- 1Department of Agroecology, Aarhus University, Tjele, Denmark
- 2Aarhus University Centre for Circular Bioeconomy, Aarhus university, Tjele, Denmark
Quantifying soil organic carbon stocks (SOC) is a critical task in decision support related to climate and land management. Carbon inputs in soils are affected by development of belowground (BGB) and aboveground (AGB) biomass. However, uncertain fixed values of root:shoot ratios (R/S) are widely used for calculating SOC inputs in agroecosystems. In this study, we 1) assessed the effect of harvest frequency (zero, one, two, and five times annually) on the root and shoot development of the perennial grasses Phalaris arundinacea (RCG), Festuca arundinacea (TF), and Festulolium (FL); 2) determined the effect of management on the carbon and nitrogen content in AGB and BGB; and 3) assessed the implications of R/S for SOC quantification. We found the highest yields of BGB in zero-cut treatments with 59% (FL)–70% (RCG) of total biomass. AGB yield was highest in the five-cut treatments with 54% (RCG)–60% (FL), resulting in a decreasing R/S with frequent management, ranging from 1.6–2.3 (zero cut) to 0.6–0.8 (five cuts). No differences in R/S between species were observed. Total carbon yield ranged between 5.5 (FL, one cut) and 18.9 t ha−1 year−1 (FL, zero cut), with a higher carbon content in AGB (45%) than BGB (40%). We showed that the input of total organic carbon into soil was highest in the zero-cut treatments, ranging between 6.6 and 7.6 t C ha−1 year−1, although, in the context of agricultural management the two-cut treatments showed the highest potential for carbon input (3.4–5.4 t C ha−1 year−1). Our results highlighted that using default values for R/S resulted in inaccurate modeling estimations of the soil carbon input, as compared to a management-specific application of R/S. We conclude that an increasing number of annual cuts significantly lowered the R/S for all grasses. Given the critical role of BGB carbon input, our study highlights the need for comprehensive long-term experiments regarding the development of perennial grass root systems under AGB manipulation by harvest. In conclusion, we indicated the importance of using more accurate R/S for perennial grasses depending on management to avoid over- and underestimation of the carbon sink functioning of grassland ecosystems.
Introduction
Undisturbed mires are wetland biomes where peat accumulates, typically at rates of ∼1 mm year−1 over centuries (Parish et al., 2008), making these ecosystems one of the largest global organic carbon (C) reserves with substantial impact on atmospheric carbon dioxide (CO2) concentrations (Moomaw et al., 2018). In Denmark, wetlands with >6% organic C cover about 291,000 ha, of which 59% are used for agriculture (Greve et al., 2021). The massive losses of C from these agroecosystems are controlled by the balance between current net C inputs and peat mineralization, which is substantial and largely depends on the drainage conditions (Straková et al., 2012). National emission factors for drained organic agricultural soils in Denmark were established by empirical gas flux measurements in 2008–2009 and averaged 35 Mg CO2 ha−1 year−1 across eight sites in crop rotation and with permanent grass (Elsgaard et al., 2012). Climate change mitigation by rewetting of agricultural soils with >6% organic C is currently supported by national governmental incentives. Following this, it is envisaged that an area of 88,500 ha potentially can be rewetted and converted to permanent natural grassland (Ministry of Food, Agriculture and Fisheries of Denmark, 2021). Whereas reductions in CO2 emissions from slower peat mineralization are well documented in relation to increasing groundwater tables (Renou-Wilson et al., 2014), there is an unmet challenge in documenting the net C sequestration from new plant biomass on wet organic soils. This is in particular true for wetlands with >12% organic C and cultivated with perennial grasses, also known as paludiculture, which may contribute to greenhouse gas (GHG) mitigation (Tanneberger et al., 2020) and nutrient retention (Giannini et al., 2017; Vroom et al., 2018).
The input and cycling of organic C in soil ecosystems is highly affected by plant mechanisms regulating the development of aboveground (AGB) and belowground (BGB) biomass (i.e., shoots and roots, respectively) and consequently the quantity of litter input (Kumar et al., 2017), while decomposition of soil organic matter (SOM) is affected by soil nutrient stoichiometry (Kumar et al., 2021). Factors controlling AGB production of perennial grasses are well studied, but little is known about BGB, in particular for flood-tolerant perennial grasses. Roots play a significant role in the soil C cycle (Puget and Drinkwater, 2001; Moore et al., 2019; Dijkstra et al., 2020), indicate productivity (Thakur et al., 2021) and are crucial for the buildup of SOM on both mineral soils and peatlands (Klingenfuß et al., 2014; Leifeld et al., 2020). Not only root biomass but also in particular root exudates, secretions, lysates, cap cells, and mucilages (Carminati and Vetterlein, 2013; van Veelen et al., 2018) are important C inputs affecting the soil status of being either a source or a sink of C. For the estimation and modeling of changes in soil C stocks, a fixed default root:shoot ratio (R/S) is widely used to account for total biomass C. However, R/S is known to vary as a result of multiple environmental and climatic factors as well as management (Kibet et al., 2016; Sainju et al., 2017a; Hu et al., 2018). The optimal partitioning theory (OPT) of plant biomass allocation between AGB and BGB proposes that environmental factors will force plants to allocate new biomass to those parts needed to secure the most deficient resources for optimal plant growth (Fraser et al., 2015; Yang et al., 2018). In contrast, the isometric allocation hypothesis (IA) states that BGB scales linearly with AGB, independent of abiotic factors. Further, it has been stated that defoliation of AGB by harvest or grazing will decrease total BGB (Reid et al., 2015). However, due to the high on-site variability and the challenge of root extraction, in particular for perennial grasses, accurate estimations of R/S under different conditions are rare (Bolinder et al., 2002). Instead, and notably for grassland ecosystems, the allometric approach, using a fixed R/S (Bolinder et al., 2007), is used for modeling of BGB soil C inputs. Nevertheless, recent research highlighted the potential overestimation as well as uncertainty of this modeling approach (e.g., Mokany et al., 2006; Taghizadeh-Toosi et al., 2016; Keel et al., 2017).
While currently an effort is made to review R/S for different biomes and climate zones (e.g., Qi et al., 2019), an assessment of the R/S of grasses under different harvest frequencies is still lacking. This is particularly true for flood-tolerant grass species, which are increasingly introduced on both wetland and upland soils for both climate change mitigation and added-value products, such as grass protein as a substitute for soy (Nielsen et al., 2021). Hence, there is a need for consolidated estimates of R/S for commonly used paludiculture crops under different harvest frequencies (Karki et al., 2014). In the present study, we addressed this need in an annual trial and hypothesized that different R/S would be observed in flood-tolerant perennial grasses by manipulating the harvest frequency during the growth season under provision of adequate nutrient availability. The specific aims of the study were 1) to determine the effect of harvest frequency on the root and shoot development in the first year of cultivation of the perennial grasses reed canary grass (RCG; Phalaris arundinacea L.), tall fescue (TF; Festuca arundinacea Schreb.) and festulolium (FL; Festuca spp. × Lolium spp.), 2) to assess species-specific differences in R/S biomass ratios, 3) to determine the effect of harvest frequency on the C and nitrogen content in above- and belowground biomass, and 4) to assess the implications of R/S for soil C modeling.
Materials and Methods
Site Description and Experimental Design
The experiment was performed at the outdoor semi-field facilities of Aarhus University Foulum, Denmark. The average air temperature in the 8-month study period from March to November 2019, representing the annual growth period of grasses, ranged between 5.0°C and 16.8°C, with August as the warmest month. Monthly average precipitation ranged between 12 and 122 mm, with April as the driest and October as the wettest month. Global and net radiation was highest in June, with 20 and 8 MJ m−2, respectively (Figure 1).
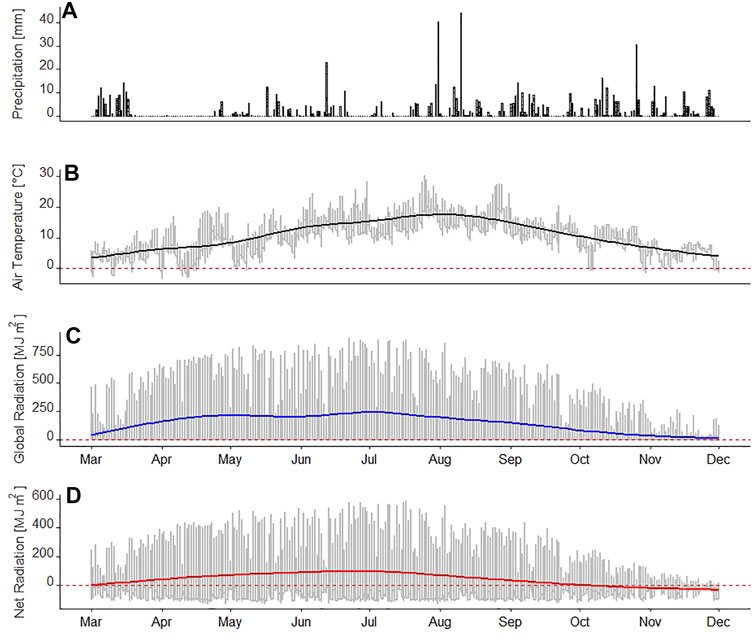
FIGURE 1. Environmental data for the year 2019 showing (A) precipitation (in mm), (B) temperature in Celsius, (C) global radiation (MJ m−2), and (D) net radiation (MJ m−2). Bold lines for temperature and radiation indicate the daily means, while dashed red lines indicate zero.
The perennial grasses RCG (cultivar: Lipaula), TF (cultivar: Kora), and FL (cultivar: Hykor) were grown in polyvinyl carbonate (PVC) cylinders (diameter 15 cm, depth 50 cm) that were placed in three trenches at the semi-field facility. The PVC cores were filled with coarse sandy soil (1.5% total organic C, 17.5 kg NH4-N ha−1, 35.0 kg NO3-N ha−1, pH 5.8) and maintained at a controlled water table depth (WTD) of ‐20 cm. This setup was chosen to simplify root washing as compared to peat soil where separation of new and old plant remains is unfeasible. The WTD control was ensured by placing the PVC tubes in tubs (78.5 cm × 48.5 cm × 30 cm) with the soil surface at ground elevation. The tubs allowed for overflow of excess water and were automatically filled twice daily with demineralized water to maintain a stable WTD (Figure 2). Sowing of seeds (25 kg ha−1) was performed by hand on March 14, 2019 (week 11). The cylinders (n = 20) in each cultivar group were randomly assigned to four harvest and fertilization treatments, including zero, one, two, and five annual cuts with five replicates each (Table 1). The treatment with one annual biomass harvest was chosen to determine BGB development in the first half of the growing period. Initial fertilization of all treatments was applied on March 19, 2019. The setup was exposed to natural changes in temperature and precipitation.
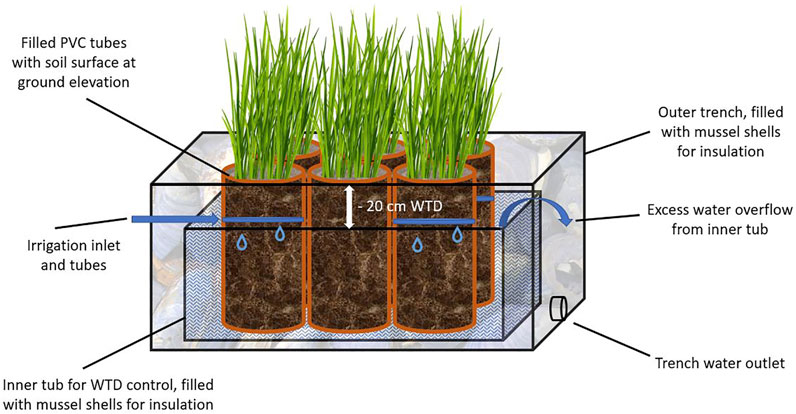
FIGURE 2. Schematic sketch of the experimental setup representing water table depth (WTD) control of the polyvinyl carbonate (PVC) cylinders, filled with soil and cultivated with the various grass species.

TABLE 1. Dates and amounts of fertilizer application, calendar weeks of aboveground biomass harvest occurrences, and dates for root extraction following the final biomass harvest for the various treatments as indicated by number of cuts.
Above and Belowground Biomass and Net Primary Productivity
AGB was harvested at a stubble height of 5 cm in calendar weeks 21, 25, 31, 37, and 44, depending on treatment regarding harvest frequency (Table 1). Stubble and BGB were separated and determined following the last AGB harvest. Roots were extracted from the soil by fine washing: two rinsing cycles using a soft spray nozzle with demineralized water and a 20-cm-diameter soil sieve with 2-mm mesh size, followed by three rinsing cycles and a 250-µm mesh size sieve. Total biomass dry matter (DM) for each cut and plant fraction was determined after oven-drying at 60°C to constant weight. Following drying, all samples were milled (Retsch SM 200, Retsch GmbH, Haan, Germany) and analyzed for total nitrogen (TN) and total organic carbon (TC) concentrations using a vario MAX CN (Elementar Analysesysteme GmbH, Hanau, Germany). Recovered roots were considered as BGB, and harvested yields and stubble were considered as AGB. Root:shoot ratios and NPP were calculated as
The calculation of NPP has been chosen to be simplified, in our study excluding the, unquantified contribution of, e.g., root secretions and exudates to NPP. The amount of C in AGB and BGB plant parts was calculated by multiplying the biomass (t DM) and the TC concentration in biomass (Mg C t−1 DM).
Extrapolation of Results for Calculation of Soil Carbon Input
We calculated the soil carbon input from biomass for each treatment under the following observations and assumptions of 1) observed yields of AGB and BGB, 2) the determined R/S, and 3) the various individual concentrations of TC in AGB and BGB as well as the stubble fraction of AGB. The method has been adapted from Kätterer et al. (2011) and Poeplau (2016) under the modification to account for specific TC concentrations in the stubble fraction, and BGB. Hence, we calculated the TC input into soil using the following assumptions and equations: ANPP is the aboveground NPP, which was calculated by multiplying the AGB yield by the carbon concentration in AGB as derived by biomass analyses for the various treatments (Eq. 3).
BNPP is the belowground NPP, calculated as the harvested AGB yield multiplied with the derived R/S for the various treatments and multiplied with the carbon concentration in BGB as derived by biomass analyses for the various treatments (Eq. 4).
ACin (t C ha−1 year−1), the TC input into the soil from AGB, was calculated as the yield of the not harvested stubbles multiplied by the carbon concentration is those, divided by two. This was a conservative estimate, based on the assumption that only approximately 50% of the stubble biomass (S) fraction becomes available as structural soil carbon input according to Schneider et al. (2006) (Eq. 5).
BCin, the TC input into soil (t C ha−1 year−1) for a depth of 50 cm, as equivalent to the length of the used PVC tubes, was calculated according to Equation 6. This is in detail described by Poeplau (2016), where d is the sampling depth (in cm), dr is the assumed maximum rooting depth for a flooded soil, and d50 is the depth of 50% of BNPP distribution. In our calculations, d was set to 50 cm as the depth of the PVC tubes, dr to 70 cm, since the maximum rooting depth under high WTDs is not likely to significantly exceed the sampling depth (Kohzu et al., 2003; Fan et al., 2017; D’Imperio et al., 2018), and d50 to 15 cm, according to average observations from this study across treatments. This was multiplied by 0.65 according to a conservative root turnover estimation for temperate wetlands with similar mean annual average temperature and precipitation values as our study site (Gill and Jackson, 2000; DuPont et al., 2014; Leifeld et al., 2015).
This resulted in the final TC input into soil (t C ha−1 year−1), which was calculated as the sum of ACin and BCin (Eq. 7).
Scenarios of Soil Carbon Input Based on Default R/S
Two commonly used R/S were applied for default calculation of soil carbon input: first, an R/S of 0.8 as stated by Bolinder et al. (2007) for grass species in eastern and western Canada. This ratio is based on a literature review on 35 publications. Second, we used the R/S of 2.8, which is derived from semi-arid grassland data, but used as a default expansion factor by the IPCC (2006) and applied in Denmark’s National Inventory Report (2020). These R/S values were applied to averaged total AGB yields and the commonly used average of 45% TC within biomass (Kätterer et al., 2011).
In addition, the carbon input into soil for RCG treatments was exemplarily calculated identical to the R/S scenarios, assuming identical biomass yields, for better comparability.
Statistical Analyses
Observations were averaged and summed up to yields over the entire growing period. Standard error was reported to present the distribution of data. Two-way analyses of variance were performed using linear mixed models with the function lmer of the package lme4 (Bates et al. (2015), Version 1.1–23, 2020) in the statistical software R (R Core Team (2020) Version 4.0.2—“Taking Off Again”), in which the following model was used:
where
Results
Root and Shoot Measurements
Cumulated Biomass Yield
Cumulated DM plant biomass at the end of the growing season ranged between 16.8 t ha−1 year−1 (FL, five cuts) and 46.2 t ha−1 year−1 (FL, zero cuts) across all treatments (Table 2) and were affected by the annual harvest strategy [χ2 (3) = 110.4, p < 0.001]. Generally, and for all species, the highest yields were found in the zero-cut treatments, ranging between 39.4 (TF) and 46.2 (FL) t ha−1 year−1. There was a consistent decrease of BGB and cumulative biomass yield with increasing number of annual cuts, with the one-cut treatment being an exception due to the different timing of harvest, presumably in combination with lesser N availability. However, while for RCG and TF there was no difference of total yields between the one-to five-cut treatments, there was, for FL, a significant (p < 0.001) increase of both AGB and BGB development, when comparing the one- and two-cut treatments. Species alone did not affect total biomass yield despite the observation of high overall yields for the FL two-cut treatment, close to three-fold as compared to the FL five-cut treatment. For all species, there was a significant difference (p < 0.001) between yields of the zero-cut treatment and all other treatments. Overall and across treatments, RCG and TF yields were near identical.
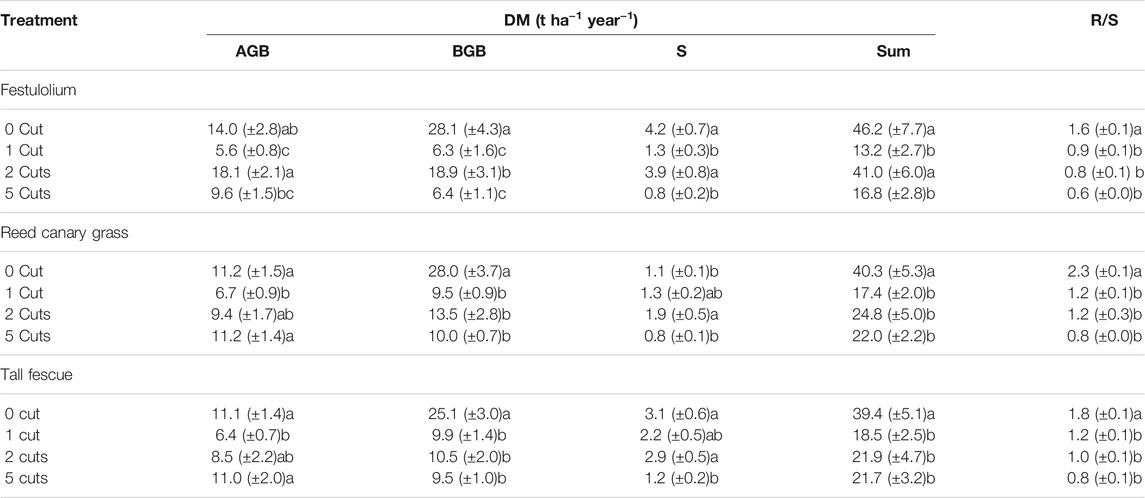
TABLE 2. Yields of dry matter (DM) of aboveground (AGB), belowground (BGB), and stubble (S) biomass for the various species and treatments. Total yields for above- and belowground plant fractions are indicated as sums. The root to shoot (R/S) ratio indicates the ratio of belowground to combined aboveground and stubble biomass. Letters indicate differences between treatments, where treatments with the same letters are not significantly different. Standard error is given in brackets (n = 5).
Root:shoot Ratio
The ratio between AGB and BGB (R/S) varied between 0.6 (FL, five cuts) to 2.3 (RCG, zero-cuts), significantly (p < 0.001) affected by the annual harvest strategy (Table 2). The smallest contribution of BGB to total biomass was observed for the five-cut treatments, ranging between 38% (FL) and 45% (RCG). In the zero-cut treatments, BGB contributed with 61% (FL) to 70% (RCG) of total biomass. For all species, the R/S of the zero-cut treatment was significantly (p < 0.001) higher than for the other treatments [χ2 (2) = 46.8, p < 0.001], while no differences in R/S were observed for the one-to five-cut treatments. There was no significant (p > 0.5) difference of R/S in between species across the various treatments. The differences between one and two annual cuts and between two and five annual cuts were non-significant (Figure 3). However, the Pearson correlation identified strong positive correlations between AGB and BGB based on yield results combined for treatment but differentiated for species (minimum R > 0.61), combined for species but differentiated for treatment (minimum R > 0.76), and differentiated for both species and treatment (minimum R > 0.71) (Figures 4A–C).
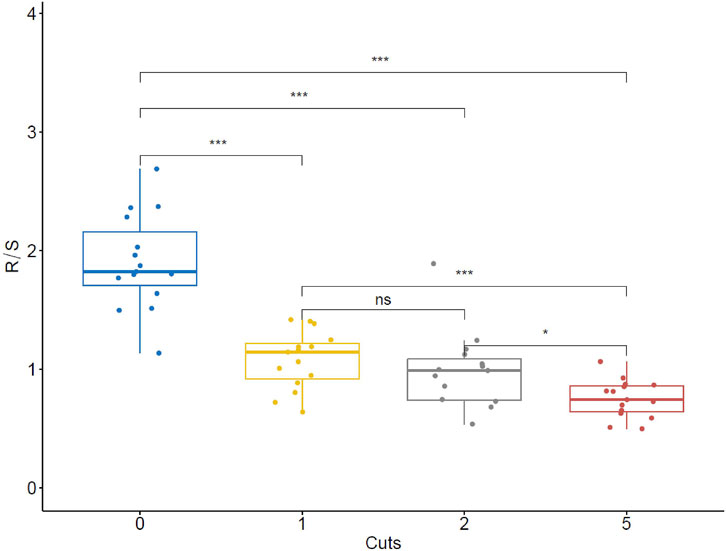
FIGURE 3. Differences of root:shoot ratios (R/S) for the various treatments of zero, one, two, and five annual cuts across species. Stars denote statistical significances between treatments according to p-values with ns indicating non-significance.
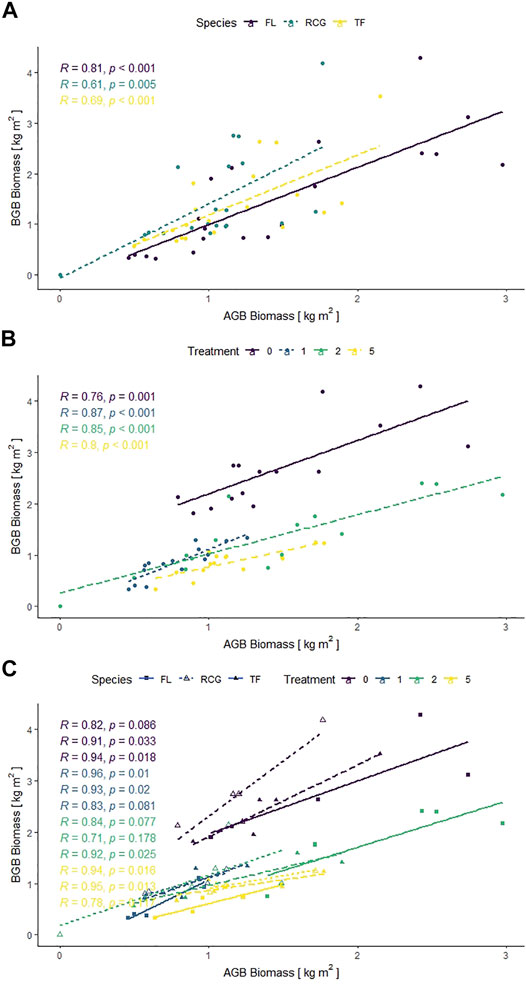
FIGURE 4. Pearson correlation showing the relation between aboveground (AGB) biomass (kg m−2) and belowground (BGB) biomass (kg m−2) for (A) species and treatment, (B) treatment across species, and (C) species across treatments.
Total Carbon
The mean content of TC across all species and treatments was 45% for aboveground grass biomass, 44% for stubble biomass, and 40% for belowground biomass. A decreasing trend of TC content within AGB was observed with increasing number of cuts for all species (Table 3). TC yield (t TC ha−1 year−1) within biomass generally followed the pattern of total DM biomass yield, with an increasing aboveground TC yield with increasing number of annual cuts and an increasing belowground TC yield with fewer annual cuts. The highest total plant TC yeild was found in the FL zero-cut treatment (18.9 t ha−1 year−1) and the lowest in FL one-cut (5.5 t ha−1 year−1). There were no significant differences for TC yields between the zero- and five-cut treatments in AGB. Generally, TC yield was highly affected by management [χ2 (15) = 80.9, p < 0.001].
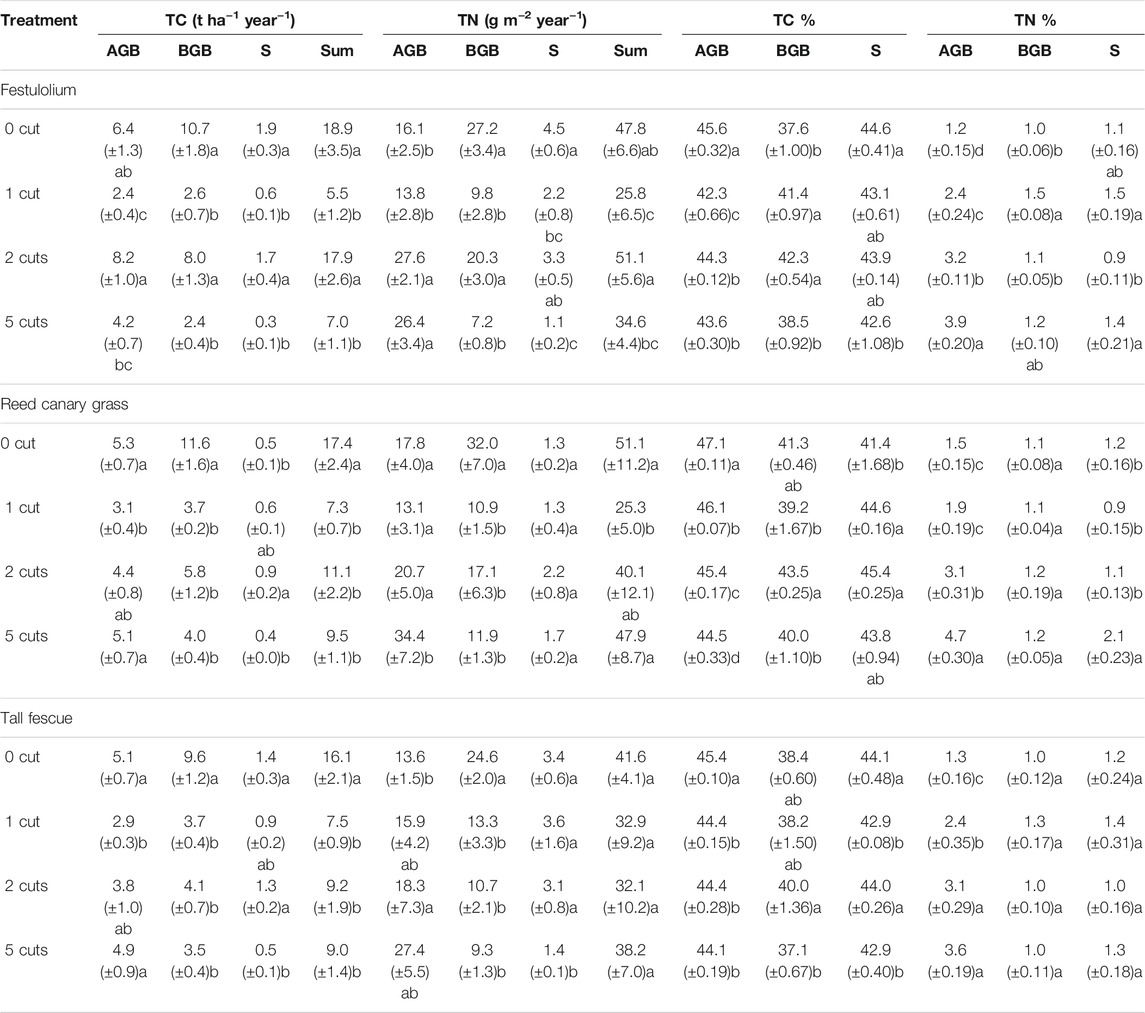
TABLE 3. Yields of total carbon (TC) and total nitrogen (TN), as well as TC and TN content in percentages, of aboveground (AGB), belowground (BGB), and stubble (S) biomass for the various species and treatments. Total yields for above- and belowground plant fractions are indicated as sums. Letters indicate differences between treatments, where treatments with the same letters are not significantly different. Standard error is given in brackets (n = 5).
Total Nitrogen
TN in biomass was significantly affected by treatment [χ2 (3) = 53.2, p < 0.001]. For all species and treatments, the content of TN was higher in AGB than in BGB and stubble biomass. Averaged across species, TN content in AGB increased from 1.4% in the zero-cut treatment to 4.0% in the five-cut treatments (Table 3). This is also depicted in TN yields, where more TN was harvested in the five-cut treatments (26.4–34.4 g N m−2 year−1) as compared to the zero-cut treatments (13.6–17.8 g N m−2 year−1), despite similar or lower AGB yields. In contrast to AGB, the TN content in BGB was not affected by increasing harvest and fertilization frequencies (p < 0.5). On a cumulative basis, the highest plant TN yield was found in the zero-cut treatment for RCG (51.1 g m−2 year−1) and TF (41.6 g m−2 year−1), while for FL, most TN (51.1 g m−2 year−1) was found in the two-cut treatment.
Carbon-to-Nitrogen Ratio
We found that for all three grass species, the carbon-to-nitrogen (C/N) ratio within AGB, as well as in the combined AGB and S (AGB + S) biomass, decreased significantly (p < 0.001) with increasing number of annual cuts. For instance, in AGB + S, the C/N decreased from 32.1 (RCG)–39.3 (FL) for the zero-cut treatment to 15.4 (RCG)–19.0 (TF) for the treatment with five annual cuts (Table 4). Regarding S biomass, the C/N was for all treatments higher as compared to the C/N of AGB, with significant (p < 0.001) differences for the one-to five-cut treatments. For BGB, no significant difference of the C/N ratio between the various treatments was observed, except for FL. However, cumulative across all plant parts, the C/N ratio followed the pattern of the C/N in AGB + S, showing significant (p < 0.001) differences between the treatments with zero and five annual cuts, with one- and two-cut treatments ranging in between.
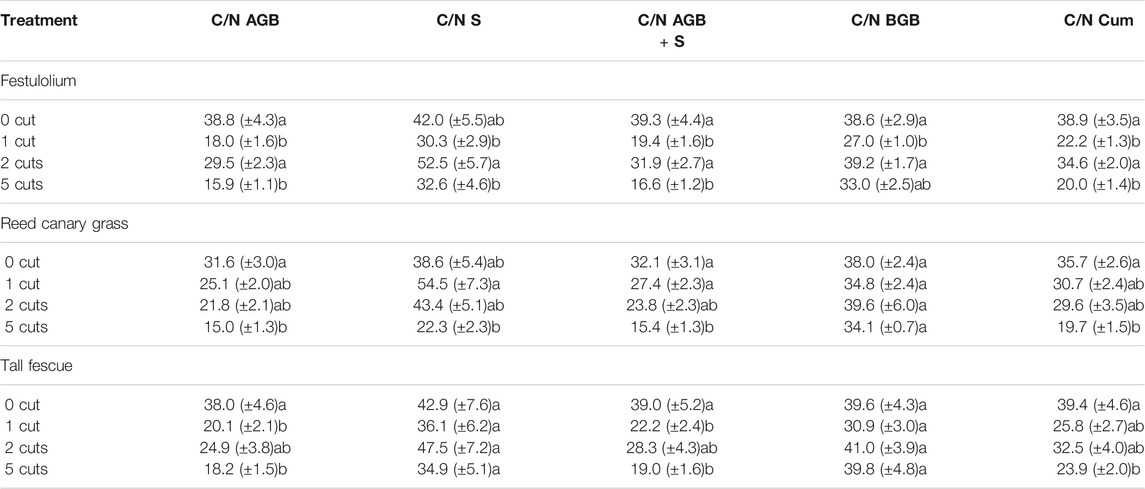
TABLE 4. Carbon-to-nitrogen (C/N) ratios in aboveground biomass (AGB), stubble biomass (S), combined aboveground and stubble biomass (AGB + S), and belowground biomass (BGB), as well as across all plant parts (cum) for the various treatments and species. Letters indicate differences between treatments, where treatments with the same letters are not significantly different.
Scenarios of Soil Carbon Input
The input of TC into soil was for all species highest in the zero-cut treatments, ranging between 6.6 t C ha−1 year−1 (TF) and 7.6 t C ha−1 year−1 (FL). A gradient of lesser TC input with increasing number of cuts was observed for all species, with the five-cut treatment being significantly lower than the treatment with zero harvests (Table 5). The one-cut treatment, harvested in August, was not significantly different to the five-cut treatment. Generally, TCin was significantly (p < 0.001) affected by the random effect of harvest and fertilization treatment. When theoretically assuming equal AGB yields for all treatments on the example of RCG and the two literature-derived R/S scenarios (Table 6), TCin ranged between 2.8 t C ha−1 year−1 (five cuts) and 7.3 t C ha−1 year−1 (zero cuts) for RCG. TCin using the R/S from Bolinder (2007) and the IPCC (2006) was 3.1 and 9.6 t C ha−1 year−1, respectively. For all treatments and scenarios, TCin was significantly affected by the R/S [X2 (1) = 56.4, p < 0.001].
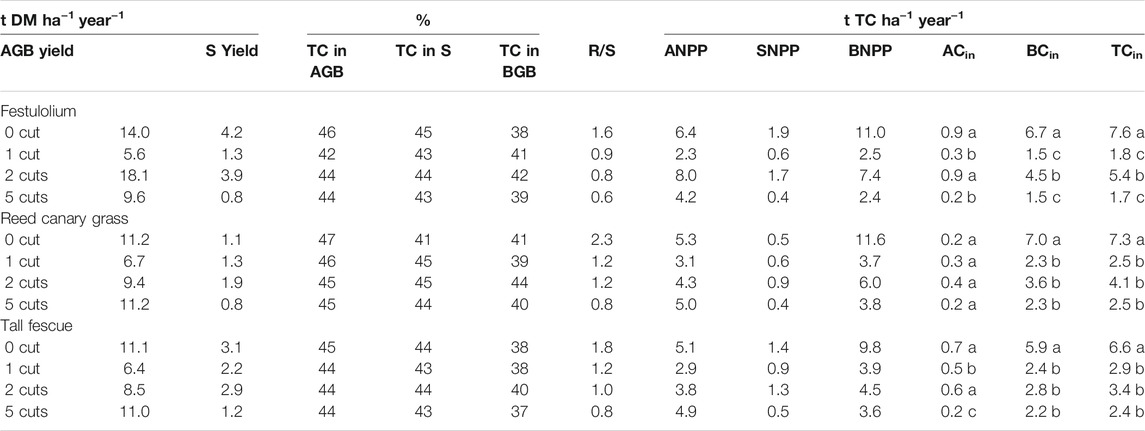
TABLE 5. Total annual harvested biomass yields in t dry matter (DM) ha−1 year−1 of the three perennial grasses under the various treatments, the content of total carbon (TC) in aboveground (AGB), stubble (S), and belowground (BGB) biomass and the determined root:shoot ratio (R/S), used for the calculation of aboveground net primary productivity (ANPP), stubble net primary productivity (SNPP), and belowground net primary productivity (BNPP), the input of carbon into soil from aboveground biomass residues (ACin) and belowground biomass (BCin), resulting in the total carbon input (TCin) over a rooting depth of 50 cm. Letters indicate differences between treatments, where treatments with the same letters are not significantly different.
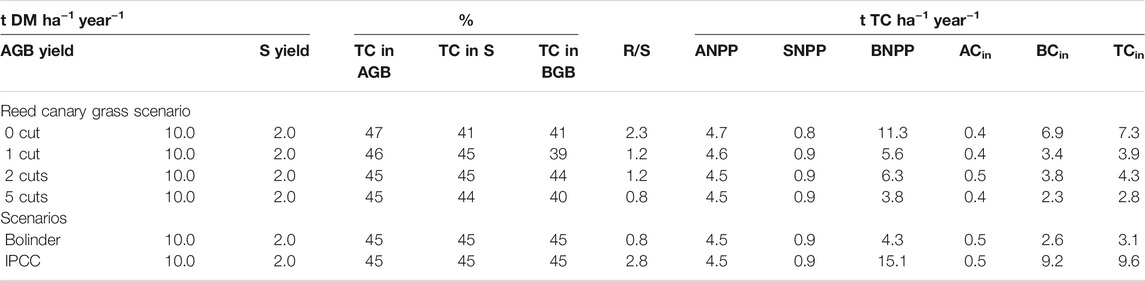
TABLE 6. Calculation of the total carbon input into soil using averaged biomass yields on the example of reed canary grass and two literature-derived scenarios, applying different root to shoot (R/S) ratios. The contents of total carbon (TC) in aboveground (AGB), stubble (S), and belowground (BGB) biomass and the determined R/S were used for the calculation of aboveground net primary productivity (ANPP), stubble net primary productivity (SNPP), and belowground net primary productivity (BNPP), the input of carbon into soil from aboveground biomass residues (ACin), and belowground biomass (BCin), resulting in the total carbon input (TCin) over a rooting depth of 50 cm. Yields are indicated in t dry matter (DM) ha−1 year−1.
Discussion
In this study, we highlight that AGB and BGB as well as R/S differed greatly among the various harvest frequencies, with frequent cuts resulting in reduced BGB yields and lower R/S for all assessed species. However, while the effects of water saturation and nutrient availability on biomass development and the R/S are relatively well known (e.g. Guo et al., 2016), there are only little comparable data available regarding R/S for RCG, TF, and FL under various annual cuts within the first year of establishment. Mander et al. (2012) reported an R/S of 0.91 (unfertilized) and 0.67 (fertilized) for RCG on an abandoned peat extraction site in Estonia without harvest, while Klimesová (1994) found a R/S of between 1.9 and 2.1 for RCG in a pot experiment under similar soil and water conditions and for the same timeframe as in this study. The latter values are similar to the R/S of 2.3 for the RCG treatment without harvest in our study. Bolinder et al. (2002) reported for RCG and TF in the second year after cultivation R/S values of 1.0 and 0.6 for a treatment with two annual cuts. These values, 0.2 and 0.4 lower than the corresponding R/S from RCG and TF under two annual cuts observed in our study, are within a similar range. However, the higher R/S observed in our study probably results from a younger sward age, indicating the plant’s need for optimal biomass allocation under the establishing growth period. Cougnon et al. (2013) reported 1 kg DM m−2 more BGB for TF with five annual cuts than in our experiment for an already established sward, receiving 100 kg N ha−1 and 100 kg K ha−1 more than in this study, and 300 (FL)–700 (TF) g DM m−2 more BGB in 3-year-old swards with five annual cuts, receiving similar fertilization amounts as in our study (Cougnon et al., 2017). We also found such differences in R/S for treatments without any harvest. For instance, Xiong et al. (2009) determined an R/S of 6.5 for RCG after a full year of growth, which differs significantly to our observed value of 2.3 after 210 days. Since the water table has been permanently controlled to –20 cm, a depth indicated as optimal for AGB development of flooding-tolerant perennial grasses (Miller and Zedler, 2003; Ustak et al., 2019), and adequate nutrients were provided, we interpret the observed differences in R/S for all species regarding harvest frequencies as a response of the plant’s biomass allocation. This is in accordance with the OPT (Kobe et al., 2010), where, as a consequence of more frequent harvest and removal of biomass involved in light energy capturing, more biomass is allocated to AGB organs in order to maximize photosynthesis. Further, the IA was supported by significant linear relationships between AGB and BGB, which is in line with other studies for temperate grasslands (e.g., Wang et al., 2010; Yang et al., 2018), indicating a coexistence of the OPT and IA theories. However, even though we were able to confirm our hypothesis, that different ratios between AGB and BGB will be observed in flood-tolerant perennial grasses under different harvest frequencies during the growth season under provision of adequate nutrient availability, there might remain a limitation of our observation for wet organic soils: that the R/S analysis was not performed on samples grown on peat soil cores. However, since R/S is rather affected by nutrient availability than soil types (Lambert et al., 2014; Pinno et al., 2014; Lehtonen et al., 2016), we assume a reliable validity of the indicated R/S for similar growth conditions, including fertilizer management, also on peat soils. This is supported by other research, comparing differences in R/S for certain species and treatments between cultivation on mineral and organic soil types. Xiong et al. (2009) for example found similar R/S for RCG under different fertilization rates on both mineral and organic soils. Björk et al. (2007) reported similar findings for root mass in Swedish heath and meadow tundra ecosystems, and Lambert et al. (2014) stated that R/S of Arundo donax was stable across soil types. Nonetheless, we propose that future research not only has to emphasize an assessment of cultivation treatments on R/S on mineral and organic soils but also needs to monitor TC and TN in AGB and BGB, as well as their development with increasing production years, due to expected variations (Sainju et al., 2017b). The C/N ratio can aid as an indicator for litter quality, with easier decomposable substrates having low C/N ratios (Rydin and Jeglum, 2013). Recalcitrant plant litter, indicated by high C/N ratios (Poirier et al., 2018), hence has the potential to increase soil C input-either directly to the pool of particulate organic matter (POM) or as microbial necromass following decomposition by microorganisms in the acrotelm (Worrall et al., 2017; Rossi et al., 2020). The potential for long-term C storage in the POM pool is in particular high for wet organic soils due to anoxic conditions in the catotelm, indicated by higher contents of lignin with increasing peat depth (Williams and Yavitt, 2003). Further, in soils with <12% SOC, additional C storage through the POM pool has the potential to overcome soil C saturation (Cotrufo et al., 2019). However, for drained soils where microbial activity is found in deeper layers, there is no consensus whether the C/N in BGB can be used as a predictor for the decomposability of root litter, and hence the C storage potential, as indicated by interspecific variation (Bonanomi et al., 2021). However, while R/S and C in biomass have been assessed for RCG on mineral and organic soils (Xiong et al., 2009; Xiong and Kätterer, 2010), this study was to our knowledge among the first to, besides R/S, also assess C/N in AGB and BGB parts.
The input of carbon into soil is a critical component of the global C cycle, thus significantly contributing to various aspects of ecosystem functioning. Modeling existing and changing soil organic carbon (SOC) stocks hence is a critical task in decision support related to optimal climate and land management (Taghizadeh-Toosi et al., 2016). However, while it is recognized that C allocation in belowground plant organs depends on vegetation type (Keller and Phillips, 2019), growing conditions (Whitehead, 2020), and plant development stages and management (Pausch and Kuzyakov, 2018), only few of these complex relationships (Cheng et al., 2014) are set into context with soil geochemistry and accounted for during SOC modeling (Finke et al., 2019). For instance, previously reported R/S for perennial grasses showed a broad distribution of median values, which has been shown in reviews by Bolinder et al. (2007) and Pausch and Kuzyakov (2018). Given the critical role of BGB carbon input, our study highlights the importance of acquiring more extensive knowledge regarding the development of perennial grass root systems depending on AGB manipulation by harvest. We only found marginally significant differences in R/S for the various treatments and species, apart for the, in agriculturally used grasslands, uncommon zero-cut strategy, which could indicate that a fixed R/S for perennial grasses might be reasonable to use if accurately defined.
However, our calculation of soil C input for perennial grasses under different harvest frequencies revealed significant differences for the various management options and applied R/S. This highlighted a potential risk for over- and underestimation of the C sink functioning of wetlands and grassland ecosystems. While, for instance, using the IPCC default R/S of 2.8 resulted in an estimated annual carbon input into soil of 9.6 t C ha−1 year−1 for a RCG yield of 10 t DM ha−1 year−1, our results ranged between 2.8 and 7.3 t C ha−1 year−1 for the same AGB yield, depending on annual harvest frequencies. Since, for instance, the default IPCC (2006) estimate of R/S of 2.8 is applied in the Danish National Inventory Report (2020), the discrepancy of TC input from BGB resulting from varying R/S might have far-reaching consequences for policymaking. For instance, depending on whether management measures are extensive, e.g., designated nature areas without any biomass manipulation, or intensive, e.g., biomass harvest up to five times annually, the choice of R/S for the quantification of an organic soil C sink function must be made carefully and adapted to the ecosystem in question. This is in particular true for the designation of rewetting measures on wetland areas, including the choice of land use and land cover, for climate considerations. In the context of optimum grassland management for agricultural production, we showed that a strategy with two annual cuts has the highest potential to contribute to SOC buildup. We hence advocate an inclusion of more accurate R/S for modeling, taking differences resulting from grass management, as well as site-specific climatic and biogeochemical conditions (Sahoo et al., 2021), into consideration to reduce uncertainties for policymaking on agroecosystems.
However, a limitation of this study is the assessment of harvest frequency on R/S during the establishment year only, which emphasizes the need for more long-term data on root growth of managed and unmanaged perennial grasses in wet environments. Hence, we suggest a future multi-annual study, with at least 2 years of trial, to increase the validity of results by accounting for the plants long-term response to management, taking interannual climatic variability into consideration. Previous studies demonstrated that R/S varies with ley age (Bolinder et al., 2002; Acharya et al., 2012; Huang et al., 2021), the associated interannual climatic variability (Poorter et al., 2012; Li et al., 2021), and nutrient availability (Cong et al., 2019); hence, further years of experimental analysis have to determine whether our findings regarding the effect of harvest frequency on R/S and the associated implications of management for SOC input are applicable on the long term. Further, it is yet unclear how the determined R/S for the assessed grasses can be applied in the evaluation of SOC input by plant biomass in wet or rewetted agricultural wetlands. For wet organic soils and in the context of paludiculture, we suggest that not only root growth but also rooting depth and specific root turnover rates have to be assessed. Only few studies assessed the maximum potential rooting depth by flood-tolerant perennial grasses in correlation with the WTD profile on organic soils, as compiled by Fan et al. (2017). Houde et al. (2020) highlighted the significance of turnover of perennial grass roots for the C storage potential, and Schwieger et al. (2020) emphasized the significance of roots as the main peat-forming component in grass-covered fen peatlands. However, while wetland ecosystems potentially have the second-highest root turnover rates of all ecosystems (Gill and Jackson, 2000), the complexity between rooting depth, WTD, C/N, and litter recalcitrance (Shurpali et al., 2010; Straková et al., 2012; Leifeld et al., 2015; D’Imperio et al., 2018) as well as AGB manipulation and root turnover rates for soil C input still needs to be defined.
With an increasing policy focus on wetland restoration, including paludiculture, for GHG mitigation and nutrient retention, as well as the concomitant need to point out sites with the highest mitigation potential, not only assessments of GHG emissions and optimum management (e.g., Geurts et al., 2019; Tanneberger et al., 2020; Nielsen et al., 2021) but also site- and plant community-specific BGB NPP should be taken into consideration for an accurate evaluation of the C sink potential.
Conclusion
While it is known that C inputs in soils are affected by development of AGB and BGB, as well as a variety of biotic and abiotic factors, little has been known so far on the effect of harvest frequency on the R/S of perennial grasses. In conclusion, this study found significant differences in the R/S for the flood-tolerant perennial grasses Phalaris ar., Festuca ar., and Festuca × Lolium, affected by annual harvest frequencies of AGB, with less biomass allocated to belowground parts with increasing number of cuts. No species-specific differences in the ratio were observed for any treatments. In addition, our results showed that both the OPT of plant biomass allocation and the IA hypothesis seemed to coexisted. Further, we demonstrated the importance to accurately define R/S for the calculation of carbon input into soils to avoid significant over- or underestimation. Our results showed that there are significant differences regarding the annual carbon input into soils, depending on the R/S applied. We found that using the IPCC default factor for R/S of 2.8, applied for both managed and unmanaged grasslands, resulted in 55%–71% higher carbon input rates as compared to our scenarios with two and five annual cuts, commonly applied in agricultural systems. This discrepancy indicates a significant inaccuracy for modeling and quantification of the C sink or source function of wet organic grassland areas, which might have far-reaching consequences for policymaking and carbon accounting. Further, we not only demonstrated how measurements of AGB and BGB provided a more accurate baseline for estimation of soil carbon input but also indicated the need for further assessment of R/S and C/N of perennial grasses, particularly for those cultivated on wet organic soils, to define the soil C sink capacity.
Data Availability Statement
The raw data supporting the conclusions of this article will be made available by the authors, without undue reservation.
Author Contributions
CN developed and performed the study design and experimental work, the analysis of the data, and the writing of the manuscript. All authors contributed to the study design and the writing and reading of the manuscript and approved the final manuscript.
Funding
This study was financially supported by the PEATWISE project (https://www.eragas.eu/en/eragas/Research-projects/PEATWISE.htm) in the frame of the ERA-NET FACCE ERA-GAS. FACCE ERA-GAS received funding from the European Union's Horizon 2020 research and innovation program under the grant agreement no. 696356. This publication further was funded by the Interreg project CANAPE under the North Sea Region Programme and the European Regional Development Fund. In addition, the study was partly supported by the Aarhus University Centre for Circular Bioeconomy (CBIO, https://cbio.au.dk/en/).
Conflict of Interest
The authors declare that the research was conducted in the absence of any commercial or financial relationships that could be construed as a potential conflict of interest.
Publisher’s Note
All claims expressed in this article are solely those of the authors and do not necessarily represent those of their affiliated organizations, or those of the publisher, the editors, and the reviewers. Any product that may be evaluated in this article, or claim that may be made by its manufacturer, is not guaranteed or endorsed by the publisher.
Acknowledgments
The authors want to thank Lars Elsgaard for valuable and highly appreciated comments to the manuscript during the stage of writing.
References
Acharya, B. S., Rasmussen, J., and Eriksen, J. (2012). Grassland Carbon Sequestration and Emissions Following Cultivation in a Mixed Crop Rotation. Agric. Ecosyst. Environ. 153, 33–39. doi:10.1016/j.agee.2012.03.001
Bates, D., Mächler, M., Bolker, B., and Walker, S. (2015). Fitting Linear Mixed-Effects Models Usinglme4. J. Stat. Soft. 67 (1), 1–48. doi:10.18637/jss.v067.i01
Björk, R. G., Majdi, H., Klemedtsson, L., Lewis‐Jonsson, L., and Molau, U. (2007). Long‐term Warming Effects on Root Morphology, Root Mass Distribution, and Microbial Activity in Two Dry Tundra Plant Communities in Northern Sweden. New Phytol. 176 (4), 862–873. doi:10.1111/j.1469-8137.2007.02231.x
Bolinder, M. A., Angers, D. A., Bélanger, G., Michaud, R., and Laverdière, M. R. (2002). Root Biomass and Shoot to Root Ratios of Perennial Forage Crops in Eastern Canada. Can. J. Plant Sci. 82 (4), 731–737. doi:10.4141/P01-139
Bolinder, M. A., Janzen, H. H., Gregorich, E. G., Angers, D. A., and VandenBygaart, A. J. (2007). An Approach for Estimating Net Primary Productivity and Annual Carbon Inputs to Soil for Common Agricultural Crops in Canada. Agric. Ecosyst. Environ. 118 (1), 29–42. doi:10.1016/j.agee.2006.05.013
Bonanomi, G., Idbella, M., Zotti, M., Santorufo, L., Motti, R., Maisto, G., et al. (2021). Decomposition and Temperature Sensitivity of fine Root and Leaf Litter of 43 Mediterranean Species. Plant Soil 464, 453–465. doi:10.1007/s11104-021-04974-1
Carminati, A., and Vetterlein, D. (2013). Plasticity of Rhizosphere Hydraulic Properties as a Key for Efficient Utilization of Scarce Resources. Ann. Bot. 112 (2), 277–290. doi:10.1093/aob/mcs262
Cheng, W., Parton, W. J., Gonzalez‐Meler, M. A., Phillips, R., Asao, S., McNickle, G. G., et al. (2014). Synthesis and Modeling Perspectives of Rhizosphere Priming. New Phytol. 201 (1), 31–44. doi:10.1111/nph.12440
Cong, W.-F., Christensen, B. T., and Eriksen, J. (2019). Soil Nutrient Levels Define Herbage Yield but Not Root Biomass in a Multispecies Grass-Legume Ley. Agric. Ecosyst. Environ. 276, 47–54. doi:10.1016/j.agee.2019.02.014
Cotrufo, M. F., Ranalli, M. G., Haddix, M. L., Six, J., and Lugato, E. (2019). Soil Carbon Storage Informed by Particulate and mineral-associated Organic Matter. Nat. Geosci. 12 (12), 989–994. doi:10.1038/s41561-019-0484-6
Cougnon, M., De Swaef, T., Lootens, P., Baert, J., De Frenne, P., Shahidi, R., et al. (2017). In Situ quantification of Forage Grass Root Biomass, Distribution and Diameter Classes under Two N Fertilisation Rates. Plant Soil 411 (1), 409–422. doi:10.1007/s11104-016-3034-7
Cougnon, M., Deru, J., Eekeren, N. v., Baert, J., and Reheul, D. (2013). “Root Depth and Biomass of Tall Fescue vs. Perennial Ryegrass,” in Paper Presented at the the Role of Grasslands in a green Future: Threats and Perspectives in Less Favoured Areas (Akureyri, Iceland: Proceedings of the 17th Symposium of the European Grassland Federation), 23–26.
Dijkstra, F. A., Zhu, B., and Cheng, W. (2020). Root Effects on Soil Organic Carbon: a Double‐edged Sword. New Phytol. 230, 60–65. doi:10.1111/nph.17082
D’Imperio, L., Arndal, M. F., Nielsen, C. S., Elberling, B., and Schmidt, I. K. (2018). Fast Responses of Root Dynamics to Increased Snow Deposition and Summer Air Temperature in an Arctic Wetland. Front. Plant Sci. 9 (1258). doi:10.3389/fpls.2018.01258
Dupont, S. T., Beniston, J., Glover, J. D., Hodson, A., Culman, S. W., Lal, R., et al. (2014). Root Traits and Soil Properties in Harvested Perennial Grassland, Annual Wheat, and Never-Tilled Annual Wheat. Plant Soil 381 (1-2), 405–420. doi:10.1007/s11104-014-2145-2
Elsgaard, L., Görres, C.-M., Hoffmann, C. C., Blicher-Mathiesen, G., Schelde, K., and Petersen, S. O. (2012). Net Ecosystem Exchange of CO2 and Carbon Balance for Eight Temperate Organic Soils under Agricultural Management. Agric. Ecosyst. Environ. 162, 52–67. doi:10.1016/j.agee.2012.09.001
Fan, Y., Miguez-Macho, G., Jobbágy, E. G., Jackson, R. B., and Otero-Casal, C. (2017). Hydrologic Regulation of Plant Rooting Depth. Proc. Natl. Acad. Sci. USA 114 (40), 10572–10577. doi:10.1073/pnas.1712381114
Finke, P., Opolot, E., Balesdent, J., Berhe, A. A., Boeckx, P., Cornu, S., et al. (2019). Can SOC Modelling Be Improved by Accounting for Pedogenesis. Geoderma 338, 513–524. doi:10.1016/j.geoderma.2018.10.018
Fraser, L. H., Pither, J., Jentsch, A., Sternberg, M., Zobel, M., Askarizadeh, D., et al. (2015). Worldwide Evidence of a Unimodal Relationship between Productivity and Plant Species Richness. Science 349 (6245), 302–305. doi:10.1126/science.aab3916
Geurts, J. J., van Duinen, G.-J. A., van Belle, J., Wichmann, S., Wichtmann, W., and Fritz, C. (2019). Recognize the High Potential of Paludiculture on Rewetted Peat Soils to Mitigate Climate Change. J. Sust. Org. Agric. Syst., 69(1), 5–8. doi:10.3220/LBF1576769203000
Giannini, V., Silvestri, N., Dragoni, F., Pistocchi, C., Sabbatini, T., and Bonari, E. (2017). Growth and Nutrient Uptake of Perennial Crops in a Paludicultural Approach in a Drained Mediterranean Peatland. Ecol. Eng. 103, 478–487. doi:10.1016/j.ecoleng.2015.11.049
Gill, R. A., and Jackson, R. B. (2000). Global Patterns of Root Turnover for Terrestrial Ecosystems. New Phytol. 147 (1), 13–31. doi:10.1046/j.1469-8137.2000.00681.x
Greve, M. H., Greve, M. B., Peng, Y., Pedersen, B. F., Møller, A. B., Lærke, P. E., et al. (2021). Vidensyntese Om Kulstofrig Lavbundsjord. Tjele, Denmark: Aarhus University, 137.
Guo, H., Xu, B., Wu, Y., Shi, F., Wu, C., and Wu, N. (2016). Allometric Partitioning Theory versus Optimal Partitioning Theory: The Adjustment of Biomass Allocation and Internal C-N Balance to Shading and Nitrogen Addition inFritillaria unibracteata(Liliaceae). Polish J. Ecol. 64 (2), 189–199. doi:10.3161/15052249pje2016.64.2.004
Houde, S., Thivierge, M.-N., Fort, F., Bélanger, G., Chantigny, M. H., Angers, D. A., et al. (2020). Root Growth and Turnover in Perennial Forages as Affected by Management Systems and Soil Depth. Plant Soil 451 (1), 371–387. doi:10.1007/s11104-020-04532-1
IPCC (2006). IPCC Guidelines for National Greenhouse Gas Inventories, Prepared by the National Greenhouse Gas Inventories Programme. Editors H. S. Eggleston, L. Buendia, K. Miwa, T. Ngara, and K. Tanabe. ISBN 4-88788-032-4.
Hu, T., Sørensen, P., Wahlström, E. M., Chirinda, N., Sharif, B., Li, X., et al. (2018). Root Biomass in Cereals, Catch Crops and Weeds Can Be Reliably Estimated without Considering Aboveground Biomass. Agric. Ecosyst. Environ. 251, 141–148. doi:10.1016/j.agee.2017.09.024
Huang, J., Liu, W., Yang, S., Yang, L., Peng, Z., Deng, M., et al. (2021). Plant Carbon Inputs through Shoot, Root, and Mycorrhizal Pathways Affect Soil Organic Carbon Turnover Differently. Soil Biol. Biochem. 160, 108322. doi:10.1016/j.soilbio.2021.108322
Karki, S., Elsgaard, L., Audet, J., Lærke, P. E., Audet, J., and Lærke, P. E. (2014). Mitigation of Greenhouse Gas Emissions from Reed Canary Grass in Paludiculture: Effect of Groundwater Level. Plant Soil 383 (1), 217–230. doi:10.1007/s11104-014-2164-z
Kätterer, T., Bolinder, M. A., Andrén, O., Kirchmann, H., and Menichetti, L. (2011). Roots Contribute More to Refractory Soil Organic Matter Than Above-Ground Crop Residues, as Revealed by a Long-Term Field experiment. Agric. Ecosyst. Environ. 141 (1-2), 184–192. doi:10.1016/j.agee.2011.02.029
Keel, S. G., Leifeld, J., Mayer, J., Taghizadeh-Toosi, A., and Olesen, J. E. (2017). Large Uncertainty in Soil Carbon Modelling Related to Method of Calculation of Plant Carbon Input in Agricultural Systems. Eur. J. Soil Sci. 68 (6), 953–963. doi:10.1111/ejss.12454
Keller, A. B., and Phillips, R. P. (2019). Relationship between Belowground Carbon Allocation and Nitrogen Uptake in Saplings Varies by Plant Mycorrhizal Type. Front. For. Glob. Change 2 (81), 81. doi:10.3389/ffgc.2019.00081
Kibet, L. C., Blanco-Canqui, H., Mitchell, R. B., and Schacht, W. H. (2016). Root Biomass and Soil Carbon Response to Growing Perennial Grasses for Bioenergy. Energ Sustain. Soc. 6 (1), 1. doi:10.1186/s13705-015-0065-5
Klimešová, J. (1994). The Effects of Timing and Duration of Floods on Growth of Young Plants of Phalaris Arundinacea L. And Urtica Dioica L.: an Experimental Study. Aquat. Bot. 48 (1), 21–29. doi:10.1016/0304-3770(94)90071-X
Klingenfuß, C., Roßkopf, N., Walter, J., Heller, C., and Zeitz, J. (2014). Soil Organic Matter to Soil Organic Carbon Ratios of Peatland Soil Substrates. Geoderma 235-236, 410–417. doi:10.1016/j.geoderma.2014.07.010
Kobe, R. K., Iyer, M., and Walters, M. B. (2010). Optimal Partitioning Theory Revisited: Nonstructural Carbohydrates Dominate Root Mass Responses to Nitrogen. Ecology 91 (1), 166–179. doi:10.1890/09-0027.1
Kohzu, A., Matsui, K., Yamada, T., Sugimoto, A., and Fujita, N. (2003). Significance of Rooting Depth in Mire Plants: Evidence from Natural 15 N Abundance. Ecol. Res. 18 (3), 257–266. doi:10.1046/j.1440-1703.2003.00552.x
Kumar, A., Kumar, M., Pandey, R., ZhiGuo, Y., and Cabral-Pinto, M. (2021). Forest Soil Nutrient Stocks along Altitudinal Range of Uttarakhand Himalayas: An Aid to Nature Based Climate Solutions. CATENA 207, 105667. doi:10.1016/j.catena.2021.105667
Kumar, A., Sharma, M. P., and Taxak, A. K. (2017). Effect of Vegetation Communities and Altitudes on the Soil Organic Carbon Stock in Kotli Bhel-1A Catchment, India. Clean. - Soil Air Water 45 (8), 1600650. doi:10.1002/clen.201600650
Lambert, A. M., Dudley, T. L., and Robbins, J. (2014). Nutrient Enrichment and Soil Conditions Drive Productivity in the Large-Statured Invasive Grass Arundo donax. Aquat. Bot. 112, 16–22. doi:10.1016/j.aquabot.2013.07.004
Lehtonen, A., Palviainen, M., Ojanen, P., Kalliokoski, T., Nöjd, P., Kukkola, M., et al. (2016). Modelling fine Root Biomass of Boreal Tree Stands Using Site and Stand Variables. For. Ecol. Manag. 359, 361–369. doi:10.1016/j.foreco.2015.06.023
Leifeld, J., Klein, K., and Wüst-Galley, C. (2020). Soil Organic Matter Stoichiometry as Indicator for Peatland Degradation. Sci. Rep. 10 (1), 7634. doi:10.1038/s41598-020-64275-y
Leifeld, J., Meyer, S., Budge, K., Sebastia, M. T., Zimmermann, M., and Fuhrer, J. (2015). Turnover of Grassland Roots in Mountain Ecosystems Revealed by Their Radiocarbon Signature: Role of Temperature and Management. PLOS ONE 10 (3), e0119184. doi:10.1371/journal.pone.0119184
Li, J., Pan, P., Wang, C.-t., Hu, L., Chen, K.-y., and Yang, W.-g. (2021). Root Dynamics of Artificial Grassland for Swards of Differing Ages in the ‘Three-River Source’region. Acta Prataculturae Sinica 30 (3), 28. doi:10.11686/cyxb2020161
Mander, Ü., Järveoja, J., Maddison, M., Soosaar, K., Aavola, R., Ostonen, I., et al. (2012). Reed Canary Grass Cultivation Mitigates Greenhouse Gas Emissions from Abandoned Peat Extraction Areas. Glob. Change Biol. Bioenergy 4 (4), 462–474. doi:10.1111/j.1757-1707.2011.01138.x
Miller, R. C., and Zedler, J. B. (2003). Responses of Native and Invasive Wetland Plants to Hydroperiod and Water Depth. Plant Ecol. 167 (1), 57–69. doi:10.1023/A:1023918619073
Ministry of Food, Agriculture and Fisheries of Denmark (2021). Overblik over arealkategorier vedr. udtagning af kulstofrige landbrugsjorder. MOF Alm. del Bilag 514. Available at: https://www.ft.dk/samling/20201/almdel/MOF/bilag/514/2382777.pdf (accessed 0707, , 2021).
Mokany, K., Raison, R. J., and Prokushkin, A. S. (2006). Critical Analysis of Root : Shoot Ratios in Terrestrial Biomes. Glob. Change Biol. 12 (1), 84–96. doi:10.1111/j.1365-2486.2005.001043.x
Moomaw, W. R., Chmura, G. L., Davies, G. T., Finlayson, C. M., Middleton, B. A., Natali, S. M., et al. (2018). Wetlands in a Changing Climate: Science, Policy and Management. Wetlands 38 (2), 183–205. doi:10.1007/s13157-018-1023-8
Moore, J. A. M., Sulman, B. N., Mayes, M. A., Patterson, C. M., and Classen, A. T. (2020). Plant Roots Stimulate the Decomposition of Complex, but Not Simple, Soil Carbon. Funct. Ecol. 34 (4), 899–910. doi:10.1111/1365-2435.13510
Nielsen, C. K., Stødkilde, L., Jørgensen, U., and Lærke, P. E. (2021). Effects of Harvest and Fertilization Frequency on Protein Yield and Extractability from Flood-Tolerant Perennial Grasses Cultivated on a Fen Peatland. Front. Environ. Sci. 9 (47), 258. doi:10.3389/fenvs.2021.619258
Denmark’s National Inventory Report, Nielsen, O.-K., Plejdrup, M. S., Winther, M., Nielsen, M., Gyldenkærne, S., Mikkelsen, M. H., et al. (2020). Denmark's National Inventory Report 2020. Emission Inventories 1990-2018-Submitted under the United Nations Framework Convention on Climate Change and the Kyoto Protocol. Aarhus University, DCE –Danish Centre for Environment and Energy, 900pp. Scientific Report No. 372, available at: http://dce2.au.dk/pub/SR372.pdf (assessed 07 07, 2021).
Parish, F., Sirin, A., Charman, D., Joosten, H., Minaeva, T. Y., and Silvius, M. (2008). Assessment on Peatlands, Biodiversity and Climate Change: Main Report. Wageningen: Global Environment Centre, Kuala Lumpur and Wetlands International. ISBN 978-983-43751-0-2.
Pausch, J., and Kuzyakov, Y. (2018). Carbon Input by Roots into the Soil: Quantification of Rhizodeposition from Root to Ecosystem Scale. Glob. Change Biol. 24 (1), 1–12. doi:10.1111/gcb.13850
Pinno, B. D., Landhäusser, S. M., Chow, P. S., Quideau, S. A., and MacKenzie, M. D. (2014). Nutrient Uptake and Growth of Fireweed (Chamerion Angustifolium) on Reclamation Soils. Can. J. For. Res. 44 (1), 1–7. doi:10.1139/cjfr-2013-0091
Poeplau, C. (2016). Estimating Root: Shoot Ratio and Soil Carbon Inputs in Temperate Grasslands with the RothC Model. Plant Soil 407 (1), 293–305. doi:10.1007/s11104-016-3017-8
Poirier, V., Roumet, C., and Munson, A. D. (2018). The Root of the Matter: Linking Root Traits and Soil Organic Matter Stabilization Processes. Soil Biol. Biochem. 120, 246–259. doi:10.1016/j.soilbio.2018.02.016
Poorter, H., Niklas, K. J., Reich, P. B., Oleksyn, J., Poot, P., and Mommer, L. (2012). Biomass Allocation to Leaves, Stems and Roots: Meta‐analyses of Interspecific Variation and Environmental Control. New Phytol. 193 (1), 30–50. doi:10.1111/j.1469-8137.2011.03952.x
Puget, P., and Drinkwater, L. E. (2001). Short‐Term Dynamics of Root‐ and Shoot‐Derived Carbon from a Leguminous Green Manure. Soil Sci. Soc. Am. J. 65 (3), 771–779. doi:10.2136/sssaj2001.653771x
Qi, Y., Wei, W., Chen, C., and Chen, L. (2019). Plant Root-Shoot Biomass Allocation over Diverse Biomes: A Global Synthesis. Glob. Ecol. Conservation 18, e00606. doi:10.1016/j.gecco.2019.e00606
R Core Team (2020). R: A Language and Environment for Statistical Computing. Vienna, Austria: R Foundation for Statistical Computing. Available at: https://www.R-project.org/.
Reid, J. B., Gray, R. A. J., Springett, J. A., and Crush, J. R. (2015). Root Turnover in Pasture Species: Chicory, lucerne, Perennial Ryegrass and white clover. Ann. Appl. Biol. 167 (3), 327–342. doi:10.1111/aab.12228
Renou-Wilson, F., Barry, C., Müller, C., and Wilson, D. (2014). The Impacts of Drainage, Nutrient Status and Management Practice on the Full Carbon Balance of Grasslands on Organic Soils in a Maritime Temperate Zone. Biogeosciences 11 (16), 4361–4379. doi:10.5194/bg-11-4361-2014
Rossi, L. M. W., Mao, Z., Merino-Martín, L., Roumet, C., Fort, F., Taugourdeau, O., et al. (2020). Pathways to Persistence: Plant Root Traits Alter Carbon Accumulation in Different Soil Carbon Pools. Plant Soil 452 (1-2), 457–478. doi:10.1007/s11104-020-04469-5
Rydin, H., Jeglum, J. K., and Bennett, K. D. (2013). The Biology of Peatlands. 2e. Oxford University Press. doi:10.1093/acprof:osobl/9780199602995.001.0001
Sahoo, U. K., Tripathi, O. P., Nath, A. J., Deb, S., Das, D. J., Gupta, A., et al. (2021). Quantifying Tree Diversity, Carbon Stocks, and Sequestration Potential for Diverse Land Uses in Northeast India. Front. Environ. Sci. 9, 724950. doi:10.3389/fenvs.2021.724950
Sainju, U. M., Allen, B. L., Lenssen, A. W., and Ghimire, R. P. (2017a). Root Biomass, Root/shoot Ratio, and Soil Water Content under Perennial Grasses with Different Nitrogen Rates. Field Crops Res. 210, 183–191. doi:10.1016/j.fcr.2017.05.029
Sainju, U. M., Allen, B. L., Lenssen, A. W., and Mikha, M. (2017b). Root and Soil Total Carbon and Nitrogen under Bioenergy Perennial Grasses with Various Nitrogen Rates. Biomass and Bioenergy 107, 326–334. doi:10.1016/j.biombioe.2017.10.021
Schneider, M. K., Lüscher, A., Frossard, E., and Nösberger, J. (2006). An Overlooked Carbon Source for Grassland Soils: Loss of Structural Carbon from Stubble in Response to Elevated pCO 2 and Nitrogen Supply. New Phytol. 172 (1), 117–126. doi:10.1111/j.1469-8137.2006.01796.x
Schwieger, S., Kreyling, J., Couwenberg, J., Smiljanić, M., Weigel, R., Wilmking, M., et al. (2020). Wetter Is Better: Rewetting of Minerotrophic Peatlands Increases Plant Production and Moves Them towards Carbon Sinks in a Dry Year. Ecosystems 24, 1093–1109. doi:10.1007/s10021-020-00570-z
Shurpali, N. J., Strandman, H., Kilpeläinen, A., Huttunen, J., Hyvönen, N., Biasi, C., et al. (2010). Atmospheric Impact of Bioenergy Based on Perennial Crop (Reed Canary Grass, Phalaris Arundinaceae, L.) Cultivation on a Drained Boreal Organic Soil. Gcb Bioenergy 2 (3), no. doi:10.1111/j.1757-1707.2010.01048.x
Straková, P., Penttilä, T., Laine, J., and Laiho, R. (2012). Disentangling Direct and Indirect Effects of Water Table Drawdown on above- and Belowground Plant Litter Decomposition: Consequences for Accumulation of Organic Matter in Boreal Peatlands. Glob. Change Biol. 18 (1), 322–335. doi:10.1111/j.1365-2486.2011.02503.x
Taghizadeh-Toosi, A., Christensen, B. T., Glendining, M., and Olesen, J. E. (2016). Consolidating Soil Carbon Turnover Models by Improved Estimates of Belowground Carbon Input. Sci. Rep. 6 (1), 32568. doi:10.1038/srep32568
Tanneberger, F., Schröder, C., Hohlbein, M., Lenschow, U., Permien, T., Wichmann, S., et al. (2020). Climate Change Mitigation through Land Use on Rewetted Peatlands - Cross-Sectoral Spatial Planning for Paludiculture in Northeast Germany. Wetlands 40 (6), 2309–2320. doi:10.1007/s13157-020-01310-8
Thakur, T. K., Patel, D. K., Thakur, A., Kumar, A., Bijalwan, A., Bhat, J. A., et al. (2021). Biomass Production Assessment in a Protected Area of Dry Tropical forest Ecosystem of India: A Field to Satellite Observation Approach. Front. Environ. Sci. 9, 757976. doi:10.3389/fenvs.2021.757976
Usťak, S., Šinko, J., and Muňoz, J. (2019). Reed Canary Grass (Phalaris Arundinacea L.) as a Promising Energy Crop. Jcea 20 (4), 1143–1168. doi:10.5513/JCEA01/20.4.2267
van Veelen, A., Tourell, M. C., Koebernick, N., Pileio, G., and Roose, T. (2018). Correlative Visualization of Root Mucilage Degradation Using X-ray CT and MRI. Front. Environ. Sci. 6, 32. doi:10.3389/fenvs.2018.00032
Vroom, R. J. E., Xie, F., Geurts, J. J. M., Chojnowska, A., Smolders, A. J. P., Lamers, L. P. M., et al. (2018). Typha Latifolia Paludiculture Effectively Improves Water Quality and Reduces Greenhouse Gas Emissions in Rewetted Peatlands. Ecol. Eng. 124, 88–98. doi:10.1016/j.ecoleng.2018.09.008
Wang, L., Niu, K., Yang, Y., and Zhou, P. (2010). Patterns of above- and Belowground Biomass Allocation in China's Grasslands: Evidence from Individual-Level Observations. Sci. China Life Sci. 53 (7), 851–857. doi:10.1007/s11427-010-4027-z
Whitehead, D. (2020). Management of Grazed Landscapes to Increase Soil Carbon Stocks in Temperate, Dryland Grasslands. Front. Sustain. Food Syst. 4, 913. doi:10.3389/fsufs.2020.585913
Williams, C. J., and Yavitt, J. B. (2003). Botanical Composition of Peat and Degree of Peat Decomposition in Three Temperate Peatlands. Écoscience 10 (1), 85–95. doi:10.1080/11956860.2003.11682755
Worrall, F., Moody, C. S., Clay, G. D., Burt, T. P., and Rose, R. (2017). The Flux of Organic Matter through a Peatland Ecosystem: The Role of Cellulose, Lignin, and Their Control of the Ecosystem Oxidation State. J. Geophys. Res. Biogeosci. 122 (7), 1655–1671. doi:10.1002/2016JG003697
Xiong, S., and Kätterer, T. (2010). Carbon-allocation Dynamics in Reed Canary Grass as Affected by Soil Type and Fertilization Rates in Northern Sweden. Acta Agriculturae Scand. Section B - Soil Plant Sci. 60 (1), 24–32. doi:10.1080/09064710802558518
Xiong, S., Landström, S., and Olsson, R. (2009). Delayed Harvest of Reed Canary Grass Translocates More Nutrients in Rhizomes. Acta Agriculturae Scand. Section B - Soil Plant Sci. 59 (4), 306–316. doi:10.1080/09064710802154722
Keywords: root:shoot ratio, perennial grass, peatland, soil organic carbon, paludiculture, wetland, carbon sink
Citation: Nielsen CK, Jørgensen U and Lærke PE (2021) Root-To-Shoot Ratios of Flood-Tolerant Perennial Grasses Depend on Harvest and Fertilization Management: Implications for Quantification of Soil Carbon Input. Front. Environ. Sci. 9:785531. doi: 10.3389/fenvs.2021.785531
Received: 30 September 2021; Accepted: 27 October 2021;
Published: 22 November 2021.
Edited by:
Munesh Kumar, Hemwati Nandan Bahuguna Garhwal University, IndiaReviewed by:
Amit Kumar, Nanjing University of Information Science and Technology, ChinaCalogero Schillaci, Joint Research Centre, Italy
Copyright © 2021 Nielsen, Jørgensen and Lærke. This is an open-access article distributed under the terms of the Creative Commons Attribution License (CC BY). The use, distribution or reproduction in other forums is permitted, provided the original author(s) and the copyright owner(s) are credited and that the original publication in this journal is cited, in accordance with accepted academic practice. No use, distribution or reproduction is permitted which does not comply with these terms.
*Correspondence: Claudia Kalla Nielsen, Y2xhdWRpYUBhZ3JvLmF1LmRr