- College of Fisheries, Guangdong Ocean University, Zhanjiang, China
The alcohol industry discharges large quantities of wastewater, which is hazardous and has a considerable pollution potential. Cultivating microalgae in wastewater is an alternative way of overcoming the current high cost of microalgae cultivation and an environmentally friendly treatment method for industrial effluents. The study analyzed the growth and biochemical composition of Chlorella vulgaris cultivated in membrane-treated distillery wastewater (MTDW) and nutrients removal efficiency. The results showed biomass productivity of 0.04 g L−1 d−1 for MTDW with the contents of content of protein, carbohydrate, and lipid at 49.6 ± 1.4%, 26.1 ± 0.6%, and 10.4 ± 1.8%, respectively. The removal efficiencies of TN, TP, and COD were 80, 94, and 72.24% in MTDW, respectively. In addition, removal efficiencies of 100, 85.37, and 42.86% for Ca2+, Mg2+, and Mo2− were achieved, respectively. The study added to our growing knowledge on the cultivation of Chlorella with wastewater, suggesting that it was feasible to cultivate Chlorella with MTDW and represented an economical and environmentally friendly strategy for microalgae biomass production and reuse of wastewater resources.
Introduction
Industrial wastewater is one of the main sources of pollution of the water environment, and its production has a serious negative impact on the ecosystem and human life. Therefore, the fight against wastewater has become a major issue in terms of health, environment, and economy (Abdel-Raouf et al., 2012). The quality and quantity of industrial wastewater vary according to the type of industry. The metal processing industry emits chromium, nickel, zinc, cadmium, lead, iron, and titanium compounds. The printing plant releases inks and dyes (Hanchang, 2009). Wastewater from paper mills contains chloride organics and dioxins, as well as suspended solids and organic wastes (Lindholm-Lehto et al., 2015). The petrochemical industry discharges a large number of phenols and mineral oil (Éverton et al., 2018). The content of suspended solids and organic matter in wastewater from food processing plants is very high (Qasim and Mane, 2013). In contrast, distilleries produce large amounts of acidic, stubborn, and colored wastewater with high organic content (Sanjay and Jamaluddin, 2018), which may lead to the destruction of the aquatic environment causing eutrophication, affecting human health and recreational activities (Sanjay and Jamaluddin, 2018; Stutter et al., 2018; Thoré et al., 2021). The alcohol industry discharges up to 0.3 billion m3 of high-concentration wastewater each year in China (Guo et al., 2006), which has become the second largest source of organic pollution. Cassava alcohol wastewater is widely produced in cassava-based bioethanol industries (Quan et al., 2014). In general, about 12 tons of wastewater would be generated to produce 1 ton of cassava ethanol (Lin et al., 2016). Industrial wastewater with high organic and acidic substances many contain as high as 40,000∼130,000 mg L−1 chemical oxygen demand (COD) concentration (Gang et al., 2008).
The source of industrial wastewater comes with different characteristics. Hence, the treatment of industrial wastewater should be specifically designed for specific wastewater. Recently, several technologies such as photocatalysis (Al-Mamun et al., 2019), electrodialysis (Deng et al., 2020; Liu et al., 2020), iron-oxides-doped granular activated carbon catalyst (Deng et al., 2021), and Fe/C galvanic cells strengthened A2O process (Fe/C-A2O) (Peng et al., 2020) have been developed with significant effects for industrial wastewater treatment. Presently, distilleries use a multi-stage strategy in wastewater treatment, including pretreatment, secondary treatment, and tertiary treatment. Pretreatment reduces temperature of the wastewater as well as removes suspended solids of large particles in the wastewater (Yang and Wyman, 2007), while an anaerobic wastewater treatment system removed most of the organic matter in the wastewater (Sanjay and Jamaluddin, 2018). Finally, most of the N and P in the wastewater is removed through advanced treatment such as anaerobic and aerobic processes and membrane bioreactor (MBR) (Noor et al., 2013). However, the wastewater after membrane treatment still contains some amount of organic matter, nutrients, and other substances, which may be harmful to the environment. Conventional treatment methods are extensively used, they are however characterized by excessive use of chemicals, high operational and maintenance cost. These methods also generate huge amounts of sludge, thereby making conventional treatment methods environmentally and economically unfavorable (Amenorfenyo et al., 2020).
The microalgae-based wastewater treatment is an environmentally friendly wastewater treatment method, which is often used to treat secondary or tertiary wastewater and is considered one of the promising technologies for wastewater treatment. As a kind of eukaryotic green microalgae with strong photosynthesis ability, Chlorella is considered as one of the easily cultivated microalgae that contains high-quality protein, carotenoids, vitamins, and minerals, it has long been proposed as a healthy food substitute for humans and animals (Liu et al., 2013; Liu et al., 2014; Znad et al., 2018). The use of Chlorella for wastewater treatment and nutrient recovery reduces cost of wastewater treatment, and production of useful biomass (Sánchez-Zurano et al., 2021). In recent years, cultivation of microalgae especially Chlorella vulgaris in wastewater have attracted more and more attention. According to previous studies, Chlorella could remove organic contaminants, and heavy metals from as urban wastewater (Tercero et al., 2014), domestic wastewater (Aziz and Ng, 1992), textile wastewater (Chu et al., 2009), and piggery wastewater (Ji et al., 2012). However, little is known about cultivation of Chlorella in membrane-treated distillery wastewater (MTDW) for nutrient recovery and biomass production, and its feasibility.
In this study, we performed the biomass production of Chlorella coupled with the treatment of MTDW, which seeks to explore the nutrient removal efficiency, biomass production and productivity, and biochemical content of C. vulgaris grown in MTDW. The work sought to give useful information that will lead to the understanding of the cost-effective method of wastewater treatment and microalgae biomass production.
Materials and Methods
Collection and Pre-Inoculation of Microalgae
Freshwater algae C. vulgaris was acquired from the laboratory of Ecology of Water Area and Aquaculture Environment of Fisheries College, Guangdong Ocean University, South China. The vegetative cells were grown photoautotrophically at 2000 lx (white light). The 7-day-old algal cells were collected and inoculated into 2 L Erlenmeyer flasks filled with 1 L of BG11 medium at an initial optical density of 0.2 (OD680) in FDFF illuminated incubator 2000 lx (white light) and 25°C for 9 days.
Wastewater Collection and Analysis
The wastewater used in this study, MTDW was collected from SDIC Guangdong Bio-Energy Co., Ltd., Zhanjiang, South China. The wastewater sample was collected in a 5 L plastic container that was thoroughly pre-washed with the wastewater from the company. To reduce the decomposition of a substrate, MTDW sample was stored at 4°C before the wastewater characteristics analysis. Then wastewater was pretreated by means of filtration using a glass microfiber filter (934-AH, Whatman, United States) to remove turbidity and large particles. The filtered sample was autoclaved at 121°C for 30 min to eliminate bacteria and other algal growth inhibitors.
Determination of Dry Weight and Chlorophyll a
Microalgae biomass concentration were measured every 72 h. Dry weight (DW) was determined by filtering a 10 ml samples of the algal suspension through pre-weighed (m1) filters (47 mm, 1.2 μm, Whatman). Then drying the filters (105°C, overnight) to a constant weight and weighing with microbalance (m2). The DW (g L−1) was calculated with Eq. 1.
Biomass productivity (g L−1 day−1) was calculated with Eq. 2.
where DWi and DW0 represent the dry biomass (g L−1) at time ti and t0 (day).
The pigment contents of the microalgae thus chlorophyll a was analyzed after extraction in 95% ethanol (w/v). Briefly, 5 ml of the suspensions were filtered and freeze-dried at −20°C for 12 h; the dried biomass was suspended in ethanol for 4 h in dark. The suspensions were later centrifuged at 5,000 rpm for 10 min and the pigment contents of the supernatant were spectrophotometrically measured at 665 and 649 nm. The Eq. 3 was used to calculate the pigment contents (Hartmut and Alan, 1983).
Carbohydrate, Protein and Lipid Quantification
Protein extractions were determined according to the modified method described in Barbarino and Lourenço (2005) and Ge et al. (2018). As follows: weigh 30 mg powder of Chlorella, and then add 8 ml of distillery water. After soaking for 12 h, centrifuge at 15,000 rpm (4°C) for 20 min to collect the supernatant. Then use 2.0 ml 0.1 N (or 2 M) NaOH to re-extract the concentrated pellets. After centrifugation at 15,000 rpm (21°C) for 20 min, the supernatant was collected and mixed with the previous supernatant. 10 ml of the extract was taken to determine protein concentration with the aid of Bio-Rad DC protein as-say (Cat. 500-0111, Bio-Rad Laboratories, Hercules, United States). Anthrone colorimetric approach was used to assess carbohydrate and protein content of the supernatants using a Hach model DR 2800 spectrophotometer, glucose, and serum albumin were used as the standard for carbohydrate and protein.
Ge and Champagne (2016) methods were used to assess lipid content. Briefly, the microalgal suspension was harvested via centrifugation (4°C, 5000 rpm, 10 min), the bio-mass was washed twice with distillery water, and then oven-dried overnight at 60°C. A 0.1 g dry biomass of Chlorella was immersed in 3 ml of distillery water and vortexed at 3,000 rpm for 30 s, then placed in a water bath at 90°C for 20 min. Methanol/chloroform (extraction solution) of a proportion of 1:2 v/v was added after it attained room temperature. The lipids of sample were extracted overnight at room temperature after which, 1 ml of distillery water was added. The organic phase was collected by centrifugation (20°C, 10 min) and transferred into a pre-weighted dish. The chloroform was evaporated at 50°C, and then the extracted lipids were subjected to gravimetric analysis.
Nutrients Concentration and Removal Efficiency
All samples were filtered through filter paper (0.22 μm, Whatman) and analyzed for nutrients, TN, TP, and COD. Macro and Micronutrients were tested by Qingdao Sci-tech Innovation Quality Testing Co., Ltd. (China). Total Nitrogen (TN), Total phosphorus (TP), and COD were determined every 3 days starting from the day of inoculation. Persulfate digestion method and acid-persulfate digestion method were used for analyzing TN and TP, respectively. COD concentration was measured with a multi-functional water quality analyzer (LIANHUA, 5B-3B, China).
Nutrient removal efficiency (%) is calculated by the Eq. 4.
where C0 is the nutrient concentration of the influent and C1 is the nutrient concentration of the effluent.
FAMEs Test and GC Analysis
A 0.1 g wet sample of Chlorella was hydrolyzed and methylated with 2 ml of 100% acetyl chloride in 20 ml of methanol solution at 90°C (Ge et al., 2018). Then, filter the sample with filter paper (90 mm, Whatman) by washing it with 10 ml of methanol. Next, use a rotary evaporator to evaporate the methanol, and then add 10 ml of hexane and vortex the sample for 5 min. Use a glass pipette (Fisherbrand™ Pasteur) to remove the hexane layer and evaporate the hexane, and then analyze the recovered FAME by gas chromatography (GC). Helium was used as a carrier gas. The temperature of the injector and detector is 260°C. The FAME peaks in the samples were identified by comparing their retention times with those of the standards (Supelco TM 37 component FAME mix, Sigma-Aldrich).
Results
Microalgae Growth
C. vulgaris was cultivated in MTDW and BG11 (control) under 30°C, 4,000 lx, 40% Vinoculation/Vmedia growth condition for 15 days to assess biomass and biomass productivity. The growth curve of C. vulgaris was shown in Figure 1A. The algal biomass in MTDW and control are 0.65 g L−1 and 0.26 g L−1 with biomass productivity of 0.04 g L−1 d−1 (MTDW) and 0.02 g L−1 d−1 (control) respectively, after 15 days. This result was totally higher than the results obtained by Tan et al. (2018). The study showed maximum Chl-a (see Figure 1B) content in both the MTDW and the control with 6.48 ± 0.67 mg L−1 and 1.80 ± 0.65 mg L−1 on day 14 and day 8 respectively. However, both media showed a decreasing trend in Chl-a, with 5.73 ± 0.94 mg L−1 and 0.76 ± 0.16 mg L−1 at the end of the cultivation period. Both treatments showed totally different growth patterns. The MTDW experienced a lag phase between day 1 and 2, and the exponential growth phase was experienced in day 3 and lasted for 14 days. The stationary phase set in toward the end of the cultivation period as the nutrient concentration diminished. The control showed steady growth throughout the cultivation period. The lag phase lasted for a day whiles exponential growth begun on the third day and lasted up to day 14.
Biochemical Composition of C. vulgaris Cultivated in MTDW and BG11 Media
Table 1 depicts the biochemical composition of C. vulgaris biomass cultivated in MTDW and control (BG11) at the end of the treatment. As the results showed, MTDW demonstrated 2-fold higher protein content than the control. The MTDW recorded 49.6 ± 1.4% protein content compared to 22.4 ± 2.3% of the control medium. And 26.1 ± 0.6% and 29.9 ± 1.1%, and 10.4 ± 1.8% and 16.2 ± 0.4% of carbohydrate and lipids for MTDW and the control respectively. Compared with the control, the reason for the lower carbohydrate and lipid content in MTDW may be related to the light intensity. This is in agreement with a similar result obtained by Qiu et al. (2019). It is however clear that MTDW can promote protein production in C. vulgaris than BG11 medium. This result was slightly higher than that of Miao et al. (2016). It is documented that higher nitrogen content induces algae growth that could lead to accumulation of amino acid (Martínez et al., 2000). C. vulgaris showed higher biomass growth in MTDW than BG11 which translated into higher protein production.
The amino acid content and composition were analyzed in MTDW and BG11 at the end of the cultivation. As shown in Table 2, all tested amino acids in the MTDW group were higher than those in the control group.
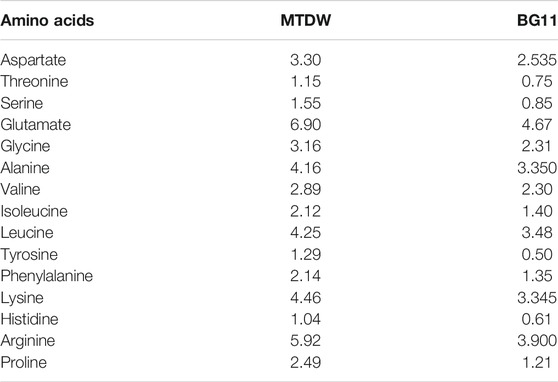
TABLE 2. Amino acids compositions and contents (g/100 g dry biomass) of C. vulgaris cultivated in MTDW and BG11.
GC analysis of fatty acid composition C. vulgaris in both MTDW and the control are shown in Table 3. The contents of Myristic Acid, Palmitic Acid, Margaric Acid, Oleic Acid, Linoleic Acid, Erucic Acid, and 11,14,17-Eicosatrienoic Acid in the MTDW group were lower than those in the control group, and the contents of Palmitoleic Acid, Stearic Acid, Arachidonic Acid, and α-Linolenic Acid were higher than those in the control group. Pentadecanoic Acid, γ-Linolenic Acid, Behenic Acid, Arachidonic Acid, 13-16-Docosadienoic Acid, Lignoceric Acid, and 15-Tetracosenoic Acid were similar in both MTDW and the control.
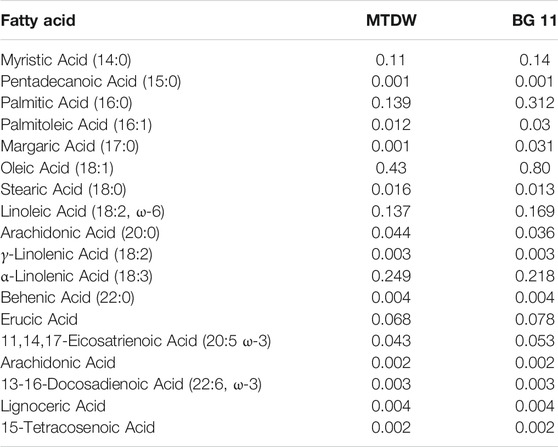
TABLE 3. Fatty acid compositions and contents (g/100 g dry biomass) of C. vulgaris cultivated in MTDW and BG11.
MTDW Treatment Using C. vulgaris
TN, TP, and COD Removal
The concentration of TN, TP, and COD were presented in Figure 2. Nitrogen, is the main component of algal proteins and enzymes catalyst, and are responsible for microalgae growth, photosynthesis and metabolism (Kong et al., 2021). The study showed 80% TN removal efficiency. However, the highest removal rate was observed on the exponential growth phase. This coincides with a study reported by Iasimone et al. (2018).
Phosphorus is another key element for microalgae growth and other cellular activities (energy transfer and biosynthesis of nucleic acids) (Kong et al., 2021). Affected by the utilization of C. vulgaris, TP concentration decreased steadily within the first 12 days until stable on day 15. Affected by C. vulgaris, 94% TP removal efficiency was recorded at the end of cultivation period. Based on the results, phosphorus (TP) removal could directly be affected by C. vulgaris growth due to culture conditions. Asian and Kapdan (2006) reported 78% phosphate removal efficiency for C. vulgaris cultivation at 7.7 mg L−1 initial concentration, less than 30% removal efficiency at higher concentration, this is clear that cultivation conditions of microalgae could affect phosphorus removal efficiency.
COD concentration (Figure 2C) varied during the period of measurement. There was a slide increase in concentration on day 6 and day 9. Again, the highest COD removal was recorded at the exponential growth phase of C. vulgaris with 72.24% removal efficiency. It was observed from the results that COD in MTWD was effectively utilized by C. vulgaris.
Macro and Micronutrient Removal
As shown in Table 4, at the end of the cultivation period, removal efficiency obtained for macronutrients ranged between 3.92 and 100% compared to 15.56–42.86% for micronutrients. Ca2+ reduced from the initial concentration of 0.01 mg L−1 to 0.00 mg L−1 in MTDW with a removal efficiency of 100%. The concentration of Mg2+ reduced from 18.8 mg L−1 to 2.75 mg L−1, and the removal efficiency reached 85.37%. The concentration of Na+ and K+ decreased from 269 mg L−1 to 1,020 mg L−1–253 mg L−1 and 980 mg L−1, and the corresponding removal efficiencies were 5.59 and 3.92%, respectively. For micronutrients, Mo2- had the highest removal efficiency of 42.86%, and the concentration reduced from 0.07 mg L−1 to 0.04 mg L−1. Followed by As3+, B−, and Pb2+, their concentrations were reduced from 0.03 mg L−1, 0.30 mg L−1, and 0.03 mg L−1 to 0.02 mg L−1, 0.20 mg L−1, and 0.02 mg L−1, respectively, and the corresponding removal efficiencies were all 33.3%. The concentration of Cu2+ and Fe3+ in MTDW decreased from the initial 0.18 mg L−1 and 1.80 mg L−1 to 0.13 mg L−1 and 1.52 mg L−1, and the removal efficiency reached 27.78 and 15.56%, which were lower than other micronutrients. In addition, Zn2+ and Mn2+ were not detected in this experiment.
Discussion
Microalgae is a single-cell bioreactor driven by sunlight that converts carbon dioxide into potential proteins, lipids, carbohydrates, and high-value biological compounds, in the presence of a sufficient amount of nitrogen, phosphorous, and some trace elements. By 2024, the overall market potential of algae-based products expected to reach approximately USD 1.143 billion (Mehta et al., 2018). Meanwhile, the viable market potential of microalgae in the phytoremediation of wastewater and biofuels is currently increasing (Mustafa et al., 2021). Wastewater treatment with microalgae has been considered an environmentally sound bioremediation method and applied for more than 60 years (Jing et al., 2007). Many microalgae can grow effectively under wastewater conditions by utilizing the rich inorganic nitrogen and phosphorus in wastewater, such as Desmdesmus sp. (Benítez et al., 2018), Scenedesmus sp. (Han et al., 2020), Acutodesmus dimorphus (Chokshi et al., 2016), C. vulgaris (Lv et al., 2018; Mujtaba et al., 2018), and so on. Due to its rich in protein and other nutrients, bio-safety, and the feasibility of large-scale outdoor cultivation and maintenance, Chlorella has become one of the most in-depth studies of microalgae in biomass production and wastewater treatment (Liu and Chen, 2014). Previous studies have shown that Chlorella can grow and produce biomass in wastewater such as urban wastewater (Tercero et al., 2014), domestic wastewater (Aziz and Ng, 1992), textile wastewater (Chu et al., 2009), piggery wastewater (Ji et al., 2012), etc. Our study showed that cultivating Chlorella with MTDW was also a feasible strategy.
Although Chlorella is easily adaptable to different wastewater media, the nutrients in the wastewater significantly affect the growth of microalgae and the production of biomass (Cai et al., 2013). When wastewater is used as a nutrient source for wastewater-based microalgae cultivation, carbon: nitrogen (C: N) and carbon: phosphorus (C:P) ratios could be considered (Chiu et al., 2015). Lee and Lee (2002) pointed out that Chlorella kessleri culture could successfully remove high concentrations of nitrogen from wastewater supplemented with glucose, indicating that sufficient carbon source supply was beneficial to the utilization of nitrogen and phosphorus. Chui et al. (2015) suggested that the carbon limitation in wastewater could be overcome by adding waste CO2, such as flue gas. In addition, the typical N/P ratio for the optimal conditions for microalgal biomass production is 8:1 (Chui et al., 2015). However, the N/P ratio of MTDW is close to 5:2, which means that the nitrogen source in the wastewater would be another limiting factor for microalgae growth. According to Chiu et al. (2015), the biomass productivity of Chlorella in different wastewater ranged from 0.029 g L−1 d−1 to 0.64 g L−1 d−1. In contrast, 0.04 g L−1 d−1 biomass productivity of Chlorella cultured in MTDW is not high, indicating that the use of MTDW to cultivate Chlorella to produce biomass still has much room for improvement.
Due to microalgae can use nutrients in wastewater to promote their growth, microalgal are particularly useful for reducing the concentration of inorganic nitrogen and phosphorus of wastewater (Ahluwalia and Goyal, 2007). Previous studies have shown that Chlorella has a very significant removal effect on nitrogen, phosphorus, and COD in different wastewater (Lam et al., 2017; Benítez et al., 2018; Lv et al., 2018; Mujtaba et al., 2018). However, the nutrient concentration of wastewater from different sources is different, which has a direct impact on the removal of nutrients. Kumar et al. (2019) reported that the nitrogen removal efficiency of Chlorella in sewage wastewater (38%) was lower than that of kitchen wastewater (67%), while the removal efficiency of phosphorus (88%) is higher than that of kitchen wastewater (75%). In this study, the removal rate of TP in MTDW by Chlorella reached 94%, the removal rate of TN was 80%, and the removal rate of COD exceeded 70%. These results indicate that Chlorella was very effective in MTDW treatment to reduce the organic and inorganic nutrients released into natural water, thereby preventing eutrophication problems.
Many studies have shown that utilizing microalgae could effectively remove metal elements from wastewater (Cabanelas et al., 2013; Cho et al., 2013; Zhu et al., 2013). Some metal ions can be attached to the cell surface through one or more surface complexation, ion exchange, and redox (Sheng et al., 2004; Vinod et al., 2010). Biosorption also involves cell metabolism and other processes, during which metal ions enter the cell through metal transporters, and are finally stored in vacuoles or organelles (Mehta and Gaur, 2005; Flórez-Miranda et al., 2017). Chlorella has been reported to remove many metals from wastewater, including Al, Ca, Cd, Cu, Fe, Mg, Mn, Ni, Ur, and Zn (Sandau et al., 1996; Lau et al., 1999; Chong et al., 2000; Mehta and Gaur, 2001; Mehta and Gaur, 2005; Wang et al., 2009). Similar to previous studies’ conclusions, our study shown that Chlorella could effectively remove Ca, Mg, Mo, Fe, As, B, Pb, and Cu in distillery wastewater.
Cultivation of microalgae in large quantities is challenged by the high cost of nutrients and freshwater. According to (Kadir et al., 2018), the cultivation of microalgae in wastewater is an alternative way of overcoming the current high cost of microalgae cultivation. Slade and Bauen (2013) estimated more than 50% reduction in production cost by cultivation microalgae in wastewater as a nutrient, CO2 and freshwater source. The composition of MTDW is stable and could be used for Chlorella cultivation without complicated treatment, which can effectively reduce the cost of Chlorella cultivation. On the other hand, Chlorella can remove nutrients, organic matter, and metals from MTDW. These substances may lead to the destruction of the aquatic environment causing eutrophication, affecting human health and recreational activities (Stutter et al., 2018). In addition, because biomass is rich in protein and fatty acids, C. vulgaris could be cultivated in MTDW as a high-quality protein source in aquaculture.
Conclusion
The growth and biochemical composition of C. vulgaris cultivated in MTDW and nutrients removal efficiency from the wastewater were analyzed. After cultivated 15 days in MTDW, 0.65 g L−1 algal biomass with biomass productivity of 0.04 g L−1 d−1 were obtained. The protein content, carbohydrate, and lipids reached 49.6 ± 1.4%, 26.1 ± 0.6%, and 10.4 ± 1.8%, respectively. 94% of phosphorus and 80% of nitrogen were removed from MTDW, and the removal efficiency of COD reached 72.24%. In addition, there was the highest removal efficiency of Ca2+ in MTDW with recording a 100%. Followed by Mg2+, an 85.37% removal efficiency was reached. The removal efficiency of other nutrients Na+, K+, Fe3+, As3+, B−, Mo2-, and Cu2+ obtained ranged 3.92–42.86%. This study proved that it was feasible to cultivate Chlorella with MTDW and represented an economical and environmentally friendly Chlorella cultivation strategy. There appears to be a great potential for Chlorella in the area of tertiary distillery wastewater treatment. The feasibility of applying it to full-scale requires further research in culture strategy to maximize biomass production and improve the removal efficiency of nutrients in wastewater.
Data Availability Statement
The original contributions presented in the study are included in the article/Supplementary Material, further inquiries can be directed to the corresponding author.
Author Contributions
FL: Data curation, methodology, writing-original draft, writing-review and editing; DA: Data curation, investigation, writing-original draft; YZ: Methodology; NZ: Methodology; CL: Project administration; XH: Project administration, resources.
Conflict of Interest
The authors declare that the research was conducted in the absence of any commercial or financial relationships that could be construed as a potential conflict of interest.
Publisher’s Note
All claims expressed in this article are solely those of the authors and do not necessarily represent those of their affiliated organizations, or those of the publisher, the editors and the reviewers. Any product that may be evaluated in this article, or claim that may be made by its manufacturer, is not guaranteed or endorsed by the publisher.
References
Abdel-Raouf, N., Al-Homaidan, A. A., and Ibraheem, I. B. M. (2012). Microalgae and Wastewater Treatment. Saudi J. Biol. Sci. 19 (3), 257–275. doi:10.1016/j.sjbs.2012.04.005
Ahluwalia, S. S., and Goyal, D. (2007). Microbial and Plant Derived Biomass for Removal of Heavy Metals from Wastewater. Bioresour. Techn. 98, 2243–2257. doi:10.1016/j.biortech.2005.12.006
Al-Mamun, M. R., Kader, S., Islam, M. S., and Khan, M. Z. H. (2019). Photocatalytic Activity Improvement and Application of UV-TiO2 Photocatalysis in Textile Wastewater Treatment: A Review. J. Environ. Chem. Eng. 7 (5), 103248–103437. doi:10.1016/j.jece.2019.103248
Amenorfenyo, D. K., Huang, X., Li, C., Li, F., Zeng, Q., Zhang, N., et al. (2020). A Review of Microalgae and Other Treatment Methods of Distillery Wastewater. Water Environ. J. 34, 988–1002. doi:10.1111/wej.12552
Aslan, S., and Kapdan, I. K. (2006). Batch Kinetics of Nitrogen and Phosphorus Removal from Synthetic Wastewater by Algae. Ecol. Eng. 28 (1), 64–70. doi:10.1016/j.ecoleng.2006.04.003
Aziz, M. A., and Ng, W. J. (1992). Feasibility of Wastewater Treatment Using the Activated-Algae Process. Bioresour. Techn. 40, 205–208. doi:10.1016/0960-8524(92)90143-L
Barbarino, E., and Lourenço, S. O. (2005). An Evaluation of Methods for Extraction and Quantification of Protein from Marine Macro- and Microalgae. J. Appl. Phycology 17 (5), 447–460. doi:10.1007/s10811-005-1641-4
Benítez, M. B., Champagne, P., Ramos, A., Torres, A. F., and Ochoa-Herrera, V. (2018). Wastewater Treatment for Nutrient Removal with Ecuadorian Native Microalgae. Environ. Techn. 40, 2977–2985. doi:10.1080/09593330.2018.1459874
Cabanelas, I. T. D., Ruiz, J., Arbib, Z., Chinalia, F. A., Garrido-Pérez, C., Rogalla, F., et al. (2013). Comparing the Use of Different Domestic Wastewaters for Coupling Microalgal Production and Nutrient Removal. Bioresour. Techn. 131, 429–436. doi:10.1016/j.biortech.2012.12.152
Cai, T., Park, S. Y., and Li, Y. (2013). Nutrient Recovery from Wastewater Streams by Microalgae: Status and Prospects. Renew. Sust. Energ. Rev. 19, 1364–0321. doi:10.1016/j.rser.2012.11.030
Chiu, S., Kao, C., Chen, T., Chang, Y., Kuo, C., and Lin, C. (2015). Cultivation of Microalgal Chlorella for Biomass and Lipid Production Using Wastewater as Nutrient Resource. Bioresour. Techn. 184, 179–189. doi:10.1016/j.biortech.2014.11.080
Cho, S., Lee, N., Park, S., Yu, J., Luong, T. T., Oh, Y. K., et al. (2013). Microalgae Cultivation for Bioenergy Production Using Wastewaters from A Municipal WWTP as Nutritional Sources. Bioresour. Techn. 131, 515–520. doi:10.1016/j.biortech.2012.12.176
Chokshi, K., Pancha, I., Ghosh, A., and Mishra, S. (2016). Microalgal Biomass Generation by Phycoremediation of Dairy Industry Wastewater: An Integrated Approach towards Sustainable Biofuel Production. Bioresour. Techn. 221, 455–460. doi:10.1016/j.biortech.2016.09.070
Chong, A. M. Y., Wong, Y. S., and Tam, N. F. Y. (2000). Performance of Different Microalgal Species in Removing Nickel and Zinc from Industrial Wastewater. Chemosphere 41, 251–257. doi:10.1016/S0045-6535(99)00418-X
Chu, W. L., See, Y. C., and Phang, S. M. (2009). Use of Immobilised Chlorella Vulgaris for the Removal of Colour from Textile Dyes. J. Appl. Phycology 21, 641–648. doi:10.1007/s10811-008-9396-3
Deng, S., Jothinathan, L., Cai, Q., Li, R., Wu, M., Ong, S. L., et al. (2021). FeOx@GAC Catalyzed Microbubble Ozonation Coupled with Biological Process for Industrial Phenolic Wastewater Treatment: Catalytic Performance, Biological Process Screening and Microbial Characteristics. Water Res. 190, 116687–121354. doi:10.1016/j.watres.2020.116687
Deng, S., Peng, S., Xie, B., Yang, X., Sun, S., Yao, H., et al. (2020). Influence Characteristics and Mechanism of Organic Carbon on Denitrification, N2O Emission and NO2− Accumulation in the Iron [Fe(0)]-Oxidizing Supported Autotrophic Denitrification Process. Chem. Eng. J. 393, 124736–128947. doi:10.1016/j.cej.2020.124736
Flórez-Miranda, L., Cañizares-Villanueva, R. O., Melchy-Antonio, O., Martínez-Jerónimo, F., and Flores-Ortíz, C. M. (2017). Two Stage Heterotrophy/Photoinduction Culture of Scenedesmus Incrassatulus: Potential for Lutein Production. J. Biotechnol. 262, 67–74. doi:10.1016/j.jbiotec.2017.09.002
Gang, L., Li, X., Qi, Z., and Sun, J. (2008). Status Quo and Development of the Resource Recycling Treatment Technologies of Cassava Ethanol Wastewater. Ind. Water Treat. 4 (08), 1–5. (In Chinese).
Ge, S., and Champagne, P. (2016). Nutrient Removal, Microalgal Biomass Growth, Harvesting and Lipid Yield in Response to Centrate Wastewater Loadings. Water Res. 88, 604–612. doi:10.1016/j.watres.2015.10.054
Ge, S., Qiu, S., Tremblay, D., Viner, K., Champagne, P., and Jessop, P. G. (2018). Centrate Wastewater Treatment with Chlorella Vulgaris: Simultaneous Enhancement of Nutrient Removal, Biomass and Lipid Production. Chem. Eng. J. 342, 310–320. doi:10.1016/j.cej.2018.02.058
Guo, H. C., Chen, B., Yu, X. L., Huang, G. H., Liu, L., and Nie, X. H. (2006). Assessment of Cleaner Production Options for Alcohol Industry of China: A Study in the Shouguang Alcohol Factory. J. Clean. Prod. 14 (1), 94–103. doi:10.1016/j.jclepro.2004.07.006
Han, J., Thomsen, L., Pan, K., Wang, P., Wawilow, T., Osundeko, O., et al. (2020). Treating Wastewater by Indigenous Microalgae Strain in Pilot Platform Located inside a Municipal Wastewater Treatment Plant. Environ. Techn. 41, 3271. doi:10.1080/09593330.2019.1604816
Hanchang, S. H. I. (2009). Industrial Wastewater-Types, Amounts and Effects. Point Sourc. Pollut. Local Effects Their Control. 2, 191–203.
Hansen, É., Rodrigues, M. A. S., Aragão, M. E., and de Aquim, P. M. (2018). Water and Wastewater Minimization in A Petrochemical Industry through Mathematical Programming. J. Clean. Prod. 172, 1814–1822. doi:10.1016/j.jclepro.2017.12.005
Hartmut, K., and Alan, R. W. (1983). Determinations of Total Carotenoids and Chlorophylls a and B of Leaf Extracts in Different Solvents. Analysis 4, 142–196. doi:10.1042/bst0110591
Iasimone, F., Felice, V. D., Fantasma, F., Iorizzi, M., and Pirozzi, F. (2018). Effect of Light Intensity and Nutrients Supply on Microalgae Cultivated in Urban Wastewater: Biomass Production, Lipids Accumulation and Settleability Characteristics. J. Environ. Manage. 223, 1078–1085. doi:10.1016/j.jenvman.2018.07.024
Ji, M. K., Kim, H. C., Sapireddy, V. R., Yun, H. S., Abou-Shanab, R. A. I., Choi, J., et al. (2012). Simultaneous Nutrient Removal and Lipid Production from Pretreated Piggery Wastewater by Chlorella Vulgaris YSW-04. Appl. Microbiol. Biotechnol. 97, 2701–2710. doi:10.1007/s00253-012-4097-x
Jing, S., Podola, B., and Melkonian, M. (2007). Removal of Nitrogen and Phosphorus from Wastewater Using Microalgae Immobilized on Twin Layers: An Experimental Study. J. Appl. Phycology 19, 417–423. doi:10.1007/s10811-006-9148-1
Kadir, W. N. A., Lam, M. K., Uemura, Y., Lim, J. W., and Lee, K. T. (2018). Harvesting and Pre-treatment of Microalgae Cultivated in Wastewater for Biodiesel Production: A Review. Energ. Convers. Manag. 171, 1416–1429. doi:10.1016/j.enconman.2018.06.074
Kong, W., Kong, J., Ma, J., Lyu, Hong., Feng, S., Wang, Z., et al. (2021). Chlorella Vulgaris Cultivation in Simulated Wastewater for the Biomass Production, Nutrients Removal and CO2 Fixation Simultaneously. J. Environ. Manage. 284, 112070. doi:10.1016/j.jenvman.2021.112070
Kumar, P. K., Krishna, S. V., Naidu, S. S., Kavita, V., Bhagawan, D., and Himabindu, V. (2019). Biomass Production from Microalgae Chlorella Grown in Sewage, Kitchen Wastewater Using Industrial CO2 Emissions: Comparative Study. Carbon Resour. Convers. 2 (2), 126–133. doi:10.1016/j.crcon.2019.06.002
Lam, M. K., Yusoff, M. I., Uemura, Y., Lim, J. W., Khoo, C. G., Lee, K. T., et al. (2017). Cultivation of Chlorella Vulgaris Using Nutrients Source from Domestic Wastewater for Biodiesel Production: Growth Condition and Kinetic Studies. Renew. Energ. 103, 197–207. doi:10.1016/j.renene.2016.11.032
Lau, P. S., Lee, H. Y., Tsang, C. C. K., Tam, N. F. Y., and Wong, Y. S. (1999). Effect of Metal Interference, pH and Temperature on Cu and Ni Biosorption by Chlorella Vulgaris and Chlorella Miniata. Environ. Techn. 20, 953–961. doi:10.1080/09593332008616890
Lee, K., and Lee, C. G. (2002). Nitrogen Removal from Wastewaters by Microalgae without Consuming Organic Carbon Sources. J. Microbiol. Biotechnol. 12 (6), 979–985.
Lin, R., Cheng, J., Yang, Z., Ding, L., Zhang, J., Zhou, J., et al. (2016). Enhanced Energy Recovery from Cassava Ethanol Wastewater through Sequential Dark Hydrogen, Photo Hydrogen and Methane Fermentation Combined with Ammonium Removal. Bioresour. Techn. 214, 686–691. doi:10.1016/j.biortech.2016.05.037
Lindholm-Lehto, P. C., Knuutinen, J. S., AhkolaHerve, H. S. J. S. H., and Herve, S. H. (2015). Refractory Organic Pollutants and Toxicity in Pulp and Paper Mill Wastewaters. Environ. Sci. Pollut. Res. 22, 6473–6499. doi:10.1007/s11356-015-4163-x
Liu, J., and Chen, F. (2014). “Biology and Industrial Applications of Chlorella: Advances and Prospects,” in Microalgae Biotechnology. Editors C Posten, and F Chen (Springer. Part of the Advances in Biochemical Engineering/Biotechnology book series), 1–35. doi:10.1007/10_2014_286
Liu, J., and Hu, Q. (2013). “Chlorella: Industrial Production of Cell Mass and Chemicals,” in Handbook of Microalgal Culture: Applied Phycology and Biotechnology. Editors A Richmond, and Q Hu (Wiley), 329–338. doi:10.1002/9781118567166.ch16
Liu, Y., Ke, X., Zhu, H., Chen, R., Chen, X., Zheng, X., et al. (2020). Treatment of Raffinate Generated via Copper Ore Hydrometallurgical Processing Using a Bipolar Membrane Electrodialysis System. Chem. Eng. J. 382, 122956–128947. doi:10.1016/j.cej.2019.122956
Lv, J. P., Liu, Y., Feng, J., Liu, Q., Nan, F. R., and Xie, S. L. (2018). Nutrients Removal from Undiluted Cattle Farm Wastewater by the Two-Stage Process of Microalgae-Based Wastewater Treatment. Bioresour. Techn. 264, 311–318. doi:10.1016/j.biortech.2018.05.085
Martínez, M. E., Sánchez, S., Jimenez, J. M., El Yousfi, F., and Munoz, L. (2000). Nitrogen and Phosphorus Removal from Urban Wastewater by the Microalga Scenedesmus Obliquus. Bioresour. Technol. 73 (3), 263–272. doi:10.1016/S0960-8524(99)00121-2
Mehta, P., Singh, D., Saxena, R., Rani, R., Gupta, R. P., Puri, S. K., et al. (2018). ““High-Value Coproducts from Algae—An Innovational Way to Deal with Advance Algal Industry”,” in Waste to Wealth. Editors R Singhania, R Agarwal, R Kumar, and R Sukumaran Springer. Part of the Energy, Environment, and Sustainability Book Series, 343–363. doi:10.1007/978-981-10-7431-8_15
Mehta, S. K., and Gaur, J. P. (2001). Removal of Ni and Cu from Single and Binary Metalsolutions by Free and Immobilized Chlorella Vulgaris. Eur. J. Protistology 37, 261–271. doi:10.1078/0932-4739-00813
Mehta, S. K., and Gaur, J. P. (2005). Use of Algae for Removing Heavy Metal Ions from Wastewater: Progress and Prospects. Crit. Rev. Biotechnol. 25, 113–152. doi:10.1080/07388550500248571
Miao, M. S., Yao, X. D., Shu, L., Yan, Y. J., Wang, Z., Li, N., et al. (2016). Mixotrophic Growth and Biochemical Analysis of Chlorella Vulgaris Cultivated with Synthetic Domestic Wastewater. Int. Biodeterioration Biodegradation 113, 120–125. doi:10.1016/j.ibiod.2016.04.005
Mujtaba, G., Rizwan, M., Kim, G., and Lee, K. (2018). Removal of Nutrients and COD through Co-culturing Activated Sludge and Immobilized Chlorella Vulgaris. Chem. Eng. J. 343, 155–162. doi:10.1016/j.cej.2018.03.007
Mustafa, H. M., Hayder, G., and Jagaba, A. H. (2021). Microalgae: A Renewable Source for Wastewater Treatment and Feedstock Supply for Biofuel Generation. Biointerface Res. Appl. Chem. 11, 7431–7444. doi:10.33263/BRIAC111.74317444
Mutamim, N. S. A., Noor, Z. Z., Hassan, M. A. A., and Yuniarto, A. (2013). Membrane Bioreactor: Applications and Limitations in Treating High Strength Industrial Wastewater. Chem. Eng. J. 225, 109–119. doi:10.1016/j.cej.2013.02.131
Peng, S., Deng, S., Li, D., Xie, B., Yang, X., Lai, C., et al. (2020). Iron-Carbon Galvanic Cells Strengthened Anaerobic/Anoxic/Oxic Process (Fe/C-A2o) for High-Nitrogen/Phosphorus and Low-Carbon Sewage Treatment. Sci. Total Environ. 722, 137657–139697. doi:10.1016/j.scitotenv.2020.137657
Qasim, W., and Mane, A. V. (2013). Characterization and Treatment of Selected Food Industrial Effluents by Coagulation and Adsorption Techniques. Water Resour. Industry 4, 1–12. doi:10.1016/j.wri.2013.09.005
Qiu, S., Wang, L., Pascale, C., Gao, G., Chen, Z., Wang, S., et al. (2019). Effects of Crystalline Nanocellulose on Wastewater-Cultivated Microalgal Separation and Biomass Composition. Appl. Energ. 239, 0306–2619. doi:10.1016/j.apenergy.2019.01.212
Quan, X., Tao, K., Mei, Y., and Jiang, X. (2014). Power Generation from Cassava Alcohol Wastewater: Effects of Pretreatment and Anode Aeration. Bioproc. Biosyst Eng 37 (11), 2325–2332. doi:10.1007/s00449-014-1210-9
Sánchez-Zurano, A., Guzmán, J. L., Acién, F. G., and Fernández-Sevilla, J. M. (2021). An Interactive Tool for Simulation of Biological Models into the Wastewater Treatment with Microalgae. Front. Environ. Sci. 9, 298. doi:10.3389/fenvs.2021.721324
Sandau, E., Sandau, P., and Pulz, O. (1996). Heavy Metal Sorption by Microalgae. Acta Biotechnologica 16, 227–235. doi:10.1002/abio.370160402
Sanjay, P., and Janmaluddin, (2018). Treatment of Distillery Waste Water: A Review. Int. J. Theor. Appl. Sci. 10 (1), 117–139.
Sheng, P. X., Ting, Y. P., Chen, J. P., and Hong, L. (2004). Sorption of Lead, Copper, Cadmium, Zinc, and Nickel by Marine Algal Biomass: Characterization of Biosorptive Capacity and Investigation of Mechanisms. J. Colloid Interf. Sci. 275, 131–141. doi:10.1016/j.jcis.2004.01.036
Slade, R., and Bauen, A. (2013). Micro-algae Cultivation for Biofuels: Cost, Energy Balance, Environmental Impacts and Future Prospects. Biomass and Bioenergy 53, 29–38. doi:10.1016/j.biombioe.2012.12.019
Stutter, M. I., Graeber, D., Evans, C. D., Wade, A. J., and Withers, P. J. A. (2018). Balancing Macronutrient Stoichiometry to Alleviate Eutrophication. Sci. Total Environ. 634, 439–447. doi:10.1016/j.scitotenv.2018.03.298
Tan, X. B., Zhao, X. C., Zhang, Y. L., Zhou, Y. Y., Yang, L. B., and Zhang, W. W. (2018). Enhanced Lipid and Biomass Production Using Alcohol Wastewater as Carbon Source for Chlorella Pyrenoidosa Cultivation in Anaerobically Digested Starch Wastewater in Outdoors. Bioresour. Technol. 247, 784–793. doi:10.1016/j.biortech.2017.09.152
Tercero, E. A. R., Sforza, E., Morandini, M., and Bertucco, A. (2014). Cultivation of Chlorella Protothecoides with Urban Wastewater in Continuous Photobioreactor: Biomass Productivity and Nutrient Removal. Appl. Biochem. Biotechnol. 172, 1470–1485. doi:10.1007/s12010-013-0629-9
Thoré, E. S. J., Schoeters, F., De Cuyper, A., Vleugels, R., Noyens, I., Bleyen, P., et al. (2021). Waste Is the New Wealth - Recovering Resources from Poultry Wastewater for Multifunctional Microalgae Feedstock. Front. Environ. Sci. 9, 253. doi:10.3389/fenvs.2021.679917
Vinod, V. T. P., Sashidhar, R. B., and Sreedhar, B. (2010). Biosorption of Nickel and Total Chromium from Aqueous Solution by Gum Kondagogu (Cochlospermum Gossypium): A Carbohydrate Biopolymer. J. Hazard. Mater. 178, 851–860. doi:10.1016/j.jhazmat.2010.02.016
Wang, L., Min, M., Li, Y. C., Chen, P., Chen, Y. F., Liu, Y. H., et al. (2009). Cultivation of Green Algae Chlorella Sp. In Different Wastewaters from Municipal Wastewater Treatment Plant. Appl. Biochem. Biotechnol. 162, 1174–1186. doi:10.1007/s12010-009-8866-7
Yang, B., and Wyman, C. E. (2008). Pretreatment: The Key to Unlocking Low-Cost Cellulosic Ethanol. Biofuels, Bioprod. Bioref. 2 (1), 26–40. doi:10.1002/bbb.49
Zhu, L., Wang, Z., Takala, J., Hiltunen, E., Qin, L., Xu, Z., et al. (2013). Scale-up Potential of Cultivating Chlorella Zofingiensis in Piggery Wastewater for Biodiesel Production. Bioresour. Techn. 137, 318–325. doi:10.1016/j.biortech.2013.03.144
Keywords: wastewater treatment, microalgae, Chlorella, distiller wastewater, biomass production
Citation: Li F, Amenorfenyo DK, Zhang Y, Zhang N, Li C and Huang X (2021) Cultivation of Chlorella vulgaris in Membrane-Treated Industrial Distillery Wastewater: Growth and Wastewater Treatment. Front. Environ. Sci. 9:770633. doi: 10.3389/fenvs.2021.770633
Received: 04 September 2021; Accepted: 08 October 2021;
Published: 21 October 2021.
Edited by:
Shihai Deng, Xi’an Jiaotong University, ChinaReviewed by:
Binghan Xie, Harbin Institute of Technology, Weihai, ChinaXu Zhou, Harbin Institute of Technology, Shenzhen, China
Pengfei Cheng, Ningbo University, China
Vineet Kumar, Guru Ghasidas Vishwavidyalaya, India
Copyright © 2021 Li, Amenorfenyo, Zhang, Zhang, Li and Huang. This is an open-access article distributed under the terms of the Creative Commons Attribution License (CC BY). The use, distribution or reproduction in other forums is permitted, provided the original author(s) and the copyright owner(s) are credited and that the original publication in this journal is cited, in accordance with accepted academic practice. No use, distribution or reproduction is permitted which does not comply with these terms.
*Correspondence: Xianghu Huang, aHVhbmd4aEBnZG91LmVkdS5jbg==
†These authors have contributed equally to this work and share first authorship