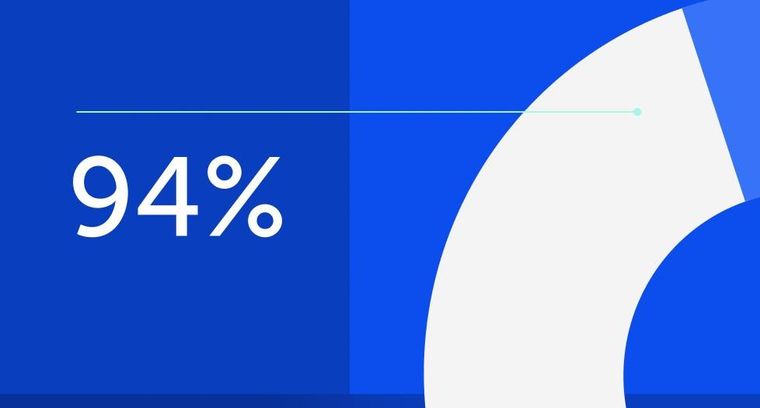
94% of researchers rate our articles as excellent or good
Learn more about the work of our research integrity team to safeguard the quality of each article we publish.
Find out more
ORIGINAL RESEARCH article
Front. Environ. Sci., 07 January 2022
Sec. Soil Processes
Volume 9 - 2021 | https://doi.org/10.3389/fenvs.2021.769871
This article is part of the Research TopicAgricultural Diversification: Benefits and Barriers for Sustainable Soil ManagementView all 12 articles
Agricultural yield of major crops is low due to the injudicious use of chemical fertilizers that affects soil fertility and biodiversity severely and thereby affecting plant growth. Soil health is regulated by various factors such as physicochemical properties of the soil, availability of micro/macronutrients, soil health indicator enzymes and microbial diversity which are essential for agriculture productivity. Thus, it is required to draw attention towards an eco-friendly approach that protects the beneficial microbial population of soil. Application of different bioinoculants and agriusable nanocompounds has been reported to enhance soil quality with increased nutrient status and beneficial bacterial population, but additive effects of combined treatments on soil microbial population are largely unknown. The present study investigated the impact of nanozeolite and nanochitosan along with two Bacillus spp. on rhizospheric microbial flora and indicator enzymes to signify soil health under field conditions on maize. Soil health was ascertained by evaluating physicochemical analysis; total bacterial counts including N, P, and K solubilizing bacteria; and soil health indicator enzymes like fluorescein diacetate hydrolysis, alkaline phosphatase, β-glucosidase, dehydrogenase, amylase, and arylesterase. Change in copy number of 16S rRNA as a marker gene was used to quantify the bacterial population using quantitative PCR (qPCR) in different treatments. Our study revealed that nanocompounds with Bacillus spp. significantly (p < 0.05) enhanced total microbial count (16.89%), NPK solubilizing bacteria (46%, 41.37%, and 57.14%), and the level of soil health indicator enzymes up to twofold over control after 20, 40, and 60 days of the experiment. qPCR analysis showed a higher copy number of the 16S rRNA gene in treated samples, which also indicates a positive impact on soil bacterial population. This study presents a valuable approach to improve soil quality in combined treatments of nanocompounds and bioinoculants which can be used as a good alternative to chemical fertilizers for sustainable agriculture.
Progression of life in all forms depends on the agriculture sector in most of the developing countries worldwide. Excessive and indiscriminate use of agrochemicals has inadvertently damaged soil health over time (Bunemann et al., 2018). Toxic chemicals have a detrimental effect on the key drivers of biogeochemical cycles and in the soil microbial community (Rousk and Bengtson, 2014; Kumar et al., 2021). It is therefore imperative to find safe and effective strategies contributing towards higher agronomic yield without jeopardizing the natural microflora of soil (Bargaz et al., 2018). Combined applications of plant growth promoting rhizobacteria (PGPR) and nanocompounds has potential to significantly improve the overall plant and soil health status. Use of microbes in the agricultural sector has a lengthy history, created through broad-scale inoculation of legumes in the 20th century (Desbrosses and Stougaard, 2011). Exploitation of beneficial PGPR such as Azotobacter, Azospirillum, Bacillus, and Pseudomonas in the form of biofertilizers can be an alternative to conventional chemical fertilizer (Vessey, 2003; Schütz et al., 2018). They promote plant growth by influencing plant hormone production, iron sequestration via siderophore, stress management via key enzymes such as 1-aminocyclopropane-1-carboxylate (ACC), and soil organic matter decomposition (Jahanian et al., 2012; Pandey and Gupta, 2019). Most importantly, they help to access macro/micro nutrients from the soil system and improve the plant growth (Beneduzi et al., 2012; Kour et al., 2020a). Microbial inoculants enhance nitrogen, phosphorus, and potassium fertilizer resource use efficiency, which is typically lost due to run-off and leaching in the atmosphere (Adesemoye and Kloepper, 2009). In particular, Bacillus and Pseudomonas species are best known to solubilize growth-limiting nutrients such as phosphate and potassium efficiently, which finally enhanced plant progress (Santoyo et al., 2012; Sharma et al., 2013; Chaudhary A. et al., 2021). Acinetobacter calcoaceticus is involved in phosphate solubilization and mitigated the drought toxic effects in foxtail (Setaria italica) (Kour et al., 2020b). More than 75% of globally marketed biofertilizers are associated with nitrogen fixing and P solubilizing/mobilizing property (Timmusk et al., 2017). High availability of NPK could extend survival rates of microorganisms in soil (Yang et al., 2011). Extracellular enzymes like dehydrogenase, fluorescein diacetate, alkaline phosphatase, and β-glucosidase produced by PGPR helps in functioning of soil ecosystem as well as nutrient cycling (Liu et al., 2017). Various reports support the positive impact of PGPR on seed germination, stimulation of root growth, and plant growth regulation though enzymatic activities (Vacheron et al., 2013). However, inconsistent behavior of biofertilizers under field conditions often limits their widespread adoption by farmers. Developing inoculant using beneficial microorganisms that have a longer shelf life and high efficacy is a major commercialization challenge (Backer et al., 2018).
The inclusion of nano-encapsulation knowledge might be used as a resourceful means to defend PGPR against environmental factors such as UV radiation and heat (Prasad et al., 2017). Enhancing their shelf life and allowing the controlled release of biofertilizers would allow their practical application worldwide (Vejan et al., 2016). Numerous studies have supported the possible application of nanocompounds in agricultural field to boost agricultural yield (Duhan et al., 2017). Foliar application of silver nanoparticles (AgNPs-40 mg L−1) significantly improved agronomical parameters (shoot height, shoot weight, and number of leaves) of fenugreek (Trigonella foenum-graecum) by twofold (Sadak, 2019). Nanofertilizers can sustain slow release of nutrients due to higher surface tension than conventional surfaces (Ghormade et al., 2011). Out of the different agriusable nanocompounds, nanozeolites and nanochitosan have found their wide application in the agriculture sector due to their small size, high surface tension, chelation capacity, and biocompatibility, which are helpful in improving bacterial population and agronomic yield (Ming and Allen, 2001; Chaudhary and Sharma, 2019). High porosity of zeolites and their selectivity for cations make them useful to promote nutrient use efficiency (Ramesh and Reddy, 2011). Nanozeolite as a natural substrate can support microbial growth. Positive response of nanozeolite (50 mg L−1) towards soil health indicator enzymes and thus microbial activity under in vitro conditions (Khati et al., 2018). A study by Yuvaraj and Subramanian (2018) suggested the possible application of nano-sized zeolites (90 nm) as Zn fertilizer carrier for slow release of zinc in soil. Another nontoxic polysaccharide-like chitosan being biodegradable and biocompatible is useful in the agricultural sector (Katiyar et al., 2015). It is known as a plant growth regulatory agent and suppresses the growth of fungal pathogens (Popova et al., 2016). According to Siddaiah et al. (2018), chitosan nanoparticles enhanced seed germination in pearl millet and protected from downy mildew. Nanocompounds (50 mg L−1) and Bacillus spp. enhanced agronomical/biochemical attributes and maize productivity (Chaudhary A. et al., 2021). To study the beneficial effects of nanocompounds, it is important to focus on their impact over factors involved in soil health, which are critical for soil fertility and agricultural productivity. NPs in higher concentration not only affect the functional diversity of microorganism’s enzyme activity in soil but indirectly pose risk to plant growth (Chavan and Nadanathangam, 2019). Soil microbial dynamics is a key factor for sustainable agricultural practice in the long term as a slight change in microbial population can severely deteriorate the soil quality (FAO, 2012; Jacoby et al., 2017). Microbial population, activities of soil enzymes, and availability of micro/macronutrients maintain soil health and its quality (Tahat et al., 2020). Physicochemical properties of the soil exhibit seasonal variation and are influenced by the nutrient content of the soil, which can modify the structure and composition of the bacterial community in the rhizosphere/bulk soil (Li et al., 2020). Among soil physicochemical properties, pH is known to affect bacterial community and enzymes, involved in solubilization of organic (C, N, P) and nutrient availability in soil/plant system (Lopez-Monejar et al., 2015; Ju et al., 2019).
Therefore, the main aim of this research was to investigate the role of nanocompounds along with Bacillus spp. on total bacterial count, nitrogen fixers (Azotobacter), potassium and phosphorus solubilizers, soil enzymes, and microbial community using advanced molecular techniques under field conditions on maize for the first time. Molecular methods provide distinctive insight into the composition, structure, and functioning of microbial population of an ecosystem (Griffiths et al., 2003). Relatively few studies have been conducted on the effect of agriusable nanocompound on soil health. The information obtained from these parameters provides the beneficial role of nanocompounds along with bioinoculants on soil, particularly focusing on soil management and the overall richness and diversity of bacterial population of maize rhizosphere.
Bioinoculants Bacillus spp. (Bacillus sp. PS2 and PS10) with accession nos. KX650178 and KX650179 were isolated from the agricultural field of the University. Both the bacterial cultures had plant growth-promoting properties like phosphorus solubilization, indole acetic acid, and siderophore production (Khati et al., 2019a). Nanozeolite and nanochitosan used in this study have the following parameters: size < 80 nm; refractive index, 1.47; pH, 7–8 and 7–9; and 99.90% purity (Khati et al., 2019b).
The field experiment was carried out in June to September 2017 at Govind Ballabh Pant University of Agriculture and Technology, Pantnagar (GBPUA&T). This site lies Southward of Shivalik Himalayas (79°E longitude and 29°N latitude). Summers are warm in this region with a maximum temperature of 35.5°C and a minimum temperature of 23°C, and a relative humidity of about 35% was recorded during the experiment. Maximum rainfall occurred in July. This study was carried out in randomized block design (RBD) with three replications for all treatment. A plot size of 14.70 m2 was used for the experiment. Each plot has a length of 4.2 m and a width of 3.5 m, with a row-to-row space of 60 cm and a plant-to-plant space of 20 cm (Supplementary Material S1).
Maize seed variety (DH296) was taken from the Crop Research Centre of GBPUAT, Pantnagar. Seeds were disinfected by ethanol (70%) and hydrogen peroxide (3%) followed by distilled water (Khati et al., 2017). Sterilized seeds were treated with bacterial cultures and nanocompounds (50 mg L−1). There were a total of nine treatments used in the experiment: control (T1), PS2 (T2), PS10 (T3), nanozeolite (T4), PS2 + nanozeolite (T5), PS10 + nanozeolite (T6), nanochitosan (T7), PS2 + nanochitosan (T8), and PS10 + nanochitosan (T9). Different treatments received 2 × 106 cfu population per seed. After proper treatment, seeds were kept under an incubator shaker at 25°C for 15 min at 100 rpm. Treated seeds were further used for field trial (Supplementary Material S2).
Sampling was carried out after 20, 40, and 60D (days) of the experiment. Rhizospheric soil from a maize root depth of 15 cm was collected from each replicate randomly and mixed appropriately. Soil samples were passed through a 2-mm sieve and used for physicochemical analyses, total bacterial count. and soil enzyme activities (Chaudhary et al., 2021a) (Figure 1).
FIGURE 1. Impact of nanocompounds and Bacillus spp. on soil health indicators under maize cultivation.
Soil pH was measured by making the solution of soil in distilled water (1:3) using a pH meter. Soil organic carbon was measured by using the method of Black (1965), while total nitrogen, available phosphorus, and potassium were detected by using the method of Jackson (1973) and Jackson, (1958).
Bacterial count was checked on diverse media such as nutrient agar, Ashby, Aleksandrow, and Pikovaskaya agar for total bacteria, nitrogen fixers (Azotobacter), potassium and phosphorus solubilizing bacterial count. Plates were incubated for 2–4 days at 30°C and bacterial colonies were counted. This analysis was performed in triplicate (Messer and Johnson 2000; Chai et al., 2015).
One gram of soil, sodium phosphate buffer (50 ml, pH 7.6), and 0.5 ml of FDA solution were added in a flask and incubated for 1 h at 24°C. Acetone (2 ml) was added in the flask to stop the reaction, centrifuged for 5 min at 8,000 rpm, and filtered using Whatman filter paper. Enzyme activity was assessed at 490 nm and expressed as g fluorescein g−1 dry soil h−1 (Schnurer and Rosswall, 1982).
Triphenyl tetrazolium chloride (TTC) solution was used to estimate the dehydrogenase activity. Tris buffer (0.1 M, pH 7.4) and TTC solution (5 ml) were added in 5 g of soil and placed in an incubator for 8 h. Acetone (25 ml) was added in a reaction mixture to stop the reaction and centrifuged for 10 min at 4,000 rpm. Obtained supernatant was filtered and absorbance was measured at 485 nm (Casida et al. 1964).
In a test tube, 1 g of soil, toluene (250 µl), modified universal buffer (MUB 4 ml), and p-nitrophenyl phosphate (1 ml, 25 mM) were added and incubated for 2 h at 37°C under shaking condition. Reaction was stopped using CaCl2 and Tris buffer, centrifuged, and filtered using Whatman filter paper. Enzyme activity was measured by taking the absorbance at 400 nm (Tabatabai and Bremner, 1969).
One gram of soil was taken in a flask, and toluene (0.25 ml), p-nitrophenyl-D-glucoside (1 ml), and MUB (4 ml, pH 6) were also added in the same flask. The mixture was incubated for 1 h at 37°C; tris buffer (4 ml) and CaCl2 (1 ml) were added to terminate the reaction and centrifuged at 8,000 rpm for 10 min. The obtained supernatant was filtered and color intensity was measured at 415 nm (Tabatabai, 1994).
One gram of soil, 1 ml of starch, and phosphate buffer (2.5 ml, pH 6) were added in a flask. The flask was incubated for 6 h at 30°C and centrifuged for 10 min at 12,000 rpm. The obtained supernatant (1 ml) and 1 ml of dinitro salicylate (DNS) were added in a test tube and placed for 5 min in a water bath. Enzyme activity was calculated by taking the absorbance at 540 nm (Bernfeld, 1951).
One gram of soil, 2 ml of MUB, and p-nitrophenyl phosphate (0.5 ml, 200 mM) were added in a flask and kept for 1 h in a water bath. The mixture was centrifuged for 5 min at 6,500 rpm. The obtained supernatant (1 ml) and 2 ml of n-hexane were added in a test tube. Aqueous layer (0.5 ml) was taken; NaOH (0.5 ml) and 4 ml of distilled water were added. Enzyme activity was calculated by taking the absorbance at 400 nm (Nakamura et al. 1990).
One gram of soil was used to isolate the DNA from different soil samples by using the DNA Purification Kit (HiMedia). Purity of DNA was checked at 260 and 280 nm. qPCR was performed in an iCycler iQ™ Multicolor instrument using universal primers (EUB 341F-5′CCTACGGGAGGCAGCAG 3′ and EUB 534R-5′ATTACCGCGGCTGCTGG 3′) to quantify the 16S rRNA gene (Muyzer et al. 1993). Total volume of reaction mixture was 25 µl containing both primers (0.5 µl), SYBR green supermix (12.5 µl), and soil DNA (1 µl).
Statistical analysis was carried out using one-way analysis of variance (ANOVA) using SPSS software 16.0. Significant differences were calculated using Duncan’s test at p < 0.05. All analyses were made in triplicate.
Physiochemical analysis of the soil samples revealed significant variations in the chemical properties of treated soil samples. Soil pH showed variation in different treatments: T1 (7.2), T2 (7.4), T3 (7.5), T4 (7.44), T5 (7.6), T6 (7.9), T7 (7.65), T8 (7.7), and T9 (7.8). Different treatments showed enhanced level of total organic carbon, nitrogen, and phosphorus compared to control. Potassium was comparatively higher in T6 and T9 treatments (139.23 and 140.12 kg ha⁻1) in comparison to other treatments (Supplementary Material S3). Variations in soil physiochemical properties can have a significant impact on the microbial population and, as a result, plant growth (Sui et al., 2021). Nanocompounds can improve nutrient mobilization, chelation, and release slowly, which could assist with nutrient utilization efficiency (Chaudhary et al., 2021c). A significant link between accessible macronutrients and soil microbial flora was found in this study, indicating that nanocompounds have a good impact on soil health. The treated soil had high levels of organic carbon, nitrogen, phosphate, and potassium, which could greatly boost the beneficial microbial population in maize rhizosphere soil.
Improved bacterial count over control was observed in nanocompound-treated soil at 50 mg L−1 concentration on nutrient agar. Level of bacterial counts (CFU g−1) in different samples was 2.19 × 106 in T1, 2.42 × 106 in T2, 2.44 × 106 in T3, 2.43 × 106 in T4, 2.52 × 106 in T5, 2.56 × 106 in T6, 2.44 × 106 in T7, 2.52 × 106 in T8, and 2.53 × 106 in T9 after 60 days of sowing (Table 1). Nitrogen fixing bacterial count was higher in the combination of nanocompounds and bioinoculants while control had low N2 fixing population. Counts of phosphate were significantly better in treated soil over control. The order for phosphate solubilizers was control having 8.75 × 105 (T1), 1.01 × 106 (T2), 1.04 × 106 (T3), 1.08 × 106 (T4), 1.23 × 106 (T5), 1.20 × 106 (T6), 1.07 × 106 (T7), 1.13 × 106 (T8), and 1.17 × 106 (T9) treatment, respectively (Table 2). Potassium solubilizing bacterial counts was highest in T9 (8.80 × 105) treatment followed by T6 (8.00 × 105), T5 (7.63 × 105), T8 (7.40 × 105), T4 (7.00 × 105), T7 (6.96 × 105), T3 (6.90 × 105), and T2 (6.80 × 105) respectively. Bacterial counts were significantly better in treated soil compared to control. Application of nanocompounds with test bacterial cultures enhanced bacterial counts in the rhizospheric soil of maize. Number of bacteria per gram soil was high in treated soil over control. Similarly, bacterial population involved in NPK recycling was high when maize was given a combined treatment of nanocompounds and Bacillus spp. Presence of these bacteria improves soil quality by providing essential nutrients to soil and then to the plants. Aziz et al. (2016) reported that nano-formulations based on chitosan, zeolites, and clay, known to reduce the loss of nitrogen, had helped in enhancing nutrient uptake process in plant leaves. Pallavi et al. (2016) examined the impact of silver nanoparticle (50 mg L−1) concentration on total bacterial count, nitrogen fixers, and phosphorus solubilizers and found improved bacterial population in rhizospheric soil of Brassica juncea in India. Improved functional population of nitrogen fixers and potassium and phosphorus solubilizers was observed under the influence of SiO2 in maize soil, but ZnO, TiO2, and CeO2 (1 mg g−1) decreased the microbial count through uptake of free ions released by nanoparticles in soil (Chai et al., 2015). Chaudhary et al. (2021b) reported that application of nanocompounds enhanced beneficial bacterial population of maize rhizosphere soil using metagenomics. Biochar along with Bacillus megaterium improved the soil urease activity and NPK concentration (Ren et al. 2019). Toxic impact of ZnO and TiO2NPs (1–2 mg L−1) in microcosm on nitrogen fixing bacteria was observed using DNA-based fingerprinting (Ge et al., 2012). Total bacterial count and Azotobacter population were decreased when Cambisols treated with copper and zinc NPs (Kolesnikov et al., 2021). Bacterial consortium of Bacillus sp., Agrobacterium tumefaciens, and Pseudomonas sp. improved the NPK content in rhizosphere soil of wheat (Triticum) (Wang et al., 2020).
TABLE 1. Effect of nanocompounds and Bacillus spp. on total bacterial count and nitrogen fixers under maize cultivation.
TABLE 2. Effect of nanocompounds and Bacillus spp. on phosphate and potassium solubilizers under maize cultivation.
Soil of T5, T6, T8, and T9 treatments had highest activity of FDA hydrolysis, and the values of enzymes activity were 38.62, 40.58, 40.87, and 40.12 µg fluorescein g−1 h−1 followed by 31.24, 30.79, 30.70, and 30.6 2 µg fluorescein g−1 h−1 shown by T7, T3, T2, and T4 treatments, respectively, after 40 days of sowing. Minimum enzyme activity was observed in control (17.37 µg fluorescein g−1 h−1). A gradual increase in FDA hydrolysis with time was observed after 20, 40, and 60 days of the experiment in all the treatments (Figure 2). Microbial population and activities of soil enzymes are important parameters to measure the quality of a soil. Activities of different enzymes act as an indicator to identify changes in soil quality, measurement of microbial diversity, and community structure (Yang et al., 2017; Chaudhary et al., 2021b). Nanoparticles may influence the activity and immovability of microbial enzymes. So, it is important to measure the specific enzyme activity, which can be used to identify changes in the soil environment, if any. FDA hydrolysis level was twofold higher in treated soil samples over control. It indicates that protease, lipase, and esterase hydrolyzing bacterial population were enhanced by the application of nanocompounds and PGPR. The effect of nanochitosan, titanium oxide, and nanosilicon dioxide NPs was checked on soil enzymes, and a twofold increase in dehydrogenase and alkaline phosphatase was found (Kukreti et al., 2020; Kumari et al., 2020; Kumari et al., 2021). The presence of higher activity of FDA hydrolysis in treated soil might be correlated to availability of more substrate. The toxic effect of ZnO NPs on protease activity was due to dissolution of ions in treated soil when applied at the rate of 5 g in wheat soil (Du et al., 2011). Nanogypsum and Pseudomonas taiwanensis improved the soil enzyme activities by improving the nutrient status of soil reported by Chaudhary et al. (2021d).
FIGURE 2. Fluorescein diacetate hydrolysis, dehydrogenase, and alkaline phosphatase enzyme activities of soil in different treatments.
Combined treatment of nanocompounds along with bioinoculants showed twofold increase in dehydrogenase activity over control. T5, T6, T8, and T9 treatments showed maximum dehydrogenase activity and were in the range of 7.45, 7.65, 7.62, and 7.73 µg TPFg−1 h−1, followed by T3 (6.56), T4 (6.23), T7 (6.02), and T2 (5.97 µg TPFg−1 h−1) after 40 days of sowing. Enzyme activity was consistent up to the end of the experiment. Minimum enzyme activity was found in control (3.61 µg TPFg−1 h−1). Similarly, the highest phosphatase activity was observed in the treatment of nanocompounds with bioinoculants. The order of enzyme in the different treatments after 40 days was as follows: T1 (171.67), T2 (318.83), T3 (322), T4 (320.17), T5 (340.50), T6 (342.33), T7 (323), T8 (339), and T9 (344.83 µg pNP g−1 h−1) (Figure 2). Treated soil showed up to 2-fold increases in enzyme activity compared to control. Our results showed a significant increase in dehydrogenase activity after different time intervals. Dehydrogenase is an intercellular enzyme, present only in viable cells and very sensitive to pollutants/heavy metals (Trevors, 1984). Increase in activity might be due to the increase in metabolic activities of bacterial population. Awet et al. (2018) observed a toxic effect of polystyrene nanoparticles (100–1,000 ng) on dehydrogenase activity due to the decrease in microbial biomass. An increase in dehydrogenase activity of up to twofold was found in a glass container by applying the nano CuO (Josko et al., 2019). The positive effect of Cu is due to the fact that it is used as a cofactor for enzyme activity. Alkaline phosphatase, a soil indicator enzyme, is involved in enhancement of soil fertility by mineralization of phosphorus. A positive correlation was observed between phosphorus solubilizing bacteria and alkaline phosphatase activity in soil. An increase in phosphatase activity may be due to the presence of more phosphate solubilizing bacteria in treated soil over control. Enhancement in dehydrogenase activity by 108.7% and alkaline phosphatase by 72% as compared to control was observed by Raliya et al. (2015) in mung bean (Vigna radiata) in the presence of TiO2 (10 mg L−1). Tarafdar et al. (2013) reported significant improvement of rhizospheric microbial population and activities of acid and alkaline phosphatase and phytase in cluster bean rhizosphere when treated with ZnONPs (10 mg L−1). Application of nanophos, which consists of the phosphate solubilizing bacteria, also improved the different soil enzyme activities under maize cultivation (Chaudhary et al., 2021e). Silver NPs were not affected by the soil enzyme activities but decrease the actinobacterial population in tropical soil cultivated with Coffea arabica (Oca-Vasquez et al., 2020).
β-glucosidase activity was maximum in T8 and T9 treatments in the experimental soil throughout the experimental period. Twofold increases in glucosidase activity in all treated samples was observed over control (Figure 3). Amylase activity was highest (2.5 times than control) in T5 (140.90) and T6 (139.67 μg h−1), respectively, followed by T9 (130.67), T8 (128.13), T4 (122.67), T7 (121.10), T3 (119), and T2 (116.23 μg h−1). Least activity was observed in control (52.53 μg h−1). Level of β-glucosidase was also high in treated soil in the present study. This enzyme takes part in the carbon cycle, which points out the existence of a higher population of microbes in treated soil. More enzyme activity indicated the presence of a high population of microbes involved in cellulose degradation in treated soil. Eivazi et al. (2018) reported inhibition of β-glucosidase activity in soil by nano silver NPs (3,200 μg kg−1) over 1-month incubation. Li et al. (2017) reported that application of cerium oxide at different concentrations (100 and 500 mg kg−1) increased phosphatase activity significantly due to the antioxidant property of NPs, which helps in the improvement of cell lifespan and strength in the soil grass microcosm system but observed a negative effect on β-glucosidase activity due to the accumulation of reactive oxygen species.
FIGURE 3. β-glucosidase, amylase, and arylesterase enzyme activities of soil in different treatments.
Amylase enzyme is involved in the conversion of starch to glucose and maltose. The level of this enzyme was higher in treated soil, which indicates that bacterial population responsible for carbon cycle was also high than control. The higher level of arylesterase activity in treated soil in comparison to untreated soil may be related to degradation of organophosphates and polymers in soil. Untreated soil (T1) had the lowest level (49.11–52.55 μg h−1) of arylesterase activity in comparison to other treatments throughout the experiment. There was a twofold increase in enzyme activity from 20 days onwards in all the treatments (Figure 3). An increased level of enzyme activity is also a marker of action of microbes, that is, related to reprocessing of chemical elements by the enzymes (Tejada et al., 2006; Bastida et al., 2008). Our results revealed that nanocompounds along with bacterial culture did not have any toxic consequence on the soil enzyme activities. Cao et al. (2017) found that high concentration of iron oxide NPs (10 mg kg−1) had a negative effect on bacterial abundance, but Arbuscular Mycorrhizal Fungi treatment altered the effect of nanoparticles and improved maize growth and bacterial abundance in test soil. He et al. (2011) did not find any significant increase in population size when soil was treated with Fe2O3 and Fe3O4 (1.26 mg g−1). Mishra et al (2021) observed the toxic effect of silver NPs (100 mg kg−1) on soil arylamidase and phenol oxidase enzyme activities.
A steady increase in copy number of 16S rRNA was observed in T6 and T9 treatments until the end of the experiment. Abundance of 16S rRNA was 2.57 × 107 and 1.98 × 107 in T6 and T9 treatments, respectively. After 60 days, the pattern of abundance of total bacterial gene in other treatments was: T7 > T5 > T2 > T8 > T3 > T4 > T1, which showed 5.87 × 106 > 5.54 × 106 > 4.29 × 106 > 1.99 × 106 > 1.95 × 106 > 9.60 × 105 > 4.22 × 104, respectively, in terms of copy number (Table 3). Quantification of 16S rRNA showed high copy number in treated soil over control. This may be due to the positive effect of nanochitosan and nanozeolite on other bacterial populations, which helps in mobilization, chelation, and slow release of nutrients, improved the nutrient status of the soil, and enhanced plant growth (Alori et al., 2017; Agri et al., 2021). Titania nanoparticles and PGPR enhanced the valuable microorganism around roots and helped in the growth of wheat (Timmusk et al., 2018). Application of silver nanoparticles (0.01 mg kg−1) significantly reduced the population of ammonia oxidizers (−17%) but have a positive impact on the population of Bacteroidetes and Actinobacteria (Grun et al., 2019). Overall observation determined that enhanced level of enzymes and bacterial population supported the biological efficiency of nanocompounds along with bioinoculants on maize.
TABLE 3. Comparative 16S rRNA gene abundance at different sampling times as revealed by qPCR analysis.
The present study provides important implications of two nanocompounds on nutrient status, quality, and soil health if applied along with indigenous bioinoculants in maize. Application of combined treatments has potentially improved nutrient status, total microbial counts, nitrogen fixers, phosphorus, and potassium solubilizing bacteria in the experimental soil. Level of activities of signature soil enzymes was also improved in treated soil, which revealed that more nutrients are available to enhance the metabolic rate of soil bacteria. qPCR analysis also confirmed our observations as higher bacterial population in treated soil. The stimulation effect of nanocompounds was assumed to be increased due to better nutrient efficacy and survival of microbial population for longer duration by slow release of nutrients. The findings of the present study offer a possibility to use combined treatment of nanocompounds and bioinoculants in agricultural practices for better crop production as well as soil health.
The original contributions presented in the study are included in the article/Supplementary Material. Further inquiries can be directed to the corresponding author.
PC: Conceptualization, wrote the manuscript, and participated in all the experiments; AC, PB, and GK: Visualization and editing the manuscript; HK and AR: Editing the manuscript; SK: Helped in qPCR analysis; AS: Experimental design and provided the laboratory facilities.
The authors declare that the research was conducted in the absence of any commercial or financial relationships that could be construed as a potential conflict of interest.
All claims expressed in this article are solely those of the authors and do not necessarily represent those of their affiliated organizations, or those of the publisher, the editors, and the reviewers. Any product that may be evaluated in this article, or claim that may be made by its manufacturer, is not guaranteed or endorsed by the publisher.
The authors acknowledge the facilities provided by the Department of Microbiology and Agronomy, Govind Ballabh Pant University of Agriculture and Technology, Pantnagar.
The Supplementary Material for this article can be found online at: https://www.frontiersin.org/articles/10.3389/fenvs.2021.769871/full#supplementary-material
Adesemoye, A. O., and Kloepper, J. W. (2009). Plant-microbes Interactions in Enhanced Fertilizer-Use Efficiency. Appl. Microbiol. Biotechnol. 85, 1–12. doi:10.1007/s00253-009-2196-0
Agri, U., Chaudhary, P., and Sharma, A. (2021). In Vitro compatibility Evaluation of Agriusable Nanochitosan on Beneficial Plant Growth-Promoting Rhizobacteria and maize Plant. Natl. Acad. Sci. Lett. 44, 555–559. doi:10.1007/s40009-021-01047-w
Alori, E. T., Glick, B. R., and Babalola, O. O. (2017). Microbial Phosphorus Solubilization and its Potential for Use in Sustainable Agriculture. Front. Microbiol. 8, 971. doi:10.3389/fmicb.2017.00971
Awet, T. T., Kohl, Y., Meier, F., Straskraba, S., Grün, A.-L., Ruf, T., et al. (2018). Effects of Polystyrene Nanoparticles on the Microbiota and Functional Diversity of Enzymes in Soil. Environ. Sci. Eur. 30, 11. doi:10.1186/s12302-018-0140-6
Aziz, H. M. A., Hasaneen, M. N., and Omer, A. M. (2016). Nano Chitosan-NPK Fertilizer Enhances the Growth and Productivity of Wheat Plants Grown in sandy Soil. Spanish J. Agric. Res 14, 17. doi:10.5424/sjar/2016141-8205
Backer, R., Rokem, J. S., Ilangumaran, G., Lamont, J., Praslickova, D., Ricci, E., et al. (2018). Plant Growth-Promoting Rhizobacteria: Context, Mechanisms of Action, and Roadmap to Commercialization of Biostimulants for Sustainable Agriculture. Front. Plant Sci. 9, 473. doi:10.3389/fpls.2018.01473
Bargaz, A., Lyamlouli, K., Chtouki, M., Zeroual, Y., and Dhiba, D. (2018). Soil Microbial Resources for Improving Fertilizers Efficiency in an Integrated Plant Nutrient Management System. Front. Microbiol. 9, 1606. doi:10.3389/fmicb.2018.01606
Bastida, F., Zsolnay, A., Hernández, T., and García, C. (2008). Past, Present and Future of Soil Quality Indices: a Biological Perspective. Geoderma 147, 159–171. doi:10.1016/j.geoderma.2008.08.007
Beneduzi, A., Ambrosini, A., and Passaglia, L. M. P. (2012). Plant Growth-Promoting Rhizobacteria (PGPR): Their Potential as Antagonists and Biocontrol Agents. Genet. Mol. Biol. 35, 1044–1051. doi:10.1590/s1415-47572012000600020
Bernfeld, P. (1951). Enzymes of Starch Degradation and Synthesis. Adv. Enzymol. Relat. Subject Biochem. 12, 379–428. doi:10.1002/9780470122570.ch7
Bünemann, E. K., Bongiorno, G., Bai, Z., Creamer, R. E., De Deyn, G., de Goede, R., et al. (2018). Soil Quality - A Critical Review. Soil Biol. Biochem. 120, 105–125. doi:10.1016/j.soilbio.2018.01.030
C. A. Black (Editor) (1965). Methods of Soil Analysis. Part 2, Chemical and Microbiological Properties (Madison, Wisconsin USA: American Society of Agronomy. Inc, Publisher).
Cao, J., Feng, Y., Lin, X., Wang, J., and Xie, X. (2017). Iron Oxide Magnetic Nanoparticles Deteriorate the Mutual Interaction between Arbuscular Mycorrhizal Fungi and Plant. J. Soils Sediments 17, 841–851. doi:10.1007/s11368-016-1561-8
Casida, L. E., Klein, D. A., and Santoro, T. (1964). Soil Dehydrogenase Activity. Soil Sci. 98, 371–376. doi:10.1097/00010694-196412000-00004
Chai, H., Yao, J., Sun, J., Zhang, C., Liu, W., Zhu, M., et al. (2015). The Effect of Metal Oxide Nanoparticles on Functional Bacteria and Metabolic Profiles in Agricultural Soil. Bull. Environ. Contam. Toxicol. 94, 490–495. doi:10.1007/s00128-015-1485-9
Chaudhary, A., Parveen, H., Chaudhary, P., Khatoon, H., and Bhatt, P. (2021). “Rhizospheric Microbes and Their Mechanism,” in Microbial Technology for Sustainable Environment. Editors P. Bhatt, S. Gangola, D. Udayanga, and G. Kumar (Singapore: Springer), 79–93. doi:10.1007/978-981-16-3840-4_6
Chaudhary, P., Chaudhary, A., Parveen, H., Rani, A., Kumar, G., Kumar, R., et al. (2021e). Impact of Nanophos in Agriculture to Improve Functional Bacterial Community and Crop Productivity. BMC Plant Biol. 21, 519. doi:10.1186/s12870-021-03298-7
Chaudhary, P., Khati, P., Chaudhary, A., Gangola, S., Kumar, R., and Sharma, A. (2021a). Bioinoculation Using Indigenous Bacillus Spp. Improves Growth and Yield of Zea mays under the Influence of Nanozeolite. 3 Biotech. 11, 11. doi:10.1007/s13205-020-02561-2
Chaudhary, P., Khati, P., Chaudhary, A., Maithani, D., Kumar, G., and Sharma, A. (2021d). Cultivable and Metagenomic Approach to Study the Combined Impact of Nanogypsum and Pseudomonas Taiwanensis on maize Plant Health and its Rhizospheric Microbiome. PLoS ONE 16, e0250574. doi:10.1371/journal.pone.0250574
Chaudhary, P., Khati, P., Gangola, S., Kumar, A., Kumar, R., and Sharma, A. (2021c). Impact of Nanochitosan and Bacillus Spp. On Health, Productivity and Defence Response in Zea mays under Field Condition. 3 Biotech. 11, 237. doi:10.1007/s13205-021-02790-z
Chaudhary, P., Parveen, H., Gangola, S., Kumar, G., Bhatt, P., and Chaudhary, A. (2021). “Plant Growth-Promoting Rhizobacteria and Their Application in Sustainable Crop Production,” in Microbial Technology for Sustainable Environment. Editors P. Bhatt, S. Gangola, D. Udayanga, and G. Kumar (Singapore: Springer), 217–234. doi:10.1007/978-981-16-3840-4_13
Chaudhary, P., Sharma, A., Chaudhary, A., Khati, P., Gangola, S., and Maithani, D. (2021b). Illumina Based High Throughput Analysis of Microbial Diversity of maize Rhizosphere Treated with Nanocompounds and Bacillus Sp. Appl. Soil Ecol. 159, 103836. doi:10.1016/j.apsoil.2020.103836
Chaudhary, P., and Sharma, A. (2019). Response of Nanogypsum on the Performance of Plant Growth Promotory Bacteria Recovered from Nanocompound Infested Agriculture Field. Environ. Ecol. 37, 363–372.
Chavan, S., and Nadanathangam, V. (2019). Effects of Nanoparticles on Plant Growth-Promoting Bacteria in Indian Agricultural Soil. Agronomy 9, 140. doi:10.3390/agronomy9030140
Desbrosses, G. J., and Stougaard, J. (2011). Root Nodulation: a Paradigm for How Plant-Microbe Symbiosis Influences Host Developmental Pathways. Cell Host & Microbe 10, 348–358. doi:10.1016/j.chom.2011.09.005
Du, W., Sun, Y., Ji, R., Zhu, J., Wu, J., and Guo, H. (2011). TiO2 and ZnO Nanoparticles Negatively Affect Wheat Growth and Soil Enzyme Activities in Agricultural Soil. J. Environ. Monit. 13, 822–828. doi:10.1039/C0EM00611D
Duhan, J. S., Kumar, R., Kumar, N., Kaur, P., Nehra, K., and Duhan, S. (2017). Nanotechnology: The New Perspective in Precision Agriculture. Biotechnol. Rep. 15, 11–23. doi:10.1016/j.btre.2017.03.002
Eivazi, F., Afrasiabi, Z., and Jose, E. (2018). Effects of Silver Nanoparticles on the Activities of Soil Enzymes Involved in Carbon and Nutrient Cycling. Pedosphere 28, 209–214. doi:10.1016/s1002-0160(18)60019-0
FAO (2012). Food and Agriculture Organization of the United Nations, Agriculture and Consumer Protection Department. Rome, Italy: Conservation Agriculture.
Ge, Y., Schimel, J. P., and Holden, P. A. (2012). Evidence for Negative Effects of TiO2 and ZnO Nanoparticles on Soil Bacterial Communities. Environ. Sci. Technol 45, 1659–1664. doi:10.1021/es103040t
Ghormade, V., Deshpande, M. V., and Paknikar, K. M. (2011). Perspectives for Nano-Biotechnology Enabled protection and Nutrition of Plants. Biotechnol. Adv. 29, 792–803. doi:10.1016/j.biotechadv.2011.06.007
Griffiths, R. I., Whiteley, A. S., O'Donnell, A. G., and Bailey, M. J. (2000). Rapid Method for Coextraction of DNA and RNA from Natural Environments for Analysis of Ribosomal DNA- and rRNA-Based Microbial Community Composition. Appl. Environ. Microbiol. 66, 5488–5491. doi:10.1128/AEM.66.12.5488-5491.2000
Grün, A.-L., Manz, W., Kohl, Y. L., Meier, F., Straskraba, S., Jost, C., et al. (2019). Impact of Silver Nanoparticles (AgNP) on Soil Microbial Community Depending on Functionalization, Concentration, Exposure Time, and Soil Texture. Environ. Sci. Eur. 31, 15. doi:10.1186/s12302-019-0196-y
Gupta, S., and Pandey, S. (2019). ACC Deaminase Producing Bacteria with Multifarious Plant Growth Promoting Traits Alleviates Salinity Stress in French Bean (Phaseolus vulgaris) Plants. Front. Microbiol. 10, 1506. doi:10.3389/fmicb.2019.01506
He, S., Feng, Y., Ren, H., Zhang, Y., Gu, N., and Lin, X. (2011). The Impact of Iron Oxide Magnetic Nanoparticles on the Soil Bacterial Community. J. Soils Sediments 11, 1408–1417. doi:10.1007/s11368-011-0415-7
Jacoby, R., Peukert, M., Succurro, A., Koprivova, A., and Kopriva, S. (2017). The Role of Soil Microorganisms in Plant Mineral Nutrition-Current Knowledge and Future Directions. Front. Plant Sci. 8, 1617. doi:10.3389/fpls.2017.01617
Jahanian, A., Chaichi, M. R., Rezaei, K., Rezayazdi, K., and Khavazi, K. (2012). The Effect of Plant Growth Promoting Rhizobacteria (Pgpr) on Germination and Primary Growth of Artichoke (Cynara Scolymus). Int. J Agric. Crop Sci 4, 923–929.
Jośko, I., Oleszczuk, P., Dobrzyńska, J., Futa, B., Joniec, J., and Dobrowolski, R. (2019). Long-term Effect of ZnO and CuO Nanoparticles on Soil Microbial Community in Different Types of Soil. Geoderma 352, 204–212. doi:10.1016/j.geoderma.2019.06.010
Ju, W., Liu, L., Fang, L., Cui, Y., Duan, C., and Wu, H. (2019). Impact of Co-inoculation with Plant-Growth-Promoting Rhizobacteria and Rhizobium on the Biochemical Responses of Alfalfa-Soil System in Copper Contaminated Soil. Ecotoxicology Environ. Saf. 167, 218–226. doi:10.1016/j.ecoenv.2018.10.016
Katiyar, D., Hemantaranjan, A., and Singh, B. (2015). Chitosan as a Promising Natural Compound to Enhance Potential Physiological Responses in Plant: a Review. Ind. J. Plant Physiol. 20, 1–9. doi:10.1007/s40502-015-0139-6
Khati, P., Chaudhary, P., Gangola, S., Bhatt, P., and Sharma, A. (2017). Nanochitosan Supports Growth of Zea mays and Also Maintains Soil Health Following Growth. 3 Biotech. 7, 81. doi:10.1007/s13205-017-0668-y
Khati, P., Chaudhary, P., Gangola, S., and Sharma, P. (2019a). Influence of Nanozeolite on Plant Growth Promotory Bacterial Isolates Recovered from Nanocompound Infested Agriculture Field. Environ. Ecol. 37, 521–527.
Khati, P., ParulBhatt, P., BhattKumar, P., Nisha, A., Kumar, R., and Sharma, A. (2018). Effect of Nanozeolite and Plant Growth Promoting Rhizobacteria on maize. 3 Biotech. 8, 141. doi:10.1007/s13205-018-1142-1
Khati, P., Sharma, A., Chaudhary, P., Singh, A. K., Gangola, S., and Kumar, R. (2019b). High-throughput Sequencing Approach to Access the Impact of Nanozeolite Treatment on Species Richness and Evenness of Soil Metagenome. Biocatal. Agric. Biotechnol. 20, 101249. doi:10.1016/j.bcab.2019.101249
Kolesnikov, S., Timoshenko, A., Minnikova, T., Tsepina, N., Kazeev, K., Akimenko, Y., et al. (2021). Impact of Metal-Based Nanoparticles on Cambisol Microbial Functionality, Enzyme Activity, and Plant Growth. Plants 10, 2080. doi:10.3390/plants10102080
Kour, D., Rana, K. L., Yadav, A. N., Sheikh, I., Kumar, V., Dhaliwal, H. S., et al. (2020a). Amelioration of Drought Stress in Foxtail Millet (Setaria Italica L.) by P-Solubilizing Drought-Tolerant Microbes with Multifarious Plant Growth Promoting Attributes. Environ. Sustainability 3, 23–34. doi:10.1007/s42398-020-00094-1
Kour, D., Rana, K. L., Yadav, A. N., Yadav, N., Kumar, M., Kumar, V., et al. (2020b). Microbial Biofertilizers: Bioresources and Eco-Friendly Technologies for Agricultural and Environmental Sustainability. Biocatal. Agric. Biotechnol. 23, 101487. doi:10.1016/j.bcab.2019.101487
Kukreti, B., Sharma, A., Chaudhary, P., Agri, U., and Maithani, D. (2020). Influence of Nanosilicon Dioxide along with Bioinoculants on Zea mays and its Rhizospheric Soil. 3 Biotech. 10, 345. doi:10.1007/s13205-020-02329-8
Kumar, A., Sharma, A., Chaudhary, P., and Gangola, S. (2021). Chlorpyrifos Degradation Using Binary Fungal Strains Isolated from Industrial Waste Soil. Biologia 76, 3071–3080. doi:10.1007/s11756-021-00816-8
Kumari, H., Khati, P., Gangola, S., Chaudhary, P., and Sharma, A. (2021). Performance of Plant Growth Promotory Rhizobacteria on maize and Soil Characteristics under the Influence of TiO2 Nanoparticles. Pantnagar J. Res 19, 28–39.
Kumari, S., Sharma, A., Chaudhary, P., and Khati, P. (2020). Management of Plant Vigor and Soil Health Using Two Agriusable Nanocompounds and Plant Growth Promotory Rhizobacteria in Fenugreek. 3 Biotech. 10, 461. doi:10.1007/s13205-020-02448-2
Li, B., Chen, Y., Liang, W.-z., Mu, L., Bridges, W. C., Jacobson, A. R., et al. (2017). Influence of Cerium Oxide Nanoparticles on the Soil Enzyme Activities in a Soil-Grass Microcosm System. Geoderma 299, 54–62. doi:10.1016/j.geoderma.2017.03.027
Li, J., Luo, Z., Zhang, C., Qu, X., Chen, M., Song, T., et al. (2020). Seasonal Variation in the Rhizosphere and Non-rhizosphere Microbial Community Structures and Functions of Camellia Yuhsienensis Hu. Microorganisms 8 (9), 1385. doi:10.3390/microorganisms8091385
Liu, D., Yang, Q., Ge, K., Hu, X., Qi, G., Du, B., et al. (2017). Promotion of Iron Nutrition and Growth on Peanut by Paenibacillus Illinoisensis and Bacillus Sp. Strains in Calcareous Soil. Braz. J. Microbiol. 48, 656–670. doi:10.1016/j.bjm.2017.02.006
Lopez-Monejar, R., Vorískov, A. J., Vetrovský, T., and Baldrian, P. (2015). The Bacterial Community Inhabiting Temperate Deciduous Forests Is Vertically Stratified and Undergoes Seasonal Dynamics. Soil Biol. Biochem. 87, 43–50.
Messer, J. W., and Johnson, C. H. (2000). “Total Viable Counts. Pour Plate Technique,”. Encyclopedia of Food Microbiology. Editors R. K. Robinson, C. A. Batt, and P. D. Patel (London: Academic Press), Vol. 3, 2154–2158.
Ming, D. W., and Allen, E. R. (2001). Use of Natural Zeolites in Agronomy, Horticulture and Environmental Soil Remediation. Rev. Mineralogy Geochem. 45, 619–654. doi:10.2138/rmg.2001.45.18
Mishra, P., Xue, Y., Eivazi, F., and Afrasiabi, Z. (2021). Size, Concentration, Coating, and Exposure Time Effects of Silver Nanoparticles on the Activities of Selected Soil Enzymes. Geoderma 381, 114682. doi:10.1016/j.geoderma.2020.114682
Montes de Oca-Vásquez, G., Solano-Campos, F., Vega-Baudrit, J. R., López-Mondéjar, R., Vera, A., Moreno, J. L., et al. (2020). Organic Amendments Exacerbate the Effects of Silver Nanoparticles on Microbial Biomass and Community Composition of a Semiarid Soil. Sci. Total Environ. 744, 140919. doi:10.1016/j.scitotenv.2020.140919
M. Tahat, M., M. Alananbeh, K., A. Othman, Y., and I. Leskovar, D. (2020). Soil Health and Sustainable Agriculture. Sustainability 12 (12), 4859. doi:10.3390/su12124859
Muyzer, G., DeWaal, E. C., and Uitterlinden, A. G. (1993). Profiling of Complex Microbial Populations by Denaturing Gradient Gel Electrophoresis Analysis of Polymerase Chain Reaction-Amplified Genes Coding for 16S rRNA. Appl. Environ. Microbiol. 59, 695–700. doi:10.1128/AEM.59.3.695-700.1993
Nakamura, T., Mochida, K., Ozoe, Y., Ukawa, S., Sakai, M., and Mitsugi, S. (1990). Enzymological Properties of Three Soil Hydrolases and Effects of Several Pesticides on Their Activities. J. Pestic. Sci. 15, 593–598. doi:10.1584/jpestics.15.593
Pallavi, , Mehta, C. M., Srivastava, R., Arora, S., and Sharma, A. K. (2016). Impact Assessment of Silver Nanoparticles on Plant Growth and Soil Bacterial Diversity. 3 Biotech. 6, 254. doi:10.1007/s13205-016-0567-7
Popova, E. V., Domnina, N. S., Kovalenko, N. M., Sokornova, S. V., and Tyuterev, S. L. (2016). Effect of Chitosan and Vanillin-Modified Chitosan on Wheat Resistance to Spot Blotch. Appl. Biochem. Microbiol. 52, 537–540. doi:10.1134/S0003683816050124
Prasad, R., Bhattacharyya, A., and Nguyen, Q. D. (2017). Nanotechnology in Sustainable Agriculture: Recent Developments, Challenges, and Perspectives. Front. Microbiol. 8, 1014. doi:10.3389/fmicb.2017.01014
Raliya, R., Biswas, P., and Tarafdar, J. C. (2015). TiO2 Nanoparticle Biosynthesis and its Physiological Effect on Mung Bean (Vigna Radiata L.). Biotechnol. Rep. 5, 22–26. doi:10.1016/j.btre.2014.10.009
Ramesh, K., and Reddy, D. D. (2011). Zeolites and Their Potential Uses in Agriculture, Adv. Agron., 113, 219–241. doi:10.1016/b978-0-12-386473-4.00004-x
Ren, C., Chen, J., Deng, J., Zhao, F., Han, X., Yang, G., et al. (2017). Response of Microbial Diversity to C:N:P Stoichiometry in fine Root and Microbial Biomass Following Afforestation. Biol. Fertil. Soils 53, 457–468. doi:10.1007/s00374-017-1197-x
Rousk, J., and Bengtson, P. (2014). Microbial Regulation of Global Biogeochemical Cycles. Front. Microbiol. 5, 103. doi:10.3389/fmicb.2014.00103
Sadak, M. S. (2019). Impact of Silver Nanoparticles on Plant Growth, Some Biochemical Aspects, and Yield of Fenugreek Plant (Trigonella Foenum-graecum). Bull. Natl. Res. Cent. 43, 38. doi:10.1186/s42269-019-0077-y
Santoyo, G., Orozco-Mosqueda, M. d. C., and Govindappa, M. (2012). Mechanisms of Biocontrol and Plant Growth-Promoting Activity in Soil Bacterial Species ofBacillusandPseudomonas: a Review. Biocontrol Sci. Tech. 22, 855–872. doi:10.1080/09583157.2012.694413
Schnürer, J., and Rosswall, T. (1982). Fluorescein Diacetate Hydrolysis as a Measure of Total Microbial Activity in Soil and Litter. Appl. Environ. Microbiol. 43, 1256–1261. doi:10.1128/AEM.43.6.1256-1261.1982
Schütz, L., Gattinger, A., Meier, M., Müller, A., Boller, T., Mäder, P., et al. (2018). Improving Crop Yield and Nutrient Use Efficiency via Biofertilization-A Global Meta-Analysis. Front. Plant Sci. 8, 2204. doi:10.3389/fpls.2017.02204
Sharma, S. B., Sayyed, R. Z., Trivedi, M. H., and Gobi, T. A. (2013). Phosphate Solubilizing Microbes: Sustainable Approach for Managing Phosphorus Deficiency in Agricultural Soils. SpringerPlus 2, 587. doi:10.1186/2193-1801-2-587
Siddaiah, C. N., Prasanth, K. V. H., Satyanarayana, N. R., Mudili, V., Gupta, V. K., Kalagatur, N. K., et al. (2018). Chitosan Nanoparticles Having Higher Degree of Acetylation Induce Resistance against Pearl Millet Downy Mildew through Nitric Oxide Generation. Sci. Rep. 8, 2485. doi:10.1038/s41598-017-19016-z
Sui, X., Zhang, R., Frey, B., Yang, L., Liu, Y., Ni, H., et al. (2021). Soil Physicochemical Properties Drive the Variation in Soil Microbial Communities along a forest Successional Series in a Degraded Wetland in Northeastern China. Ecol. Evol. 11 (5), 2194–2208. doi:10.1002/ece3.7184
Tabatabai, M. A., and Bremner, J. M. (1969). Use of P-Nitrophenyl Phosphate for Assay of Soil Phosphatase Activity. Soil Biol. Biochem. 1, 301–307. doi:10.1016/0038-0717(69)90012-1
Tabatabai, M. A. (1994). “Soil Enzymes,” in Methods of Soil Analysis, Part 2. Microbiological and Biochemical Properties. Editors R. W. Weaver, J. S. Angle, and P. S. Bottomley (Madison: Soil Science Society of America), 775–833. doi:10.2136/sssabookser5.2.c37
Tarafdar, J. C., Shikha, S., and Ramesh, R. (2013). Nanotechnology: Interdisciplinary Science of Applications. Afr. J. Biotechnol. 12, 219–226. doi:10.5897/AJB12.2481
Tejada, M., Hernandez, M. T., and Garcia, C. (2006). Application of Two Organic Amendments on Soil Restoration: Effects on the Soil Biological Properties. J. Environ. Qual. 35, 1010–1017. doi:10.2134/jeq2005.0460
Timmusk, S., Behers, L., Muthoni, J., Muraya, A., and Aronsson, A.-C. (2017). Perspectives and Challenges of Microbial Application for Crop Improvement. Front. Plant Sci. 8, 49. doi:10.3389/fpls.2017.00049
Timmusk, S., Seisenbaeva, G., and Behers, L. (2018). Titania (TiO2) Nanoparticles Enhance the Performance of Growth-Promoting Rhizobacteria. Sci. Rep. 8, 617. doi:10.1038/s41598-017-18939-x
Trevors, J. T. (1984). Effect of Substrate Concentration, Inorganic Nitrogen, O2 Concentration, Temperature and pH on Dehydrogenase Activity in Soil. Plant Soil 77, 285–293. doi:10.1007/BF02182931
Vacheron, J., Desbrosses, G., Bouffaud, M.-L., Touraine, B., Moënne-Loccoz, Y., Muller, D., et al. (2013). Plant Growth-Promoting Rhizobacteria and Root System Functioning. Front. Plant Sci. 4, 356. doi:10.3389/fpls.2013.00356
Vejan, P., Abdullah, R., Khadiran, T., Ismail, S., and NasrulhaqBoyce, A. (2016). Role of Plant Growth Promoting Rhizobacteria in Agricultural Sustainability-A Review. Molecules 21, 573. doi:10.3390/molecules21050573
Vessey, J. K. (2003). Plant Growth Promoting Rhizobacteria as Biofertilizers. Plant and Soil 255, 571–586. doi:10.1023/A:1026037216893
Wang, J., Li, R., Zhang, H., Wei, G., and Li, Z. (2020). Beneficial Bacteria Activate Nutrients and Promote Wheat Growth under Conditions of Reduced Fertilizer Application. BMC Microbiol. 20, 38. doi:10.1186/s12866-020-1708-z
Yang, S., Xu, Z., Wang, R., Zhang, Y., Yao, F., Zhang, Y., et al. (2017). Variations in Soil Microbial Community Composition and Enzymatic Activities in Response to Increased N Deposition and Precipitation in Inner Mongolian Grassland. Appl. Soil Ecol. 119, 275–285. doi:10.1016/j.apsoil.2017.06.041
Yang, X., Chen, L., Yong, X., and Shen, Q. (2011). Formulations Can Affect Rhizosphere Colonization and Biocontrol Efficiency of Trichoderma harzianum SQR-T037 against Fusarium Wilt of Cucumbers. Biol. Fertil. Soils 47, 239–248. doi:10.1007/s00374-010-0527-z
Yuvaraj, M., and Subramanian, K. S. (2018). Development of Slow Release Zn Fertilizer Using Nano-Zeolite as Carrier. J. Plant Nutr. 41, 311–320. doi:10.1080/01904167.2017.1381729Available From: https://doi.org/10.1080/01904167.2017.1381729
Keywords: soil enzymes, Bacillus spp., nanocompounds, qPCR, soil health
Citation: Chaudhary P, Chaudhary A, Bhatt P, Kumar G, Khatoon H, Rani A, Kumar S and Sharma A (2022) Assessment of Soil Health Indicators Under the Influence of Nanocompounds and Bacillus spp. in Field Condition. Front. Environ. Sci. 9:769871. doi: 10.3389/fenvs.2021.769871
Received: 03 September 2021; Accepted: 30 November 2021;
Published: 07 January 2022.
Edited by:
Jesús Rodrigo-Comino, University of Granada, SpainReviewed by:
Ajar Nath Yadav, Eternal University, IndiaCopyright © 2022 Chaudhary, Chaudhary, Bhatt, Kumar, Khatoon, Rani, Kumar and Sharma. This is an open-access article distributed under the terms of the Creative Commons Attribution License (CC BY). The use, distribution or reproduction in other forums is permitted, provided the original author(s) and the copyright owner(s) are credited and that the original publication in this journal is cited, in accordance with accepted academic practice. No use, distribution or reproduction is permitted which does not comply with these terms.
*Correspondence: Parul Chaudhary, cGFydWxjaGF1ZGhhcnkxNDIzQGdtYWlsLmNvbQ==
Disclaimer: All claims expressed in this article are solely those of the authors and do not necessarily represent those of their affiliated organizations, or those of the publisher, the editors and the reviewers. Any product that may be evaluated in this article or claim that may be made by its manufacturer is not guaranteed or endorsed by the publisher.
Research integrity at Frontiers
Learn more about the work of our research integrity team to safeguard the quality of each article we publish.