Small Hydropower Plants on Headwater Stream Fish: from Individual to Community Effects. Ecol. Freshw. Fish. 25, 295–306. doi:10.1111/eff.12210
Bishop, P. L., Gibbs, J. T., and Cunningham, B. E. (1997). Relationship between Concentration and Hydrodynamic Boundary Layers over Biofilms. Environ. Tech. 18, 375–385. doi:10.1080/09593331808616551
BOE (2016). Real Decreto 1/2016, de 8 de enero, por el que se aprueba la revisión de los Planes Hidrológicos de las demarcaciones hidrográficas del Cantábrico Occidental, Guadalquivir, Ceuta, Melilla, Segura y Júcar, y de la parte española de las demarcaciones hidrog. Off. J. Spanish Goverment 16, 2972–4301.
Boodoo, K. S., Schelker, J., Trauth, N., Battin, T. J., and Schmidt, C. (2019). Sources and Variability of CO 2 in a Prealpine Stream Gravel Bar. Hydrological Process. 33, 2279–2299. doi:10.1002/hyp.13450
Borges, A. V., Darchambeau, F., Teodoru, C. R., Marwick, T. R., Tamooh, F., Geeraert, N., et al. (2015). Globally Significant Greenhouse-Gas Emissions from African Inland Waters. Nat. Geosci 8, 637–642. doi:10.1038/ngeo2486
Bruder, A., Salis, R. K., McHugh, N. J., and Matthaei, C. D. (2016). Multiple‐stressor Effects on Leaf Litter Decomposition and Fungal Decomposers in Agricultural Streams Contrast between Litter Species. Funct. Ecol. 30, 1257–1266. doi:10.1111/1365-2435.12598
Carey, R. O., and Migliaccio, K. W. (2009). Contribution of Wastewater Treatment Plant Effluents to Nutrient Dynamics in Aquatic Systems: A Review. Environ. Manage. 44, 205–217. doi:10.1007/s00267-009-9309-5
Carlisle, D. M., and Clements, W. H. (2005). Leaf Litter Breakdown, Microbial Respiration and Shredder Production in Metal-Polluted Streams. Freshw. Biol. 50, 380–390. doi:10.1111/j.1365-2427.2004.01323.x
CHC (2013). Plan Hidrológico. Demarcación hidrográfica del Cantábrico Oriental. Apendice VI. Descripción de los sistemas de explotación. Ministerio de Agricultura, Alimentación y Medio Ambiente. Goboierno de España.
Couto, T. B., and Olden, J. D. (2018). Global Proliferation of Small Hydropower Plants - Science and Policy. Front. Ecol. Environ. 16, 91–100. doi:10.1002/fee.1746
Crist, E., Mora, C., and Engelman, R. (2017). The Interaction of Human Population, Food Production, and Biodiversity protection. Science 356, 260–264. doi:10.1126/science.aal2011
Datry, T., Foulquier, A., Corti, R., Von Schiller, D., Tockner, K., Mendoza-Lera, C., et al. (2018). A Global Analysis of Terrestrial Plant Litter Dynamics in Non-perennial Waterways. Nat. Geosci. 11, 497–503. doi:10.1038/s41561-018-0134-4
Death, R. G., Dewson, Z. S., and James, A. B. W. (2009). Is Structure or Function a Better Measure of the Effects of Water Abstraction on Ecosystem Integrity. Freshw. Biol. 54, 2037–2050. doi:10.1111/j.1365-2427.2009.02182.x
Dewson, Z. S., James, A. B. W., and Death, R. G. (2007). Invertebrate Community Responses to Experimentally Reduced Discharge in Small Streams of Different Water Quality. J. North Am. Benthological Soc. 26, 754–766. doi:10.1899/07-003r.1
Deycard, V. N., Schäfer, J., Blanc, G., Coynel, A., Petit, J. C. J., Lanceleur, L., et al. (2014). Contributions and Potential Impacts of Seven Priority Substances (As, Cd, Cu, Cr, Ni, Pb, and Zn) to a Major European Estuary (Gironde Estuary, France) from Urban Wastewater. Mar. Chem. 167, 123–134. doi:10.1016/j.marchem.2014.05.005
Dunck, B., Lima-Fernandes, E., Cássio, F., Cunha, A., Rodrigues, L., and Pascoal, C. (2015). Responses of Primary Production, Leaf Litter Decomposition and Associated Communities to Stream Eutrophication. Environ. Pollut. 202, 32–40. doi:10.1016/j.envpol.2015.03.014
Elosegi, A., Basaguren, A., and Pozo, J. (2006). A Functional Approach to the Ecology of Atlantic Basque Streams. Limnetica 25, 123–134.
Englert, D., Zubrod, J. P., Schulz, R., and Bundschuh, M. (2013). Effects of Municipal Wastewater on Aquatic Ecosystem Structure and Function in the Receiving Stream. Sci. Total Environ. 454-455, 401–410. doi:10.1016/j.scitotenv.2013.03.025
Ferreira, V., Castagneyrol, B., Koricheva, J., Gulis, V., Chauvet, E., and Graça, M. A. S. (2014). A Meta-Analysis of the Effects of Nutrient Enrichment on Litter Decomposition in Streams. Biol. Rev. 90, 669–688. doi:10.1111/brv.12125
Gómez-Gener, L., Obrador, B., Marcé, R., Acuña, V., Catalán, N., Casas-Ruiz, J. P., et al. (2016). When Water Vanishes: Magnitude and Regulation of Carbon Dioxide Emissions from Dry Temporary Streams. Ecosystems 19, 710–723. doi:10.1007/s10021-016-9963-4
Gómez-Gener, L., Obrador, B., von Schiller, D., Marcé, R., Casas-Ruiz, J. P., Proia, L., et al. (2015). Hot Spots for Carbon Emissions from Mediterranean Fluvial Networks during Summer Drought. Biogeochemistry 125, 409–426. doi:10.1007/s10533-015-0139-7
González, J. M., and Elosegi, A. (2021). Water Abstraction Reduces Taxonomic and Functional Diversity of Stream Invertebrate Assemblages. Freshw. Sci. 40, 524–536. doi:10.1086/716201
González, J. M., Recuerda, M., and Elosegi, A. (2018). Crowded Waters: Short-Term Response of Invertebrate Drift to Water Abstraction. Hydrobiologia 819, 39–51. doi:10.1007/s10750-018-3620-1
Graça, M. A. S. (2001). The Role of Invertebrates on Leaf Litter Decomposition in Streams - a Review. Internat. Rev. Hydrobiol. 86, 383–393. doi:10.1002/1522-2632(200107)86:4/5<383:aid-iroh383>3.0.co;2-d
Grill, G., Lehner, B., Thieme, M., Geenen, B., Tickner, D., Antonelli, F., et al. (2019). Mapping the World's Free-Flowing Rivers. Nature 569, 215–221. doi:10.1038/s41586-019-1111-9
Gücker, B., Brauns, M., and Pusch, M. T. (2006). Effects of Wastewater Treatment Plant Discharge on Ecosystem Structure and Function of lowland Streams. J. North Am. Benthological Soc. 25, 313–329. doi:10.1899/0887-3593(2006)25[313:eowtpd]2.0.co;2
Gulis, V., and Suberkropp, K. (2003). Effect of Inorganic Nutrients on Relative Contributions of Fungi and Bacteria to Carbon Flow from Submerged Decomposing Leaf Litter. Microb. Ecol. 45, 11–19. doi:10.1007/s00248-002-1032-1
Hering, D., Carvalho, L., Argillier, C., Beklioglu, M., Borja, A., Cardoso, A. C., et al. (2015). Managing Aquatic Ecosystems and Water Resources under Multiple Stress - an Introduction to the MARS Project. Sci. Total Environ. 503-504, 10–21. doi:10.1016/j.scitotenv.2014.06.106
Hieber, M., and Gessner, M. O. (2002). Contribution of Stream Detrivores, Fungi, and Bacteria to Leaf Breakdown Based on Biomass Estimates. Ecology 83, 1026–1038. doi:10.1890/0012-9658(2002)083[1026:cosdfa]2.0.co;2
Hornberger, G. M., and Kelly, M. G. (1972). The Determination of Primary Production in a Stream Using an Exact Solution to the Oxygen Balance Equation. J. Am. Water Resour. Assoc 8, 795–801. doi:10.1111/j.1752-1688.1972.tb05222.x
Hotchkiss, E. R., Hall, R. O., Sponseller, R. A., Butman, D., Klaminder, J., Laudon, H., et al. (2015). Sources of and Processes Controlling CO2 Emissions Change with the Size of Streams and Rivers. Nat. Geosci 8, 696–699. doi:10.1038/ngeo2507
Jones, B., and O’Neill, B. C. (2016). Spatially Explicit Global Population Scenarios Consistent with the Shared Socioeconomic Pathways. Environ. Res. Lett. 11, 084003. doi:10.1088/1748-9326/11/8/084003
Keller, P. S., Catalán, N., von Schiller, D., Grossart, H. P., Koschorreck, M., Obrador, B., et al. (2020). Global CO2 Emissions from Dry Inland Waters Share Common Drivers across Ecosystems. Nat. Commun. 11, 2126–2128. doi:10.1038/s41467-020-15929-y
Kelso, J. E., and Baker, M. A. (2020). Organic Matter Is a Mixture of Terrestrial, Autochthonous, and Wastewater Effluent in an Urban River. Front. Environ. Sci. 7, 202. doi:10.3389/fenvs.2019.00202
Kuzmanović, M., Ginebreda, A., Petrović, M., and Barceló, D. (2015). Risk Assessment Based Prioritization of 200 Organic Micropollutants in 4 Iberian Rivers. Sci. Total Environ. 503-504, 289–299. doi:10.1016/j.scitotenv.2014.06.056
Lake, P. S. (2003). Ecological Effects of Perturbation by Drought in Flowing Waters. Freshw. Biol. 48, 1161–1172. doi:10.1046/j.1365-2427.2003.01086.x
Lecerf, A. (2017). Methods for Estimating the Effect of Litterbag Mesh Size on Decomposition. Ecol. Model. 362, 65–68. doi:10.1016/j.ecolmodel.2017.08.011
Liu, S., and Raymond, P. A. (2018). Hydrologic Controls on pCO2 and CO2 Efflux in US Streams and Rivers. Limnol. Oceanogr. Lett. 3, 428–435. doi:10.1002/lol2.10095
Livingston, G. P., and Hutchinson, G. L. (1995). Enclosure-based Measurement of Trace Gas Exchange: Applications and Sources of Error. Biog. Trace Gases Meas. Emiss. Soil Water 51, 14–51.
Luo, Y., and Zhou, X. (2010). Soil Respiration and the Environment. Amsterdam: Elsevier Academy Press.
Magali, B., Sylvain, L., and Eric, C. (2016). Litter Breakdown for Ecosystem Integrity Assessment Also Applies to Streams Affected by Pesticides. Hydrobiologia 773, 87–102. doi:10.1007/s10750-016-2681-2
Mandaric, L., Mor, J.-R., Sabater, S., and Petrovic, M. (2018). Impact of Urban Chemical Pollution on Water Quality in Small, Rural and Effluent-Dominated Mediterranean Streams and Rivers. Sci. Total Environ. 613-614, 763–772. doi:10.1016/j.scitotenv.2017.09.128
Marcé, R., Obrador, B., Gómez-Gener, L., Catalán, N., Koschorreck, M., Arce, M. I., et al. (2019). Emissions from Dry Inland Waters Are a Blind Spot in the Global Carbon Cycle. Earth-Science Rev. 188, 240–248. doi:10.1016/j.earscirev.2018.11.012
Marks, J. C. (2019). Revisiting the Fates of Dead Leaves that Fall into Streams. Annu. Rev. Ecol. Evol. Syst. 50, 547–568. doi:10.1146/annurev-ecolsys-110218-024755
Martí, E., Riera, J. L., and Sabater, F. (2009). “Effects of Wastewater Treatment Plants on Stream Nutrient Dynamics under Water Scarcity Conditions,” in BT - Water Scarcity in the Mediterranean: Perspectives under Global Change. Editors S. Sabater, and D. Barceló (Berlin, Heidelberg: Springer Berlin Heidelberg), 173–195. doi:10.1007/698_2009_33
Martí, E., and Sabater, F. (2009). “Retencion de nutrientes en ecosistemas fluviales,” in Conceptos y técnicas en ecología fluvial. Editors A. Elosegi, and S. Sabater (Bilbao: Fundación BBVA), 117–132. 10261/76865.
Martínez, A., Larrañaga, A., Pérez, J., Casado, C., Casas, J. J., González, J. M., et al. (2017). Climate Modulates the Magnitude of the Effects of Flow Regulation on Leaf-Litter Decomposition. Aquat. Sci. 79, 507–514. doi:10.1007/s00027-016-0513-0
Marx, A., Dusek, J., Jankovec, J., Sanda, M., Vogel, T., van Geldern, R., et al. (2017). A Review of CO2 and Associated Carbon Dynamics in Headwater Streams: A Global Perspective. Rev. Geophys. 55, 560–585. doi:10.1002/2016RG000547
Matthaei, C. D., Piggott, J. J., and Townsend, C. R. (2010). Multiple Stressors in Agricultural Streams: Interactions Among Sediment Addition, Nutrient Enrichment and Water Abstraction. J. Appl. Ecol. 47, 639–649. doi:10.1111/j.1365-2664.2010.01809.x
Medeiros, A. O., Pascoal, C., and Graça, M. A. S. (2009). Diversity and Activity of Aquatic Fungi under Low Oxygen Conditions. Freshw. Biol. 54, 142–149. doi:10.1111/j.1365-2427.2008.02101.x
Millero, F. J. (1995). Thermodynamics of the Carbon Dioxide System in the Oceans. Geochimica et Cosmochimica Acta 59, 661–677. doi:10.1016/0016-7037(94)00354-o
Mor, J.-R., Dolédec, S., Acuña, V., Sabater, S., and Muñoz, I. (2019). Invertebrate Community Responses to Urban Wastewater Effluent Pollution under Different Hydro-Morphological Conditions. Environ. Pollut. 252, 483–492. doi:10.1016/j.envpol.2019.05.114
Murphy, J., and Riley, J. P. (1962). A Modified Single Solution Method for the Determination of Phosphate in Natural Waters. Analytica Chim. Acta 27, 31–36. doi:10.1016/S0003-2670(00)88444-5
Northington, R. M., and Webster, J. R. (2017). Experimental Reductions in Stream Flow Alter Litter Processing and Consumer Subsidies in Headwater Streams. Freshw. Biol. 62, 737–750. doi:10.1111/fwb.12898
Osorio, V., Larrañaga, A., Aceña, J., Pérez, S., and Barceló, D. (2016). Concentration and Risk of Pharmaceuticals in Freshwater Systems Are Related to the Population Density and the Livestock Units in Iberian Rivers. Sci. Total Environ. 540, 267–277. doi:10.1016/j.scitotenv.2015.06.143
Pennington, D. N., Hansel, J. R., and Gorchov, D. L. (2010). Urbanization and Riparian Forest Woody Communities: Diversity, Composition, and Structure within a Metropolitan Landscape. Biol. Conservation 143, 182–194. doi:10.1016/j.biocon.2009.10.002
Pereda, O., Solagaistua, L., Atristain, M., de Guzmán, I., Larrañaga, A., von Schiller, D., et al. (2020). Impact of Wastewater Effluent Pollution on Stream Functioning: A Whole-Ecosystem Manipulation Experiment. Environ. Pollut. 258, 113719. doi:10.1016/j.envpol.2019.113719
Pérez-Marrero, J. (2017). Catálogo de presas españolas anteriores a 1926 asociadas a procesos industriales. Tomo IV.
Petersen, R. C., and Cummins, K. W. (1974). Leaf Processing in a Woodland Stream*. Freshw. Biol 4, 343–368. doi:10.1111/j.1365-2427.1974.tb00103.x
Piggott, J. J., Townsend, C. R., and Matthaei, C. D. (2015). Reconceptualizing synergism and antagonism among multiple stressors. Ecol. Evol. 5, 1538–1547.
Poff, N. L., and Hart, D. D. (2002). How Dams Vary and Why it Matters for the Emerging Science of Dam Removal. Bioscience 52, 659–668. doi:10.1641/0006-3568(2002)052[0659:hdvawi]2.0.co;2
Prasad, M. B. K., Kaushal, S. S., and Murtugudde, R. (2013). Long-term pCO2 Dynamics in Rivers in the Chesapeake Bay Watershed. Appl. Geochem. 31, 209–215. doi:10.1016/j.apgeochem.2013.01.006
Reardon, J., Foreman, J. A., and Searcy, R. L. (1966). New Reactants for the Colorimetric Determination of Ammonia. Clinica Chim. Acta 14, 403–405. doi:10.1016/0009-8981(66)90120-3
Ribot, M., von Schiller, D., Sabater, F., and Martí, E. (2015). Biofilm Growth and Nitrogen Uptake Responses to Increases in Nitrate and Ammonium Availability. Aquat. Sci. 77, 695–707. doi:10.1007/s00027-015-0412-9
Riis, T., Levi, P. S., Baattrup-Pedersen, A., Jeppesen, K. G., and Rosenhøj Leth, S. (2017). Experimental Drought Changes Ecosystem Structure and Function in a Macrophyte-Rich Stream. Aquat. Sci. 79, 841–853. doi:10.1007/s00027-017-0536-1
Riveros-Iregui, D. A., and McGlynn, B. L. (2009). Landscape Structure Control on Soil CO2 efflux Variability in Complex Terrain: Scaling from point Observations to Watershed Scale Fluxes. J. Geophys. Res. 114, a–n. doi:10.1029/2008JG000885
Romaní, A. M., Chauvet, E., Febria, C., Mora-Gómez, J., Risse-Buhl, U., Timoner, X., et al. (2017). “The Biota of Intermittent Rivers and Ephemeral Streams: Prokaryotes, Fungi, and Protozoans,” in Intermittent Rivers and Ephemeral Streams (Elsevier), 161–188. doi:10.1016/b978-0-12-803835-2.00009-7
Schinegger, R., Trautwein, C., Melcher, A., and Schmutz, S. (2012). Multiple Human Pressures and Their Spatial Patterns in European Running Waters. Water Environ. J. 26, 261–273. doi:10.1111/j.1747-6593.2011.00285.x
Schlief, J., and Mutz, M. (2009). Effect of Sudden Flow Reduction on the Decomposition of Alder Leaves (Alnus glutinosa [L.] Gaertn.) in a Temperate lowland Stream: A Mesocosm Study. Hydrobiologia 624, 205–217. doi:10.1007/s10750-008-9694-4
Schmutz, S., and Moog, O. (2018). “Riverine Ecosystem Management,” in Science for Governing towards a Sustainable Future. Editors S. Schmutz, and J. Sendzimir (Cham (Switzerland): Springer Nature). doi:10.1007/978-3-319-73250-3
Striegl, R. G., and Michmerhuizen, C. M. (1998). Hydrologic Influence on Methane and Carbon Dioxide Dynamics at Two north-central Minnesota Lakes. Limnol. Oceanogr. 43, 1519–1529. doi:10.4319/lo.1998.43.7.1519
Syvitski, J. P. M., Vörösmarty, C. J., Kettner, A. J., and Green, P. (2005). Impact of Humans on the Flux of Terrestrial Sediment to the Global Coastal Ocean. Science 308, 376–380. doi:10.1126/science.1109454
Teodoru, C. R., Prairie, Y. T., and Del Giorgio, P. A. (2011). Spatial Heterogeneity of Surface CO2 Fluxes in a Newly Created Eastmain-1 Reservoir in Northern Quebec, Canada. Ecosystems 14, 28–46. doi:10.1007/s10021-010-9393-7
Tiegs, S. D., Costello, D. M., Isken, M. W., Woodward, G., McIntyre, P. B., Gessner, M. O., et al. (2019). Global Patterns and Drivers of Ecosystem Functioning in Rivers and Riparian Zones. Sci. Adv. 5, eaav0486. doi:10.1126/sciadv.aav0486
Tockner, K., Uehlinger, U., and Robinson, C. T. (2009). Rivers of Europe. San Diego: Academic Press Elsevier.
URA (2016). Red de seguimiento del estado biológico de los ríos de la CAPV. Informe de resultados campaña 2016.
von Schiller, D., Bernal, S., Dahm, C. N., and Martí, E. (2017). “Nutrient and Organic Matter Dynamics in Intermittent Rivers and Ephemeral Streams,” in Intermittent Rivers and Ephemeral Streams (Elsevier), 135–160. doi:10.1016/B978-0-12-803835-2.00006-1
Vörösmarty, C. J., McIntyre, P. B., Gessner, M. O., Dudgeon, D., Prusevich, A., Green, P., et al. (2010). Global Threats to Human Water Security and River Biodiversity. Nature 467, 555–561. doi:10.1038/nature09440
Walters, A. W. (2011). Resistance of Aquatic Insects to a Low-Flow Disturbance: Exploring a Trait-Based Approach. J. North Am. Benthological Soc. 30, 346–356. doi:10.1899/10-041.1
Wanninkhof, R. (1992). Relationship between Wind Speed and Gas Exchange over the Ocean. J. Geophys. Res. 97, 7373–7382. doi:10.1029/92jc00188
Wardle, D. A., Bardgett, R. D., Klironomos, J. N., Setälä, H., van der Putten, W. H., and Wall, D. H. (2004). Ecological Linkages between Aboveground and Belowground Biota. Science 304, 1629–1633. LP – 1633. doi:10.1126/science.1094875
Weiss, R. F. (1974). Carbon Dioxide in Water and Seawater: the Solubility of a Non-ideal Gas. Mar. Chem. 2, 203–215. doi:10.1016/0304-4203(74)90015-2
Woodward, G., Gessner, M. O., Giller, P. S., Gulis, V., Hladyz, S., Lecerf, A., et al. (2012). Continental-scale Effects of Nutrient Pollution on Stream Ecosystem Functioning. Science 336, 1438–1440. doi:10.1126/science.1219534
Keywords: hydropower, water diversion, pollution, multiple stressors, dry riverbeds, organic matter decomposition, carbon dioxide flux
Citation: Pérez-Calpe AV, de Guzman I, Larrañaga A, von Schiller D and Elosegi A (2022) Organic Matter Processing on Dry Riverbeds is More Reactive to Water Diversion and Pollution Than on Wet Channels. Front. Environ. Sci. 9:817665. doi: 10.3389/fenvs.2021.817665
Received: 18 November 2021; Accepted: 29 December 2021;
Published: 14 February 2022.
Edited by:
Silvia Quadroni, University of Insubria, ItalyCopyright © 2022 Pérez-Calpe, de Guzman, Larrañaga, von Schiller and Elosegi. This is an open-access article distributed under the terms of the Creative Commons Attribution License (CC BY). The use, distribution or reproduction in other forums is permitted, provided the original author(s) and the copyright owner(s) are credited and that the original publication in this journal is cited, in accordance with accepted academic practice. No use, distribution or reproduction is permitted which does not comply with these terms.
*Correspondence: Ana Victoria Pérez-Calpe, YW5hdmljdG9yaWEucGVyZXpAZWh1LmV1cw==