- 1The National Natural History Collections, The Hebrew University of Jerusalem, Jerusalem, Israel
- 2Department of Evolutionary and Environmental Biology, University of Haifa, Haifa, Israel
- 3Department of Biology and Environment, University of Haifa–Oranim, Tiv′on, Israel
In order to integrate parasitoid wasps in agroecosystems as biological control agents, we need to understand how insecticides affect the parasitoids in the crops and their surroundings. We investigated the non-target effect of Indoxacarb, an insecticide commonly used against European grapevine moth, on parasitoid wasp communities in vineyards. We focused on characterizing: 1. The dynamics of common wasp species, and 2. Wasp abundance and species richness in the vineyard center, edge, and nearby natural area. Seven vineyards, with neighboring natural areas, were sampled before, and up to 2 weeks after, Indoxacarb applications over 2 years. We expected initial negative effects of spraying in the vineyard with some effect of Indoxacarb drift into the natural habitat, followed by wasp recovery, first in natural areas, then at the vineyard edge and finally in the center. Sticky traps were hung at the vineyard edge and center to evaluate migration into and out of the vineyard. Vacuum sampling was used to obtain parasitoid total abundance and species richness, and the abundances of four common species (43% of the wasps collected). From the vacuum samples we found that total wasp abundance and richness declined after spraying in the vineyards’ margins and center but rose over time in the natural area. Vineyard wasp abundance was restored to pre-spraying levels within 2 weeks. Among the abundant species, Trichogramma sp. and Telenomus sp., which parasitize lepidopteran hosts, declined after spraying, and Trichogramma sp. recovered more quickly than Telenomus sp. Two other abundant species, Lymaenon litoralis and Oligosita sp., did not decline after spraying. In the sticky traps, wasp abundance increased at the vineyard edge but not center after spraying, suggesting that there was migration of wasps at the vineyard edge, into or out of the crop. The results indicate an effect of Indoxacarb on the parasitoid wasp community, particularly on parasitoids of lepidopterans, the target group of Indoxacarb. The results also indicate a potential for recovery of the parasitoid community through migration from neighboring natural vegetation.
1 Introduction
Conservation of biological control agents, such as parasitoid wasps, in agricultural areas can aid in integrated pest management schemes. However, to successfully integrate chemical and biological control it is necessary to determine the effects of pesticides on natural enemies and balance insecticide use with conservation of natural populations of predators and parasitoids (Janssen and van Rijn 2021). Parasitoid wasps in particular can serve as good indicators of effects of pesticides, since they are particularly sensitive to pesticides compared to other natural enemies (Mates et al., 2012).
Most tests of pesticide non-target effects are done in laboratory settings, focusing on the effect of a specific pesticide on a specific species of parasitic wasp. The ecological realism of such studies is often low. Laboratory experiments generally lack potential sheltered locations where wasps could avoid direct pesticide exposure and the wasps lack the ability to escape pesticides in space (Macfadyen et al., 2014). Also, the wasp populations tested may lack genetic variability which could be protective and may be affected by conditions of laboratory rearing (Macfadyen et al., 2014). On the other hand, abiotic stressors (such as suboptimal temperature or humidity), which may interact with pesticides to exacerbate parasitoid mortality, are usually eliminated from laboratory tests. Further, toxicology assays under laboratory conditions generally test one species at a time, and do not consider community-level effects of pesticides. Field studies can serve as a more realistic model of the effects of pesticides on entire parasitoid communities. For example, a study of the effects of Deltamethrin, a pyrethroid, in wheat fields indicated a decrease in density of wasps in the center of fields compared to edges, with recovery within 12 days (Longley et al., 1997). Another study of wheat fields sprayed with Dimethoate, an organophosphate, showed a decline in wasps of the genus Aphidius in sprayed and neighboring unsprayed areas of the fields, up to 20 days after insecticide application (Holland et al., 2000). In contrast, another wheat field study where Fenvalerate, a pyrethroid, and Dimethoate were sprayed found no effect on aphid parasitoids throughout the season following pesticide applications (Giller et al., 1995). An additional study in various field crops found negative effects of the insecticides Demeton-S-methyl, an organothiophosphate, and Cypermethrin, a pyrethroid, on abundance of aphid parasitizing wasps, and less so of the insecticide Pirimicarb, a carbamate (Smart et al., 1989). While these examples suggest some potential harmful side effects of pesticides to parasitoid assemblages, communities-level field studies remain few and scattered. In particular, how parasitoid communities recover after spraying remains poorly explored.
To address this gap, here we focus on Indoxacarb use in vineyards and its impacts on the resident parasitoid assemblages. Indoxacarb is an oxadiazine insecticide used against the European grapevine moth, Lobesia botrana Denis and Schiffermüller, 1775. It is applied as a spray to control lepidopterans at the larval stage and works by blocking voltage-gated slow-inactivated sodium channels, disrupting the nervous system (von Stein et al., 2013). It is one of the most commonly applied pesticides in vineyards (Nash et al., 2010). Laboratory experiments have been used to test the effects of Indoxacarb on specific parasitoid wasp species, and it has been shown to be harmful to Cotesia plutellae Kurdjumov, 1834, a larval endoparasitoid, in the adult stage (Haseeb et al., 2004), adult Trichogramma pretiosum Riley, 1879, an egg parasitoid (Scholz and Zalucki 2000), and Aphidius colemani Viereck, 1912 adults, which parasitize aphids (Bostanian and Akalach 2004). Fecundity and longevity of females were also reduced in C. plutellae and species in the genus Trichogramma (Haseeb et al., 2004; de Paiva et al., 2018; Gallego et al., 2019). Effects on emergence after insecticide application on immature stages varied among studies. There was a reduction in emergence of Trichogramma achaeae Nagaraja and Nagarkatti, 1970 (Gallego et al., 2019) and Trichogramma cacoeciae Marchal, 1927 from host eggs (Asma et al., 2018), but no effect on Trichogramma pretiosum (de Paiva et al., 2018) and Aphidius colemani emergence from eggs and aphid mummies, respectively (Bostanian and Akalach 2004). Altogether, the effect of Indoxacarb in laboratory experiments was highly dependent on the species and stage affected, and the dose the individual receives. Laboratory tests of Indoxacarb’s effects on predatory mites and bugs also yielded mixed results (Bostanian and Akalach 2006). The consequences of Indoxacarb applications for parasitoid communities under field conditions have not been evaluated yet.
One factor which may protect from harmful effects of pesticides could be the availability of field margins. A study testing for effects of pesticides on parasitism in field margins found none (Bakker et al., 2021), suggesting that the margins may serve as a source for recolonization of fields. The possibility of migration from non-sprayed habitats has been raised by several studies (Longley et al., 1997; Mates et al., 2012; Bakker et al., 2021), and this could allow recovery of parasitoid communities in agricultural habitats. However, it is necessary to examine the dynamics of the parasitoid community over space and time to further understanding of the effects of pesticides and mechanisms of recovery.
The aims of this study are to characterize dynamics of populations of common parasitoid wasps after insecticide application in vineyards and the distribution of parasitoid wasps in crop and non-crop habitat. Specifically, we tested the effect of Indoxacarb application on parasitoid abundance and diversity, and abundance of dominant species. We asked: 1) What is the effect in vineyard center, vineyard border, and natural habitat? 2) What is the effect in herbaceous and vine vegetation in the vineyard? 3) What is the effect over the course of 2 weeks after pesticide application? 4) Do wasps migrate between the vineyard and the natural habitat in response to insecticide application?
2 Methods
We sampled the parasitoid communities in wine-producing vineyards in Binyamina, Israel (32°30′14″N, 34°56′30″E-32°32′12″N, 34°57′24″E) during the 2019 and 2020 grape-growing seasons. The climate at the study area is Mediterranean, with cool rainy winters and hot dry summers. Pre-spray samples were taken 0–24 days (median 9 days) before Indoxacarb application. In the first year (2019), post-spray samples were taken twice or three times in the week after spraying, and in the second year (2020) samples were taken five to six times in the 2 weeks after spraying (Supplementary Table S1). For analysis, we chose sets of samples that were taken before and after application of Indoxacarb, without other insecticides, and with sampling for at least a week after spraying, with no other insecticides applied during that time. In 2019, five vineyards were sampled, one was sprayed twice, in mid-June and late July, and was included as two replicates. In 2020, four vineyards were included. Altogether, there were ten replicates across 2 years. Further information on the vineyards’ characteristics and on additional pesticides applied to them is provided in Supplementary Tables S1, S2.
Insects were sampled using vacuuming and sticky traps. A vacuum sampler (Vortis, United Kingdom) was passed through vegetation for 15 seconds. Sampling was done in ten locations at each vineyard: At the edge of the vineyard in the vine and groundcover herbaceous vegetation, 25 m into the vineyard, also in the vine and herb, and in a natural area near the vineyard, 10 m from the vineyard. Sampling was done in two such transects (Figure 1). The dominant species in the groundcover vegetation are listed in the Supplementary Table S3; most were summer flowering annuals, about half were weeds, and the most common family was Asteraceae. Sticky traps were hung at the edge and in the center of the vine at a height of ∼1.2 m (Figure 1), for two-three days at a time. Additionally, to explore effects of spraying at a greater distance from the vineyard, sampling was done at distances of 10–50 m from one vineyard, in 10 m intervals, in the second year.
Vacuum samples were stored in 75% ethanol. Parasitoid wasps were sorted out from each sample and identified to morphospecies, to obtain species richness per sample. The most abundant species were identified to genus or species. The keys used for identification of dominant species were Doutt and Viggiani 1968; Gibson et al., 1997; Goulet and Huber 1993; Huber et al., 2009; Masner 1980.
The effects of Indoxacarb spraying on parasitoid wasp abundance and species richness were analyzed in a generalized linear model with a Poisson distribution, including location of sample, vineyard, time in relation to spraying, year, and the interaction of location and time from spraying as factors. The abundances of the dominant species were also analyzed in generalized linear models with the same explanatory variables. We used two models for each species. The first model compared all vineyard locations to nature locations, and the second model included only vineyard locations with vegetation type and location in vineyard as factors. Thus, it was possible to determine what aspect of location affected populations. Both models also included year, time in relation to spraying, and interactions as factors. Wasp abundance per day in sticky traps was log transformed to fit a normal distribution and was analyzed in a general linear model with time in relation to spraying, location, year, and interactions as factors. The correlation between distance from the vineyard and wasp abundance, species richness, and Telenomus sp. abundance was tested for each date in relation to spraying, using a Pearson correlation. The analysis of the transect into the natural area focused on Telenomus sp. because the abundance of other species was low in this area.
To avoid confounding effects between date of sampling and time after spraying, samples included different vineyards which were sprayed at different dates. This might affect the results if there are seasonal trends in parasitoid abundance. To test for this possibility, we correlated the dates of pre-spraying sampling and the abundance of all wasps and of the dominant species using Spearman correlations. All analyses were done using IBM SPSS version 24 software.
3 Results
3.1 Overall Parasitoid Abundance and Species Richness
Wasp abundance declined during the first and second days after spraying in most vineyard locations except the vine at the border of vineyards, and gradually recovered over the next days (Figure 2A). In the natural area there was an initial increase in the first and second days after spraying, followed by large fluctuations in wasp abundance in the later post-spraying samples (Figure 2A). Species richness declined only in the inner vineyard vine during the first and second days after insecticide application, while increasing in the natural habitat (Figure 2B). There was also an interaction between location and time of sampling, so different locations within and around the vineyard responded differently to Indoxacarb application (Table 1). Specifically, abundance and richness were highest in nature, lower at the border of the vineyard in both the vine and herbaceous vegetation, and lowest in the inner vineyard locations in both vine and herbaceous vegetation (Figure 2). In the second year of the experiment, species richness and wasp abundance were lower than in the first year. Thus, there was an effect of time in relation to spraying and of location of sampling on wasp abundance and species richness, as well as an effect of vineyard and year (Table 1).
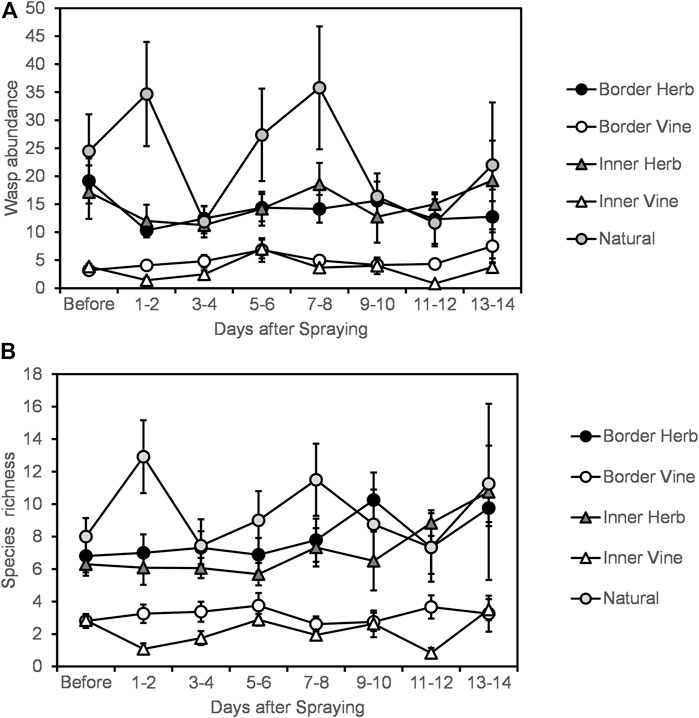
FIGURE 2. (A) Average per-sample parasitoid wasp abundance and (B) average per-sample species richness before and after Indoxacarb application in and near vineyard: border of vineyard (border) and 25 m into vineyard (inner), in the vine and herbaceous vegetation (herb), and in a natural area 10 m outside the vineyard. Error bars indicate standard errors.
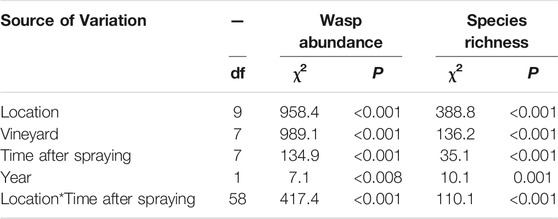
TABLE 1. Results of generalized linear model of factors affecting wasp abundance and species richness.
Sticky trap samples showed that wasp abundance increased at the border of the vineyard, but not in inner vineyard locations, one to 2 days after spraying (Figure 3). Location in vineyard alone, and time after spraying alone, did not affect wasp abundance; only the interaction of these two factors had an effect (Table 2). Also, the different locations in the vineyard had an interactive effect with year of sampling (Table 2). Namely, in the second year of sampling there was a greater difference in abundance between border and inner locations compared to the first year.
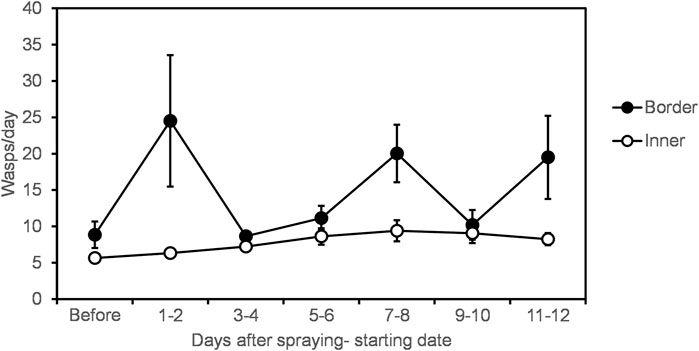
FIGURE 3. Average wasp abundance per day of trap placement in vineyard border and 25 m into vineyard, over time after Indoxacarb application. Data from both years were pooled. Error bars indicate standard error.
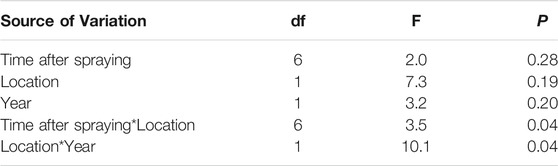
TABLE 2. Results of repeated measures general linear model of factors affecting abundance of wasps per day on sticky traps.
3.2 Dominant Parasitoid Species
Four dominant species made up 43% of parasitoid wasp assemblages in vacuum samples; Trichogramma Westwood, 1833 sp. (17% of all parasitoid wasps collected), Telenomus Haliday, 1833 sp. (10%), Lymaenon litoralis Haliday, 1833 (9%), and Oligosita Walker, 1851 sp. (7%). The following trends are reflected in the interaction between time after spraying and the vineyard/nature habitats: All species except Trichogramma sp. were more abundant in the natural area than in the vineyards. Trichogramma sp., which dominated the vineyards in 2019, declined in abundance after Indoxacarb application in this year (Figure 4A). Telenomus sp., which dominated the vineyards in 2020 and the natural areas in both years, declined for several days after spraying as well (Figures 4B–D). Both L. litoralis and Oligosita sp., mostly occupied the natural areas and did not show a clear decline though there were changes in population throughout the sampling period (Figure 4). All species except Telenomus sp. showed a different response to spraying in vineyard locations compared to natural areas (Table 3, top). Both Trichogramma sp. and Telenomus sp. declined after spraying in 2019, but Trichogramma sp. recovered more quickly (Figure 4). There was however a difference in response between years (Table 3, top), and Trichogramma sp. did not decline after spraying in 2020 (Figure 4). There was an increase in other dominant species as Telenomus sp. declined, particularly in the natural areas (Figures 4B,D). Within the vineyard, L. litoralis and Trichogramma sp. responded differently to spraying in different locations and vegetation types (Table 3 bottom). Both species declined most in herbaceous vegetation in 2019, but in 2020 increased after spraying. L. litoralis also showed a greater decline in inner vineyard locations than in edge locations. Telenomus sp. was consistently negatively affected, and Oligosita sp. showed no effect (Figure 4).
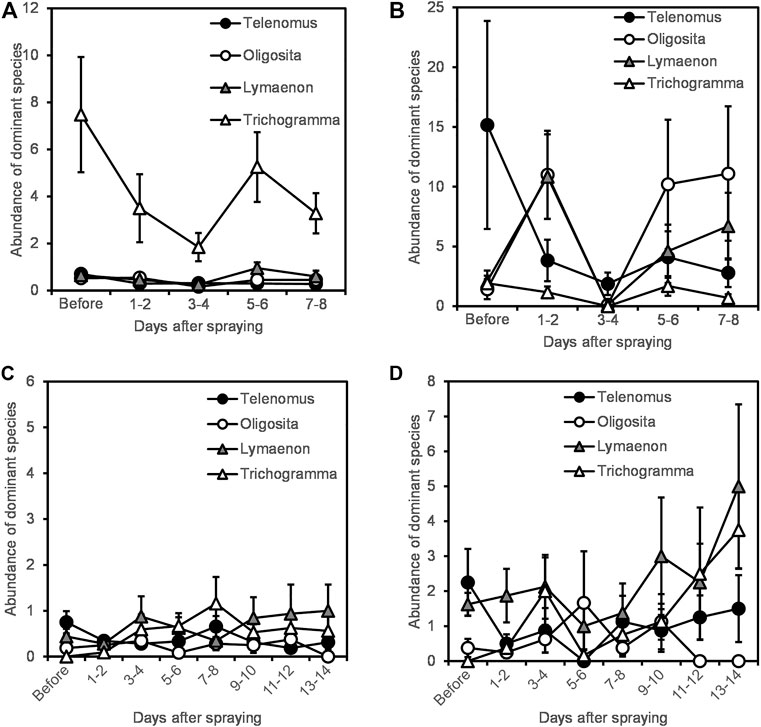
FIGURE 4. Abundance of the four dominant species in vineyard and natural areas in each of the 2 years of study. (A) Vineyard, 2019. (B) Nature, 2019. (C) Vineyard, 2020. (D) Nature, 2020. Note that we used different y-axis scales for 2019 and 2020 for visual clarity.
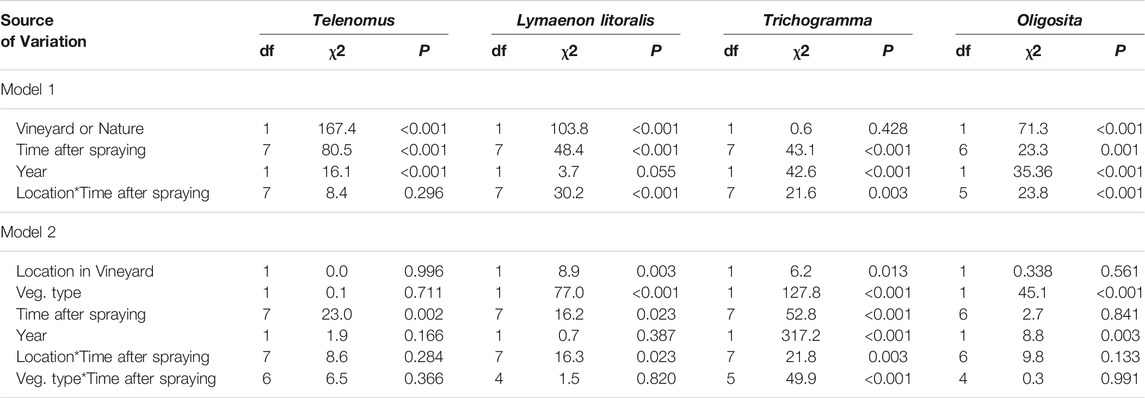
TABLE 3. Results of generalized linear models of factors affecting abundance of the four dominant wasp species. Model 1 included a comparison of all vineyard locations to natural locations and model 2 compared vegetation types and locations within the vineyard.
3.3 Natural Habitat at Increasing Distance From a Vineyard
Measurements of wasp abundance, species richness, and Telenomus sp. abundance in the natural area, up to 50 m from one of the vineyards, increased for a few days after spraying, and then declined again. However, there was no correlation between distance from the vineyard and these measures on any day after spraying or before spraying, except for wasp abundance which was positively correlated with distance from the vineyard on the third and fourth days after spraying (Figure 5; Table 4).
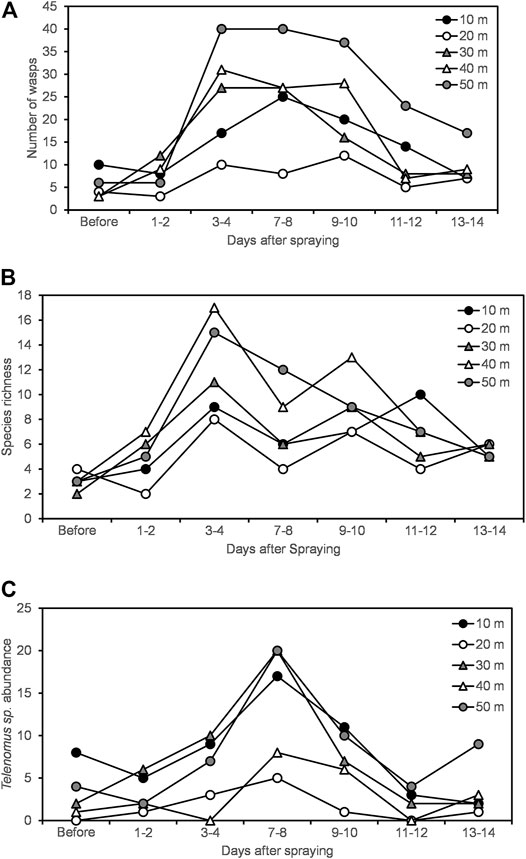
FIGURE 5. (A) Number of wasps (B) species richness (C) abundance of Telenomus sp. in a natural area near one of the vineyards at varying distances from the vineyard across time after spraying.
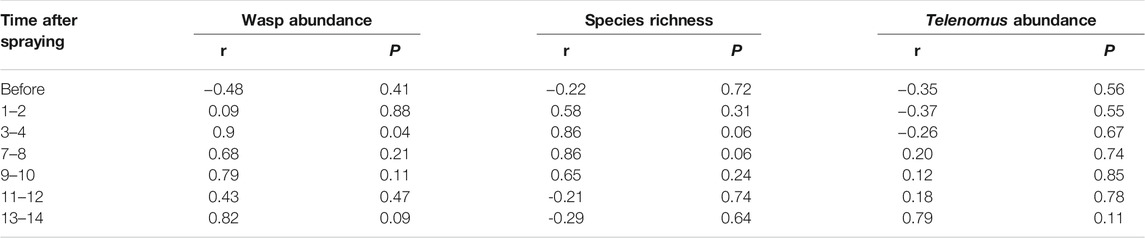
TABLE 4. Pearson correlation between wasp community measures and distance from the vineyard on different days since spraying. N = 5 (10, 20, 30, 40, 50 m from the vineyard’s edge) for each test.
In the test for correlation between pre-spraying date and parasitoid abundances, total abundance, Telenomus sp. and Oligosita sp. abundance were not correlated with date. L. litoralis and Trichogramma sp. abundance were negatively correlated with date (Supplementary Figure S1).
4 Discussion
Parasitoid richness and abundance were highest in natural habitat, higher in the herbaceous vegetation than in the vines, and higher in the vineyard margins than at their center (Figure 2), i.e., vineyard habitats that were close or similar in vegetation to the natural areas were richer in parasitoids. Similar patterns of parasitoid abundance and diversity were observed in previous surveys of vineyards in Israel’s Mediterranean region (Shapira et al., 2018), but not in desert vineyards (Segoli et al., 2020). The difference between the natural and the vineyard habitats became more marked in the first 2 days after Indoxacarb application, as parasitoid abundance and richness declined in the vineyards but not in the natural habitat. Yet, all areas in the vineyards also showed a recovery in total abundance and in species richness within 2 weeks. These findings may result from a combination of demographic processes: mortality and emigration of adult parasitoids due to insecticide exposure can lead to population declines. The subsequent recovery in their abundance may reflect the emergence of new adult cohorts within the vineyard and recolonization from nearby natural areas. A study of beetles, for example, found that recovery patterns differed among families, with some recovering from within fields and some from boundaries (Jepson and Thacker 1990). Our sampling design did not allow assessment of in-situ recovery within the vineyards. We did, however, find evidence for parasitoid migration out of the vineyard following Indoxacarb applications.
The increases in wasp capture on sticky traps suggest that there was migration to the natural habitats and subsequent migration back to the vineyard. The trend towards increasing abundance that was observed in the natural habitat transect in days 3–10, particularly at greater distances from the vineyard, provides additional suggestive evidence of migration to and recovery from the natural habitat. The distance of 50 m from the vineyard which was sampled represents a reasonable distance that the wasps could have traveled since a previous study has indicated that Anagrus erythroneurae Trjapitzin and Chiappini, 1994 wasps, which are similar in size to the common species in this study, were observed traveling 30 m in 6 days (Irvin et al., 2018). The mechanism driving adult wasp migration from the vineyard to natural habitats could be a repellent effect of the insecticide, which has been shown to increase parasitoid mobility (Desneux et al., 2007). These results provide support for the suggestion in previous studies that parasitoid recovery occurs from non-sprayed areas that contain potential habitat for parasitoids (Mates et al., 2012). Spatial analysis of recovery of predatory beetles after insecticide application has also shown gradual increase from unsprayed edges towards the sprayed center of fields over time (Duffield and Aebischer 1994). Further, in a study in corn fields, the presence of strips of natural vegetation reduced the negative effect of pesticide applications on predatory beetles (Lee et al., 2001). Since the vineyards in this study were small, the center of the vineyards was close to the margins, potentially allowing rapid recovery (Kattwinkel et al., 2015).
Heterogeneous vegetation within and around vineyards improves biodiversity and pest control (Paiola et al., 2020). Our study supports this idea by providing evidence that natural enemies migrate between vineyards and neighboring natural habitats after insecticide applications. Increased presence of natural habitat has been shown to increase biocontrol, though by predators and not parasitoids, when pesticide pressure was low, suggesting that if pesticides are sprayed more frequently, there is insufficient time for recovery from natural habitats (Ricci et al., 2019). Another study where low toxicity pesticides were used found no effect of agrochemical use, but a positive effect of plant diversity and complexity, on arthropod diversity, suggesting that the combination of low pesticide intensity and presence of appropriate habitat for arthropods promote higher arthropod diversity in agricultural habitats (Geldenhuys et al., 2021).
Of the four abundant species, Telenomus sp. and Trichogramma sp. were more affected by spraying than Oligosita sp. and Lymaenon litoralis. All the abundant species are egg parasitoids; Telenomus sp. parasitize Lepidoptera and Hemiptera, Trichogramma sp. parasitize Lepidoptera, Oligosita sp. parasitize Hemiptera and Thysanoptera, and Lymaenon litoralis parasitize Hempitera. Telenomus sp. and Trichogramma sp. may be most affected because their hosts are targeted by Indoxacarb, in addition to the toxic effect by contact on adult wasps that could be experienced by all species. The decline within the first 2 days in total wasp abundance suggests a direct toxic effect, while the decline in species parasitizing Lepidoptera suggests an indirect effect on the parasitoids via their hosts, if the insecticide is toxic to the moth eggs, which are parasitized by the wasps. On the other hand, egg parasitizing species may be protected by the chorion of their hosts while they develop and survive if they emerge after the effect of pesticide residue subsides (Loch 2005; Bueno et al., 2017). For Telenomus sp. and Trichogramma sp., emergence of adults from eggs that were parasitized before spraying would occur up to 8–13 days later (Navasero and Oatman 1989; Reda Abd el Monsef 2004), within the sampling time in the second year of the study.
Interestingly, Trichogramma sp. was among the most abundant species inside the vineyards, though this is a genus that has been shown to be relatively sensitive to pesticides (Theiling and Croft 1988), and specifically to Indoxacarb (Scholz and Zalucki 2000; Asma et al., 2018; de Paiva et al., 2018; Gallego et al., 2019; Madhusudan and Bhushanam 2020). It is, however, a genus that has been previously found in high densities inside vineyards, reflecting abundance of lepidopteran hosts (Reda Abd el Monsef 2004).
Our study documented the consequences of Indoxacarb applications that were administered by the farmers, rather than our own controlled manipulations of the spraying schedules. This observational approach did not allow us to set up Indoxacarb-free plots as matched controls to the sprayed vineyards. Untreated control plots are important for detecting seasonal population trends that are independent of the insecticide’s effects (Longley et al., 1997). For example, population declines that occur both in insecticide-treated plots and in their matched control plots should not be attributed to the insecticide, but instead to the species’ seasonal phenology. In our study, we reduced the potential confound between Indoxacarb-induced and seasonal population changes by sampling Indoxacarb-treated vineyards at various dates along the whole grape-growing season (Supplementary Table S1). In addition, we found no seasonal trend in the pre-spray total abundance of all parasitoids combined, suggesting that their post-spray decline reflects mortality associated with Indoxacarb exposure.
5 Conclusion
Overall, the dominant species in the natural and vineyard habitat, as well as overall parasitoid abundance and species richness appeared to recover within 2 weeks. The natural habitat present near the vineyards could provide a non-sprayed shelter for parasitoids repelled by the pesticide and could be a source to replace vineyard wasp communities. Thus, preservation of some natural habitat near vineyards, along with infrequent pesticide applications, could contribute to conservation of natural parasitoid communities, as shown here. In fact, a retrospective analysis of a large pest control dataset from vineyards found that natural habitats around the vineyards reduce pest outbreaks, as well as the need to apply chemical insecticides (Paredes et al., 2021). A further question to be explored would be the dynamics of the community outside the vineyard in space and time after spraying, and how the frequency of sprayings affects the community over an entire season.
Data Availability Statement
The datasets presented in this study can be found in online repositories. The names of the repository/repositories and accession number(s) can be found below: https://tamarkeasarlab.weebly.com/data-sets.html
Author Contributions
BS and TK planned the study. EG-R and TK provided funding and equipment. BS conducted field and laboratory work, analyzed the data and wrote the paper. All authors edited and revised the paper.
Funding
This research was supported by a post-doctoral fellowship to BS from the National Natural History Collections, The Hebrew University of Jerusalem and the Planning and Budgeting Committee of the Council for Higher Education in Israel (“VATAT”).
Conflict of Interest
The authors declare that the research was conducted in the absence of any commercial or financial relationships that could be construed as a potential conflict of interest.
Publisher’s Note
All claims expressed in this article are solely those of the authors and do not necessarily represent those of their affiliated organizations, or those of the publisher, the editors and the reviewers. Any product that may be evaluated in this article, or claim that may be made by its manufacturer, is not guaranteed or endorsed by the publisher.
Acknowledgments
We thank Nitzan Nachtom for assistance in field work and sample processing, Avi Goldstein, Ofer Lauer, Nir Altshuler, Yisrael Susnovik, and Sivan Harat for providing access to the vineyards and providing information about pesticide applications, and Miriam Kishinevsky and Gabriella Möller for assistance in parasitoid identification. The collected arthropods are housed at the the Margolin House Natural History collections at Oranim College and at the National Natural History Collections, The Hebrew University of Jerusalem.
Supplementary Material
The Supplementary Material for this article can be found online at: https://www.frontiersin.org/articles/10.3389/fenvs.2021.785669/full#supplementary-material
References
Asma, C., Ons, I., Sabrine, B.-A., and Kaouthar, L.-G. (2018). Life-stage-dependent Side Effects of Selected Insecticides on Trichogramma Cacoeciae (Marchal) (Hymenoptera: Trichogrammatidae) under Laboratory Conditions. Phytoparasitica 46, 105–113. doi:10.1007/s12600-018-0638-x
Bakker, L., van der Werf, W., and Bianchi, F. J. J. A. (2021). No Significant Effects of Insecticide Use Indicators and Landscape Variables on Biocontrol in Field Margins. Agric. Ecosyst. Environ. 308, 107253. doi:10.1016/j.agee.2020.107253
Bostanian, N. J., and Akalach, M. (2004). The Contact Toxicity of Indoxacarb and Five Other Insecticides toOrius Insidiosus (Hemiptera: Anthocoridae) andAphidius Colemani (Hymenoptera: Braconidae), Beneficials Used in the Greenhouse Industry. Pest Manag. Sci. 60, 1231–1236. doi:10.1002/ps.938
Bostanian, N. J., and Akalach, M. (2006). The Effect of Indoxacarb and Five Other Insecticides on Phytoseiulus Persimilis (Acari: Phytoseiidae), Amblyseius Fallacis (Acari: Phytoseiidae) and Nymphs of Orius Insidiosus (Hemiptera: Anthocoridae). Pest Manag. Sci. 62, 334–339. . doi:10.1002/ps.1171
Bueno, A. d. F., Carvalho, G. A., SantosdosSosa-Gómez, A. C. D. R., Sosa-Gómez, D. R., and Silva, D. M. d. (2017). Pesticide Selectivity to Natural Enemies: Challenges and Constraints for Research and Field Recommendation. Cienc. Rural 47. doi:10.1590/0103-8478cr20160829
de Paiva, A. C. R., Beloti, V. H., and Yamamoto, P. T. (2018). Sublethal Effects of Insecticides Used in Soybean on the Parasitoid Trichogramma Pretiosum. Ecotoxicology 27, 448–456. doi:10.1007/s10646-018-1909-5
Desneux, N., Decourtye, A., and Delpuech, J.-M. (2007). The Sublethal Effects of Pesticides on Beneficial Arthropods. Annu. Rev. Entomol. 52, 81–106. doi:10.1146/annurev.ento.52.110405.091440
Doutt, R. L., and Viggiani, G. (1968). The Classification of the Trichogrammatidae (Hymenoptera: Chalcidoidea). Proc. Calif. Acad. Sci. 35, 477–586.
Duffield, S. J., and Aebischer, N. J. (1994). The Effect of Spatial Scale of Treatment with Dimethoate on Invertebrate Population Recovery in winter Wheat. J. Appl. Ecol. 31, 263–281. doi:10.2307/2404542
Gallego, J. R., Guerrero-Manzano, J., Fernández-Maldonado, F. J., and Cabello, T. (2019). Susceptibility of the Egg Parasitoid Trichogramma Achaeae (Hymenoptera: Trichogrammatidae) to Selected Insecticides Used in Tomato Greenhouses. Span J. Agric. Res. 17, e1009. doi:10.5424/sjar/2019172-14413
Geldenhuys, M., Gaigher, R., Pryke, J. S., and Samways, M. J. (2021). Diverse Herbaceous Cover Crops Promote Vineyard Arthropod Diversity across Different Management Regimes. Agric. Ecosyst. Environ. 307, 107222. doi:10.1016/j.agee.2020.107222
Gibson, G., Huber, I., and Woolley, J. (Editors) (1997). Annotated Keys to the Genera of Nearctic Chalcidoidea (Hymenoptera). (Ottawa, Ontario, Canada: NRC Research Press).
Giller, P. S., Ryan, B., Kennedy, T., and Connery, J. (1995). Aphid-parasitoid Interactions in a winter Cereal Crop: Field Trials Involving Insecticide Application. J. Appl. Entomol. 119, 233–239. doi:10.1111/j.1439-0418.1995.tb01276.x
Goulet,, H., and Huber, J.T. (Editors) (1993). Hymenoptera of the World: An Identification Guide to Families. (Ottawa, Ontario, Canada: Agriculture Canada).
Haseeb, M., Liu, T.-X., and Jones, W. A. (2004). Effects of Selected Insecticides on Cotesia Plutellae, Endoparasitoid of Plutella Xylostella. BioControl 49, 33–46. doi:10.1023/B:BICO.0000009377.75941.d7
Holland, J. M., Winder, L., and Perry, J. N. (2000). The Impact of Dimethoate on the Spatial Distribution of Beneficial Arthropods in winter Wheat. Ann. Appl. Biol. 136, 93–105. doi:10.1111/j.1744-7348.2000.tb00013.x
Huber, J. T., Viggiani, G., and Jesu, R. (2009). “Order Hymenoptera, Family Mymaridae,” in Arthropod Fauna of the UAE. Editor A. V. Harten (Dar Al Ummah), 2, 270–297.
Irvin, N. A., Hagler, J. R., and Hoddle, M. S. (2018). Measuring Natural Enemy Dispersal from Cover Crops in a California Vineyard. Biol. Control. 126, 15–25. doi:10.1016/j.biocontrol.2018.07.008
Janssen, A., and Rijn, P. C. J. (2021). Pesticides Do not Significantly Reduce Arthropod Pest Densities in the Presence of Natural Enemies. Ecol. Lett. 24, 2010–2024. doi:10.1111/ele.13819
Jepson, P. C., and Thacker, J. R. M. (1990). Analysis of the spatial component of pesticide side-effects on non-target invertebrate populations and its relevance to hazard analysis. Functional Ecology 4, 349. doi:10.2307/2389596
Kattwinkel, M., Liess, M., Arena, M., Bopp, S., Streissl, F., and Römbke, J. (2015). Recovery of aquatic and terrestrial populations in the context of European pesticide risk assessment. Environ. Rev. 23, 382–394. doi:10.1139/er-2015-0013
Lee, J. C., Menalled, F. D., and Landis, D. A. (2001). Refuge habitats modify impact of insecticide disturbance on carabid beetle communities. J. Appl. Ecol. 38, 472–483. doi:10.1046/j.1365-2664.2001.00602.x
Loch, A. D. (2005). Mortality and recovery of eucalypt beetle pest and beneficial arthropod populations after commercial application of the insecticide alpha-cypermethrin. Forest Ecology and Management 217, 255–265. doi:10.1016/j.foreco.2005.06.006
Longley, M., Jepson, P. C., Izquierdo, J., and Sotherton, N. (1997). Temporal and spatial changes in aphid and parasitoid populations following applications of deltamethrin in winter wheat. Entomologia Experimentalis et Applicata 83, 41–52. doi:10.1023/A:100298670002110.1046/j.1570-7458.1997.00155.x
Macfadyen, S., Banks, J. E., Stark, J. D., and Davies, A. P. (2014). Using semifield studies to examine the effects of pesticides on mobile terrestrial invertebrates. Annu. Rev. Entomol. 59, 383–404. doi:10.1146/annurev-ento-011613-162109
Madhusudan, S., and Bhushanam, M. (2020). Evaluation of selected insecticides on adults of lepidopteran biocontrol egg parasitoids Trichogramma chilonis (Trichogrammatidae, Hymenoptera). J. Entomol. Zool. Stud. 8, 1037–1040.
Masner, L. (1980). Key to genera of Scelionidae of the Holarctic region, with descriptions of new genera and species (Hymenoptera: Proctotrupoidea). Mem. Entomol. Soc. Canada 113, 54. doi:10.4039/entm112113fv
Mates, S. G., Perfecto, I., and Badgley, C. (2012). Parasitoid wasp diversity in apple orchards along a pest-management gradient. Agriculture, Ecosystems & Environment 156, 82–88. doi:10.1016/j.agee.2012.04.016
Nash, M. A., Hoffmann, A. A., and Thomson, L. J. (2010). Identifying signature of chemical applications on indigenous and invasive nontarget arthropod communities in vineyards. Ecological Applications 20, 1693–1703. doi:10.1890/09-1065.1
Navasero, R. C., and Oatman, E. R. (1989). Life history, immature morphology and adult behavior ofTelenomus solitus [Hymenoptera: Scelionidae]. Entomophaga 34, 165–177. doi:10.1007/BF02372665
Paiola, A., Assandri, G., Brambilla, M., Zottini, M., Pedrini, P., and Nascimbene, J. (2020). Exploring the potential of vineyards for biodiversity conservation and delivery of biodiversity-mediated ecosystem services: A global-scale systematic review. Science of The Total Environment, 706, 135839.doi:10.1016/j.scitotenv.2019.135839
Paredes, D., Rosenheim, J. A., Chaplin‐Kramer, R., Winter, S., and Karp, D. S. (2021). Landscape simplification increases vineyard pest outbreaks and insecticide use. Ecology Letters 24, 73–83. doi:10.1111/ele.13622
Reda Abd el Monsef, A. I. (2004). Biological control of grape berry moths Eupoecilia ambiguella Hb. and Lobesia botrana Den. et Schiff. (Lepidoptera Tortricidae) by using egg parasitoids of the genus Trichogramma. Giesen: Thesis Univ, 1–103.
Ricci, B., Lavigne, C., Alignier, A., Aviron, S., Biju-Duval, L., Bouvier, J. C., Choisis, J.-P., Franck, P., Joannon, A., Ladet, S., Mezerette, F., Plantegenest, M., Savary, G., Thomas, C., Vialatte, A., and Petit, S. (2019). Local pesticide use intensity conditions landscape effects on biological pest control. Proc. R. Soc. B. 286, 20182898. doi:10.1098/rspb.2018.2898
Scholz, B., and Zalucki, M. (2000). “The effects of two new insecticides on the survival of adult Trichogramma pretiosum Riley in sweet corn,” in Hymenoptera: Evolution, Biodiversity and Biological Control. Editors A. D. Austin,, and M. Dowton (Collingwood VIC, Australia: CSIRO), 381–387.
Segoli, M., Kishinevsky, M., Rozenberg, T., and Hoffmann, I. (2020). Parasitoid abundance and community composition in desert vineyards and their adjacent natural habitats. Insects 11, 580. doi:10.3390/insects11090580
Shapira, I., Gavish-Regev, E., Sharon, R., Harari, A. R., Kishinevsky, M., and Keasar, T. (2018). Habitat use by crop pests and natural enemies in a Mediterranean vineyard agroecosystem. Agriculture, Ecosystems & Environment 267, 109–118. doi:10.1016/j.agee.2018.08.012
Smart, L. E., Stevenson, J. H., and Walters, J. H. H. (1989). Development of field trial methodology to assess short-term effects of pesticides on beneficial arthropods in arable crops. Crop Protection 8, 169–180. doi:10.1016/0261-2194(89)90023-9
Theiling, K. M., and Croft, B. A. (1988). Pesticide Side-Effects on Arthropod Natural Enemies: A Database Summary. Agric. Ecosyst. Environ. 21, 191–218. doi:10.1016/0167-8809(88)90088-6
Keywords: avaunt insecticide, conservation biological control, parasitoid wasp diversity, pesticides, vineyard
Citation: Schindler B, Gavish-Regev E and Keasar T (2022) Parasitoid Wasp Community Dynamics in Vineyards Following Insecticide Application. Front. Environ. Sci. 9:785669. doi: 10.3389/fenvs.2021.785669
Received: 29 September 2021; Accepted: 20 December 2021;
Published: 11 January 2022.
Edited by:
Christopher Williams, Liverpool John Moores University, United KingdomCopyright © 2022 Schindler, Gavish-Regev and Keasar. This is an open-access article distributed under the terms of the Creative Commons Attribution License (CC BY). The use, distribution or reproduction in other forums is permitted, provided the original author(s) and the copyright owner(s) are credited and that the original publication in this journal is cited, in accordance with accepted academic practice. No use, distribution or reproduction is permitted which does not comply with these terms.
*Correspondence: Tamar Keasar, dGtlYXNhckBnbWFpbC5jb20=