- 1Department of Plant and Environmental Sciences, New Mexico State University, Las Cruces, NM, United States
- 2Natural Resource Ecology Laboratory, Colorado State University, Fort Collins, CO, United States
- 3World Agroforestry Centre, Nairobi, Kenya
Drylands are a critical part of the earth system in terms of total area, socioeconomic and ecological importance. However, while drylands are known for their contribution to inter-annual atmospheric CO2 variability, they are sometimes overlooked in discussions of global carbon stocks. Here, in preparation for the November 2021 UN Climate Change Conference (COP26), we review dryland systems with emphasis on their role in current and future carbon storage, response to climate change and potential to contribute to a carbon neutral future. Current estimates of carbon in dryland soils and vegetation suggest they are significant at global scale, containing approximately 30% of global carbon in above and below-ground biomass, and surface-layer soil carbon (top 30 cm). As ecosystems that are limited by water, the drylands are vulnerable to climate change. Climate change impacts are, however, dependent on future trends in rainfall that include both drying and wetting trends at regional scales. Regional rainfall trends will initiate trends in dryland productivity, vegetation structure and soil carbon storage. However, while management of fire and herbivory can contribute to increased carbon sequestration, impacts are dependent on locally unique ecosystem responses and climate-soil-plant interactions. Similarly, while community based agroforestry initiatives have been successful in some areas, large-scale afforestation programs are logistically infeasible and sometimes ecologically inappropriate at larger scales. As climate changes, top-down prescriptive measures designed to increase carbon storage should be avoided in favour of locally-adapted approaches that balance carbon management priorities with local livelihoods, ecosystem function, biodiversity and cultural, social and economic priorities.
1 Introduction
1.1 Drylands in the Earth System
The term “dryland” is used to describe water-limited ecosystems where the amount of precipitation (P, mm) is consistently less than the potential evapotranspiration (PET, mm). The balance between water supply and water demand can be conveniently represented using the ratio P/PET (Figure 1), with drylands typically defined for P/PET <0.65. Historically, drylands have been estimated to cover ∼40% of the earth’s land surface (excluding Greenland and Antarctica) (UNCCD 2017), but recent studies suggest that the bioclimatic conditions supporting dryland areas has increased in recent decades (Prăvălie et al., 2019; Huang et al., 2015; Yao et al., 2020; Feng & Fu, 2013). Drylands can be found in every continent, with the largest areas in Eurasia and Africa and significant drylands in Africa north and south of the equator, across most of Australia, parts of South America and western North American (Figure 1; Trabucco and Zomer, 2019). Rainfall in dryland areas can be highly variable, both temporally and spatially, increasing variability in vegetation production, and increasing uncertainty and risk for the rural and often marginalized communities that depend on the drylands (Global Mechanism of the UNCCD, Conservation International, 2019).
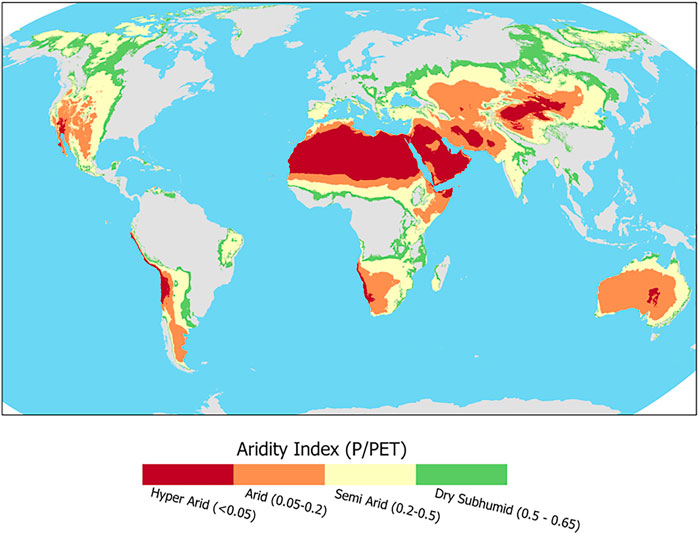
FIGURE 1. Location of the drylands based on annual precipitation (P) and potential evapotranspiration (PET), with hyper-arid (HA, P/PET <0.05), arid (A, 0.05 < P/PET <0.2), semi-arid (SA, 0.2 < P/PET <0.5) and dry sub-humid (DS, 0.5 < P/PET <0.65). Data used to construct this map derived from the Global Aridity and Potential Evapotranspiration (ETO) Climate Database (V2; Trabucco and Zomer, 2019) and the WorldClim historical climate data (V1; Fick and Hijmans, 2017).
Projections for future rainfall trends in global drylands vary between and within continents, with reduced annual rainfall totals projected in some areas, but increases possible in others (Asadi Zarch et al., 2017; Ross et al., 2021). However, the net impact of climate change in most drylands will be towards increasing aridity as temperatures and evaporative demands increase (Prăvălie et al., 2019). Here we review what is known about the current role of the drylands in the global carbon cycle and climate regulation, potential changes with climate change, and strategies for the drylands to contribute to a carbon neutral future.
1.2 Dryland Livelihoods
Drylands are home to more than 2 billion people, the majority of whom rely directly on the ecosystems in which they live for food production and incomes (FAO, 2011). Drylands are predominantly grasslands, but include savanna and drought seasonal shrublands, drought-seasonal woodlands, cold deserts of high latitude and high altitude, and hot deserts of the mid-latitudes (FAO 2011). Human land use in the drylands is dominated by livestock grazing, but with significant areas devoted to small-grain rain-fed agriculture in the dry sub-humid zones and irrigated crop production in areas with available water (Plaza et al., 2018). Over the last decades, land use in drylands has undergone significant change, particularly in tropical and mid-latitude drylands. Increasing population numbers, migration and other political and socio-economic drivers have reduced mobility, reducing prevalence of transhumant and nomadic herding systems, and shifting land use and tenure systems towards a greater reliance on settled grazing systems and more permanent cropland (Herrera et al., 2014). The move away from traditional land use systems, which in many regions use mobility as an effective adaptation to rainfall fluctuations, has contributed to increased incidence of land degradation and loss of vegetation cover (Reid et al., 2016).
1.3 Dryland Biodiversity
Drylands include a range of biomes from desert and arid steppe, to dry savannas and shrublands and seasonal woodlands, each with unique communities of flora and fauna (Maestre et al., 2021). Dryland species have adapted to a range of environmental extremes (particularly drought, herbivory and fire) which in some areas has led to high levels of diversification and hotspots of plant biodiversity, including the dry shrublands and forests of South and Central America, interior Australia and the Cape Floristic Region of South Africa. Indeed, about one third of global hotspots for biodiversity can be found in drylands (Davies et al., 2012). The association between grasses and large mammalian herbivores has also supported high faunal diversity in many drylands, including the mega-fauna, large mammalian grazers and diverse carnivore guilds that persist today in the savannas of Africa and Asia (Olff et al., 2002; Hempson et al., 2015). Many traditional land use systems practiced in drylands maintain the biodiversity on which they rely. For example, transhumance and nomadic livestock systems that emerged in many parts of the world in response to drought and cold-seasonality, use mobility as a strategy that not only reduces overgrazing and degradation, but also enhances coexistence with wild herbivores (Niamir-Fuller et al., 2012).
1.4 Dryland Carbon
Carbon stocks in drylands soils and vegetation are generally low, when expressed as carbon per unit area, relative to forested ecosystems, due to the constraints that temperature, moisture and coarse textured sandy soils place on annual primary productivity. Thus, for example, carbon stocks in above- and below-ground plant biomass are generally less than 100 Mg ha−1 C, except in the wetter dry subhumid regions (Figures 2A, B). Similarly, organic carbon stocks in the top 30 cm of soil (Figure 2C) are generally less than 50 Mg ha−1 in tropical (hot) drylands, but can be much higher in the high latitude (cold) drylands and mesic grasslands (Bardgett et al., 2021). In combination, however, total carbon (the sum of above and below-ground plant biomass and soil organic matter) can be considerable (e.g. > 200 Mg ha−1) across a large fraction of the drylands.
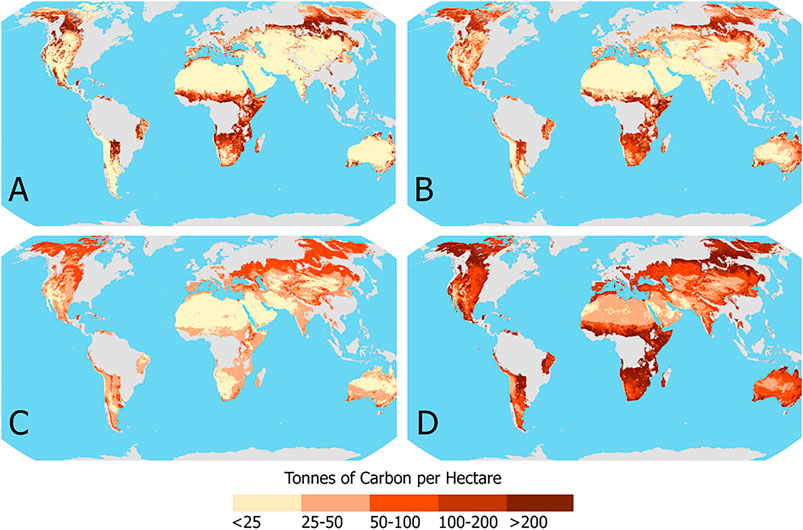
FIGURE 2. Maps showing global dryland carbon stocks (Mg ha−1 of C) in (A) above ground plant biomass, (B) below ground plant biomass, (C) soil organic matter (0–30 cm), and (D) the summed total carbon stocks (total = A + B + C). Data used to construct these maps are from Spawn and Gibbs, 2020, available at the Oak Ridge National Laboratories Distributed Active Archive Center (https://doi.org/10.3334/ORNLDAAC/1763) for biomass in above- and below-ground vegetation, and from Safriel, 2017 for carbon in the upper soil horizons (30 cm).
Global dryland carbon stock approximations, based on the data shown in Figures 2, 3, suggest that above- and below-ground biomass in the drylands sum to 790 Pg and 614 Pg, respectively, representing approximately 22 and 38% of global totals in these pools (Table 1). These biomass numbers for the drylands are high, relative to more conventional global carbon pool estimates (e.g. Friedlingstein et al., 2020), in part because tree biomass in the drylands is often assumed to be at or near zero (i.e. under-counted), and in part because the data in Figure 2 includes estimates for annual average herbaceous carbon stocks, which are a feature of dryland systems and not generally included in global carbon pool analyses. Table 1 also shows that soil organic matter carbon (SOMc) in shallow, more active, soil layers (0–30 cm) totals ∼407 Pg across the drylands, equivalent to ∼44% of global SOMc in surface soils. Independent estimates, including organic matter in deeper soils (0–200 cm), suggest total soil organic matter carbon (SOMc) in drylands on the order of 646 Pg, equivalent to approximately 32% of global shallow and deep soil carbon (Plaza et al., 2018). Further, soil inorganic carbon stocks tend to be higher in drylands, representing ∼79% of global inorganic soil carbon (1,558 Pg; Plaza et al., 2018).
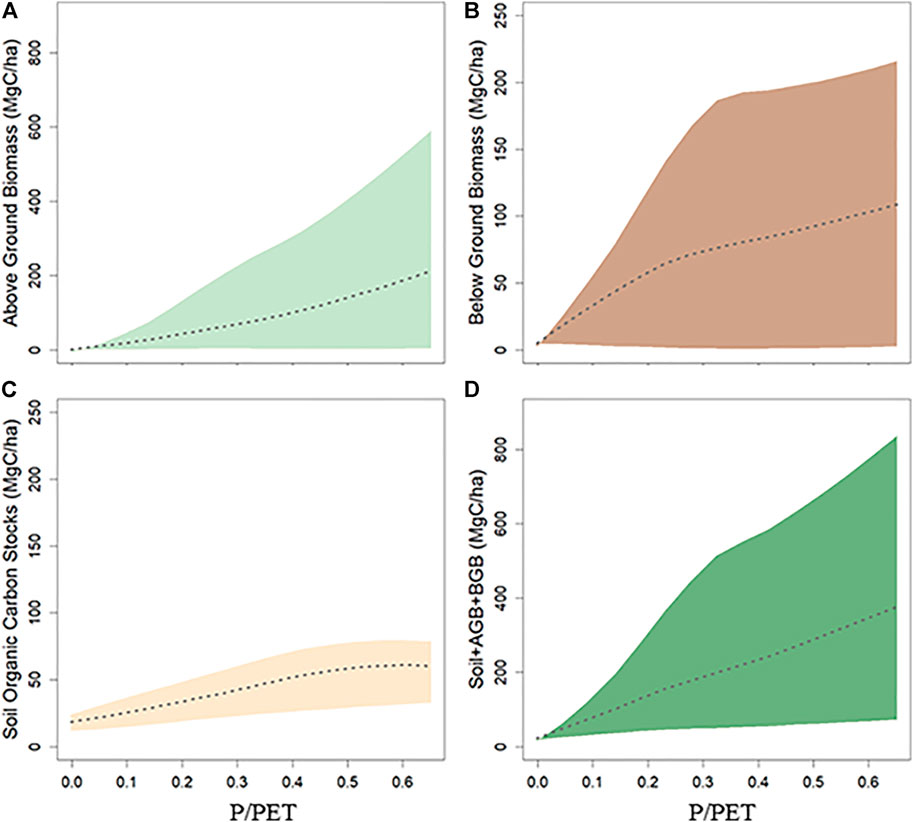
FIGURE 3. Average and variability in carbon stocks in global drylands increases with increasing water availability (P/PET). (A) Above ground biomass carbon, (B) Below ground biomass carbon, (C) Soil organic matter in the first 30 cm, and (D) Summed total carbon. Plots show means (dotted lines) and the 5th and 95th percentiles using local polynomial regression fitting for the datasets shown in Figure 2. Note, change in y-axis scale for (A,D) relative to (B,C).
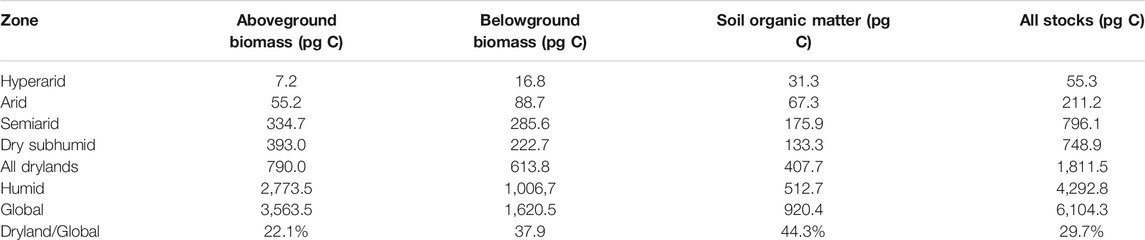
TABLE 1. Estimates of total carbon (C) in global drylands, summarizing data from Figure 2 for the aridity zones identified in Figure 1. The Spawn and Gibbs, 2020 biomass analyses are derived from data on above-ground wood and below-ground root biomass, with modelled/calibrated estimates of average carbon in herbaceous vegetation. Soil organic carbon estimates (Safriel, 2017) are for the top 30 cm of soil only, representing the more active layers. Carbon estimates for humid terrestrial systems (P/PET >0.65) and total terrestrial systems are from the same datasets (note that humid systems were not shown in Figures 1, 2 to emphasize the drylands).
While the estimates in Figures 2, 3 and Table 1 provide only broad approximations, the take-home message is clear: dryland carbon stocks are significant and should be considered a central, and essential, part of global carbon cycle calculations. It is critical to assess how climate and land use change in the coming decades will impact dryland ecosystems, altering rainfall and temperature in ways that may change vegetation dynamics and interact with changing land use, producing regions of net loss or accumulation of carbon in vegetation and both organic and inorganic carbon in soils. In particular, preservation of existing carbon stocks and restoration of degraded carbon stocks may, in some places, have the potential to play a significant role in climate change mitigation and national and international goals for a carbon neutral future.
2 Impacts of Climate Change on Drylands
2.1 Changing Climates
Inter-annual variability in rainfall, primary production and subsequent wild-fires in global drylands are the dominant contributors to inter-annual fluctuations in atmospheric CO2 concentrations (Williams et al., 2007; Ahlström et al., 2015). However, the long-term role of the drylands in mitigating carbon emissions will depend, not on inter-annual variability, but on long-term trajectories of carbon storage in vegetation (above and below ground) and soil organic and inorganic carbon. The recently released IPCC A6 Climate Change report found an increase in the incidence of hot extremes and agricultural drought in almost all dryland areas, meaning current land management strategies will need to be adapted in many drylands if they are to remain productive and habitable (IPCC 2019). For example, Prăvălie et al. (2019) used two databases of aridity to estimate the current extent of drylands and how this changed between 1950 and 2000. They found that dryland regions have already increased in all continents, with the exception of Europe and South America, with changes in bioclimatic conditions in China accounting for more than 30% of new arid land areas (Prăvălie et al., 2019). On a global scale, they attributed the expansion of dryland bioclimates to climate change experienced after 1980.
The analysis of climate change trajectories for the 21st century by Asadi Zarch et al., 2017 indicates widespread, but spatially variable, changes in aridity (P/PET; Figure 4). Their analysis used 22 general circulation models (GCM) and the “business as usual” emission scenario (i.e. assuming that effective international greenhouse emission reductions do not occur). The projections indicate that many dryland regions in North and South America, West and southern Africa, southern Europe, Central Asia and Australia will become more arid. However, significant regions in East Africa, South and East Asia, Siberia and Alaska may experience net increases in water availability. These interpretations are in substantial agreement with the earlier analysis of Feng and Fu (2013).
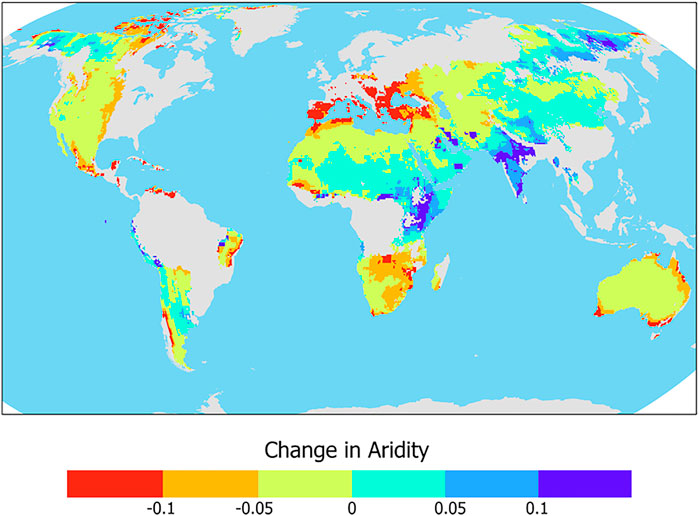
FIGURE 4. Change in aridity (P/PET) projected for 2076–2100 relative to 2006–2025 averaged across 22 GCM participating in the climate model inter-comparison project (CMIP5) for continued greenhouse gas emissions and a cumulative 8.5 W additional radiative forcing (RCP 8.5). Figure reproduced from original analysis of Zarch et al., 2017.
These regional differences in climate change trajectories represent both challenges and opportunities for carbon sequestration, with divergent responses to climate change signals between tropical, temperate and arctic drylands. In the tropics and most temperate drylands, increases in water availability will generally be correlated with increasing carbon in both soils and vegetation, while increasing aridity will tend to reduce carbon stocks (Figure 2). In arctic systems, however, increasing temperatures and melting permafrost, regardless changing rainfall, risk mobilizing huge reservoirs of ancient carbon hitherto immobilized in the permafrost (Turetsky et al., 2019).
2.2 Changing Vegetation
The impacts of climate change on dryland vegetation are both indirect and direct. Direct effects include increases in hot extremes and meteorological droughts that could push grassland and savanna systems beyond tipping points leading to dryland degradation (Bernardino et al., 2020). Indeed, recent analyses suggests that 20–35% of drylands are degraded (IUCN 2019). According to the IPCC Special Report on Climate and Land (IPCC 2019), climate change-induced degradation has reduced pastoral productivity in dryland areas of Africa, with associated reductions in livestock productivity. This is a trend that is likely to continue in drylands in Africa and Asia (IPCC 2019). Indirectly, climate change will also impact land use options and, in low-income drylands, contribute to migration, urban expansion and conversion of drylands to cropland (Fu et al., 2021).
At global and continental scales, changing rainfall patterns could lead to decreasing or increasing vegetation production and carbon stocks in woody biomass. For example, recent analysis of impacts of 21st Century climate change in Africa, suggests that at continental scales changing temperature, rainfall and land use patterns will have a small negative impact on the carbon stored in woody vegetation across the entirety of Sub-Saharan Africa (Ross et al., 2021). However, at regional scales, distinct regional drying in Southern and West Africa will tend to reduce woody biomass, while projected increases in rainfall in parts of East Africa will tend to increasing vegetation biomass in that region. The analysis of Ross et al. (2021) also indicates that the interactions between climate and land use will impact different dryland regions in different ways, depending on the changing rainfall patterns, projected changes in human populations, land use and use of fire. Thus a picture emerges of differential risks and opportunities for carbon sources and sinks across global drylands.
2.3 Changing Soil Carbon
In regions experiencing increasing drought and heat extremes, soil organic carbon can be reduced through reduced inputs from vegetation primary production and increased exposure of soils to wind and water erosion (Okin et al., 2018). Increasing frequency of other extremes, such as intense rainfall events, can lead to surface sealing preventing moisture infiltration, reduced plant growth and ultimately a reduction in soil carbon stocks (Fu et al., 2021). In common with vegetation, climate impacts on soil carbon stocks can also be indirect, with conversion to cropland driven by population growth and climate change induced migration leading to losses of up to 60% of SOC stocks in dryland soils (IUCN 2019). The differences in above-ground woody biomass across gradients in tree and shrub cover are not necessarily associated with similar changes in SOMc carbon or root biomass, because grasslands can also store significant amounts of below-ground carbon in both SOMc and root biomass pools (Jackson et al., 2002; Schutz et al., 2009; Dass et al., 2018).
2.4 Climate Feedbacks
While drylands are sensitive to climate change, their large spatial extent means they may also have key roles to play in global climate regulation, with the potential to either exacerbate or mitigate global warming. Climate feedbacks via albedo, surface roughness and boundary layer interactions (controlling surface temperature, atmospheric stability, cloud formation and rainfall) were once thought of as a key mechanism linking vegetation degradation in arid regions to future rainfall (Otterman, 1974; Charney et al., 1975). More recent observations and climate model simulations, however, indicate that global sea surface temperatures generally provide the stronger forcing for regional drought than do local or regional-scale land surface-atmosphere interactions (Giannini et al., 2003; Neelin et al., 2003; Held et al., 2005; Lu and Delworth, 2005). On the other hand, the vast spatial extent of the drylands may mean that the cumulative effects of, for example, reduced evapotranspiration could impact regional scale rainfall (as inferred from model experiments in Zemp et al., 2017). Indeed, evidence has already been found for drying climates in higher latitudes in both Asia and North America which can threaten carbon stocks stored in organic soils associated with boreal forests and permafrost (Prăvălie et al., 2019). Furthermore, increases in dust emissions from the drylands, and industrial aerosol emissions from remote urban centers, can deflect incoming radiation impacting cloud formation and precipitation (Booth et al., 2012; Choobari et al., 2014).
3 Management Opportunities for Drylands in a Carbon Neutral Future
3.1 International Agreements
The ability to limit global warming below 2 °C, the target set by the Paris Climate Agreement, is predicated on significant greenhouse gas (GHG) emission reductions in the land use sector (Wollenberg et al., 2016). While land use accounts for nearly 25% of net annual anthropogenic GHG emissions (Roe et al., 2019), it also offers opportunities to mitigate climate change. Estimates suggest that globally land use interventions could generate up to 30% of the emission reductions and carbon sequestration needed to meet the Paris Agreements (Griscom et al., 2017). Given the huge area of the drylands, efforts to preserve and enhance carbon in dryland vegetation and soils will be a critical part of this effort. This is recognised by the UN’s Convention to Combat Degradation (UNCCD), which has set forth an aim to achieve Land Degradation Neutrality by 2030. To date 127 countries have signed up to work towards LDN, including many countries in drylands where vegetation degradation, and associated loss of soil and biomass carbon, is an ongoing risk.
Various international agreements as part of the UN Decade on Ecosystem Restoration, including the Rio Conventions, the Bonn Challenge and related regional initiatives, provide a total global range of commitments from 765 million to 1 billion hectares restored, or undergoing restoration, by 2030 (Sewell et al., 2020). The commitments cover a wide range of land use types including forest (42%) and agricultural lands, including cropland and grassland (37%; Sewell et al., 2020). Almost half of all restoration commitments are in Sub-Saharan Africa. To narrow this geographic imbalance, there may be scope to expand dryland restoration and carbon management programs in underrepresented regions, including North America, Russia and Central Asia, West and Central Europe, the Middle East and Northern Africa (Sewell et al., 2020).
3.2 Land Use
The human populations inhabiting drylands have devised land use and management strategies which are resilient and adaptable. Future adaptations to climate change in drylands need to build on this history, engaging with local communities to ensure policies are informed by local practices, strategies and governance structures. In addition, management practices directed towards the preservation or enhancement of carbon stocks in the drylands must, first and foremost, be compatible with the livelihoods, sustainability and autonomy of local populations, while also addressing wildlife and conservation priorities (Reid et al., 2016). Adaptation to climate change in drylands will likely demand further diversification, meaning markets will also need to adapt to accommodate the production of drought and heat resistant crops (ICARDA/CCAFS, 2012).
3.3 Afforestation and Reforestation for Carbon Sequestration
Arguments have been made for carbon mitigation in drylands based on afforestation or reforestation of existing, and often ancient, grasslands and savannas (Bastin et al., 2019). Whether this should be considered either feasible or desirable is the topic of some considerable debate (Veldman et al., 2015; Bastin et al., 2019; Bond et al., 2019; Lewis et al., 2019; Veldman et al., 2019; Aynekulu et al., 2021). Bastin et al., 2019, modelled the potential climate change mitigation impacts of increasing global forest cover, including increasing tree cover in the drylands. However, a global assessment of this type tends to overlook issues specific to local dryland systems, and the logistical, ecological and social-ecological difficulties that so often cause large-scale reforestation programs to fail (Coleman et al., 2021; Del Campo et al., 2021). At local scales, where social-ecological conditions permit, small scale afforestation programs can be managed to contribute to carbon storage, while also meeting community needs for timber, fruit, nuts and fuelwood (Sendzimir et al., 2011; Hanan, 2018). Community-driven and locally appropriate programs have been successful, but the potential mismatch of top-down programs with local socioeconomic and cultural priorities, and impacts on ecosystem function and biodiversity, need careful consideration (Herrmann and Tappan, 2013; Bond et al., 2019).
3.4 Livestock Management
Managing livestock for carbon sequestration in drylands will depend upon an increased understanding of the interacting impacts of changing climate and grazing practices. Studies have suggested that seasonal rainfall patterns (modal, bi-modal, shorter and longer rainy seasons, etc.) are as important as total annual precipitation in determining how grazing and precipitation interact to effect soil carbon stocks. For example, von Wehrden et al., 2012 reviewed more than 50 studies and found that in higher rainfall areas with low inter-annual variability, there was a high incidence of degradation caused by grazing. This is consistent with the Ellis and Swift (1988) model, suggesting that high variability in rainfall buffers drylands against degradation due to long-term limits on livestock populations. Another review by Derner and Schuman (2007) suggested a threshold of 600 mm mean annual precipitation above which grazing tends to reduce soil carbon in rangelands. In drylands with rainfall below this threshold, however, inter-annual variability in rainfall has a larger impact on soil carbon stocks than grazing pressure. As climates change, the impact of grazing on plant community composition and palatability will also influence pastoral sustainability, diversity, structure and function of the drylands (Koerner et al., 2018).
3.5 Fire
Fire is used as a management practice in many drylands. It can increase nutrients in the soil, leading to a flush of new growth for grazing animals. However, frequent fires release biomass carbon and other greenhouse gases to the atmosphere (Scholes and Andreae, 2000) and tend to reduce long-term tree cover and tree biomass (Hanan and Lehmann, 2011). However, the short-term impacts of fire on above ground vegetation in ecosystems adapted to fire has relatively little impact on annual carbon balance, since the primary fuels are short-lived herbaceous plants where carbon uptake during the growing season inevitably turns-over rapidly either through fire, or herbivory or decomposition (Bond and Keely, 2005). For example, Hanan et al. (2008) showed that in savannas exposed to frequent fire, individual fires may remove tree seedlings, and thus prevent increase in tree cover, but they have relatively little impact on carbon stocks in larger, already established and fire resistant trees. The impacts of fire on carbon held in the soil can be equally complex. Fire can increase input of black carbon to the soil, but can also increase soil respiration causing a net loss (Scholes and Andreae, 2000). Frequency and timing of burning are a major determinant of carbon changes in soil. Burning during the dry season has been shown to result in higher losses of SOC, whereas cooler wet season fires may have no effect on SOC (Fynn et al., 2003). In addition, the loss of ground cover during fires can be associated with increased soil erosion (and loss of SOC) in subsequent rainfall events (Everson et al., 1989; Okin et al., 2018). Thus fire management provides opportunities for preservation and enhancement of carbon in soils and vegetation, but top-down proscriptive approaches should be avoided in favour of locally-adapted programs that recognize the local constraints on carbon dynamics and local social and ecological priorities.
4 Conclusion and Recommendation
Drylands are a critical part of the earth system in terms of total area, socioeconomic and ecological importance (Figure 1). While somewhat overlooked in discussions of global carbon dynamics, their large spatial extent means they are in fact highly significant for global terrestrial carbon storage in both vegetation and soils (Figure 2; Table 1). As ecosystems that are, by definition, limited by water availability, the drylands are vulnerable to long-term increases in temperature. Climate change impacts are, however, particularly dependent on future trends in rainfall that climate model scenarios indicate will include both drying and wetting trends at regional scales (Figure 4). Regional rainfall trends will initiate trends in dryland productivity, vegetation structure and soil carbon storage (Figure 3). By virtue of their existing large carbon stocks, coupled with management opportunities to protect and restore carbon, drylands can also play a significant role in climate regulation. In particular, drylands have the potential to either exacerbate or mitigate global warming based on local and regional changes in soil organic matter and biomass in woody vegetation structure, particularly via management of fire and grazing, and community-based local afforestation and agroforestry initiatives. However, while management of fire and herbivory can contribute to increased carbon sequestration, results are highly dependent on locally unique ecosystem responses and climate, soil, plant interactions. Globally, drylands are home to more than 2 billion people, meaning that future climate changes and top-down management decisions will impact about a quarter of the world’s population. However, many dryland communities have developed land management systems which are well adapted to historical and current social, ecological and climatological conditions. As the climate changes in the coming decades, top-down prescriptive measures designed to increase carbon storage should be avoided in favour of locally-adapted approaches that balance carbon management priorities with local livelihoods, ecosystem function, biodiversity and cultural, social and economic priorities.
Author Contributions
NH and EM conceived the paper and wrote the manuscript. JA and QY analyzed data for figures and table. All authors contributed to manuscript development and edited the final version.
Funding
This work was supported by funding from the National Science Foundation to New Mexico State University for the Jornada Basin Long-Term Ecological Research Program (DEB 2025166) and from the National Aeronautics and Space Administration SERVIR, ICESat2, and GEDI missions (awards 80NSSC20K0162, 80NSSC20K0976, and 80NSSC21K0201, respectively).
Conflict of Interest
The authors declare that the research was conducted in the absence of any commercial or financial relationships that could be construed as a potential conflict of interest.
Publisher’s Note
All claims expressed in this article are solely those of the authors and do not necessarily represent those of their affiliated organizations, or those of the publisher, the editors and the reviewers. Any product that may be evaluated in this article, or claim that may be made by its manufacturer, is not guaranteed or endorsed by the publisher.
Acknowledgments
Our thanks to Dr. Mohammad Amin Asadi Zarch (Yazd University, Iran) for kindly providing data for Figure 4.
References
Ahlström, A., Raupach, M. R., Schurgers, G., Smith, B., Arneth, A., Jung, M., et al. (2015). The Dominant Role of Semi-arid Ecosystems in the Trend and Variability of the Land CO 2 Sink. Science 348 (6237), 895–899. doi:10.1126/science.aaa1668
Asadi Zarch, M. A., Sivakumar, B., Malekinezhad, H., and Sharma, A. (2017). Future Aridity under Conditions of Global Climate Change. J. Hydrol. 554, 451–469. doi:10.1016/j.jhydrol.2017.08.043
Aynekulu, E., Sileshi, G. W., Rosenstock, T. S., van Noordwijk, M., Tsegaye, D., Koala, J., et al. (2021). No Changes in Soil Organic Carbon and Nitrogen Following Long-Term Prescribed Burning and Livestock Exclusion in the Sudan-savanna Woodlands of Burkina Faso. Basic Appl. Ecol. 56, 165–175. doi:10.1016/j.baae.2021.07.007
Bardgett, R. D., Bullock, J. M., Lavorel, S., Manning, P., Schaffner, U., Ostle, N., et al. (2021). Combatting Global Grassland Degradation. Nat. Rev. Earth Environ. 2, 720–735. doi:10.1038/s43017-021-00207-2
Bastin, J.-F., Finegold, Y., Garcia, C., Mollicone, D., Rezende, M., Routh, D., et al. (2019). The Global Tree Restoration Potential. Science 365, 76–79. doi:10.1126/science.aax0848
Bernardino, P. N., De Keersmaecker, W., Fensholt, R., Verbesselt, J., Somers, B., Horion, S., et al. (2020). Global‐scale Characterization of Turning Points in Arid and Semi‐arid Ecosystem Functioning. Glob. Ecol Biogeogr 29 (7), 1230–1245. doi:10.1111/geb.13099
Bond, W. J., Stevens, N., Midgley, G. F., and Lehmann, C. E. R. (2019). The Trouble with Trees: Afforestation Plans for Africa. Trends Ecol. Evol. 34 (11), 963–965. doi:10.1016/j.tree.2019.08.003
Bond, W., and Keeley, J. (2005). Fire as a Global 'herbivore': the Ecology and Evolution of Flammable Ecosystems. Trends Ecol. Evol. 20, 387–394. doi:10.1016/j.tree.2005.04.025
Booth, B. B. B., Dunstone, N. J., Halloran, P. R., Andrews, T., and Bellouin, N. (2012). Aerosols Implicated as a Prime Driver of Twentieth-century North Atlantic Climate Variability. Nature 484, 228–232. doi:10.1038/nature10946
Charney, J., Stone, P. H., and Quirk, W. J. (1975). Drought in the Sahara: A Biogeophysical Feedback Mechanism. Science 187, 434–435. doi:10.1126/science.187.4175.434
Choobari, O. A., Zawar-Reza, P., and Sturman, A. (2014). The Global Distribution of mineral Dust and its Impacts on the Climate System: A Review. Atmos. Res. 138, 152–165. doi:10.1016/j.atmosres.2013.11.007
Coleman, E. A., Schultz, B., Ramprasad, V., Fischer, H., Rana, P., Filippi, A. M., et al. (2021). Limited Effects of Tree Planting on forest Canopy Cover and Rural Livelihoods in Northern India. Nat. Sustain. doi:10.1038/s41893-021-00761-z
Dass, P., Houlton, B. Z., Wang, Y., and Warlind, D. (2018). Grasslands May Be More Reliable Carbon Sinks Than Forests in California. Environ. Res. Lett. 13, 074027. doi:10.1088/1748-9326/aacb39
Davies, J., Poulsen, L., Schulte-Herbrüggen, B., Mackinnon, K., Crawhall, N., Henwood, W. D., et al. (2012). Conserving Dryland Biodiversity.
Del Campo, A. D., Segura-Orenga, G., Bautista, I., Ceacero, C. J., González-Sanchis, M., Molina, A. J., et al. (2021). Assessing Reforestation Failure at the Project Scale: The Margin for Technical Improvement under Harsh Conditions. A Case Study in a Mediterranean Dryland. Sci. Total Environ. 796, 148952. doi:10.1016/j.scitotenv.2021.148952
Derner, J. D., and Schuman, G. E. (2007). Carbon Sequestration and Rangelands: a Synthesis of Land Management and Precipitation Effects. J. Soil Water Conservation 62, 77–85.
Ellis, J. E., and Swift, D. M. (1988). Stability of African Pastoral Ecosystems: Alternate Paradigms and Implications for Development. J. Range Manage. 41 (No. 6), 450–459. doi:10.2307/3899515
Everson, C. S., George, W. J., and Schulze, R. E. (1989). Fire Regime Effects on Canopy Cover and Sediment Yield in the Montane Grasslands of Natal South. African. J. Sci. 85, 113–116. FAO (2004). Carbon sequestration in dryland soilsWorld Soil Resour. Reports, 102.
FAO (2011). London. Available at: http://www.fao.org/3/i1688e/i1688e01.pdf.The State of the World’s Land and Water Resources for Food and Agriculture (SOLAW) – Managing Systems at Risk. Rome and Earthscan
Feng, S., and Fu, Q. (2013). Expansion of Global Drylands under a Warming Climate. Atmos. Chem. Phys. 13, 10081–10094. doi:10.5194/acp-13-10081-2013
Fick, S. E., and Hijmans, R. J. (2017). WorldClim 2: New 1‐km Spatial Resolution Climate Surfaces for Global Land Areas. Int. J. Climatol 37, 4302–4315. doi:10.1002/joc.5086
Friedlingstein, P., O’Sullivan, M., Jones, M. W., Andrew, R. M., Hauck, J., Olsen, A., et al. (2020). Global Carbon Budget 2020. Earth Syst. Sci. Data 12, 3269–3340. doi:10.5194/essd-12-3269-2020
Fu, C., Chen, Z., Wang, G., Yu, X., and Yu, G. (2021). A Comprehensive Framework for Evaluating the Impact of Land Use Change and Management on Soil Organic Carbon Stocks in Global Drylands. Curr. Opin. Environ. Sustainability 48, 103–109. doi:10.1016/j.cosust.2020.12.005
Fynn, R. W. S., Haynes, R. J., and O'Connor, T. G. (2003). Burning Causes Long-Term Changes in Soil Organic Matter Content of a South African Grassland. Soil Biol. Biochem. 35, 677–687. doi:10.1016/s0038-0717(03)00054-3
Giannini, A., Saravanan, R., and Chang, P. (2003). Oceanic Forcing of Sahel Rainfall on Interannual to Interdecadal Time Scales. Science 302, 1027–1030. doi:10.1126/science.1089357
Global Mechanism of the UNCCD, Conservation International (2019). Land Degradation, Poverty and Inequality. Bonn, Germany.
Griscom, B. W., Adams, J., Ellis, P. W., Houghton, R. A., Lomax, G., Miteva, D. A., et al. (2017). Natural Climate Solutions. Proc. Natl. Acad. Sci. USA 114, 11645–11650. doi:10.1073/pnas.1710465114
Hanan, N. P. (2018). Agroforestry in the Sahel. Nat. Geosci 11, 296–297. doi:10.1038/s41561-018-0112-x
Hanan, N. P., and Lehmann, C. E. R. (2011). “Tree-grass Interactions in Savannas: Paradigms, Contradictions and Conceptual Models,” in Ecosystem Function in Savannas: Measurement and Modeling at Landscape to Global Scales. Editors M. J. Hill, and N. P. Hanan (Boca Raton: CRC Press), 39–56.
Hanan, N. P., Sea, W. B., Dangelmayr, G., and Govender, N. (2008). Do fires in Savannas Consume Woody Biomass? A Comment on Approaches to Modeling savanna Dynamics. The Am. Naturalist 171, 851–856. doi:10.1086/587527
Held, I. M., Delworth, T. L., Lu, J., Findell, K. L., and Knutson, T. R. (2005). Simulation of Sahel Drought in the 20th and 21st Centuries. Proc. Natl. Acad. Sci. 102, 17891–17896. doi:10.1073/pnas.0509057102
Hempson, G. P., Archibald, S., and Bond, W. J. (2015). A Continent-wide Assessment of the Form and Intensity of Large Mammal Herbivory in Africa. Science 350, 1056–1061. doi:10.1126/science.aac7978
Herrmann, S. M., and Tappan, G. G. (2013). Vegetation Impoverishment Despite Greening: A Case Study from central Senegal. J. Arid Environments 90, 55–66. doi:10.1016/j.jaridenv.2012.10.020
Huang, J., Yu, H., Guan, X., Wang, G., and Guo, R. (2015). Accelerated Dryland Expansion under Climate Change. Nat. Clim Change 6 (2), 166–171. doi:10.1038/nclimate2837
ICARDA/CCAFS (2012). “Food Security in Dry Lands,” in Strategies for Combating Climate Change in Drylands Agriculture (Qatar: DohaCCAFS), 27.
IPCC (2019). “Summary for Policymakers,” in Climate Change and Land: An IPCC Special Report on Climate Change, Desertification, Land Degradation, Sustainable Land Management, Food Security, and Greenhouse Gas Fluxes in Terrestrial Ecosystems. P. R. Shukla, J. Skea, E. C. Buendia, V. Masson-Delmotte, H.-O. Pörtner, D. C. Robertset al. Editors 00020. doi:10.4337/9781784710644
IUCN (2019). Drylands and Climate, Issues Brief. 2. Available at: https://www.iucn.org/sites/dev/files/iucn_issues_brief_drylands_and_climate_change_sept_2019.pdf [Accessed September 16, 2021].
Jackson, R. B., Banner, J. L., Jobbágy, E. G., Pockman, W. T., and Wall, D. H. (2002). Ecosystem Carbon Loss with Woody Plant Invasion of Grasslands. Nature 418, 623–626. doi:10.1038/nature00910
Koerner, S. E., Smith, M. D., Burkepile, D. E., Hanan, N. P., Avolio, M. L., Collins, S. L., et al. (2018). Change in Dominance Determines Herbivore Effects on Plant Biodiversity. Nat. Ecol. Evol. 2 (12), 1925–1932. doi:10.1038/s41559-018-0696-y
Lewis, S. L., Mitchard, E. T. A., Prentice, C., Maslin, M., and Poulter, B. (2019). Comment on "The Global Tree Restoration Potential". Science 366 (6463). doi:10.1126/science.aaz0388
Lu, J., and Delworth, T. L. (2005). Oceanic Forcing of the Late 20th century Sahel Drought. Geophys. Res. Lett. 32, a–n. doi:10.1029/2005GL023316
Maestre, F. T., Benito, B. M., Berdugo, M., Concostrina‐Zubiri, L., Delgado‐Baquerizo, M., Eldridge, D. J., et al. (2021). Biogeography of Global Drylands. New Phytol. 231, 540–558. doi:10.1111/nph.17395
Magero, C. (2019). Drylands and Climate Change. Available at: https://www.iucn.org/sites/dev/files/content/documents/drylands_and_climate_change_gdi.pdf.
Neelin, J. D., Chou, C., and Su, H. (2003). Tropical Drought Regions in Global Warming and El Niño Teleconnections. Geophys. Res. Lett. 30, 1–4. doi:10.1029/2003GL018625
Niamir-Fuller, M., Kerven, C., Reid, R., and Milner-Gulland, E. (2012). Co-existence of Wildlife and Pastoralism on Extensive Rangelands: Competition or Compatibility. Pastoralism: Res. Pol. Pract. 2, 8–14. doi:10.1186/2041-7136-2-8
Okin, G. S., Sala, O. E., Vivoni, E. R., Zhang, J., and Bhattachan, A. (2018). The Interactive Role of Wind and Water in Functioning of Drylands: What Does the Future Hold. BioScience 68 (9), 670–677. doi:10.1093/biosci/biy067
Olff, H., Ritchie, M. E., and Prins, H. H. T. (2002). Global Environmental Controls of Diversity in Large Herbivores. Nature 415 (6874), 901–904. doi:10.1038/415901a
Otterman, J. (1974). Baring High-Albedo Soils by Overgrazing: A Hypothesized Desertification Mechanism. Science 186, 531–533. doi:10.1126/science.186.4163.531
P. Herrera, J. Davies, and P. Manzano (Editors) (2014). The Governance of Rangelands: Collective Action for Sustainable Pastoralism. 1st ed. (UK: Routledge).
Plaza, C., Zaccone, C., Sawicka, K., Méndez, A. M., Tarquis, A., Gascó, G., et al. (2018). Soil Resources and Element Stocks in Drylands to Face Global Issues. Sci. Rep. 8. 13788. doi:10.1038/s41598-018-32229-0
Prăvălie, R., Bandoc, G., Patriche, C., and Sternberg, T. (2019). Recent Changes in Global Drylands: Evidences from Two Major Aridity Databases. Catena 178, 209–231. doi:10.1016/j.catena.2019.03.016
Reid, R., Gichohi, H., Said, M., Nkedianye, D., Ogutu, J., Kshatriya, M., et al. (2008). “Fragmentation of a Peri-Urban savanna, Athi-Kaputiei Plains, Kenya,” in Fragmentation in Semi-arid and Arid Landscapes. Editors K. A. Galvin, R. S. Reid, R. H. Behnke, and N. T. Hobbs (Dordrecht, Netherlands: Springer), 195–224. Available at: https://link.springer.com/chapter/10.1007/978-1-4020-4906-4_9#citeas. doi:10.1007/978-1-4020-4906-4_9
Reid, R. S., Nkedianye, D., Said, M. Y., Kaelo, D., Neselle, M., Makui, O., et al. (2016). Evolution of Models to Support Community and Policy Action with Science: Balancing Pastoral Livelihoods and Wildlife Conservation in Savannas of East Africa. Proc. Natl. Acad. Sci. USA 113, 4579–4584. doi:10.1073/pnas.0900313106
Roe, S., Streck, C., Obersteiner, M., Frank, S., Griscom, B., Drouet, L., et al. (2019). Contribution of the Land Sector to a 1.5 °C World. Nat. Clim. Chang. 9, 817–828. doi:10.1038/s41558-019-0591-9
Ross, C. W., Hanan, N. P., Prihodko, L., Anchang, J., Ji, W., and Yu, Q. (2021). Woody-biomass Projections and Drivers of Change in Sub-saharan Africa. Nat. Clim. Chang. 11, 449–455. doi:10.1038/s41558-021-01034-5
Safriel, U. (2017). Land Degradation Neutrality (LDN) in Drylands and beyond – where Has it Come from and where Does it Go. Silva Fenn 51, 1–9. doi:10.14214/sf.1650
Scholes, M., and Andreae, M. O. (2000). Biogenic and Pyrogenic Emissions from Africa and Their Impact on the Global Atmosphere. AMBIO: A J. Hum. Environ. 29, 23–29. doi:10.1579/0044-7447-29.1.23
Schutz, A. E. N., Bond, W. J., and Cramer, M. D. (2009). Juggling Carbon: Allocation Patterns of a Dominant Tree in a Fire-Prone savanna. Oecologia 160, 235–246. doi:10.1007/s00442-009-1293-1
Sendzimir, J., Reij, C. P., and Magnuszewski, P. (2011). Rebuilding Resilience in the Sahel: Regreening in the Maradi and Zinder Regions of Niger. E&S 16 (3). doi:10.5751/es-04198-160301
Sewell, A., Esch, S. V. D., and Lowenhardt, H. (2020). Goals and Commitments for the Restoration Decade: A Global Overview of Countries’ Restoration Commitments under the Rio Conventions and Other Pledges. The Hague Available at: https://www.pbl.nl/sites/default/files/downloads/pbl-2020-goals-and-commitments-for-the-restoration-decade-3906.pdf.
Spawn, S. A., and Gibbs, H. K. (2020). Global Aboveground and Belowground Biomass Carbon Density Maps for the Year 2010. doi:10.3334/ORNLDAAC/1763
Trabucco, A., and Zomer, R. (2019). Global Aridity Index and Potential Evapotranspiration (ET0) Climate Database V2. Figshare. Dataset. doi:10.6084/m9.figshare.7504448.v3
Turetsky, M. R., Abbott, B. W., Jones, M. C., Walter Anthony, K., Olefeldt, D., Schuur, E. A. G., et al. (2019). Permafrost Collapse Is Accelerating Carbon Release. Nature 569 (7754), 32–34. doi:10.1038/d41586-019-01313-4
UNCCD (2017). The Global Land Outlook. 1st ed. Bonn, Germany. Available at: https://www.unccd.int/actions/global-land-outlook-glo.
Veldman, J. W., Aleman, J. C., Alvarado, S. T., Anderson, T. M., Archibald, S., Bond, W. J., et al. (2019). Comment on "The Global Tree Restoration Potential".” Science. 366, 1–5. doi:10.1126/science.aay7976
Veldman, J. W., Overbeck, G. E., Negreiros, D., Mahy, G., Le Stradic, S., Fernandes, G. W., et al. (2015). Where Tree Planting and forest Expansion Are Bad for Biodiversity and Ecosystem Services. Bioscience 65, 1011–1018. doi:10.1093/biosci/biv118
von Wehrden, H., Hanspach, J., Kaczensky, P., Fischer, J., and Wesche, K. (2012). Global Assessment of the Non-Equilibrium Concept in Rangelands. Ecol. Appl. 22 (2), 393–399. doi:10.1111/gcb.1334010.1890/11-0802.1
Williams, C. A., Hanan, N. P., Neff, J. C., Scholes, R. J., Berry, J. A., Denning, A. S., et al. (2007). Africa and the Global Carbon Cycle. Carbon Balance Manage 2 (March 2007), 3. doi:10.1186/1750-0680-2-3)
Wollenberg, E., Richards, M., Smith, P., Havlík, P., Obersteiner, M., Tubiello, F. N., et al. (2016). Reducing Emissions from Agriculture to Meet the 2 °C Target. Glob. Change Biol. 22, 3859–3864. doi:10.1111/gcb.13340
Yao, J., Liu, H., Huang, J., Gao, Z., Wang, G., Li, D., et al. (2020). Accelerated Dryland Expansion Regulates Future Variability in Dryland Gross Primary Production. Nat. Commun. 11 (1), 1665. doi:10.1038/s41467-020-15515-2
Zarch, M. A., Sivakumar, B., Malekinezhad, H., and Sharma, A. (2017). Future Aridity Under Conditions of Global Climate Change. J. Hydrol. 554, 451–469. doi:10.1016/j.jhydrol.2017.08.043
Keywords: drylands, carbon cycle, climate change, soil carbon (C) sequestration, vegetation carbon
Citation: Hanan NP, Milne E, Aynekulu E, Yu Q and Anchang J (2021) A Role for Drylands in a Carbon Neutral World?. Front. Environ. Sci. 9:786087. doi: 10.3389/fenvs.2021.786087
Received: 29 September 2021; Accepted: 27 October 2021;
Published: 05 November 2021.
Edited by:
Heping Liu, Washington State University, United StatesReviewed by:
Zhongming Gao, Sun Yat-sen University, ChinaLei Deng, Northwest A and F University, China
Copyright © 2021 Hanan, Milne, Aynekulu, Yu and Anchang. This is an open-access article distributed under the terms of the Creative Commons Attribution License (CC BY). The use, distribution or reproduction in other forums is permitted, provided the original author(s) and the copyright owner(s) are credited and that the original publication in this journal is cited, in accordance with accepted academic practice. No use, distribution or reproduction is permitted which does not comply with these terms.
*Correspondence: Niall P. Hanan, nhanan@nmsu.edu