- Water, Environment and Agriculture Program, Melbourne School of Engineering, University of Melbourne, Melbourne, VIC, Australia
Climate change presents severe risks for the implementation and success of environmental flows worldwide. Current environmental flow assessments tend to assume climate stationarity, so there is an urgent need for robust environmental flow programs that allow adaptation to changing flow regimes due to climate change. Designing and implementing robust environmental flow programs means ensuring environmental objectives are achieved under a range of uncertain, but plausible climate futures. We apply stress testing concepts previously adopted in water supply management to environmental flows at a catchment scale. We do this by exploring vulnerabilities in different river management metrics for current environmental flow arrangements in the Goulburn River, Australia, under non-stationary climatic conditions. Given the limitations of current environmental flows in supporting ecological outcomes under climate change, we tested three different adaptation options individually and in combination. Stress testing adaptation results showed that increasing environmental entitlements yielded the largest benefits in drier climate futures, whereas relaxing river capacity constraints (allowing more targeted delivery of environmental water) offered more benefits for current and wetter climates. Combining both these options led to greater than additive improvements in allocation reliability and reductions in environmental water shortfalls, and these improvements were achieved across a wider range of climatic conditions than possible with either of the individual options. However, adaptation may present additional risks to some ecological outcomes for wetter climates. Ultimately, there was a degree of plausible climate change beyond which none of the adaptation options considered were effective at improving ecological outcomes. This study demonstrates an important step for environmental flow assessments: evaluating the feasibility of environmental outcomes under climate change, and the intervention options that prove most robust under an uncertain future.
1 Introduction
The implementation of environmental flows to restore river ecosystems from anthropogenic degradation is growing globally (Arthington et al., 2018). However, climate change presents further challenges for environmental water management and freshwater ecosystems (Poff and Matthews, 2013; IPCC, 2014; Horne et al., 2019). Despite the high uncertainties involved, there is a need to test environmental water management practices and assess how they perform under different climate regimes (Poff et al., 2016). It may be that current environmental flows objectives cannot be sustained under current policy settings without significant adaptations, this issue). Interventions designed to address an existing problem, such as river regulation, may not fulfil the desired outcomes under a different climate future (Poff, 2018). This is a challenge for environmental flow implementation and may jeopardize the value of investments in environmental flows. This paper explores these issues using the Goulburn River, Australia as a case study through the evaluation of different environmental flow outcomes under a range of plausible climate futures and adaptation options.
Current environmental water planning in many parts of the world adjusts flow delivery from year to year (Rayner et al., 2009; Poff et al., 2010; Opperman et al., 2019). High flow components are usually delivered in wetter years and critical low flow refuge habitats protected in dry years when availability of environmental water is low. Environmental flow strategies generally set out objectives based on the assumption of climate stationarity which provides an estimate of the frequency with which different environmental flow components can be achieved (Horne et al., 2019). Environmental flows have been implemented around the world under varying degrees of sophistication in locations such as Africa (Brown and King, 2012), Canada (El-Jabi and Caissie, 2019), Europe (Mezger et al., 2019) and Asia (Chen and Wu, 2019). In many cases these regions are projected to experience wetter conditions under climate change (IPCC, 2013). However, drying climates are projected with varying levels of uncertainty for many parts of the world with environmental water programs such as south-east Australia, western United States and the Mediterranean (IPCC, 2013). If climate change is neglected in environmental water planning, then strategies based on annual water availability will fail to deliver the intended long term flow regime, and particularly the frequency of higher flow events. If this shift is ignored there will be a decline in environmental condition and potentially poor targeting of environmental water outcomes.
There is a need to anticipate the kind of impact climate might have on environmental water outcomes and to develop strategies to address vulnerabilities. However, this can be challenging for complex river systems given large uncertainties in climate projections. Climate stress testing methods (Brown et al., 2012; Brown and Wilby, 2012) have evolved to assess system performance for varying degrees of climate change that may not be captured by scenario-based climate projections. Generally referred to as “bottom-up” methods, they differ from scenario assessments by beginning with an exploration of system performance and vulnerability to changes in inputs (such as precipitation or temperature). To date, most riverine applications of these methods have been limited to assessing water supply vulnerability (e.g., Turner et al., 2014; Steinschneider et al., 2015; Henley et al., 2019), but they offer promising utility to assess ecological outcomes in a way that accommodates climate uncertainty (John et al., 2020). Robust interventions are needed if regulated rivers are to adapt to uncertain climate futures. Stress testing methods can help identify robust solutions by assessing which options deliver benefits over a wider range of potential climate changes (Poff et al., 2016), and can be used to gain insights into wider system performance before and after intervention (Weaver et al., 2013).
In this study, we tested the effectiveness of three different intervention options to improve environmental water outcomes in the Goulburn River for current conditions and a range of plausible climatic changes. The three options were: increasing the environmental water entitlement, relaxing river capacity constraint issues (i.e., removing barriers to environmental water delivery), and increasing the priority of some environmental flow components so they are delivered outside of the normal water allocation process. We did this by using a stress testing methodology in which the effectiveness of each individual option (and combination of options) was assessed under various degrees of climate changes to gauge robustness. Although these adaptation options are primarily designed to improve freshwater ecosystem outcomes, we compared how they perform for several key system metrics including reliability of water supply, shortfall in meeting environmental water demands, and stabilizing the long-term condition of ecological endpoints.
2 Methods
2.1 Case Study Approach—Adaptation in the Goulburn River, Australia
The Australian government’s Murray Darling Basin Plan represents a multi-billion-dollar investment in water recovery to support the delivery of environmental flows (Hart, 2016). This has delivered substantial volumes of water to the environment in the form of water entitlements (property rights with the same conditions as consumptive water rights). In the Goulburn River in northern Victoria, Basin Plan recovery has included nearly 400 GL/year (400 Hm3/year) of high reliability water (∼30% of storage and tributary inflows). However, this recovery target was established assuming stationary climate conditions with little consideration given to climate change (Prosser et al., 2021). Although there is large uncertainty, future projections for the region typically predict lower water availability through increased temperatures and decreased cool season rainfall (Timbal and Jones, 2008; Timbal and Drosdowsky, 2013). Reduced natural flows due to climate change will increase the demand for environmental water but reduce available supply through lower water allocations. In historic dry periods, environmental water managers typically focus on low flows to sustain key habitats, in line with expectations on environmental water availability and tributary inflows (Horne et al., 2020).
There are also barriers to the effective delivery of environmental water. In the Goulburn River, capacity issues relating to flooding concerns means not all flow recommendations can be met. These capacity issues currently limit controlled regulated releases to 10,000 ML/d (116 m3/s), which is less than half of the flow recommendations for overbank flows. “Piggybacking” (supplementing unregulated tributary flow pulses with regulated releases) can be used to provide higher flows, but non-linear routing considerations and existing management arrangements mean it is difficult in practice to time reservoir releases to best utilize tributary inflows (Kaur et al., 2019).
2.2 Case Study Region and Characteristics
The Goulburn River basin in northern Victoria, Australia, supports around AUD$1.4b in irrigated agricultural production annually. The Goulburn catchment spans 1.6 m Ha on the lands of both the Yorta Yorta and Taungurung traditional owner nations. The Goulburn River is notable because it provides 10% of annual flows in the wider Murray-Darling basin despite occupying only 2% of the land area. There are two major regulation structures on the Goulburn River, Lake Eildon (3,300 GL storage) in the upstream reaches and Goulburn Weir, which diverts water to an offline storage at Waranga Basin (430 GL) further downstream.
Water is allocated to users proportionately based on water entitlements that fit into two categories: high and low security shares. The focus of this paper is on high security shares as this is the bulk of consumptive water use. Seasonal allocation volumes are set such that all high security users receive a proportionate share of their entitlement (i.e., all users receive the same percentage of their entitlement based on seasonal water availability), and these are updated throughout the water year.
The Goulburn Broken Catchment Management Authority manages water entitlements on behalf of the environment. There are nearly 400 GL of high security environmental water rights in the Goulburn River, which are used to meet local environmental objectives and downstream environmental flows in the Murray River. There are also passing environmental flow requirements from Lake Eildon and Goulburn Weir ensuring minimum flow conditions are met.
There are various ecological values along the river including important fish species such as Murray Cod (Maccullochella peelii) and Golden Perch (Macquaria ambigua), and floodplain water regimes maintaining River Red Gum (Eucalyptus camaldulensis) forests. The most targeted reaches for environmental managers are those downstream of Goulburn Weir. This section of the river is known as Kaiela in the language of the Yorta Yorta traditional owners. Key features of the river basin are shown in Figure 1.
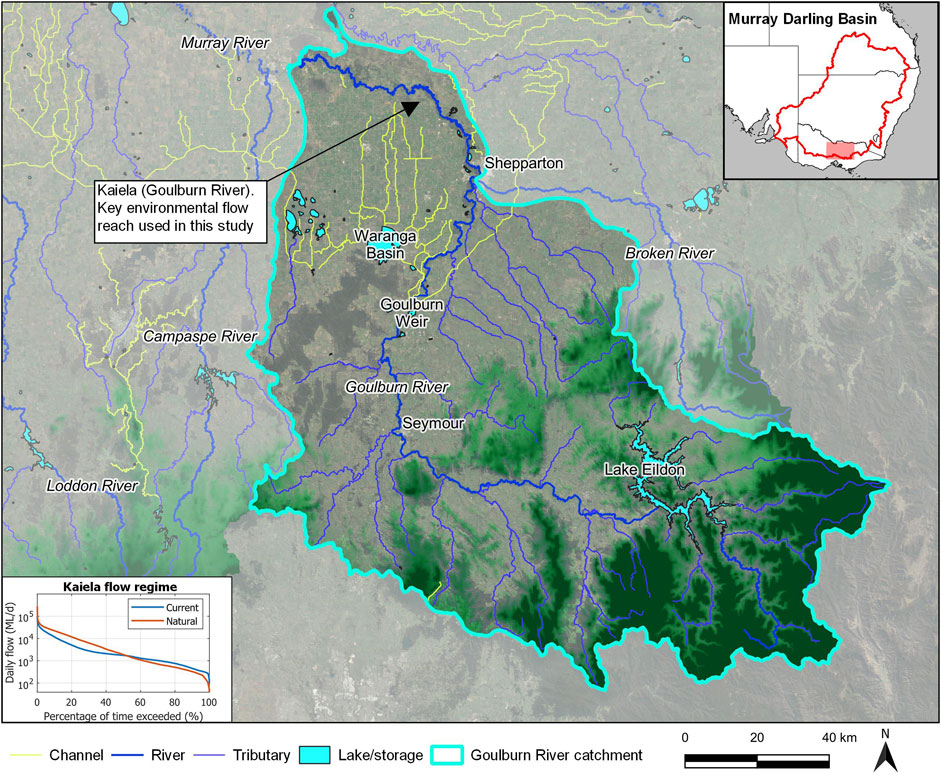
FIGURE 1. Case study catchment of the Goulburn River basin. The flow duration inset describes Kaiela flow conditions in current and “natural” (modelled without regulation structures and diversions) conditions. Compared to more natural conditions, rarer flows are lower in magnitude, and more common flows are higher in magnitude for the current regulated regime.
From a water management perspective, one of the more challenging features of the Goulburn River basin is the large quantity of water transferred downstream as inter-valley transfers. These transfers are used to supply irrigation demands much further downstream (up to 500 km) along the Murray River. The majority of this water is delivered over summer months to align with seasonal irrigation needs. Inter valley transfer volumes have grown substantially from 99 GL in 2012 to a peak of 433 GL in 2018. This now presents both an opportunity and risk for freshwater ecology. Inter valley transfers can be timed to meet some ecological demands along the lower Goulburn River, but these also substantially increase summer flows leading to severe negative impacts on bank stability and bank vegetation (Wood et al., 2021).
The main environmental flow components are summarized in Table 1. Environmental water plans change from 1 year to the next depending on prevailing climate conditions and ecological priorities. For example, in dry years water managers will prioritize low flow components to provide drought refuge habitat and delay some flow deliveries to guarantee critical supplies for the following year.
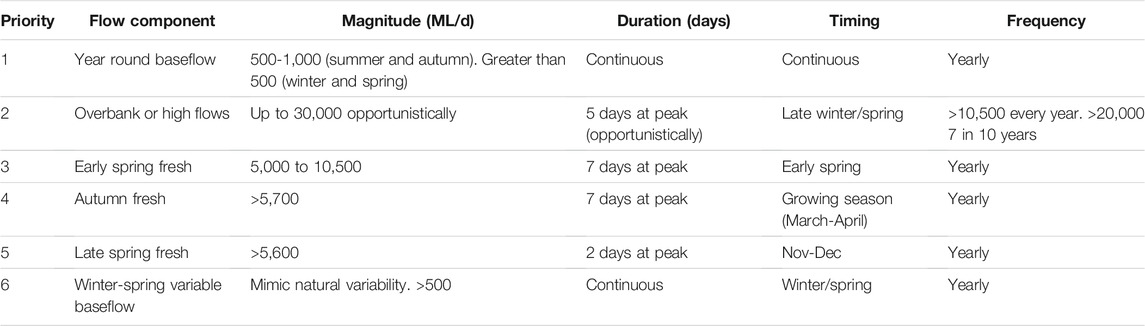
TABLE 1. Key environmental flow components in Kaiela (Goulburn River). Adapted from Horne et al. (2020).
2.3 Modelling Approach
The overall modelling framework used in this study is summarized in Figure 2, and individual method components are further discussed below. Briefly, the modelling steps comprised three main components. First, a stochastic data generation model was used to derive stochastic climate inputs (monthly precipitation, temperature, and potential evapotranspiration) that are representative of plausible future conditions. These were input into a conceptual rainfall-runoff model to provide monthly time series of reservoir and tributary inflows. A water resource system model was then used to simulate monthly water allocations, demands, diversions, flows along river reaches, and environmental flow releases. Finally, ecological models were used to project ecological condition for 12 different endpoints at the key environmental reaches. These models are sensitive to daily flow statistics, so a disaggregation method (John et al., 2021b) was used to estimate daily flows at this site. The analysis was repeated for each individual adaptation option and for different combinations of options.
2.4 Stochastic Data Generation and Climate Change
We used a stress-testing approach that models system performance under various combinations of possible climatic changes that go beyond the ranges and combinations of changes projected by general circulation models (GCMs). The benefit of this approach is that it allows the performance of current and alternative adaptation options to be assessed over a wider range of joint climate changes than is possible using more traditional scenario-based climate projections (Brown and Wilby, 2012). Whilst stress-testing does not require stochastically generated data, the use of stochastic data facilitates the exploration of system performance under plausible climatic sequences that have not been observed in the historic records (such as multi-year droughts), which may include hysteretic behavior that varies with antecedent conditions (John et al., 2021a). Many ecological processes and rates of recovery and decline are sensitive to specific flow sequences and differing antecedent conditions (Shenton et al., 2012; Wang et al., 2018; Wheeler et al., 2018).
Timeseries of monthly precipitation and monthly average daily maximum temperature were generated using the stochastic stress testing framework developed. This framework allows for perturbations of long-term precipitation and temperature statistics to produce stochastic scenarios that are representative of changes in future climate. The adopted framework includes options for simulating changes to long-term average precipitation, precipitation seasonality, low-frequency variability of precipitation, long-term average temperatures and non-stationary runoff responses [temporary or permanent changes in the relationship between rainfall and runoff linked to long-term drought (Saft et al., 2015)]. Here, we only simulate changes to long-term annual precipitation and temperature. Previous work has shown that other changes may impact on ecological outcomes, but we have limited the analysis for the following reasons: Ecological outcomes in the regulated reaches of the Goulburn River are more sensitive to changes in long-term average precipitation and temperature than other climate variables; projections of changes in rainfall-runoff relationships, whilst potentially very significant for ecological outcomes, are highly speculative and subject to considerable uncertainty; current policy guidelines for assessing the impact of climate change on water resources developed by the state water agency focus solely on changes in long-term annual rainfall and temperature.
The bounds on changes in long-term average precipitation and temperature were informed by an ensemble of 37 GCM projections from CMIP5 (Taylor et al., 2012). Climate model outputs were bias-corrected using an annual quantile-quantile scaling approach (Johnson and Sharma, 2011), and then change factors were calculated by comparing the running mean of 30-year periods centered around a given year to a baseline set at 1980–2009. For example, the change factor for 2065 represents the mean of the period 2050–2079 relative to the mean of 1980–2009. These change factor projections for the Goulburn River basin for each individual GCM and the multi-model mean are shown in Figure 3. Stress testing bounds were extended a short way beyond the total envelope of change factors from climate projections up to 2065. We tested changes in long-term average precipitation from −30 to 15% of the baseline, and increases in temperature from 0 to 4°C. These range of changes are referred to as the tested “climate space.”
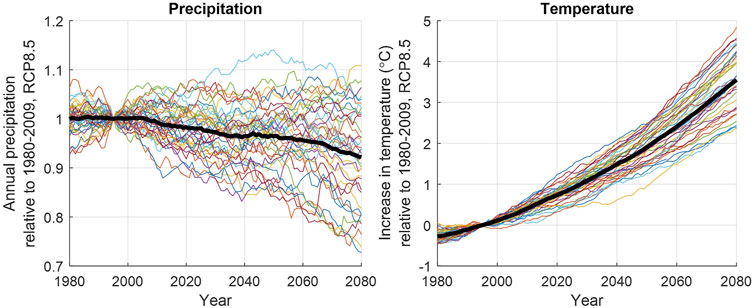
FIGURE 3. Climate model projections for annual precipitation and temperature for the Goulburn River basin for RCP8.5. Each colored line is a CMIP5 model projection. The bold black line is the multi-model-mean projection.
Later, in presenting stress testing results, we used change factors for centered around 2040 and 2065 for two emissions scenarios of RCP4.5 and RCP8.5. This is done to contextualize GCM climate projections with the modelled climate change space as part of the stress test.
2.5 Rainfall-Runoff Modelling
The WAPABA model (Wang et al., 2011) was used to simulate monthly rainfall-runoff responses. This model was selected as it has been found to outperform other monthly models (and some daily models) in simulating monthly streamflow in Australian catchments, and in this region specifically (Wang et al., 2011). The WAPABA model was calibrated using an approach which explicitly accounts for model behavior over multi-year wet and dry periods in the historic record (Fowler et al., 2016), separate calibrations were undertaken for different sub-areas of the Goulburn catchment (reflecting hydrological differences over the 1.6 m Ha area) and these were recombined spatially as required to provide the inflows for the different inflow points of the water resource model.
2.6 Water Resource Modelling
A simplified model of the river system was used to predict flows at various key areas in the regulated river network. This model was based on the state water agency’s detailed daily planning model, but with reduced spatial detail and operating on a monthly timestep. The model was calibrated to ensure adequate representation of the flow regime at key ecological flow reaches. The simplified model was necessary to reduce the time needed to undertake the stress tests, each of which requires thousands of model runs. Since ecological outcomes are sensitive to daily flow patterns, a disaggregation scheme (John et al., 2021b) was used to produce daily outputs from monthly flow data at specific river reaches. The disaggregation scheme considers both antecedent catchment wetness as well as flow magnitude, and has been shown to outperform daily rainfall-runoff modelling for a variety of purposes, but especially for modelling ecologically relevant flow statistics under a non-stationary climate (John et al., 2021b). Regulated dam releases for irrigation deliveries and bulk inter-valley water transfers (but not for environmental water releases) were disaggregated uniformly as typically their within-month variability is low.
2.7 Metrics to Define System Performance
The Goulburn River is managed for multiple objectives—mainly irrigation water supply and environmental outcomes. Environmental flows are predominantly provided through water entitlements, and the availability of environmental water is thus also a function of system reliability. Accordingly, we investigated current system performance and the effect of adaptation options on the reliability of high security water shares, as this is representative of the general reliability for water users. Reliability in this instance was represented by the proportion of years that allocations are fully provided.
We used two different metrics to assess freshwater ecosystem outcomes. The first metric is simply the average annual volume of environmental water shortfall. This shortfall is calculated by subtracting the modelled river flows from the sum of annual flow environmental components. This metric was selected as it has formed the basis for setting environmental water recovery targets in the basin (Murray–Darling Basin Authority, 2010). Ideally, environmental water demands are fully met (or exceeded) each year, but low water allocations or constraints on delivery mean that not all flow recommendations can be met.
The second, more complex metric is derived using sequence-based ecological models that explicitly calculate ecological condition through time (Bond et al., 2018; Horne et al., 2018b; Horne et al., in prep, this issue). These models have been developed for 12 different ecological endpoints reflecting key river values identified in the most recent environmental flow recommendations update (see Table 2 for short description, and Supplementary Material for more detail). The models use conditional probability networks which take certain flow components and other phenological inputs (such as habitat quality and food abundance) and project how ecological processes, and ultimately the ecological condition of the endpoints, respond (Horne et al., 2018b). The models are sensitive to different hydrological sequences since their condition at a point in time also depends on conditions in the previous time step. Some models are dependent on the outputs of others, such as fish recruitment rely on macroinvertebrate outcomes (as a source of food). All models were structured and parameterized using a combination of expert elicitation and data integration using observations where possible. Projected ecological condition is updated at an annual time step (Horne et al., 2020).
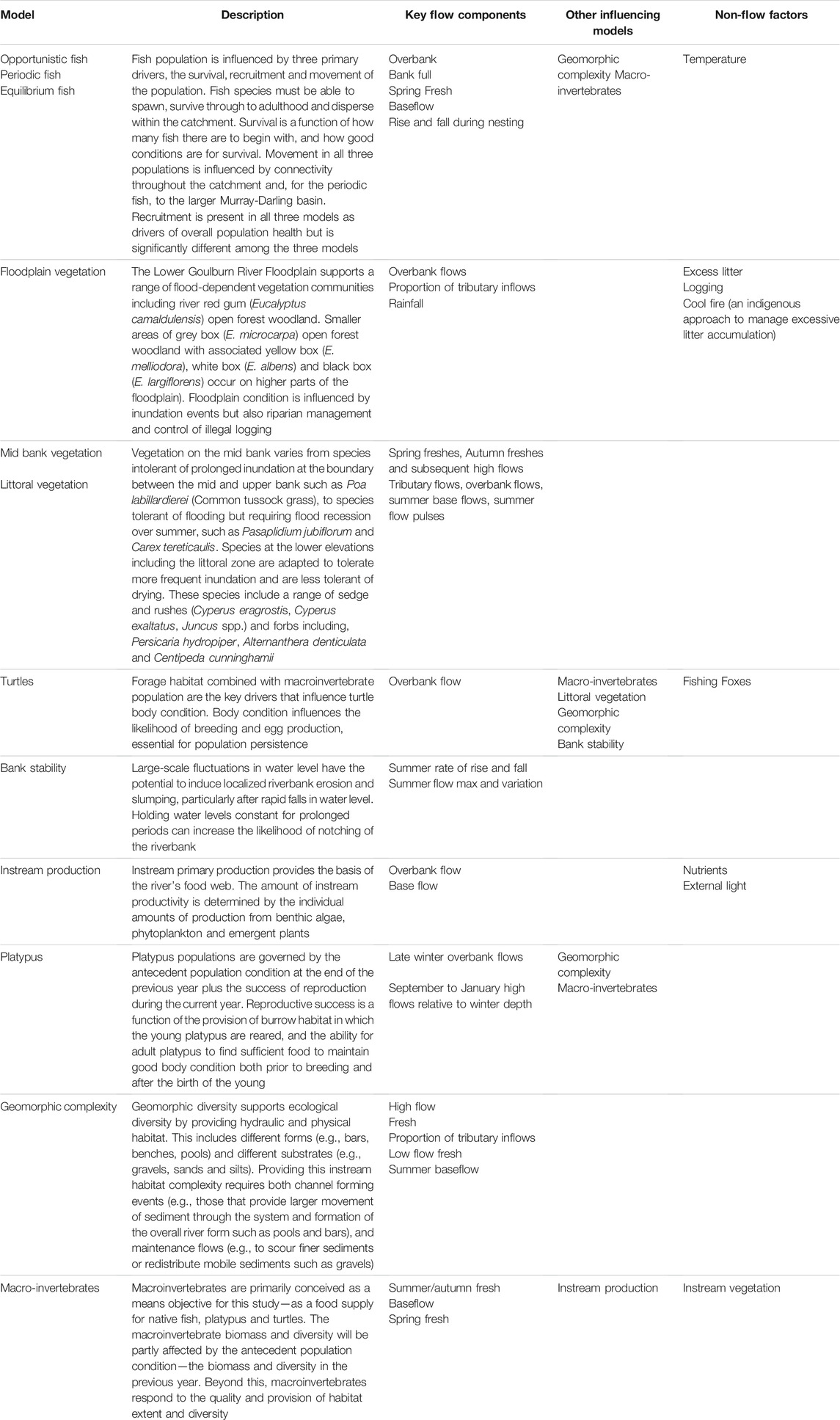
TABLE 2. Models of twelve key ecological endpoints for the Goulburn River, including the flow components they rely on to support individual ecological processes.
To summarize the results of twelve difference ecological model outcomes in one metric, we report the number of models inside a tolerability range for each climatic simulation. The tolerability range for each ecological endpoint was set according to the range of behavior found under a set of baseline conditions. An example of this calculation is shown below (Figure 4), but the method follows that of Nathan et al. (2019). The baseline was established using the distribution of ecological outcomes simulated using 100 replicates of stochastic streamflows. For each climatic scenario considered, the distribution of outcomes across 100 replicates was compared to the baseline and the proportion of the non-overlapping portion of this distribution estimated. The tolerability limit is set at −0.5. Whilst this does not represent ecological tolerability in absolute terms (i.e., strict physical limits to species survival), it does represent the point at which the influence from climate change on ecological outcomes will exceed the influence from natural climate variability based on current river management and operating rules. Such a metric was chosen as it allows direct comparison across the twelve models. It is also theoretically sound in the assumption that impacts will be felt more acutely when the range of conditions faced under future climate departs from the range typically experienced (Horne et al., 2019).
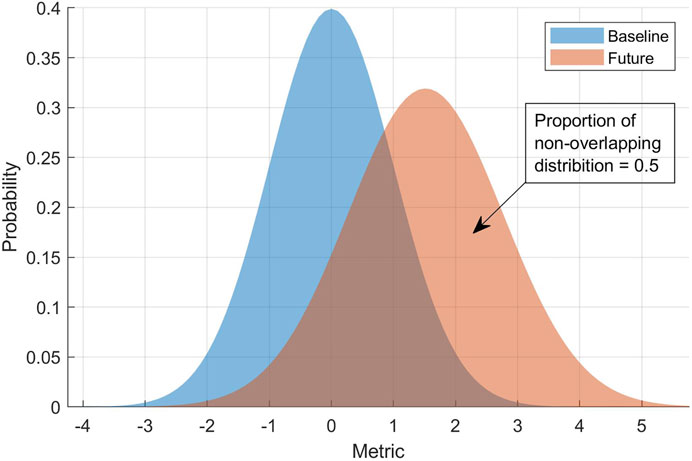
FIGURE 4. Illustration of how the tolerability range is calculated, after Nathan et al. (2019). When the proportion of the non-overlapping future distribution is greater than 0.5, the dominant influence on the distribution of outcomes shifts from existing (or natural) variability to the imposed (climate) change.
For the reliability and environmental water shortfall metrics, 50 replicates of 50 years long were generated for each combination of precipitation and temperature change. The mean of the 50 replicates was then used as the response. We used more replicates for the ecological models (100) to better characterize the distributions, but shorter sequences of 20 years were used as these are more relevant for management considerations and more closely align with the longest critical phenological period in the ecological models (generally floodplain vegetation).
2.8 Adaptation Options
Three different adaptation options were developed in cooperation with water management agencies to improve environmental water outcomes in different climatic regimes. These are summarized in Table 3 and further described below.
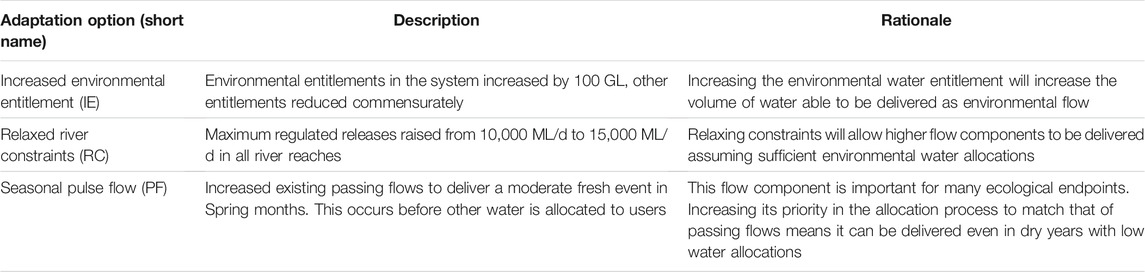
TABLE 3. Summary of different adaptation options trialed in this study. Options are modelled individually and in all combinations.
The first option involved increasing the environmental water entitlement by recovering water from other users in the system. This option increases the total pool of annual environmental water by 100 GL and reduces other water entitlements proportionately.
The second option attempts to improve the effectiveness of the environmental water delivery by relaxing river capacity issues. Existing arrangements mean controlled releases downstream of both Lake Eildon and Goulburn weir are limited to approximately 10,000 ML/d. This option assumes these limits can be raised to 15,000 ML/d, thereby allowing the delivery of higher flow pulses assuming sufficient water allocations.
The third option involves a change to environmental water management policy. There are existing minimum passing flow requirements downstream of Goulburn Weir that are delivered outside of the environmental water allocation process. This option increases seasonal passing flows to deliver a fresh event of moderate magnitude in the winter/spring months, which is an important flow component for multiple ecological endpoints. The precise timing of such events depends on water availability and environmental demands which vary year to year. It can be delivered over the July to October period, as there is some flexibility in the timing of spring pulses (see Table 1), and at a peak magnitude of 3,000 ML/d, not including additional tributary flows.
All options were modelled individually and in all possible permutations, giving a total of seven adaptation scenarios and current conditions. Options are abbreviated as follows: increased entitlement (IE); relaxed constraints (RC) and seasonal passing flows (PF). If a combination of options is discussed it uses the requisite combined abbreviation (i.e., IERC for the combination of increased entitlement and relaxed constraints).
3 Results
The results of the stress test are first presented for the three metrics of water supply reliability, environmental water shortfalls and the ecological model outcomes under baseline (i.e., no adaptation) conditions. Next, the results for the three metrics are presented for each individual adaptation option, and these are followed by the results obtained for different combinations of the options. Only a selected set of results are presented here in order to illustrate the main findings but results for all combinations of options are presented in Supplementary Material.
3.1 Current System Stress Test
System performance under current conditions (no adaptation) is shown in Figure 5 for the three different response metrics. Allocation reliability for the current climate is about 91% (see highlighted value at (0, 0) in Figure 5A). System reliability is more sensitive to changes in precipitation than temperature, indicated by the near vertical contours in Figure 5A, and this is generally true for all output metrics. Sensitivity of reliability to changes in climate increases once mean annual precipitation drops to ∼−10%, as is seen in the decrease in contour widths. This variable sensitivity of reliability is significant because a large number of climate model outputs for both emissions scenarios are clustered in this region. System reliability is nearly zero for the driest and warmest combination of tested climate changes (top left-hand corner, Figure 5A).
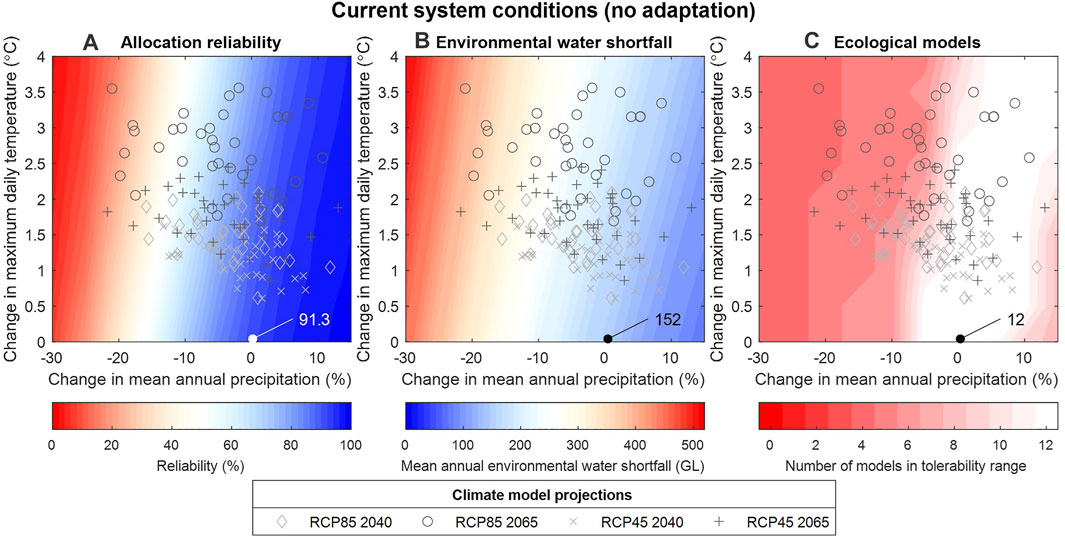
FIGURE 5. Stress testing results for current system conditions (no adaptation) according to three output metrics: (A) allocation reliability; (B) environmental water shortfalls; and (C) ecological model responses. Note the x and y-axes are identical for all three plots. GCM outputs are plotted over failure surfaces for two time periods and two emissions scenarios. Also highlighted is the system performance in the absence of climate change (0, 0).
There are many similarities between the stress test results for environmental water shortfalls and reliability since available environmental water volume depends on water allocations in the system. Despite the large environmental water entitlement, there is still on average 152 GL/year of shortfalls in meeting flow recommendations in current climatic conditions (see 0, 0 in Figure 5B). Most shortfalls occur during the wetter periods, demonstrating river capacity issues in delivering higher flows in wetter years alongside other irrigation transfers.
The ecological models show a clear window of favorable conditions for different climates (vertical white band in Figure 5C). In some cases, significantly wetter conditions are intolerable (pink area to the right of the white band—models affected were bank stability, littoral vegetation, platypus, and instream production). However, the major mode of intolerability is too little water, not too much, as evidenced by the large darker pink area to the left of Figure 5C. Typically, models that are stressed under wetter conditions also benefit from drier conditions, which explains why even under extremely dry conditions not all 12 models are outside of the tolerability range. Changes in mean annual precipitation of ∼−10% are significant as most models are outside tolerability limit of −0.5 for these drier conditions. The threshold of precipitation change that causes models to leave the tolerability range is slightly modulated by changes in temperature, with temperature increases reducing the threshold. Beyond ∼−20% changes in mean annual precipitation the maximum number of models (eight) are stressed. The ecological model outputs may be more useful for directly assessing ecological outcomes as they offer more direct information on the ecological significance of climate change compared to the environmental water shortfall metric.
It is worth further investigating the extent of existing river capacity issues. This can be done by looking at what is driving the environmental water shortfalls, especially for wetter conditions when water resources should be plentiful. Figure 6 shows a similar stress test output to Figure 5 but describing constrained water delivery (i.e., allocated environmental water that cannot be delivered due to river capacity constraints), and the proportion of shortfall categorized into low and high flow components. Here a high flow component is the portion of any flow component above 5,000 ML/d. This threshold translates to between the 10th and 20th flow exceedance percentiles based on historic records. The low flow component is any flow below 5,000 ML/d. Constraint issues are clear in baseline conditions from the large volume of 165 GL/year of environmental water that cannot be delivered (see (0, 0) in Figure 6A). This is roughly equal to the total environmental water shortfall (Figure 5B) and more than a quarter of the total environmental water entitlement. Note that this water is not “lost,” as unused water can be carried over and used later in the season. Rather it represents the annual sum of water that could have been delivered for priority flow components if not for flow constraints. There are still significant constraints of 100 GL/year for climate projections with a decrease in long term precipitation of 10%, but constraints decrease as the climate gets drier as less water is allocated for environmental water or irrigation. High flows make up approximately three quarters of the shortfall under the existing climate. This proportion stays remarkably constant through changes in climate, although as conditions get hotter and drier, low flows make up a progressively higher portion of the total shortfall.
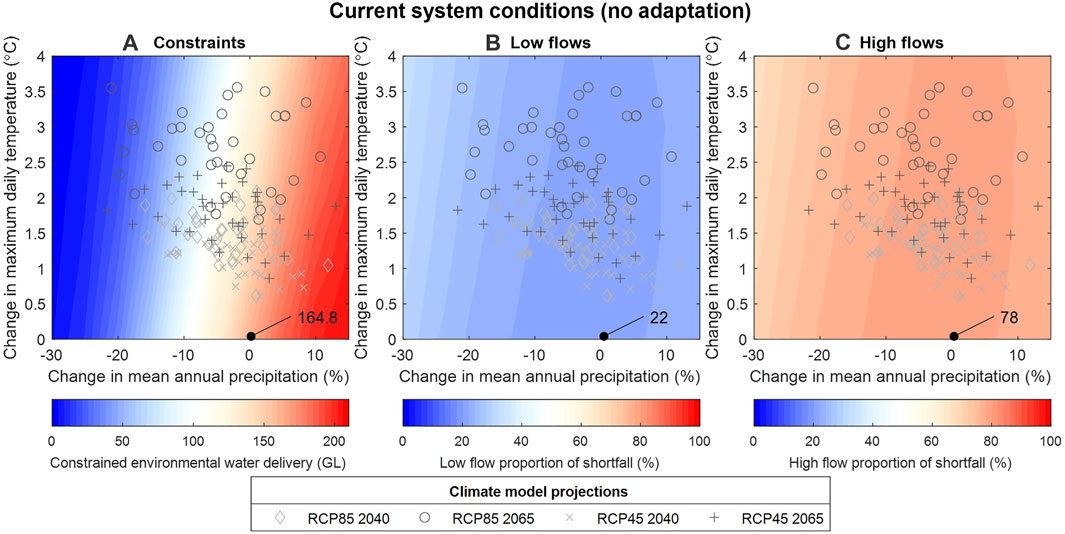
FIGURE 6. Stress testing results for current system conditions showing: (A) constraints (allocated environmental water that cannot be delivered due to river capacity constraints); (B) and the low flow proportion of the environmental water shortfall; and (C) the high flow proportion of the environmental water shortfall.
3.2 Adaptation Responses
The adaptation response using increased seasonal baseflows (PF) had almost no impact in reducing environmental water shortfall or improving ecological modelling outputs, and it reduced allocation reliability (see Supplementary Material). This was also true when combined with any other adaptation option. It is likely that the volume of water and scope of this option is insufficient to affect the flow regime and deliver tangible ecological benefits. As such, this option is no longer considered in the following results, but full results can be found in Supplementary Material.
The remaining options for adaptation include the increased environmental entitlement, relaxed constraints, and their combination. These three results are presented in Figure 7. Figure 7, which shows the difference rather than the absolute values (where “difference” refers to change relative to the “no adaptation” case in Figure 5). For example, in Figure 7 below, response surfaces show the increase in reliability, reduction in environmental water shortfall and change in number of models within the tolerability range. Results for each option not relative to the baseline (i.e., the same arrangement as Figure 5) can be found in Supplementary Material.
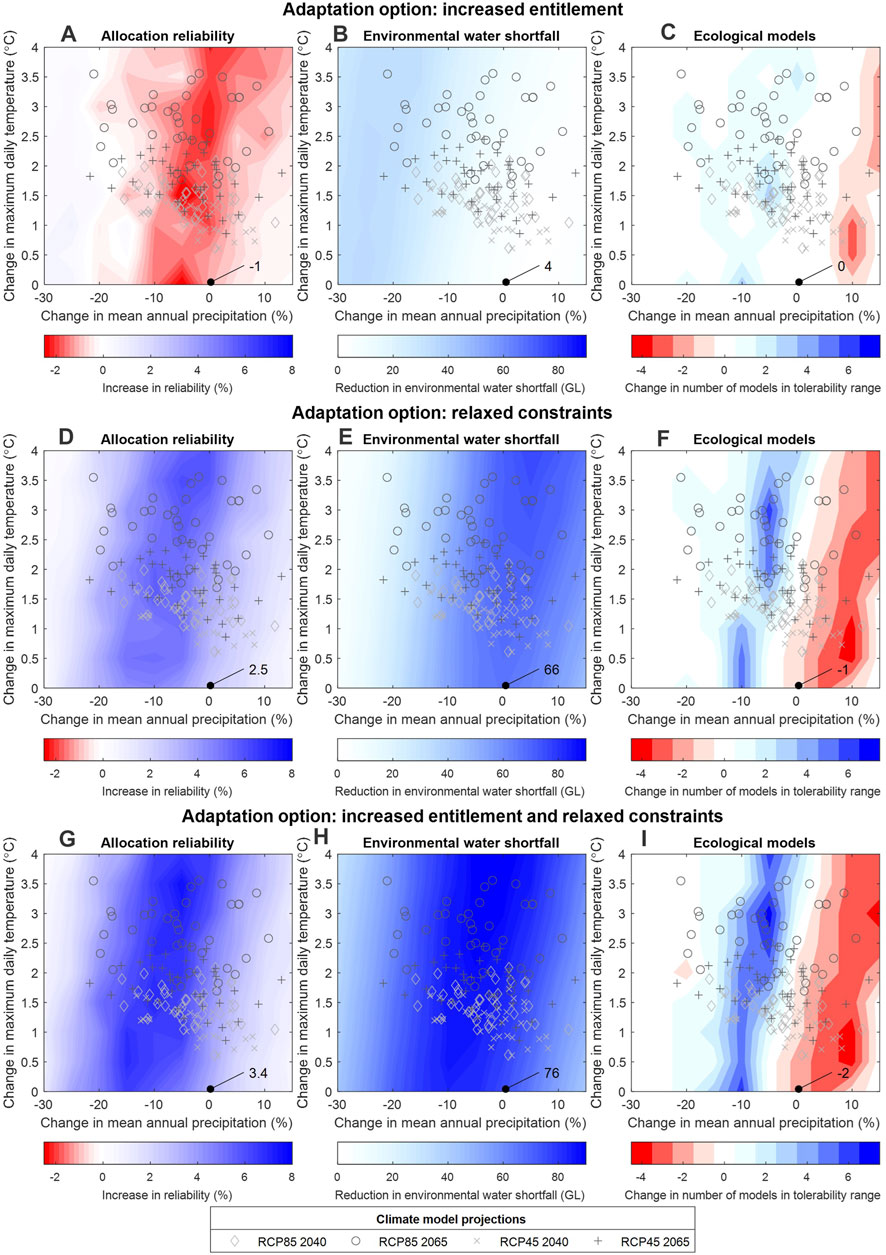
FIGURE 7. Stress testing results for selected adaptation options relative to baseline performance in Figure 4. (A–C) for increased entitlement; (d–f) for relaxed constraints; and (g–i) for both the above options. Note the x and y-axes are identical for all plots. GCM outputs are plotted over failure surfaces for two time periods and two emissions scenarios. Also highlighted is the system performance in the absence of climate change (0, 0).
The increased entitlement (IE) option reduces allocation reliability compared to the baseline for much of the tested climate space, with slightly higher reductions around moderate reductions in mean annual precipitation (Figure 7A). The reasons for reliability reductions are unclear, but seasonal water use and carryover of allocation from 1 year to the next is different between irrigators and environmental water managers, so it is conceivable that this has some influence on reliability. It is worth remembering however, that in this example the increased environmental entitlement comes from other water users in the system. From Figure 7B, there is relatively little benefit to addressing environmental water shortfalls in the current climate regime, with reductions in shortfall of only 4 GL/year. Reductions in shortfall become larger for a hotter and drier climate, presumably linked to less frequent existing constraint issues in dry climates, and thus maximizing the usefulness of the increased entitlement. Ecological model outcomes show marginal improvement for conditions around a moderate degree of drying climate (between 5 and 10% reductions in mean annual precipitation), but worse outcomes for wetter climates (Figure 7C). In this instance, higher environmental entitlements increase overall river flows in downstream reaches, which would have otherwise been diverted for irrigation. This has negative consequences for the models known to be sensitive to seasonally high flows (bank stability, littoral vegetation, platypus, and instream production).
The relaxed constraints (RC) option increases allocation reliability, from ∼2.5% in the current climate up to 5% for moderately drier and hotter conditions (Figure 7D). This also had the benefit of not reducing other water user entitlements compared to option IE. The driving factor here appears to be that RC tends to improve airspace since water can be used more rapidly (e.g., releasing relatively larger floods for environmental purposes), freeing up space in reservoirs and increasing the ability of the reservoir to intercept subsequent flows. For the current climate, overall spill volumes are 17% lower in RC compared to the baseline, but there is no difference in mean reservoir storage level in model simulations. Shortfall reductions were more apparent for scenarios with relatively small changes in mean annual precipitation (Figure 7E). Unlike IE, RC becomes less effective at reducing shortfalls for progressively drier climates, again, presumably because river capacity issues become less common with reducing streamflows. Ecological model responses are stronger than the IE case, with improvements in moderately drier climate but poorer outcomes in wetter climates (Figure 7F). The RC option ultimately leads to higher river flows in wetter climates compared to the baseline as it allows the delivery of higher flow components and larger inter valley transfers.
The option combining both increased entitlements and relaxed constraints (IERC) has some significant overall benefits for reliability and ecological outcomes. Interestingly, improvements in allocation reliability and environmental shortfalls are greater than the sum of the benefits of each option individually (Figure 7G). The reductions in reliability from IE were no longer apparent when combined RC. Reliability increased from 3.4% in the current climate up to 8% for moderately drier and hotter conditions (although this option still ultimately reduces entitlement for non-environmental water users). This greater than additive response was also true for reductions in environmental water shortfalls, which are at their highest for moderately dry and hot climates (Figure 7H). This blends the responses from IE, which offers higher benefits in dry climates, and RC, which reduces shortfall more for the current and wetter climates. Ultimately, this provides significant improvements in a large portion of the climate space projected by GCMs for all emissions scenarios and future periods investigated. Ecological model outcomes are more favorable still for drier and hotter conditions compared to IE and RC (Figure 7I). However, this comes at the expense of even poorer conditions in wetter climates for those models that are sensitive to higher river flows, including reductions in ecological outcomes in the current climate.
A simple way of assessing the sensitivity of individual ecological models to climate change is the sequence in which they leave the tolerability range. Table 4 shows this sequence as conditions get progressively drier. Hence, Table 4 generally shows descending sensitivity to a drier climate, with more sensitive models at the top and less sensitive at the bottom. Note that as some models leave their tolerability range at the same magnitude of precipitation change, their sequence is recorded as the same. Since ecological models are more sensitive to precipitation, the temperature increase is held steady at 2°C for the purposes of Table 4. Adaptation options primarily improve outcomes for fish models compared to the baseline. Adaptation increases the range of change in mean annual precipitation these models can tolerate by up to 10%. Models that have poorer outcomes under adaptation scenarios in wetter climates almost all have interdependencies on bank stability outcomes. Instream production, littoral vegetation, and platypus models also all have seasonal requirements for flows remaining below a certain range. It is conceivable that an adjustment in system management, such as changes in the way IVTs are delivered in summer, can reduce the sensitivity of these models to wetter climates. However, bank stability outcomes are sensitive to high flows at any time of the year. Since bank stability is still linked to instream production, littoral vegetation, and platypus models, there is a degree of sensitivity that likely cannot be reduced through seasonal flow management.
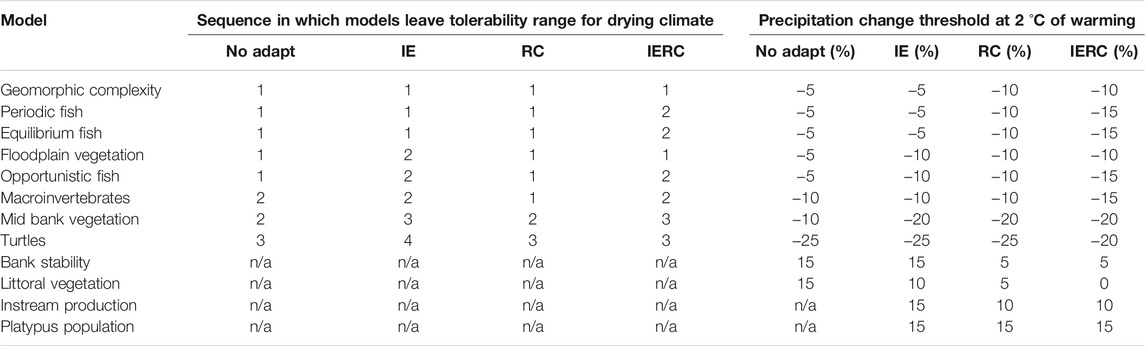
TABLE 4. Sensitivity of ecological models to changes in long-term annual precipitation for current conditions and each adaptation option. The sequence in which models leave their individual tolerability range is given, as well as the precipitation change threshold when they leave their range. Note that some models leave their tolerability range at the same precipitation change hence their sequence is recorded together.
4 Discussion
4.1 The Role of Stress Testing for Environmental Flows Management
There is a widely accepted challenge in how to include climate change considerations in environmental flow recommendations and management (Acreman et al., 2014; Horne A. C. et al., 2017; Poff, 2018). This case study demonstrates that a stress testing approach, commonly applied to other water resource challenges, can inform the appropriate combination of water recovery with other policy settings and river management practices to achieve environmental objectives under a range of plausible futures. Proposed environmental flow regimes can be evaluated for their sensitivity to different climatic regimes before substantial investments in water recovery or environmental legislation are made. Or like in this case study, existing environmental flow arrangements can be augmented to deliver more robust outcomes under climate change. Our results showed important thresholds for precipitation change that lead to non-linear reductions in system reliability and ecological change. These manifested around a decrease in precipitation of 10% (slightly modulated by temperature increases). Using a more traditional climate scenario-based assessment limits the range of uncertainty in climate inputs that can be explored and does not offer insights into potentially important thresholds of climate change or ecological response.
Stress testing approaches can also be easily adapted from assessing long-term climate change risk to shorter-term risk such as droughts (Hall and Leng, 2019), simply by changing the time period over which responses are evaluated. In such cases there might be slightly different stressors considered, and the requirements of water resource and ecological modeling may change, but the stress testing principles of exploring vulnerability to uncertainty in system inputs are equally applicable.
Adaptation for freshwater ecosystems under climate change can also be supported by advances in environmental flows optimisation (Horne et al., 2016) and machine learning approaches for hydrological and ecological models (Sit et al., 2020). Optimisation can assist in short-term active management of environmental water (Horne et al., 2018) or longer-term setting of flow recommendations (Horne A. et al., 2017). Machine learning approaches can offer significant predictive accuracy for ecological responses to change (McKay et al., 2019), although risk a loss of transparency and easily interpretable outcomes for stakeholders (Hain et al., 2018; Kennen et al., 2018). Integrating complex models into a stress-testing methodology can be computationally expensive, but typically once training has been performed machine learning models are quick to run. Approaches based on historic data must be mindful when extrapolating to potentially novel climate or ecological conditions, and in these cases mechanistic models may help in providing sounder projections (Tonkin et al., 2019). This is especially important for stress testing approaches which typically aim to expose vulnerability under highly uncertain future changes.
4.2 Significance of Results
There are distinct differences in the inferences made from comparing adaptation options using the broader shortfall metric and the outputs from ecological models. We argue that the ecological model outputs are more useful for assessing the effects of climate change on environmental flow outcomes. The shortfalls metric implies that, notwithstanding particular characteristics of individual options, all options offer benefits through reductions in shortfall in various regions of the tested climate change space. The ecological models, however, demonstrate that all adaptation options were suited for a hotter and drier future only when combined with other existing river management practices. For wetter futures (of which there is still a possibility implied by the spread of GCM projections) the adaptation options lead to poorer outcomes overall. Whilst perhaps counter intuitive, this is due to the operation of the river to meet consumptive water needs along with environmental flows; the delivery of these consumptive flows has more impact in wetter years.
This result highlights some of the challenges for devising environmental flow recommendations. In water limited locations such as Australia where the largest impact on regulated rivers has historically been irrigation diversions, most recommendations are concerned with minimum flows to meet species needs, and most environmental flow planning objectives have been framed around water recovery. In this context, the concept of a “shortfall” metric makes sense. Nonetheless, flow recommendations should always include the full range of ecologically relevant flow components including maximum flows. In other words, particularly when considering climate change, they should present the envelope of acceptable flow conditions and sequences. This concept is perhaps more familiar in the USA or Europe, where there is a more common precedent of implementing environmental flows to mitigate river regulation from hydropower infrastructure (Poff et al., 2007). The emergence in recent years of summer inter valley transfers as a flow management issue in the Goulburn River illustrates that this issue can also arise in drier climates. As the case study demonstrates, not including the full range of ecologically relevant flows in decision frameworks increases the risk of making maladaptive decisions around effective intervention under climate change. Using mechanistic ecological models to project ecological condition offers a way to make more informed decisions on the robustness of adaptation and can more clearly highlight potentially important thresholds in ecological response (Horne et al., 2019; Tonkin et al., 2019). The methods we use here are equally applicable in other basins around the world. A benefit of the stress testing approach is the flexibility to tailor the assessment to almost any degree or type of system change, whether climate-related or a more internal basin issue such as land clearing. However, in all cases, output metrics and definitions of baseline performance should carefully consider the more local water management objectives and issues within the basin.
High flow components are the first to be affected in drying climates. Increases of daily rainfall intensities can offset drier soil moisture regimes and improve natural catchment inflows, but this typically only affects larger floods that are too rare to provide year-to-year key ecological needs (Wasko and Nathan, 2019; Wasko et al., 2020). This work demonstrates the importance of maintaining high flow regimes for ecological outcomes using regulated river releases to “piggyback” declining natural catchment inflows under drying climates. As a general environmental watering strategy, more targeted delivery of key flow components is an effective way to maximize the ecological benefit of environmental flows (Horne et al., 2018a). Options that increase flexibility for environmental water managers can be more effective over a wider range of climatic conditions.
The reliability metrics conveyed that dry climate futures have significant implications for water availability in the river. Testing of adaptation options to support environmental flows suggest that while a degree of climate change can be accommodated through changed operational and policy settings, larger climate changes cannot be adapted to using any of the options tested here. Reductions in long-term average precipitation of >20% stress the maximum number of ecological endpoints regardless of interventions. Although we do not go into detail on implementation viability of any of the options presented here, they do represent significant investments in infrastructure or policy change. Under very dry climate futures preserving current ecological values may become an increasingly untenable goal in this river system, and perhaps many others. This could be considered through an adaptation pathway planning process, which may also include options for transformation and considering a shift in objectives for the environmental flows program. With regard to pathways planning, our results here imply that relaxing constraints may be a better option for the immediate future rather than increasing environmental entitlements. The stress testing approach can be useful for sequencing implementation of adaptation to highlight low-regret pathways.
4.3 Confounding Factors
There are two major sources of uncertainty that limit analyses of adaptation effectiveness for freshwater ecosystems. The first of these is deep uncertainty in how the climate may respond to greenhouse gas emissions. Stress testing methods can explore uncertainties by testing various combinations of changes that may not be considered using downscaled climate projections, but it is infeasible to test every conceivable way in which the climate may change. Changes in the seasonal distribution of precipitation is projected by GCMs in the Goulburn River basin (Timbal and Jones, 2008). Potential changes in low-frequency variability of precipitation that affects the frequency or duration of multi-year dry periods will also influence reservoir reliability and high and low tributary inflows. We originally focused on changes to long-term average precipitation and temperature because they were shown to be more influential than seasonality or low-frequency variability for ecological outcomes in the regulated reaches of the Goulburn River. Further work could assess adaptation to additional changes beyond long-term average precipitation and temperature as either knowledge of future climate changes or ecological stressors increases. There are still challenges with communicating higher dimensional (i.e., more than two) changes in a larger number of climatic variables in stress tests in a manner that assists decision making for flow managers, although this is ongoing area of research (Culley et al., 2021).
More uncertain still are changes in landscape, hydrological and ecological properties as a result of climate change. These have the potential to be highly influential to environmental water outcomes. This is a diverse set of changes but broadly includes changes in land-use patterns (Kuemmerlen et al., 2015), vegetation cover and vegetation water use behavior (Frank et al., 2015; Tietjen et al., 2017), non-stationary rainfall-runoff responses (Peterson et al., 2021), and ecological dynamics (Anderson et al., 2006; Wolkovich et al., 2014). Some of these, such as the impacts of land-use change, can be included in current hydrological models. However, there is limited opportunity to include biophysical dynamics or non-stationary runoff responses in hydrological models (Blöschl et al., 2019). Non-flow drivers of ecological outcomes are important to include when establishing flow-ecology relationships in ecological models (Poff and Zimmerman, 2010; Poff, 2018). Some of these drivers are included in the ecological models used in this study, such as bushfires, sediment and nutrient regimes and predator-prey relationships. But there are more fundamental problems with making projections for these influences compared to flows, which can be relatively more easily simulated with hydrological and water resource models. For example, in the turtles model one of the most influential factors in turtle population is coincident fox population (which predate offspring). But it is extremely challenging to project fox populations responses to climate change. Moreover, non-flow drivers of ecological responses may themselves be subject to intervention in order to improve environmental flow outcomes (Nicol et al., 2021). Such “complementary measures” are being actively explored in Australia and other water-limited jurisdictions and will almost certainly become part of the toolset for improving ecological outcomes for environmental flows under a drying climate.
5 Conclusion
This study shows that stress testing methods are an effective way to assess the sensitivity of environmental water outcomes and the robustness of adaptation options to uncertain changes in climate. The stress testing reveals shortcomings in current system management, vulnerability of irrigation and environmental water to a drying climate, and general limits to the tolerability of ecological endpoints to changes in long-term climate. It also shows the regions of the future climate space (i.e., hot or cool, dry or wet) in which different adaption options are most effective. Combining the increased environmental entitlement and relaxed constraints adaptation options together provide a very robust reduction in shortfalls across almost the entire tested climate space. However, the ecological models suggest that while these options are effective at increasing ecological resilience under drying climates, they risk deteriorating outcomes for some models under wetter climates. The apparent effectiveness of adaptation options, and potentially the recommendations from an impact or adaptation assessment depend on the type of response metric used. Therefore, the use of multiple metrics for adaptation effectiveness, particularly those incorporating mechanistic relationships and ecological dynamics, helps reduce maladaptation risk under climate change.
One of the starker results from this study is none the options tested here could adapt ecological outcomes to stronger impacts of a drying climate. Multiple GCMs project reductions in mean annual precipitation between 10 and 20% by 2065 even under a moderate emissions scenario of RCP4.5. This degree of climate change causes severely low water allocations in the system, large environmental water shortfalls and leads to worse outcomes for most ecological endpoints in the river system regardless of adaptation attempts. This suggests that river managers should be prepared for potentially inevitable transitions in regulated river ecosystems unless aggressive climate change mitigation efforts are pursued.
Data Availability Statement
Data used in this analysis is publicly available in the following repositories: historic precipitation and temperature from Australian Water Availability Project (Jones et al., 2009) http://www.bom.gov.au/jsp/awap/; potential evapotranspiration from Scientific Information for Land Owners (Jeffrey et al., 2001) https://www.longpaddock.qld.gov.au/silo/; streamflow and reservoir storage observations from the Victorian Government Water Measurement Information System https://data.water.vic.gov.au/. Matlab code to produce stochastic climate data and runoff projections is available from https://doi.org/10.5281/zenodo.4702487.
Author Contributions
AJ undertook the modeling and simulation, conceptualized adaptation responses and drafted the manuscript. AH and RN conceptualized the wider study, provided inputs to modeling and simulation and reviewed the manuscript. KF undertook rainfall-runoff modeling, assisted in concepts and reviewed the manuscript. JW provided inputs to ecological modeling and reviewed the manuscript. MS helped conceptualize the wider study and reviewed the manuscript.
Funding
This study was funded by the Australian Research Council (ARC Linkage Project LP170100598), Australian Commonwealth Government under a Research Training Program Scholarship, and several partner agencies including the Department of Environment, Land, Water and Planning, the Victorian Environmental Water Holder and the Bureau of Meteorology. AH was funded by Australian Research Council DECRA DE180100550.
Conflict of Interest
The authors declare that the research was conducted in the absence of any commercial or financial relationships that could be construed as a potential conflict of interest.
Publisher’s Note
All claims expressed in this article are solely those of the authors and do not necessarily represent those of their affiliated organizations, or those of the publisher, the editors and the reviewers. Any product that may be evaluated in this article, or claim that may be made by its manufacturer, is not guaranteed or endorsed by the publisher.
Acknowledgments
We are grateful to Goulburn Murray Water for providing data necessary to develop the water resource model, and to the Goulburn-Broken Catchment Management Authority for great effort in the development of ecological models and the update of the Kaiela environmental flow recommendations which supported this work.
Supplementary Material
The Supplementary Material for this article can be found online at: https://www.frontiersin.org/articles/10.3389/fenvs.2021.789206/full#supplementary-material
References
Acreman, M., Arthington, A. H., Colloff, M. J., Couch, C., Crossman, N. D., Dyer, F., et al. (2014). Environmental Flows for Natural, Hybrid, and Novel Riverine Ecosystems in a Changing World. Front. Ecol. Environ. 12, 466–473. doi:10.1890/130134
Anderson, K. E., Paul, A. J., McCauley, E., Jackson, L. J., Post, J. R., and Nisbet, R. M. (2006). Instream Flow Needs in Streams and Rivers: The Importance of Understanding Ecological Dynamics. Front. Ecol. Environ. 4, 309–318. doi:10.1890/1540-9295(2006)4[309:ifnisa]2.0.co;2
Arthington, A. H., Bhaduri, A., Bunn, S. E., Jackson, S. E., Tharme, R. E., Tickner, D., et al. (2018). The Brisbane Declaration and Global Action Agenda on Environmental Flows (2018). Front. Environ. Sci. 6, 45. doi:10.3389/fenvs.2018.00045
Blöschl, G., Bierkens, M. F. P., Chambel, A., Cudennec, C., Destouni, G., Fiori, A., et al. (2019). Twenty-three Unsolved Problems in Hydrology (UPH)–a Community Perspective. Hydrol. Sci. J. 64, 1141–1158. doi:10.1080/02626667.2019.1620507
Bond, N. R., Grigg, N., Roberts, J., McGinness, H., Nielsen, D., O'Brien, M., et al. (2018). Assessment of Environmental Flow Scenarios Using State-And-Transition Models. Freshw. Biol. 63, 804–816. doi:10.1111/fwb.13060
Brown, C., and King, J. (2012). Modifying Dam Operating Rules to Deliver Environmental Flows: Experiences from Southern Africa. Int. J. River Basin Manage. 10, 13–28. doi:10.1080/15715124.2011.639304
Brown, C., and Wilby, R. (2012). An Alternate Approach to Assessing Climate Risks. Eos Trans. Am. Geophys. Union 93, 401–402. doi:10.1029/2012EO410001
Brown, C., Ghile, Y., Laverty, M., and Li, K. (2012). Decision Scaling: Linking Bottom‐up Vulnerability Analysis with Climate Projections in the Water Sector. Water Resour. Res. 48, W09537. doi:10.1029/2011WR011212
Chen, A., and Wu, M. (2019). Managing for Sustainability: The Development of Environmental Flows Implementation in China. Water 11, 433. doi:10.3390/w11030433
Culley, S., Maier, H. R., Westra, S., and Bennett, B. (2021). Identifying Critical Climate Conditions for Use in Scenario-Neutral Climate Impact Assessments. Environ. Model. Softw. 136, 104948. doi:10.1016/J.ENVSOFT.2020.104948
El‐Jabi, N., and Caissie, D. (2019). Characterization of Natural and Environmental Flows in New Brunswick, Canada. River Res. Applic. 35, 14–24. doi:10.1002/rra.3387
Fowler, K. J. A., Peel, M. C., Western, A. W., Zhang, L., and Peterson, T. J. (2016). Simulating Runoff under Changing Climatic Conditions: Revisiting an Apparent Deficiency of Conceptual Rainfall-Runoff Models. Water Resour. Res. 52, 1820–1846. doi:10.1002/2015WR018068
Frank, D. C., Poulter, B., Saurer, M., Esper, J., Huntingford, C., Helle, G., et al. (2015). Water-use Efficiency and Transpiration across European Forests during the Anthropocene. Nat. Clim Change 5, 579–583. doi:10.1038/nclimate2614
Hain, E. F., Kennen, J. G., Caldwell, P. V., Nelson, S. A. C., Sun, G., and McNulty, S. G. (2018). Using Regional Scale Flow-Ecology Modeling to Identify Catchments where Fish Assemblages Are Most Vulnerable to Changes in Water Availability. Freshw. Biol. 63, 928–945. doi:10.1111/fwb.13048
Hall, J. W., and Leng, G. (2019). Can We Calculate Drought Risk… and Do We Need to? WIREs Water 6, e1349. doi:10.1002/wat2.1349
Hart, B. T. (2016). The Australian Murray-Darling Basin Plan: Factors Leading to its Successful Development. Ecohydrol. Hydrobiol. 16, 229–241. doi:10.1016/j.ecohyd.2016.09.002
Henley, B. J., Peel, M. C., Nathan, R., King, A. D., Ukkola, A. M., Karoly, D. J., et al. (2019). Amplification of Risks to Water Supply at 1.5 °C and 2 °C in Drying Climates: a Case Study for Melbourne, Australia. Environ. Res. Lett. 14, 084028. doi:10.1088/1748-9326/ab26ef
Horne, A., Szemis, J. M., Kaur, S., Webb, J. A., Stewardson, M. J., Costa, A., et al. (2016). Optimization Tools for Environmental Water Decisions: A Review of Strengths, Weaknesses, and Opportunities to Improve Adoption. Environ. Model. Softw. 84, 326–338. doi:10.1016/j.envsoft.2016.06.028
Horne, A. C., Webb, J. A., O'Donnell, E., Arthington, A. H., McClain, M., Bond, N., et al. (2017a). Research Priorities to Improve Future Environmental Water Outcomes. Front. Environ. Sci. 5, 89. doi:10.3389/fenvs.2017.00089
Horne, A., Kaur, S., Szemis, J., Costa, A., Webb, J. A., Nathan, R., et al. (2017b). Using Optimization to Develop a “Designer” Environmental Flow Regime. Environ. Model. Softw. 88, 188–199. doi:10.1016/j.envsoft.2016.11.020
Horne, A. C., Kaur, S., Szemis, J. M., Costa, A. M., Nathan, R., Angus Webb, J., et al. (2018a). Active Management of Environmental Water to Improve Ecological Outcomes. J. Water Resour. Plann. Manage. 144, 04018079. doi:10.1061/(ASCE)WR.1943-5452.0000991
Horne, A. C., Szemis, J. M., Webb, J. A., Kaur, S., Stewardson, M. J., Bond, N., et al. (2018b). Informing Environmental Water Management Decisions: Using Conditional Probability Networks to Address the Information Needs of Planning and Implementation Cycles. Environ. Manage. 61, 347–357. doi:10.1007/s00267-017-0874-8
Horne, A. C., Nathan, R., Poff, N. L., Bond, N. R., Webb, J. A., Wang, J., et al. (2019). Modeling Flow-Ecology Responses in the Anthropocene: Challenges for Sustainable Riverine Management. Bioscience 69, 789–799. doi:10.1093/biosci/biz087
Horne, A., Webb, J. A., Rumpff, L., Mussehl, M., Fowler, K., and John, A. (2020). Kaiela (Lower Goulburn River) Environmental Flows Study. Melbourne.
IPCC (2013). “Contribution of Working Group I to the Fifth Assessment Report of the Intergovernmental Panel on Climate Change,” in Climate Change 2013: The Physical Science Basis. Editors T. F. Stocker, D. Qin, G. K. Plattner, M. M. B. Tignor, S. K. Allen, J. Boschunget al. (Cambridge, United Kingdom and New York, NY, USA: Cambridge University Press). doi:10.1017/CBO9781107415324.004
IPCC (2014). “Contribution of Working Group II to the Fifth Assessment Report of the Intergovernmental Panel on Climate Change,” in Climate Change 2014: Impacts, Adaptation and Vulnerability: Part A: Global and Sectoral Aspects. Editors C. B. Field, V. R. Barros, D. J. Dokken, K. J. Mach, M. D. Mastrandrea, T. E. Biliret al. (Cambridge, United Kingdom and New York, NY, USA: Cambridge University Press). doi:10.1017/CBO9781107415379
Jeffrey, S. J., Carter, J. O., Moodie, K. B., and Beswick, A. R. (2001). Using Spatial Interpolation to Construct a Comprehensive Archive of Australian Climate Data. Environ. Model. Softw. 16, 309–330. doi:10.1016/S1364-8152(01)00008-1
John, A., Nathan, R., Horne, A., Stewardson, M., and Webb, J. A. (2020). How to Incorporate Climate Change into Modelling Environmental Water Outcomes: A Review. J. Water Clim. Chang. 11, 327–340. doi:10.2166/wcc.2020.263
John, A., Horne, A., Nathan, R., Stewardson, M., Webb, J. A., Wang, J., et al. (2021a). Climate Change and Freshwater Ecology: Hydrological and Ecological Methods of Comparable Complexity Are Needed to Predict Risk. Wires Clim. Change 12, 1–16. doi:10.1002/wcc.692
John, A., Fowler, K., Nathan, R., Horne, A., and Stewardson, M. (2021b). Disaggregated Monthly Hydrological Models Can Outperform Daily Models in Providing Daily Flow Statistics and Extrapolate Well to a Drying Climate. J. Hydrol. 598, 126471. doi:10.1016/j.jhydrol.2021.126471
Johnson, F., and Sharma, A. (2011). Accounting for Interannual Variability: A Comparison of Options for Water Resources Climate Change Impact Assessments. Water Resour. Res. 47. doi:10.1029/2010WR009272
Jones, D., Wang, W., and Fawcett, R. (2009). High-quality Spatial Climate Data-Sets for Australia. AMOJ 58, 233–248. doi:10.22499/2.5804.003
Kaur, S., Horne, A. C., Nathan, R., Szemis, J. M., Gibson, L., Costa, A. M., et al. (2019). Examining Trade-Offs in Piggybacking Flow Events while Making Environmental Release Decisions in a River System. J. Water Resour. Plann. Manage. 145, 04019019. doi:10.1061/(ASCE)WR.1943-5452.0001048
Kennen, J. G., Stein, E. D., and Webb, J. A. (2018). Evaluating and Managing Environmental Water Regimes in a Water-Scarce and Uncertain Future. Freshw. Biol. 63, 733–737. doi:10.1111/fwb.13104
Kuemmerlen, M., Schmalz, B., Cai, Q., Haase, P., Fohrer, N., and Jähnig, S. C. (2015). An Attack on Two Fronts: Predicting How Changes in Land Use and Climate Affect the Distribution of Stream Macroinvertebrates. Freshw. Biol. 60, 1443–1458. doi:10.1111/fwb.12580
McKay, S. K., Theiling, C. H., and Dougherty, M. P. (2019). Comparing Outcomes from Competing Models Assessing Environmental Flows in the Minnesota River Basin. Ecol. Eng. 142, 100014. doi:10.1016/j.ecoena.2019.100014
Mezger, G., De Stefano, L., and González del Tánago, M. (2019). Assessing the Establishment and Implementation of Environmental Flows in Spain. Environ. Manage. 64, 721–735. doi:10.1007/s00267-019-01222-2
Murray–Darling Basin Authority (2010). Guide to the Proposed Basin Plan: Technical Background. Canberra: Murray-Darling Basin Authority.
Nathan, R. J., McMahon, T. A., Peel, M. C., and Horne, A. (2019). Assessing the Degree of Hydrologic Stress Due to Climate Change. Climatic Change 156, 87–104. doi:10.1007/s10584-019-02497-4
Nicol, S., Webb, J. A., Lester, R. E., Cooling, M., Brown, P., Cresswell, I., et al. (2021). Evaluating the Ecological Benefits of Management Actions to Complement Environmental Flows in River Systems. Environ. Manage. 67, 277–290. doi:10.1007/s00267-020-01395-1
Opperman, J. J., Kendy, E., and Barrios, E. (2019). Securing Environmental Flows through System Reoperation and Management: Lessons from Case Studies of Implementation. Front. Environ. Sci. 7, 104. doi:10.3389/fenvs.2019.00104
Peterson, T. J., Saft, M., Peel, M. C., and John, A. (2021). Watersheds May Not Recover from Drought. Science 372, 745–749. doi:10.1126/science.abd5085
Poff, N. L., and Matthews, J. H. (2013). Environmental Flows in the Anthropocence: Past Progress and Future Prospects. Curr. Opin. Environ. Sustain. 5, 667–675. doi:10.1016/j.cosust.2013.11.006
Poff, N. L., and Zimmerman, J. K. H. (2010). Ecological Responses to Altered Flow Regimes: A Literature Review to Inform the Science and Management of Environmental Flows. Freshw. Biol. 55, 194–205. doi:10.1111/j.1365-2427.2009.02272.x
Poff, N. L., Olden, J. D., Merritt, D. M., and Pepin, D. M. (2007). Homogenization of Regional River Dynamics by Dams and Global Biodiversity Implications. Proc. Natl. Acad. Sci. 104, 5732–5737. doi:10.1073/pnas.0609812104
Poff, N. L., Richter, B. D., Arthington, A. H., Bunn, S. E., Naiman, R. J., Kendy, E., et al. (2010). The Ecological Limits of Hydrologic Alteration (ELOHA): A New Framework for Developing Regional Environmental Flow Standards. Freshw. Biol. 55, 147–170. doi:10.1111/j.1365-2427.2009.02204.x
Poff, N. L., Brown, C. M., Grantham, T. E., Matthews, J. H., Palmer, M. A., Spence, C. M., et al. (2016). Sustainable Water Management under Future Uncertainty with Eco-Engineering Decision Scaling. Nat. Clim Change 6, 25–34. doi:10.1038/nclimate2765
Poff, N. L. (2018). Beyond the Natural Flow Regime? Broadening the Hydro-Ecological Foundation to Meet Environmental Flows Challenges in a Non-stationary World. Freshw. Biol. 63, 1011–1021. doi:10.1111/fwb.13038
Prosser, I. P., Chiew, F. H. S., and Stafford Smith, M. (2021). Adapting Water Management to Climate Change in the Murray-Darling Basin, Australia. Water 13, 2504. doi:10.3390/W13182504
Rayner, T. S., Jenkins, K. M., and Kingsford, R. T. (2009). Small Environmental Flows, Drought and the Role of Refugia for Freshwater Fish in the Macquarie Marshes, Arid Australia. Ecohydrol. 2, 440–453. doi:10.1002/eco.73
Saft, M., Western, A. W., Zhang, L., Peel, M. C., and Potter, N. J. (2015). The Influence of Multiyear Drought on the Annual Rainfall‐runoff Relationship: An A Ustralian Perspective. Water Resour. Res. 51, 2444–2463. doi:10.1002/2014WR015348
Shenton, W., Bond, N. R., Yen, J. D. L., and Mac Nally, R. (2012). Putting the “Ecology” into Environmental Flows: Ecological Dynamics and Demographic Modelling. Environ. Manage. 50, 1–10. doi:10.1007/s00267-012-9864-z
Sit, M., Demiray, B. Z., Xiang, Z., Ewing, G. J., Sermet, Y., and Demir, I. (2020). A Comprehensive Review of Deep Learning Applications in Hydrology and Water Resources. Water Sci. Technol. 82, 2635–2670. doi:10.2166/wst.2020.369
Steinschneider, S., McCrary, R., Wi, S., Mulligan, K., Mearns, L. O., and Brown, C. (2015). Expanded Decision-Scaling Framework to Select Robust Long-Term Water-System Plans under Hydroclimatic Uncertainties. J. Water Resour. Plann. Manage. 141, 04015023. doi:10.1061/(ASCE)WR.1943-5452.0000536
Taylor, K. E., Stouffer, R. J., and Meehl, G. A. (2012). An Overview of CMIP5 and the experiment Design. Bull. Am. Meteorol. Soc. 93, 485–498. doi:10.1175/BAMS-D-11-00094.1
Tietjen, B., Schlaepfer, D. R., Bradford, J. B., Lauenroth, W. K., Hall, S. A., Duniway, M. C., et al. (2017). Climate Change‐induced Vegetation Shifts lead to More Ecological Droughts Despite Projected Rainfall Increases in many Global Temperate Drylands. Glob. Change Biol. 23, 2743–2754. doi:10.1111/gcb.13598
Timbal, B., and Drosdowsky, W. (2013). The Relationship between the Decline of Southeastern Australian Rainfall and the Strengthening of the Subtropical ridge. Int. J. Climatol. 33, 1021–1034. doi:10.1002/joc.3492
Timbal, B., and Jones, D. A. (2008). Future Projections of winter Rainfall in Southeast Australia Using a Statistical Downscaling Technique. Climatic Change 86, 165–187. doi:10.1007/s10584-007-9279-7
Tonkin, J. D., Poff, N. L., Bond, N. R., Horne, A., Merritt, D. M., Reynolds, L. V., et al. (2019). Prepare River Ecosystems for an Uncertain Future. Nature 570, 301–303. doi:10.1038/d41586-019-01877-1
Turner, S. W. D., Marlow, D., Ekström, M., Rhodes, B. G., Kularathna, U., Jeffrey, P. J., et al. (2014). Linking Climate Projections to Performance: A Yield-Based Decision Scaling Assessment of a Large Urban Water Resources System. Water Resour. Res. 50, 3553–3567. doi:10.1002/2013WR015156
Wang, Q. J., Pagano, T. C., Zhou, S. L., Hapuarachchi, H. A. P., Zhang, L., and Robertson, D. E. (2011). Monthly versus Daily Water Balance Models in Simulating Monthly Runoff. J. Hydrol. 404, 166–175. doi:10.1016/j.jhydrol.2011.04.027
Wang, J., Horne, A., Nathan, R., Peel, M., and Neave, I. (2018). Vulnerability of Ecological Condition to the Sequencing of Wet and Dry Spells Prior to and during the Murray-Darling basin Millennium Drought. J. Water Resour. Plann. Manage. 144, 04018049. doi:10.1061/(ASCE)WR.1943-5452.0000963
Wasko, C., and Nathan, R. (2019). Influence of Changes in Rainfall and Soil Moisture on Trends in Flooding. J. Hydrol. 575, 432–441. doi:10.1016/j.jhydrol.2019.05.054
Wasko, C., Nathan, R., and Peel, M. C. (2020). Changes in Antecedent Soil Moisture Modulate Flood Seasonality in a Changing Climate. Water Resour. Res. 56. doi:10.1029/2019WR026300
Weaver, C. P., Lempert, R. J., Brown, C., Hall, J. A., Revell, D., and Sarewitz, D. (2013). Improving the Contribution of Climate Model Information to Decision Making: The Value and Demands of Robust Decision Frameworks. Wires Clim. Change 4, 39–60. doi:10.1002/wcc.202
Wheeler, K., Wenger, S. J., and Freeman, M. C. (2018). States and Rates: Complementary Approaches to Developing Flow-Ecology Relationships. Freshw. Biol. 63, 906–916. doi:10.1111/fwb.13001
Wolkovich, E. M., Cook, B. I., McLauchlan, K. K., and Davies, T. J. (2014). Temporal Ecology in the Anthropocene. Ecol. Lett. 17, 1365–1379. doi:10.1111/ele.12353
Keywords: environmental flows, climate change adaptation, freshwater ecosystems, climate stress testing, mechanistic modeling
Citation: John A, Horne A, Nathan R, Fowler K, Webb JA and Stewardson M (2021) Robust Climate Change Adaptation for Environmental Flows in the Goulburn River, Australia. Front. Environ. Sci. 9:789206. doi: 10.3389/fenvs.2021.789206
Received: 04 October 2021; Accepted: 10 November 2021;
Published: 06 December 2021.
Edited by:
Sharad Kumar Jain, Indian Institute of Technology Roorkee, IndiaReviewed by:
Nathaniel K. Newlands, Agriculture and Agri-Food Canada (AAFC), CanadaJoris Eekhout, Spanish National Research Council, Spain
Copyright © 2021 John, Horne, Nathan, Fowler, Webb and Stewardson. This is an open-access article distributed under the terms of the Creative Commons Attribution License (CC BY). The use, distribution or reproduction in other forums is permitted, provided the original author(s) and the copyright owner(s) are credited and that the original publication in this journal is cited, in accordance with accepted academic practice. No use, distribution or reproduction is permitted which does not comply with these terms.
*Correspondence: Andrew John, YW5kcmV3LmpvaG5AdW5pbWVsYi5lZHUuYXU=