- 1Institute of Ecology and Evolution, Russian Academy of Sciences, Moscow, Russia
- 2Department of Hydrobiology, Faculty of Biology, University of Białystok, Białystok, Poland
- 3Institute of Biophysics of Federal Research Centre, Krasnoyarsk Science Centre of Siberian Branch of Russian Academy of Sciences, Krasnoyarsk, Russia
- 4Chair of Aquatic and Terrestrial Ecosystems, Siberian Federal University, Krasnoyarsk, Russia
- 5Papanin Institute for Biology of Inland Waters, Russian Academy of Sciences, Borok, Russia
- 6Department of Integrative Biology, Oklahoma State University, Stillwater, OK, United States
The transfer pathways of organic matter and elements from phytoplankton to zooplankton in freshwater ecosystems are important for understanding how aquatic ecosystems function. We conducted a mesocosm experiment to determine how fish and zebra mussels altered the transfer efficiencies of essential substances including carbon (C), polyunsaturated fatty acids (PUFAs), total fatty acids (FAs), phosphorus (P), and nitrogen (N) from phytoplankton to zooplankton. We assessed the transfer efficiencies of the essential substances from phytoplankton to zooplankton as the ratio of their zooplankton production (P) per unit of biomass (B) to that of phytoplankton to exclude grazing or predation effects. We hypothesized that zebra mussels and fish would affect the transfer of materials from phytoplankton to zooplankton by altering the contents of essential elements and FAs in phytoplankton and zooplankton communities and/or due to shifts in the planktonic community structure mediated by grazing and/or predation. Fish increased the transfer efficiencies of eicosapentaenoic acid 20:5 ω-3 (EPA), docosahexaenoic acid 22:6 ω-3 (DHA), and P relative to the control. We speculated that fish weakened the control of zooplankton over algal assemblage by selectively feeding on larger cladocerans such as Daphnia. Therefore, fish can increase the relative proportion of high-quality food for zooplankton, improving food conditions for the available zooplankton. In contrast, zebra mussels reduced the transfer efficiencies of EPA and DHA relative to the control treatment likely due to competition with zooplankton for PUFA-rich food particles. However, zebra mussels did not have any impact on the transfer efficiencies of C, total FAs, N, and P. EPA, DHA, and P were transferred more efficiently than C from phytoplankton to zooplankton, while total FAs, which are commonly used as an energetic source, were transferred as efficiently as C. The enrichment of consumers with the most important substances relative to their basal food sources creates the potential for the successful transport of these substances across aquatic trophic webs.
Introduction
Zooplankton production is expected to be lower than phytoplankton production because energy is lost when it is transferred from phytoplankton to zooplankton. The efficiency of carbon (C) transfer from phytoplankton to zooplankton measured as a ratio of secondary production/primary production (Gladyshev et al., 2011a) can vary between 5 and 30% (Lacroix, 1999). The flow of C from producers to consumers in aquatic ecosystems is highly variable and dependent on environmental factors. At a given biomass of phytoplankton, efficient ecosystems (e.g., marine upwelling zones) can support twenty-five times more biomass of zooplankton than inefficient ecosystems such as hypereutrophic lakes (Brett and Müller-Navarra, 1997; Karpowicz et al., 2021). However, it remains unclear how different environmental factors influence the rates at which primary production is converted to zooplankton biomass.
In addition to C, there are essential substances including polyunsaturated fatty acids (PUFAs), nitrogen (N), and phosphorus (P) that are transported from phytoplankton to zooplankton. These substances are important in the metabolic processes of zooplankton. For example, nutrients are responsible for the synthesis of major macromolecules such as lipids, proteins, and nucleic acids (Prater et al., 2018), while fatty acids (FAs) are used as the structural components of cellular membranes and storage of lipids and/or act as important precursors for regulatory compounds, such as eicosanoid hormones (Hessen and Leu, 2006; Jardine et al., 2020). FAs, including PUFAs, are crucial for maintaining growth rates, survival, and reproduction of freshwater animals (Brett and Müller-Navarra, 1997). PUFAs are synthesized mainly by some species of microalgae which then supply them to higher trophic levels (Uttaro, 2006; Lands, 2009). Although aquatic invertebrates are now recognized as net producers of long-chain omega-3 PUFAs due to the genetic code to synthesize PUFAs de novo (Kabeya et al., 2018), phytoplankton remain the main producers of PUFAs.
The content of these essential substances in algae determines the quality of phytoplankton as a food resource for zooplankton (Sterner and Schulz, 1998; Wacker and von Elert, 2001; Becker and Boersma, 2005; Gladyshev et al., 2006, 2007). It is noteworthy that PUFAs are more critical for zooplankton physiological processes than short-chain FAs (Brett and Müller-Navarra, 1997; Brett et al., 2006). Although the biochemical and elemental compositions of phytoplankton and zooplankton differ considerably (Brett and Müller-Navarra, 1997; Hessen and Leu, 2006), the chemical compositions of zooplankton and their diets are partly interrelated (Napolitano, 1999; Becker and Boersma, 2005). For example, von Elert (2002) found that when Daphnia galeata were fed diets artificially enriched with eicosapentaenoic acid 20:5 ω-3 (EPA) and docosahexaenoic acid 22:6 ω-3 (DHA), they also became enriched with these FAs. Nevertheless, the chemical composition of zooplankton can also be modified relative to their diets (Kainz et al., 2004; Brett et al., 2006; Galloway and Budge, 2020; Jardine et al., 2020). In particular, PUFAs, including EPA and DHA, can be retained or accumulated more efficiently than short-chain FAs (Kainz et al., 2004). Twining et al. (2020) indicated that still little is known about how essential FAs are processed by consumers when being incorporated. The enzymatic processes required to convert precursors to PUFAs often involve a series of elongation and desaturation processes. Therefore, the chemical composition of consumers can be considerably different from that in their diets.
Hessen and Leu (2006) suggested that grazers have evolved a set of metabolic strategies to concentrate those elements that are deficient and dispose those which are in excess. The ability of zooplankton to accumulate substances that are in shortage and excrete substances that are in excess enables them to maintain their growth and production rates (Schoo et al., 2013). One of the mechanisms that zooplankton use to maintain an appropriate chemical structure is to convert different substances in their diets with different efficiencies to their own biomass. Gladyshev et al. (2011a) found that the transfer efficiencies of essential PUFAs from producers to primary consumers were about twice as high as the transfer efficiency for bulk C in a eutrophic reservoir. The high transfer efficiency of essential substances increases the quality of planktonic zooplankton as a food resource for higher trophic levels.
Macrobiota, including fish and zebra mussels, can affect planktonic algae, thus altering the efficiencies of essential substances from phytoplankton to zooplankton. For example, planktivoros fish commonly reduce the biomass of filter-feeding zooplankton through predation, thus indirectly increasing algal abundance (Semenchenko et al., 2007). Fish also excrete nutrients into the water column that stimulate algal growth of some taxa, thus altering species composition (Brabrand et al., 1984). Vanni and Layne (1997) suggested that nutrient recycling by fish has important consequences for the community-level response of phytoplankton. Specifically, several phytoplankton taxa showed enhanced biomass in treatments in which phytoplankton were exposed to nutrient excretion by fish. However, these impacts of recycling and community shifts via grazing and predation are hard to disentangle. Therefore, we can deal only with the pooled impacts of these interactions.
Zebra mussels can also alter the nutritional quality of algal resources. In particular, zebra mussels can increase the algal nutrient content by excreting N and P into the water column (Wilson, 2003; Wojtal-Frankiewicz and Frankiewicz, 2011; Feniova et al., 2015). Zebra mussels can also selectively consume phytoplankton through grazing. For example, they decrease the EPA content in seston by selectively grazing on EPA-rich seston (Makhutova et al., 2013). Since macrobiota have a great impact on phytoplankton and zooplankton, they can change the transport efficiencies of essential substances from phytoplankton to zooplankton.
We manipulated the presence/absence of zebra mussels and fish in a series of mesocosm experiments to determine how macrobiota affected the transfer efficiencies of essential substances from phytoplankton to zooplankton. We focused on zebra mussels and fish because of their abilities to influence phytoplankton and zooplankton in lakes, both directly and indirectly through consumption and nutrient cycling. The transfer efficiencies were measured as a production factor (zooplankton:phytoplankton ratio) based on production per biomass unit (P/B ratio) which is an appropriate basis for the comparison of the production potential of various invertebrate communities (Ikeda and Shiga, 1999; Ikeda et al., 2002). We conducted two experiments using water containing natural plankton with the addition of large Daphnia from the eutrophic Lake Mikołajskie (Masurian Lake District, northeastern Poland) in 2017 and 2018. We manipulated the presence/absence of zebra mussels in the 2017 experiment and fish in the 2018 experiment. The goal of our study was to determine how the transfer efficiency of essential substances, measured as zooplankton:phytoplankton P/B ratios, responded to the presence of macrobiota (zebra mussel and fish) under eutrophic conditions. We studied eutrophic conditions because eutrophication is a great threat to aquatic ecosystems. It is known that high trophic levels and nutrient enrichment are associated with cyanobacterial blooms (Heisler et al., 2008). Lacroix (1999) showed based on the analysis of 56 lakes of different trophic statuses that the efficiencies of matter transport decreased with the increase in trophic status. In this view, it is especially important to understand how macrobiota affect matter flow under eutrophic conditions. We hypothesized that zebra mussels and fish would change the transfer efficiencies of essential substances by altering the contents of plankton communities. In addition, we predicted that FAs, including PUFAs, and nutrients (N and P) would be transferred more efficiently than C due to the ability of zooplankton to regulate the contents of essential substances and/or shifts in taxonomic transfer in planktonic communities.
Materials and Methods
We conducted mesocosm experiments in 2017 and 2018 using nearly identical methods except for the manipulation of macrobiota. The experimental setup is given in Figure 1. Six mesocosms (0.94 × 0.64 × 0.50 m; 300 L, high-density polyethylene containers) were located on the shore of the eutrophic Lake Mikołajskie (Mazurian Lake District, northeastern Poland, 21°35′E, 53°48′N) at the Research Station of the Nencki Institute of Experimental Biology, Polish Academy of Sciences. The trophic status of this lake is confirmed by the trophic state index (TSI) of Carlson (1977) which ranged from 50 to 60, and the Secchi disc visibility varied from 1 to 2 m (Chróst et al., 2009; Karpowicz et al., 2020; Karpowicz and Ejsmont-Karabin, 2021). The phytoplankton of Lake Mikołajskie are dominated by cyanobacteria, dinoflagellates, and diatoms (Feniova et al., 2020), and the chlorophyll-a concentration ranged from 10 to 50 µg L−1 (Chróst et al., 2009; Karpowicz et al., 2020). The mesocosms were filled with unfiltered water from Lake Mikołajskie (Chróst et al., 2009) that contained in situ phytoplankton and zooplankton communities. The species compositions in both years were nearly identical. The cladoceran community included Ceriodaphnia pulchella Sars, 1862 (a dominant species among cladocerans), Chydorus sphaericus (O. F. Müller, 1776), Bosmina coregoni Baird, 1857, Bosmina longirostris (O. F. Müller, 1776), and Diaphanosoma brachyurum (Liévin, 1848). The copepod community included Thermocyclops oithonoides Sars, 1863 (a dominant species among copepods), Thermocyclops crassus (Fischer, 1853), Eudiaptomus gracilis (Sars, 1863), Eudiaptomus graciloides (Lilljeborg, 1888), and Mesocyclops leuckarti (Claus, 1857). Additionally, we added the inoculums of Daphnia magna Straus (originated from Binnensee, Germany) and Daphnia pulicaria Forbes (originated from Lake Brome, Canada) obtained from laboratory cultures at densities of 1.0 ind. L−1 for each species on day 1 of each experiment. We added these two species of Daphnia to increase zooplankton species richness in the mesocosms and because Daphnia play an important role in aquatic ecosystems. They are major grazers of phytoplankton and the major step in energy transfer from phytoplankton to fish. They compete with zebra mussels for algal resources and are directly consumed by fish.
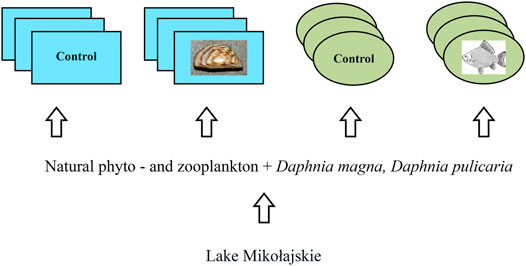
FIGURE 1. Experimental setup. Each blue rectangle (2017-experiment with zebra mussels) or green oval (2018-experiment with fish) represents a mesocosm. We filled the mesocosms with water from Lake Mikołajskie with the addition of large Daphnia.
We manipulated the presence/absence of zebra mussels in 2017 and fish in 2018. In each year, we had two treatments—control (C-2017 and C-2018) and an experimental treatment where zebra mussels (ZM-2017) or fish (F-2018) were present. The treatments were replicated in triplicate mesocosms. Both experiments were conducted for 30 days. The zebra mussel treatment was established by adding zebra mussels at a wet weight of 250 g m−2, which was approximately 200 individuals per mesocosm. Similar levels of zebra mussels have been reported in the two Polish lakes (lakes Licheńskie and Ślesińskie) where their biomass ranged from 0.02 to 2.79 kg m−2 (Sinicyna and Zdanowski, 2007). We collected zebra mussels from the nearby Lake Boczne and transported them to the laboratory in coolers. We added zebra mussels to the mesocosms within 24 h of collection on day 1 of the experiment. The size range of mussels used in the experiment was 7–24 mm. Zebra mussel mortality did not exceed 3% by the end of the experiment.
To create the fish treatment (F-2018), one individual ruffe Gymnocephalus cernuus (Linnaeus, 1758) between 7.5 and −11 cm (standard length) was added to each mesocosm. Fish were kept in 5 L boxes that were suspended in the mesocosms. The boxes had large slots that allowed zooplankton to pass freely, but kept the fish inside. The fish were let out of the cage for only an hour (between 8 and 9 p.m.) each day to limit predation on zooplankton (Feniova et al., 2015). The mesocosms were open, but they were covered with a polyethylene multilayer film during stormy weather.
We measured temperature and dissolved oxygen concentrations daily from the center of each mesocosm using a WTW multiparameter probe 3,410 with optical sensor FDO925. Temperature varied from 15.8°С to 18.5°С in 2017 and from 13.3°С to 24.4°С in 2018. The ranges of the changes in dissolved oxygen concentrations were 8.8–15.7 mg/L in 2017 and 8.4–12 mg/L in 2018.
We also collected zooplankton samples at 10-day intervals, starting with day 1, from the center of each mesocosm after gently mixing using a 2.6-L Limnos sampler. The samples were filtered through a 100 µm mesh sieve, and the collected zooplankton were fixed with 4% formaldehyde and all crustaceans were identified to species. We measured the lengths of up to 100 individuals of each taxon for biomass calculations by applying the equations from the work of Błędzki and Rybak (2016). Chlorophyll-a concentrations were recorded using a submersible spectrofluorometer FluoroProbe (bbe-Moldaenke, Germany) (Kring et al., 2014; Karpowicz and Ejsmont-Karabin, 2017). Chlorophyll concentrations were converted to C units using C to chlorophyll-a ratios based on the database of phytoplankton wet-weight biomass and chlorophyll-a concentrations from the work of Yacobi and Zohary (2010).
Gross primary production (GPP) was also estimated at 10-day intervals using the chlorophyll fluorescence method with DCMU (3-(3,4-dichlorophenyl)-1,1-dimethylurea) and the FluoroProbe. DCMU was added to the samples to inhibit the reoxidation of the reduced primary electron acceptor of the photosystem II. GPP (g L−1 h−1) was calculated by the equation proposed by Gaevsky et al. (2000):
where b is the empirical coefficient, 0.00042, Fv/Fm is the relative variable fluorescence (arbitrary units), chl-a is the chlorophyll-a concentration of algae (µg L−1), and I is the average intensity of photosynthetically active radiation (PAR, W m−2). Relative variable fluorescence was calculated using the following formula:
where F0 is the steady-state level of fluorescence and Fm describes the maximum level after the addition of 10 µM DCMU. The relative variable fluorescence summarizes the general condition of algal cells and their instant photosynthetic capacity.
GPP per hour was multiplied by daylight hours (using https://www.timeanddate.com/) for daily GPP calculations. The In situ attenuation of PAR (W m-2) was measured with a portable underwater irradiance meter with a Licor radiation sensor and a LI-193SA Spectral Quantum sensor (United States). The conversion factor for GPP from mg O2 to mg C was 0.32 (Alimov, 1989).
Secondary production (SP, crustacean zooplankton production) was calculated using regression equations given by Stockwell and Johansson (1997):
where P is the daily production (µg DW L−1 day−l), M is the mean individual dry weight (µg), and N is the abundance (individuals L−1). The dry weight of zooplankton was converted into C units dividing by 2.3 (Alimov, 1989).
We collected seston samples (3–5 L) for elemental and FA (EPA, DHA, and total FAs) analyses on day 1 and day 30. We first removed all the particles and live organisms by passing the samples through a 100 µm mesh sieve. These filtered samples were then passed through precombusted glass-fiber GF/F filters until an intensive color was reached (Whatman, United States). The filters for the FA analysis were dried at ambient temperature for about 30 min, placed in 3 ml containers with a chloroform–methanol mixture (2:1, v⁄v), and stored at −20°C. We dried the filters for organic C, P, and N overnight at 75°C and stored them dry in a desiccator until further analyses.
Zooplankton samples for elemental and FA analyses were also collected on day 1 and day 30. Zooplankton samples were collected using a 10 L bucket (20–40 L) in order to collect a minimum 50 mg of wet weight from each mesocosm. The samples were passed through a 100 µm mesh sieve. Crustaceans collected on the sieve were dried with filter paper and divided into subsamples for FA and elemental analyses. The subsamples for FAs were weighed and placed into a chloroform–methanol mixture (2:1, v/v) and frozen. The subsamples for estimating P, N, and C were weighed and heated at 75°C overnight. Then, they were stored in a desiccator at ambient temperature for further analyses.
Samples for seston and zooplankton analyses required large volumes of water from the mesocosms. Therefore, we only collected samples for seston and zooplankton on days 1 and 30 because we did not want to remove large abundances of plankton and disturb community dynamics during the middle of the experiments.
We analyzed the FA contents of seston and zooplankton using a modified protocol from the work of Gladyshev et al. (2015). Briefly, the collected mass of seston or zooplankton was triple mechanically homogenized in a mixture of chloroform–methanol. The solvents were removed from lipid extracts by rotoevaporation under vacuum. The lipid extracts were methylated in a two-step way by heating for 10 min at 90°C in a 0.2 M sodium methoxide methanolic solution; then, a portion of 1 M H2SO4 methanolic solution up to acidic reaction was added, and the mixture was again heated for 10 min at 90°C. The prepared fatty acid methyl esters (FAMEs) were dissolved in hexane and analyzed by using a gas chromatograph mass spectrometer (6890/5975C, “Agilent Technologies,” Santa Clara, CA, United States) equipped with a 30 m long and 0.25 mm internal diameter capillary column HP-FFAP. The peaks of FAMEs were identified by their retention time and mass spectra, compared to those in the integrated data base NIST-2005 and to an available authentic standard (37 FAME mixture, U-47885, Supelco, United States). FAME peaks were quantified by their area compared to a peak area of the internal standard, non–adecanoic acid methyl ester that was added to samples prior to extraction as a portion of a chloroform solution. We periodically controlled instrumental analytical precision by five replicate injections of the standard that gave satisfactory relative standard deviation of the response values <5%.
Organic C and N were measured by using a Flash EA 1112 NC Soil/MAS 200 elemental analyzer (ThermoQuest, Milan, Italy) (Gladyshev et al., 2007). Calibration curves for the elemental analyzer were generated using aspartic acid and standard soil reference material. The contents of particulate total P were estimated following the conventional photocolorimetric method (Murphy and Riley, 1962).
We calculated daily production/biomass ratios (P/B) which indicated the potential of daily production for C and essential substances (Ikeda and Shiga, 1999; Ikeda et al., 2002). Production and biomass were measured in C units. We used production per unit of biomass because zebra mussels and fish greatly impacted zooplankton abundances (mg/L). In this case, we excluded the abundance component from fish/zebra mussel effects and compared fish/zebra mussel treatments to the corresponding controls at a given biomass. If we had used production parameter per unit of volume, i.e., per L, we would have recorded the pooled impacts of macrobiota on taxon abundances and production per unit of biomass.
We calculated the production of each substance by multiplying Pzoo/B or Pphyto/B by the content of each substance (EPA:C, DHA:C, total FAs:C, N:C, and P:C). Therefore, we determined how much substance was produced in phytoplankton or zooplankton each day per unit of biomass (C units). We assessed the trophic transfer at the phytoplankton–zooplankton interface as the potential transfer efficiencies of each element (C, N, and P), EPA, DHA, and total FAs from phytoplankton to zooplankton measured as the ratio P/Bzoo:P/Bphyto × 100% (Feniova et al., 2021). This measured the percentage of a substance produced per unit of biomass in a day in zooplankton compared to that produced in a day in phytoplankton. We assumed that if the production of a substance per unit of biomass was higher in zooplankton than that of phytoplankton, then zooplankton accumulated this substance in their bodies relative to the content in phytoplankton. If this ratio was less than 100%, then it implies that some of the amount of matter was lost during its transfer from phytoplankton to zooplankton.
We used two-way Generalized Linear Model (GLM) Analysis of Variance (ANOVA) with treatment (C-2017, ZM-2017, C-2018, and F-2018) and macrobiota (zebra mussels and fish) as factors to determine if there were significant differences between mesocosms on day 1 in the 2017 and 2018 experiments. We conducted separate statistical analyses for the 2017 and 2018 experiments if the response variables were significantly different on day 1 between the 2017 and 2018 experiments. We constructed mixed GLMs to determine the effects of treatment (control and macrobiota), trophic level (phytoplankton and zooplankton), and their interactions on the dependent response variables (biomass, P/B ratio, contents of substances, and transfer efficiencies). Treatment was specified as a random factor because mesocosms in the treatments (C-2017, ZM-2017, C-2018, and F-2018) were selected randomly while the trophic level and fish/zebra mussels were specified as the fixed factor. If significant factor effects were detected, we used Fisher’s test (p < 0.05) to determine which variables differed; then, we used Fisher's post hoc tests (p < 0.05) to establish significant differences in variables including the content of substances, P/B ratio, and efficiency.
The biomasses of cladocerans and copepods were compared using Student’s t-test to determine significant differences between control and fish/zebra mussel treatments. The normality of distribution was tested using the Shapiro–Wilk test.
Statistical analyses were conducted using the integrated software BioSystem office (Petrosyan, 2014) and R 3.3 (R Core Team, 2017). RStudio Desktop version 1.1.463 was used as an IDE for R language (https://www.rstudio.com/).
The relationships between the P/B ratios of essential nutrients and FAs (N, P, EPA, DHA, and total FAs) in phytoplankton and zooplankton were analyzed using principal component analysis (PCA). PCA was also used to evaluate how experimental treatments (C-2017, ZM-2017, C-2018, and F-2018) affected the P/B ratios of essential substances in phytoplankton and zooplankton. PCA was performed with XLSTAT Ecology (Addinsoft, United States).
Results
The elemental and FA contents of the algae were significantly different on day 1 (starting point) between the 2017 and 2018 experiments (Figure 2; Table 1). The contents of EPA (Figure 2A), DHA (Figure 2B), total FAs (Figure 2C), and N (Figure 2D) in the phytoplankton were significantly higher in the 2017 experiment than those in the 2018 experiment. The content of P in algae did not significantly differ between the 2017 and 2018 experiments. In contrast, these substances did not differ in zooplankton in the 2017 and 2018 experiments (Figures 2F–J). We did not combine data for the 2 years into one statistical model because of the significant differences in the starting conditions of the algae in 2017 and 2018, and we analyzed the two experiments separately.
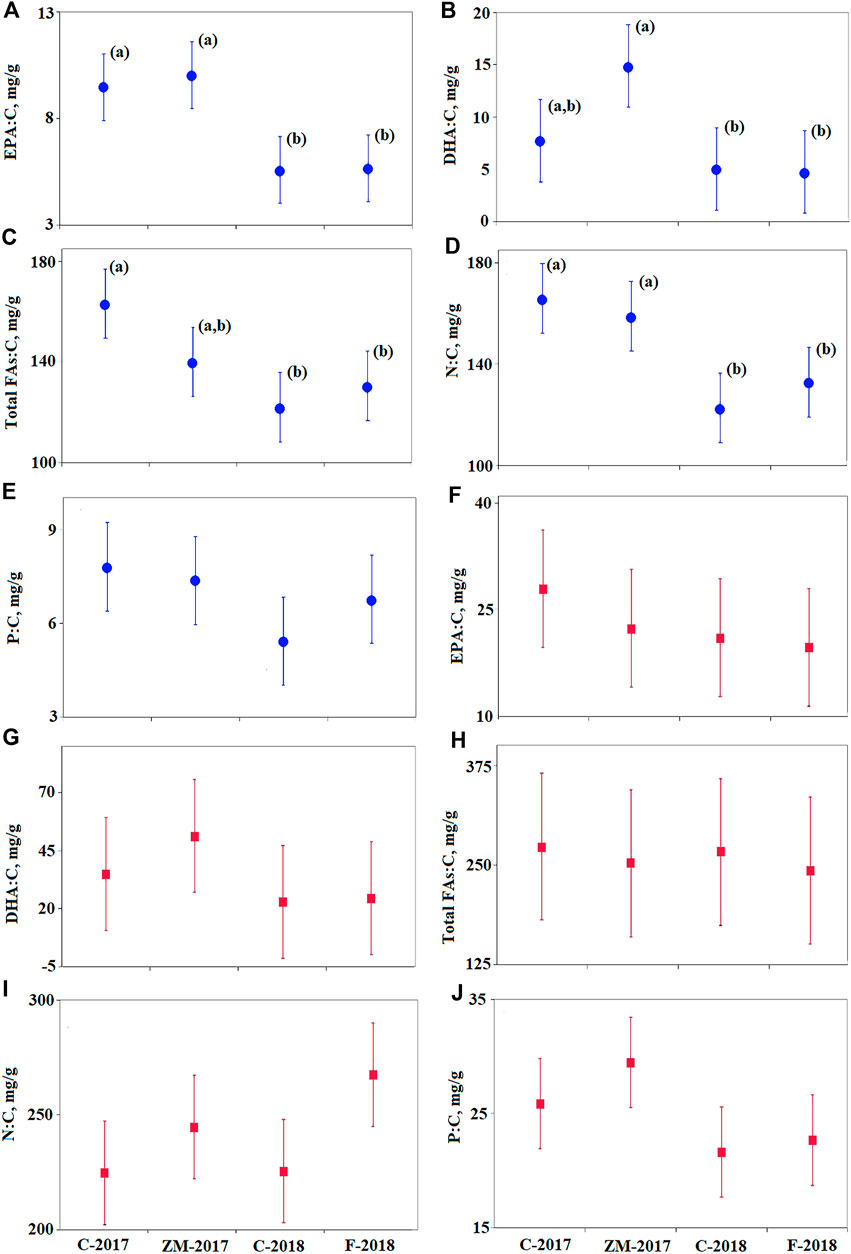
FIGURE 2. Comparison of the contents of essential elements and fatty acids in phytoplankton and zooplankton between treatments (C-2017, ZM-2017, C-2018, and F-2018) on day 1 in the 2017 and 2018 experiments. Significant differences were determined using Fisher's LSD post hoc test (p < 0.05). Vertical bars represent 95% Fisher's LSD intervals. See Table 1 for statistical parameters obtained by two-way GLM ANOVA. Significant differences between the treatments are depicted with different letters. (A–E) Plots for phytoplankton (blue circles); (F–J) plots for zooplankton (red squares).
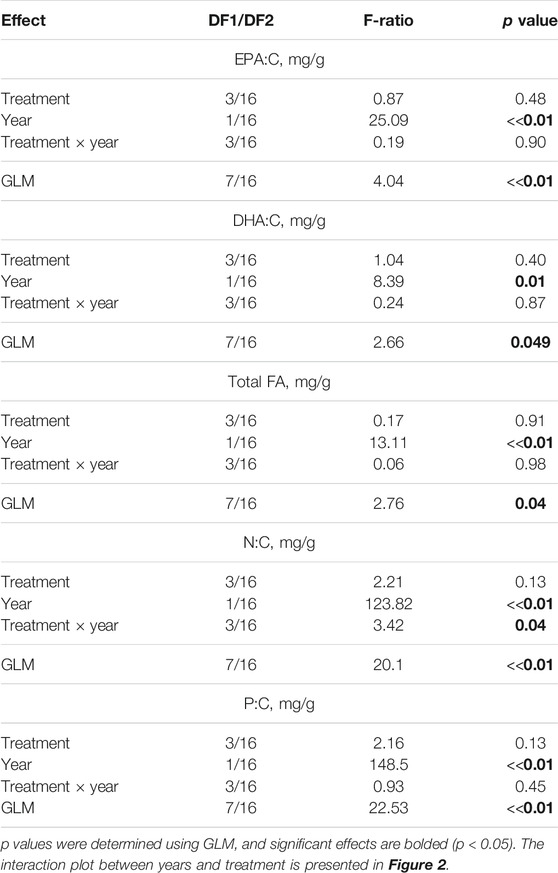
TABLE 1. Comparison of the contents of essential elements and fatty acids in phytoplankton and zooplankton using two-way GLM ANOVA between treatments (C-2017, ZM-2017, C-2018, and F-2018) on day 1 in the 2017 and 2018 experiments. The factors are year (2017 and 2018) and treatment, where treatment (C-2017, ZM-2017, C-2018, and F-2018) is the random factor.
Both fish and zebra mussels changed the size structure of crustaceans. Large Daphnia significantly declined in fish (Figure 3D) and zebra mussel (Figure 3C) treatments, while small-bodied cladocerans (Figures 3A,B) and copepods (Figures 3E,F) were not affected by macrobiota.
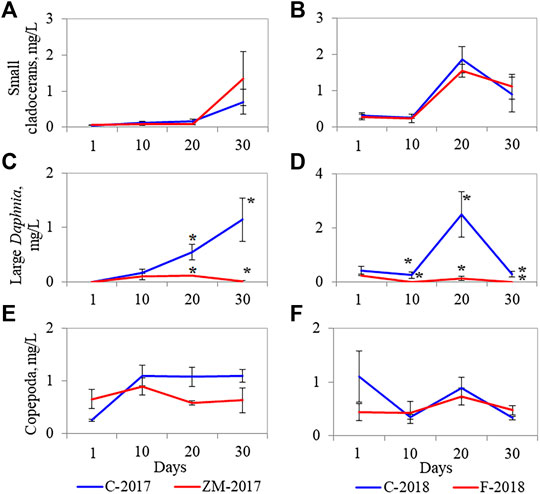
FIGURE 3. Biomass dynamics of small сladocerans (A, B), large Daphnia (C, D), and сopepods (E, F) in control (blue lines) and fish/zebra mussel (red lines) treatments in the zebra mussel (A, C, E) and fish (B, D, F) experiments. Bars represent standard errors of the means. Significant differences between control and fish/zebra mussel treatments are designated by asterisks.
Figure 4 and Table 2 show the differences in the parameters between phytoplankton and zooplankton on day 30. In the ZM-2017 treatment, phytoplankton biomass expressed in C units was significantly greater than it was in zooplankton (Figure 4A). In contrast, there were no differences between phytoplankton and zooplankton biomass in the C-2017 (Figure 4A). P/B ratios did not differ between phytoplankton and zooplankton in either treatment (Figure 4C). However, PUFAs (EPA:C and DHA:C) differed between phytoplankton (lower) and zooplankton (higher) in the C-2017, while they were similar in the ZM-2017 treatment (Figures 4E,G). Total FAs:C did not differ between phytoplankton and zooplankton in 2017 (Figure 4I). Nutrients were higher in zooplankton than in phytoplankton by factors of 1.3 (N, C-2017), 1.4 (N, ZM-2017), 2.3 (P, C-2017), and 2.5 (P, ZM-2017) (Figures 4K,M).
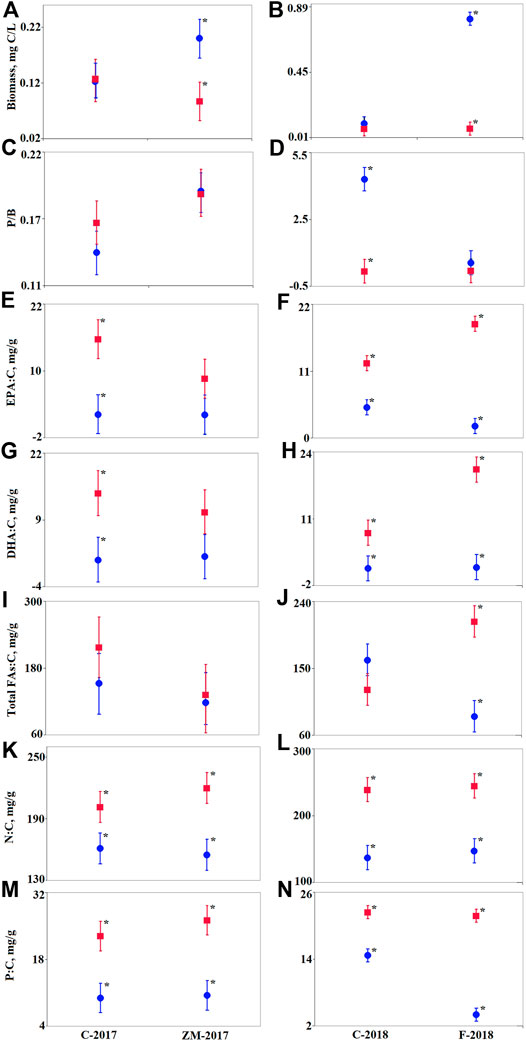
FIGURE 4. Comparison of biomass (A, B), P/B ratio (C, D), and mean contents of the main substances (E–N) on day 30 in experimental treatments [C-2017 and ZM-2017 (left column), and C-2018 and F-2018 (right column)] between phytoplankton (blue circles) and zooplankton (red squares). See Tables 2, 3 for statistical parameters obtained by two-way GLM ANOVA. The factors were treatment and trophic level (zooplankton and phytoplankton) and their interaction. Data are presented as means with 95% Fisher’s LSD intervals using the post hoc test. Significant differences between phytoplankton and zooplankton parameters are designated by asterisks.
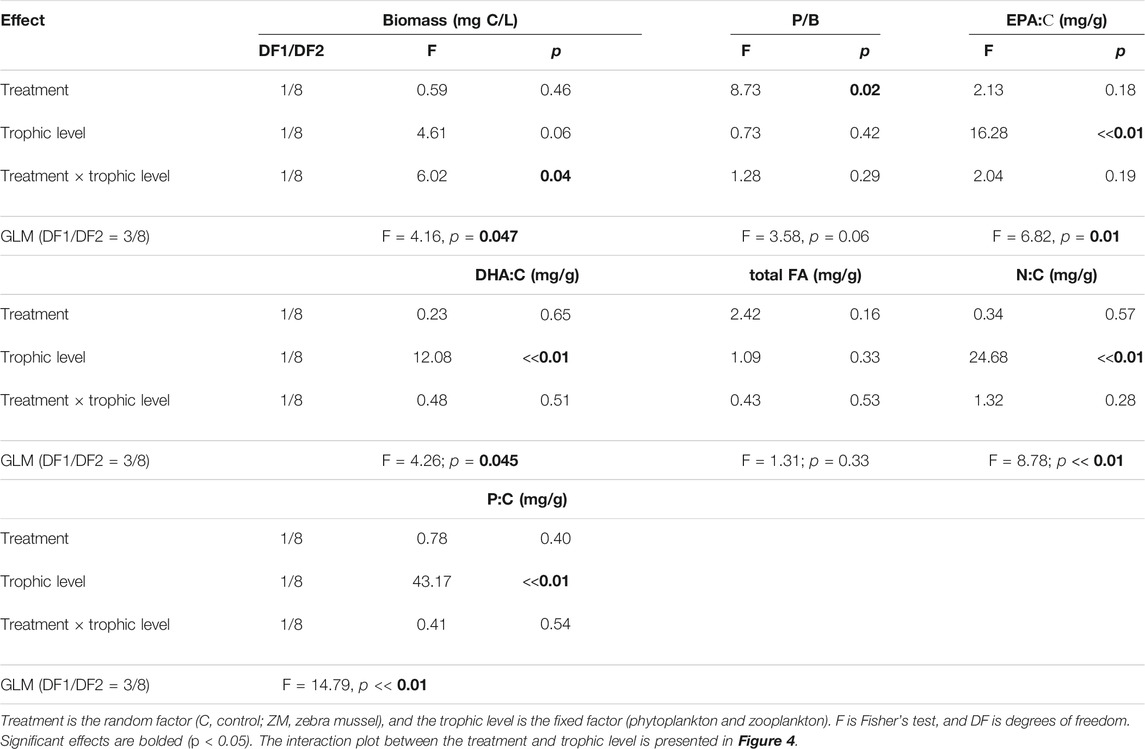
TABLE 2. Results of GLM two-way ANOVA for biomass, P/B ratios, and contents of substances on day 30 in the 2017 experiments.
In 2018, phytoplankton and zooplankton biomasses were similar in the C-2018 treatment, but phytoplankton biomass was significantly higher than zooplankton biomass in the F-2018 treatment (Figure 4B; Table 3). P/B ratio did not significantly differ between phytoplankton and zooplankton in the F-2018 treatment, while P/B was greater in phytoplankton compared to zooplankton in the C-2018 treatment (Figure 4D). EPA:C and DHA:C were significantly higher in zooplankton than those in phytoplankton in both treatments (Figures 4F,H). On average, the ratios of zooplankton:phytoplankton in terms of EPA:C were 2.4 and 9.5 in the C-2018 and F-2018 treatments, respectively, while in terms of DHA:C, these differences were 6.2 and 12.9. Total FAs:C ratios were similar in the C-2018 treatment but 2.5-fold higher in zooplankton than in phytoplankton in the F-2018 treatment (Figure 4J). Thus, the presence of fish resulted in the accumulation of total FAs and PUFAs in zooplankton. The ratio of zooplankton:phytoplankton in terms of N was, on average, 1.7 in both the treatments (Figure 4L), while in terms of P, zooplankton:phytoplankton ratios were 1.5 and 5.4 in the C-2018 and F-2018 treatments, respectively (Figure 4N).
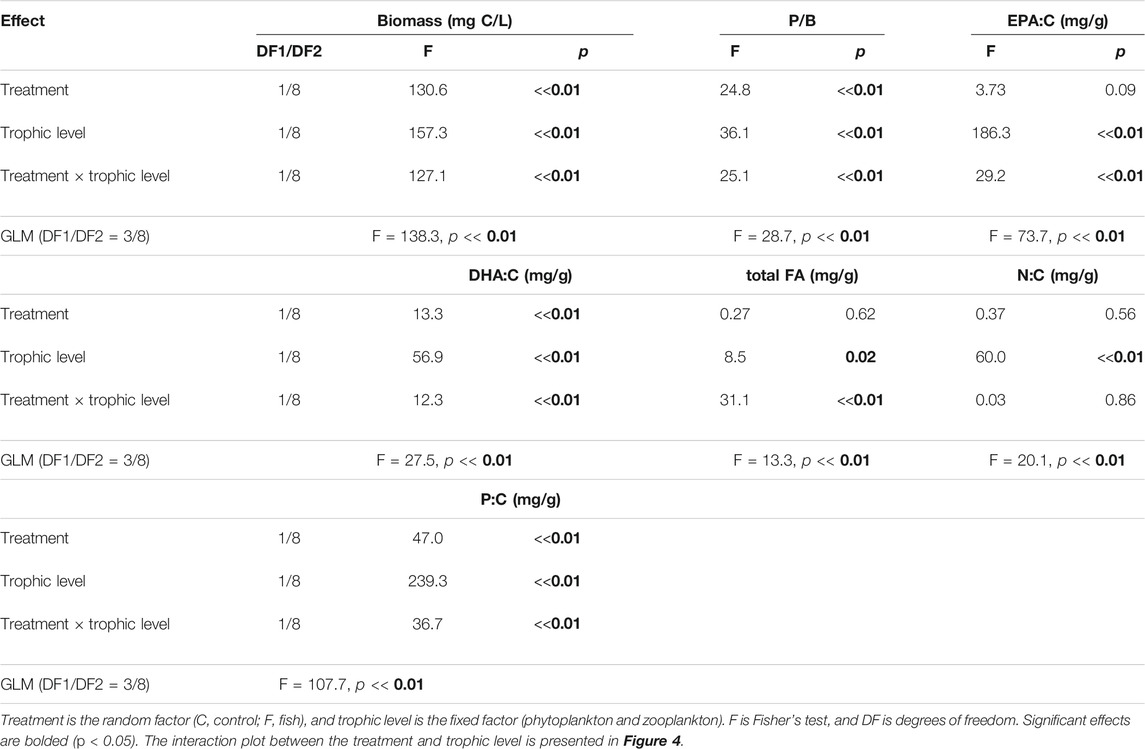
TABLE 3. Results of GLM two-way ANOVA for biomass, P/B ratios, and contents of substances on day 30 in the 2018 experiments.
Overall, PUFAs:C ratios in zooplankton were higher than PUFAs in phytoplankton except in the ZM-2017 treatment. N and P were higher in zooplankton than in phytoplankton in all treatments. In contrast, total FAs did not accumulate in zooplankton except in the F-2018 treatment. The higher contents of essential substances can result in their greater production per unit of biomass in zooplankton and, in turn, potentially increase transfer efficiencies from phytoplankton to zooplankton. The contents of FAs, including PUFA, and nutrients varied widely in the experiments. Supplementary Table S1 shows that the ranges of content variations of the studied substances were wide also in the other studies which showed their dependence on environmental conditions. Therefore, we can expect that the efficiency of their transport from phytoplankton to zooplankton would be also dependent on zebra mussel/fish effects.
The results of PCA revealed differences in the P/B ratios of N, P, total FAs, EPA, and DHA between phytoplankton and zooplankton (Figure 5). In 2017, the P/B of N, P, total FAs, and EPA was not correlated between phytoplankton and zooplankton (Figures 5A,B). However, the P/B of DHA was negatively correlated between phytoplankton and zooplankton (Figure 5A). Zebra mussels increased the P/B of both nutrients in phytoplankton and zooplankton (Figure 5B), but DHA only increased in phytoplankton (Figure 5A). In 2018, P/B of nutrients, total FAs, and PUFAs was negatively correlated between zooplankton and phytoplankton (Figures 5C,D). Fish increased the P/B of N, P, total FAs, and PUFAs in zooplankton but decreased the P/B of N, P, total FAs, and PUFAs in phytoplankton (Figures 5C,D).
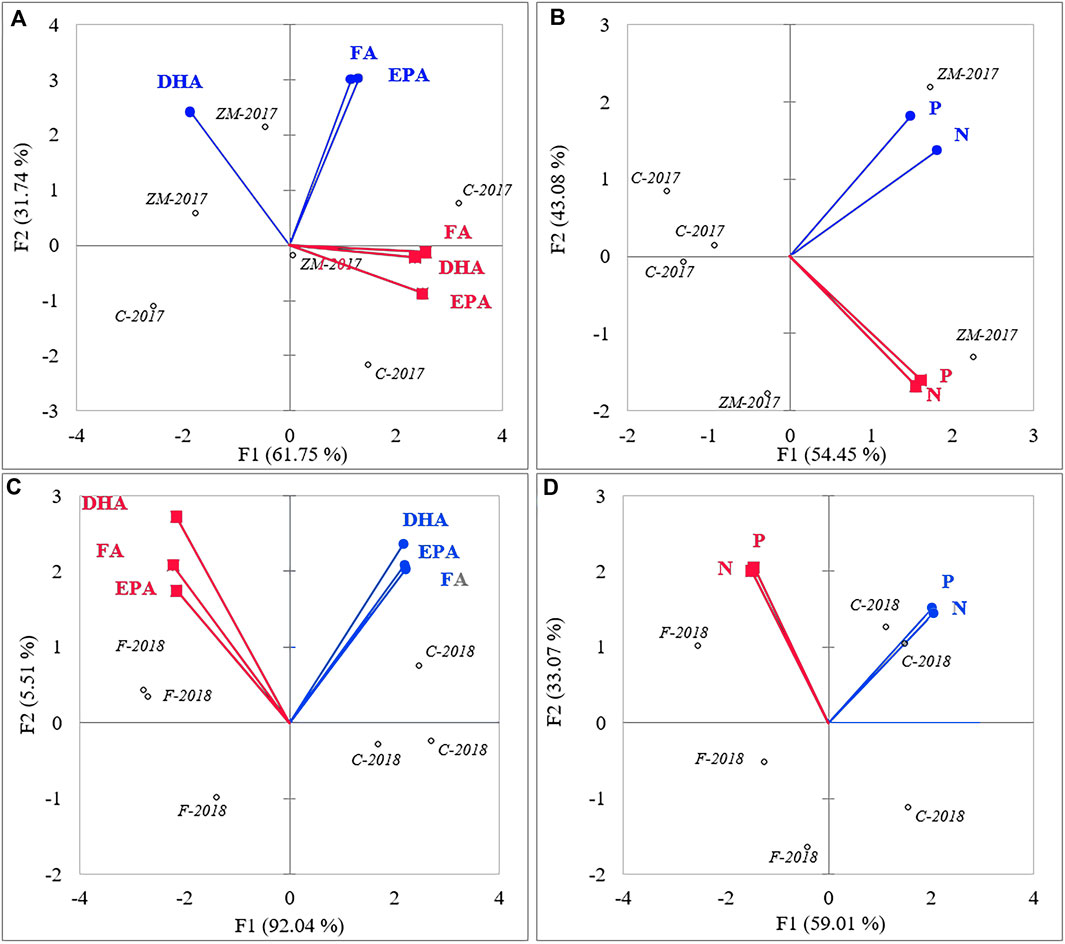
FIGURE 5. Principle component analysis map of production : biomass ratios of PUFAs (A, C) and nutrients (B, D) in phytoplankton (blue circles) and zooplankton (red squares) in the 2017 (A, B) and 2018 (C, D) treatments.
The transfer efficiencies of C, total FAs, N, and P did not differ between the C-2017 and ZM-2017 treatments (Figure 6A). However, zebra mussels reduced the transfer of EPA from phytoplankton to zooplankton by 2.1-fold and DHA transfer by 3.4-fold. In contrast, fish increased the transfer of EPA by 33.0-fold, DHA by 19.2-fold, and P by 37.2-fold, relative to the C-2018 treatment (Figure 6B). The transfer efficiencies of C, total FAs, and N from phytoplankton to zooplankton were similar in the C-2018 and F-2018 treatments.
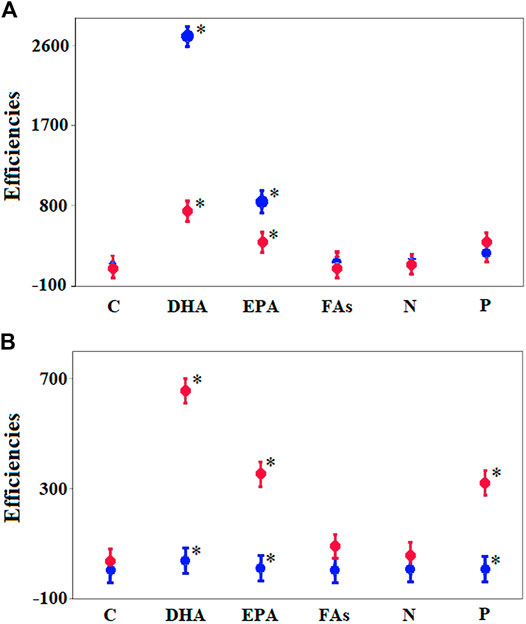
FIGURE 6. Comparison of the transfer efficiencies of essential substances on day 30 between C-2017 (blue circles) and ZM-2017 (red circles) (A) and between C-2018 (blue circles) and F-2018 (red circles) (B) using two-factor GLM ANOVA (random factor—treatments; fixed factor—macrobiota). Significant differences were determined using Fisher's LSD post hoc test (2017: F = 41.7, p << 0.001; 2018: F = 20.7, p << 0.011). Significant differences were found for DHA (2017: F = 63.6, p = 0.001; 2018: F = 25.4, p << 0.007), EPA (2017: F = 7.1, p = 0.05; 2018: F = 82.3, p << 0.001), and P (2018: F = 10.2; p = 0.047) which are designated by asterisks.
Different substances were transferred with different efficiencies (Figure 7). DHA was transferred more efficiently than all the other substances including C in both treatments in 2017 (Figures 7A,B) and 2018 (Figures 7C,D). EPA was not transferred as efficiently as DHA, but more efficiently than C (C-2017 and F-2018), total FAs (C-2017, C-2018, and F-2018), N (C-2017 and F-2018), and P (C-2017). Thus, the transport efficiency of EPA in the zebra mussel treatment, ZM-2017, did not exceed the transfer efficiencies of any of the other substances, including C, while EPA was transferred more efficiently in C-2017 than all the other substances except DHA. The transfer efficiencies of EPA were higher than those of C, total FAs, and N in the F-2018 treatment. Total FAs and N were transferred as efficiently as C in all the treatments in both years. The transfer efficiency of P was higher than that of C, total FAs, and N in the F-2018 treatment only. P was transported as efficiently as C in the other treatments. Thus, C was transferred less efficiently relative to DHA in all the treatments, relative to EPA in C-2017 and F-2018, and relative to P in the F-2018 treatment. But, the transfer efficiencies of C did not differ from those of total FAs and N.
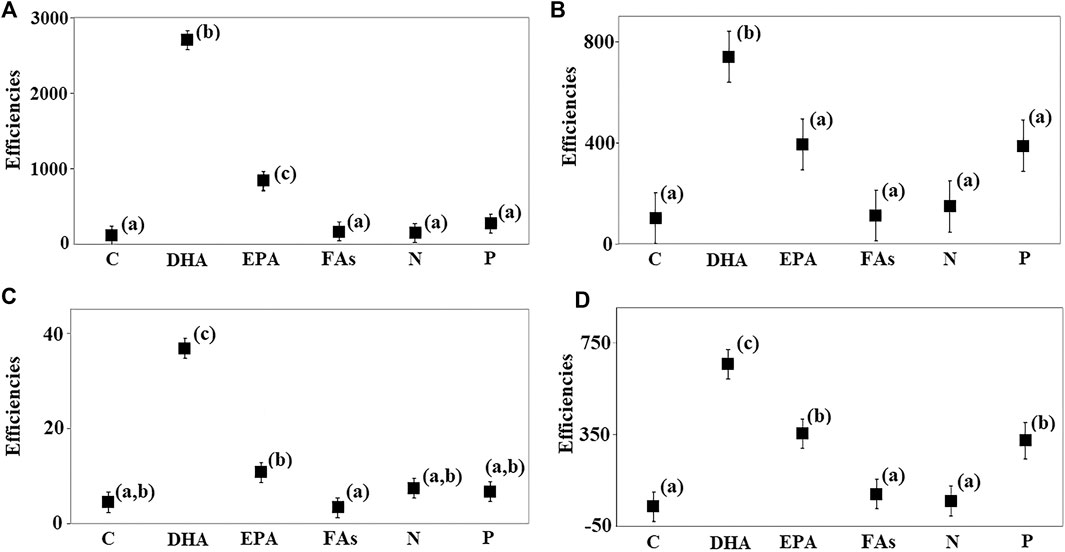
FIGURE 7. Comparison of the transfer efficiencies of essential substances on day 30 in the 2017 and 2018 experiments. Mean efficiencies were compared using one-way GLM ANOVA (fixed factor—substances). Significant differences were determined by Fisher's LSD post hoc test (C-2017: F = 66.1, p << 0.01; ZM-2017: F = 6.02, p = 0.005; C-2018: F = 35.6, p << 0.001; and F-2018: F = 17.8, p << 0.001). Different letters (a, b, and c) designate significant differences. Error bars represent the standard errors of the means. (A) C-2017; (B) ZM-2017; (C) C-2018; and (D) F-2018.
Discussion
Our experiments showed that fish and zebra mussels altered the P/B ratios of phytoplankton and zooplankton. The P/B phytoplankton:zooplankton ratios indicate efficiency of the transport of essential substances up the trophic webs per unit of biomass, i.e., under conditions that the biomasses of plankton communities were equal in the control and fish/zebra mussel treatments. Our experiments showed that EPA (in C-2017 and F-2018 treatments) and DHA (in C-2017, ZM-2017, C-2018 and F-2018 treatments) were transferred from phytoplankton to zooplankton more efficiently than C. As a result, zooplankton accumulated more PUFAs in their bodies compared to phytoplankton. Similar results were obtained in Lake Washington (Seattle, Washington, United States) where zooplankton accumulated greater percentages of PUFAs than were available in seston (Ravet et al., 2010). More efficient transfer of PUFAs than C was first reported from the eutrophic Bugach Reservoir (Gladyshev et al., 2011a) and the Enisej River (Gladyshev et al., 2009). We confirmed that EPA and DHA can be transferred from phytoplankton to zooplankton more efficiently than C, thus making zooplankton a higher quality food resource for planktivoros fish. DHA was transferred more efficiently than EPA in our experiments. DHA is a crucial molecule for fish reproduction, somatic growth, brain functioning, and vision (Ballantyne et al., 2003; Vizcaino-Ochoa et al., 2010; Jardine et al., 2020). As fish require large amounts of DHA, the enrichment of zooplankton with DHA has the potential to be very beneficial for fish fry. However, zebra mussels can decrease PUFA accumulation by zooplankton, thus decreasing the quality of zooplankton for fish.
Cladocerans preferentially accumulate EPA, while copepods accumulate DHA. As a result, cladocerans have low DHA content and a large portion of EPA while copepods have high DHA content and low EPA (Persson and Vrede, 2006; Smyntek et al., 2008; Ravet et al., 2010; Burns et al., 2011; Lau et al., 2012). Therefore, we could expect that decreases in large Daphnia in zebra mussel and fish treatments would result in the reduction of EPA transfer efficiency in both treatments compared to the control treatment. What is noteworthy is that, in contrast to large Daphnia, biomasses of copepods and small cladocerans did not change in zebra mussel or fish treatments relative to the corresponding controls. Nevertheless, the effects of zebra mussels and fish on EPA transfer efficiencies were opposite, i.e., zebra mussel decreased the EPA transfer, while fish increased it relative to the control. Therefore, the shift in the taxonomic structure of zooplankton did not determine the transfer efficiency.
PCA showed that there were no positive correlations in production/biomass ratios of the main substances between zooplankton and phytoplankton. Therefore, our data are consistent with the statement that zooplankton can regulate their body contents to maintain the homeostasis of FA composition (Lau et al., 2012) in accordance with their requirements.
Crustaceans frequently incorporate some FAs of their food without transforming them into different forms (Goulden and Place, 1990; Robin, 1995; Gulati and DeMott, 1997; Arts et al., 2001). However, there are several potential mechanisms of FA regulation, including PUFAs, by crustaceans. Crustaceans can biosynthesize PUFAs by converting FAs from one form to another through the elongation and desaturation of molecules (Brett and Müller-Navarra, 1997). For example, cyclopoid copepods are likely to biosynthesize DHA (Desvilettes et al., 1997), while diaptomid copepods may convert EPA into DHA (Ravet et al., 2010). Therefore, higher production of PUFAs in zooplankton relative to phytoplankton in some of the experiments could be attributed to the conversion of some forms of FAs into PUFAs by zooplankton. Jardine et al. (2020) showed that consumers do not simply accumulate FAs in their tissues, but rather transform them to meet their physiological and metabolic needs.
PUFAs are not commonly used as energetic resources (Sargentet et al., 2002; Jardine et al., 2020). Other FAs, including saturated fatty acids or monosaturated fatty acids, are more often subjected to catabolism than PUFAs (Gladyshev et al., 2011a). This may help to explain why total FAs were not accumulated in zooplankton relative to phytoplankton and why total FAs were transferred as efficiently as C. Thus, our findings are in accordance with the statement that, in contrast to short-chain FAs, long-chain PUFAs increased with each step up the trophic web (Guo et al., 2018).
The other reason for an observed higher content and production of PUFAs per unit of zooplankton biomass relative to phytoplankton is that zooplankton selectively incorporate the most physiologically important FAs through preferential assimilation, selective feeding on more nutritious algae, and/or feeding within higher-quality food patches (Burns et al., 2011). In support, Taipale et al. (2016) found that δ13C in crustaceans did not correlate with δ13C in phytoplankton and instead correlated with the δ13C of particular phytoplankton taxa, indicating that crustaceans selectively assimilated phytoplankton. Selective feeding of Daphnia on nutritious algae over cyanobacteria was also demonstrated by Gladyshev et al. (2000).
In the zebra mussel treatment (ZM-2017), the contents of PUFAs in phytoplankton and zooplankton were similar. However, PUFAs were higher in zooplankton relative to phytoplankton in the control treatment. Furthermore, the transfer efficiencies of EPA and DHA in ZM-2017 were on average 2.1- and 3.6-fold lower than in the control treatment. Therefore, zebra mussels might have hindered the accumulation of PUFAs in zooplankton, or this effect could be attributed to the decrease of large Daphnia. This could be caused by a competition between crustaceans and zebra mussels for PUFA-rich food particles. In support, Makhutova et al. (2013) showed that zebra mussels selectively consume EPA-rich seston particles.
In contrast to zebra mussels, the contents of FAs, including EPA and DHA, were higher in zooplankton than in phytoplankton in the fish treatment. These differences were greater in the fish treatment than in the control treatment. As a result, the transfer efficiencies of EPA, DHA, and P in the F-2018 treatment exceeded those in the control treatment. Fish likely weakened the control of zooplankton over algae assemblage by preferably consuming larger cladocerans (Brooks and Dodson, 1965; Gliwicz, 2003), thus increasing the relative proportion of high-quality food items for zooplankton.
Limitation by key dietary elements (N and P) is also important for aquatic ecosystem functioning. Shortage of nutrients can alter consumers’ metabolism (Elser et al., 1996; Wagner et al., 2015). Elemental imbalances between food resources and consumer demands can have negative effects on the life-history parameters of consumers. The ability to effectively transfer N and P from phytoplankton to zooplankton can be a regulating mechanism through which these imbalances can be reimbursed. In our experiments, the contents of both N and P were higher in zooplankton than in phytoplankton. PCA showed that there was a mismatch in nutrient P/B ratios between phytoplankton and zooplankton, thus indicating that zooplankton can regulate nutrient contents in their bodies. This result is in accordance with studies showing that zooplankton are able to regulate nutrient allocations in their bodies in different biochemical and anatomic components, depending on their environmental levels (He and Wang, 2020). Karpowicz et al. (2019) experimentally showed that the content of nutrients in zooplankton was higher than that in phytoplankton as a result of nutrient accumulation in crustacean bodies. The accumulation of P in zooplankton has also been previously found in experimental mesocosms (Feniova et al., 2019). The accumulation of nutrients may be a mechanism that supports a sufficient level of nutrients in crustaceans. In addition, the accumulation of nutrients in zooplankton relative to their basal food sources can correspondingly increase the production of nutrients per unit of biomass and, in turn, increase the transfer of elements from phytoplankton to zooplankton. Both N and P were accumulated in zooplankton relative to phytoplankton regardless of the presence or absence of fish or zebra mussels. This fact suggests that these elements were in shortage in zooplankton diets.
The transfer efficiencies of N and P were not affected by zebra mussels, and their contents were similar in both the treatments. Both of these elements were transported as efficiently as C in the zebra mussel treatment and control treatment. Thus, zebra mussel reduced the transfer efficiencies of PUFAs, but did not have any impact on the transfer efficiencies of C, total FAs, N, and P.
To conclude, aquatic macrobiota, including fish and zebra mussels, play important functional roles in the transfer of essential substances from phytoplankton to zooplankton. Fish increased the efficiency of the transfer of EPA, DHA, and P relative to the control treatment at a given biomass, while zebra mussels reduced the transfer efficiencies of EPA and DHA. Our results showed that essential substances, including EPA, DHA, and P, can be transferred more efficiently than C from phytoplankton to zooplankton, while the transfer efficiency of FAs was similar to that of C. Enrichment of consumers by the most important substances relative to their basal food sources creates the potential to successfully transport these substances across long aquatic trophic webs and further to terrestrial communities.
Data Availability Statement
The raw data supporting the conclusions of this article will be made available by the authors without undue reservation.
Ethics Statement
Ethical review and approval was not required for the animal study because we used live young fish specimens in mesocosms as predators of zooplankton. After the experiments, we released them in the pond.
Author Contributions
MG, NS, and IF originally formulated the idea. IF, MK, and AD conceived and designed the experiments. IF and MK performed the experiments. IF, MK, MG, NS, and ES analyzed the data. VP and MK performed statistical analyses. IF, MK, and AD wrote the manuscript.
Funding
This experiment was performed with support from the Polish National Science Centre (2016/21/B/NZ8/00434). The statistical analysis and its interpretation was performed with support from the Russian Science Foundation (Grant No. 21-14–00123). Biochemical analyses were performed with support by Federal Tasks for Institute of Biophysics SB RAS No. 51.1.1 and Federal Tasks for Siberian Federal University No. FSRG-2020-0019. The preparation of the manuscript by Feniova I. was supported by the Polish National Agency for Academic Exchange (Agreement No. PPN/ULM/2020/1/00258/U/DRAFT/00001).
Conflict of Interest
The authors declare that the research was conducted in the absence of any commercial or financial relationships that could be construed as a potential conflict of interest.
Publisher’s Note
All claims expressed in this article are solely those of the authors and do not necessarily represent those of their affiliated organizations, or those of the publisher, the editors, and the reviewers. Any product that may be evaluated in this article, or claim that may be made by its manufacturer, is not guaranteed or endorsed by the publisher.
Acknowledgments
The authors would like to thank the Research Station in Mikołajki, part of the Nencki Institute of Experimental Biology, Polish Academy of Sciences, for providing them the place and equipment for conducting their experiments. They also thank Piotr Ignaciuk for assistance in collecting samples.
Supplementary Material
The Supplementary Material for this article can be found online at: https://www.frontiersin.org/articles/10.3389/fenvs.2021.739014/full#supplementary-material
References
Andersen, T., and Hessen, D. O. (1991). Carbon, Nitrogen, and Phosphorus Content of Freshwater Zooplankton. Limnol. Oceanogr. 36, 807–814. doi:10.4319/lo.1991.36.4.0807
Arts, M. T., Ackman, R. G., and Holub, B. J. (2001). "Essential Fatty Acids" in Aquatic Ecosystems: a Crucial Link between Diet and Human Health and Evolution. Can. J. Fish. Aquat. Sci. 58, 122–137. doi:10.1139/f00-224
Ballantyne, A. P., Brett, M. T., and Schindler, D. E. (2003). The Importance of Dietary Phosphorus and Highly Unsaturated Fatty Acids for Sockeye (Oncorhynchus nerka) Growth in Lake Washington - a Bioenergetics Approach. Can. J. Fish. Aquat. Sci. 60, 12–22. doi:10.1139/F02-166
Becker, C., and Boersma, M. (2005). Differential Effects of Phosphorus and Fatty Acids on Daphnia magna Growth and Reproduction. Limnol. Oceanogr. 50, 388–397. doi:10.4319/lo.2005.50.1.0388
Brabrand, A., Faafeng, B., Källqvist, T., and Nilssen, J. P. (1984). Can Iron Defecation from Fish Influence Phytoplankton Production and Biomass in Eutrophic Lakes? Limnol. Oceanogr. 29, 1330–1334. doi:10.4319/lo.1984.29.6.1330
Brett, M., and Müller‐Navarra, D. (1997). The Role of Highly Unsaturated Fatty Acids in Aquatic Foodweb Processes. Freshw. Biol. 38, 483–499. doi:10.1046/j.1365-2427.1997.00220.x
Brett, M. T., Müller-Navarra, D. C., Ballantyne, A. P., Ravet, J. L., and Goldman, C. R. (2006). Daphnia Fatty Acid Composition Reflects that of Their Diet. Limnol. Oceanogr. 51, 2428–2437. doi:10.4319/lo.2006.51.5.2428
Brooks, J. L., and Dodson, S. I. (1965). Predation, Body Size, and Composition of Plankton. Science 150, 28–35. doi:10.1126/science.150.3692.28
Burns, C. W., Brett, M. T., and Schallenberg, M. (2011). A Comparison of the Trophic Transfer of Fatty Acids in Freshwater Plankton by Cladocerans and Calanoid Copepods. Freshw. Biol 56, 889–903. doi:10.1111/j.1365-2427.2010.02534.x
Błędzki, L. A., and Rybak, J. I. (2016). Freshwater Crustacean Zooplankton of Europe. Switzerland, Basel: Springer. doi:10.1007/978-3-319-29871-9
Chróst, R. J., Adamczewski, T., Kalinowska, K., and Skowrońska, A. (2009). Abundance and Structure of Microbial Loop Components (Bacteria and Protists) in Lakes of Different Trophic Status. J. Microbiol. Biotechnol. 19 (9), 858–868. doi:10.4014/jmb.0812.651
Desvilettes, C., Bourdier, G., Amblard, C., and Barth, B. (1997). Use of Fatty Acids for the Assessment of Zooplankton Grazing on Bacteria, Protozoans and Microalgae. Freshw. Biol. 38, 629–637. doi:10.1046/j.1365-2427.1997.00241.x
Elser, J. J., Dobberfuhl, D. R., MacKay, N. A., and Schampel, J. H. (1996). Organism Size, Life History, and N:P Stoichiometry. Bioscience 46, 674–684. doi:10.2307/1312897
Feniova, I., Dawidowicz, P., Gladyshev, M. I., Kostrzewska-Szlakowska, I., Rzepecki, M., Razlutskij, V., et al. (2015). Experimental Effects of Large-Bodied Daphnia, Fish and Zebra Mussels on Cladoceran Community and Size Structure. J. Plankton Res. 37, 611–625. doi:10.1093/plankt/fbv022
Feniova, I., Sakharova, E. G., Gorelysheva, Z. I., Karpowicz, M., Górniak, A., Petrosyan, V., et al. (2020). Effects of Zebra Mussels (Dreissena polymorpha) on Phytoplankton Community Structure under Eutrophic Conditions. Aquat. Invasions. 15 (3), 435–454. doi:10.3391/ai.2020.15.3.05
Feniova, I., Sakharova, E., Karpowicz, M., Gladyshev, M. I., Sushchik, N. N., Dawidowicz, P., et al. (2019). Direct and Indirect Impacts of Fish on Crustacean Zooplankton in Experimental Mesocosms. Water 11 (10), 2090. doi:10.3390/w11102090
Feniova, I. Y., Sakharova, E. G., Buseva, Z. F., Gladyshev, M. I., Sushchik, N. N., Gorelysheva, Z. I., et al. (2021). Efficiency of Transfer of Essential Substances from Phytoplankton to Planktonic Crustaceans in Mesotrophic Conditions. Inland Water Biol. 14 (1), 49–59. doi:10.1134/S1995082920040033
Gaevskii, N. A., Kolmakov, V. I., Popelnitsky, V. A., Gold, V. M., and Dubovskaya, O. P. (2000). Evaluation of the Effect of Light Intensity on the Measurement of the Photosynthetic Rate in Plankton Microalgae by the Chlorophyll Fluorescence Method. Russ. J. Plant Physiol. 47, 820–825. doi:10.1023/A:1026671531500
Galloway, A. W. E., and Budge, S. M. (2020). The Critical Importance of Experimentation in Biomarker-Based Trophic Ecology. Phil. Trans. R. Soc. B 375, 20190638. doi:10.1098/rstb.2019.0638
Gladyshev, M. I., Kolmakov, V. I., Dubovskaya, O. P., and Ivanova, E. A. (2000). Studying of Algae Food Composition of Daphnia Longispina during Bluegreen Bloom of Eutrophic Pond. Doklady Akademii Nauk 371, 556–558.
Gladyshev, M. I., Semenchenko, V. P., Dubovskaya, O. P., Fefilova, E. B., Makhutova, O. N., Buseva, Z. F., et al. (2011b). Effect of Temperature on Contents of Essential Highly Unsaturated Fatty Acids in Freshwater Zooplankton. Limnologica 41, 339–347. doi:10.1016/j.limno.2011.03.001
Gladyshev, M. I., Sushchik, N. N., Anishchenko, O. V., Makhutova, O. N., Kolmakov, V. I., Kalachova, G. S., et al. (2011a). Efficiency of Transfer of Essential Polyunsaturated Fatty Acids versus Organic Carbon from Producers to Consumers in a Eutrophic Reservoir. Oecologia 165, 521–531. doi:10.1007/s00442-010-1843-6
Gladyshev, M. I., Sushchik, N. N., Dubovskaya, O. P., Buseva, Z. F., Makhutova, O. N., Fefilova, E. B., et al. (2015). Fatty Acid Composition of Cladocera and Copepoda from Lakes of Contrasting Temperature. Freshw. Biol. 60, 373–386. doi:10.1111/fwb.12499
Gladyshev, M. I., Sushchik, N. N., Dubovskaya, O. P., Makhutova, O. N., and Kalachova, G. S. (2006). Influence of Sestonic Elemental and Essential Fatty Acid Contents in a Eutrophic Reservoir in Siberia on Population Growth of Daphnia (Longispina Group). J. Plankton Res. 28, 907–917. doi:10.1093/plankt/fbl028
Gladyshev, M. I., Sushchik, N. N., Kolmakova, A. A., Kalachova, G. S., Kravchuk, E. S., Ivanova, E. A., et al. (2007). Seasonal Correlations of Elemental and ω3 PUFA Composition of Seston and Dominant Phytoplankton Species in a Eutrophic Siberian Reservoir. Aquat. Ecol. 41, 9–23. doi:10.1007/s10452-006-9040-8
Gladyshev, M. I., Sushchik, N. N., Makhutova, O. N., Kalacheva, G. S., Kolmakova, A. A., Kravchuk, E. S., et al. (2009). Efficiency of Transfer of Essential Polyunsaturated Fatty Acids along Trophic Chains in Aquatic Ecosystems. Dokl Biochem. Biophys. 426, 158–160. doi:10.1134/S1607672909030089
Gliwicz, Z. M. (2003). Between Hazards of Starvation and Risk of Predation: The Ecology of Off-Shore Animals Excellence in Ecology, Book 12. Oldendorf/Luhe, Germany: International Ecology Institute.
Goulden, C. E., and Place, A. R. (1990). Fatty Acid Synthesis and Accumulation Rates in Daphniids. J. Exp. Zool. 256, 168–178. doi:10.1002/jez.1402560207
Gulati, R., and DeMott, W. (1997). The Role of Food Quality for Zooplankton: Remarks on the State‐of‐the‐art, Perspectives and Priorities. Freshw. Biol. 38, 753–768. doi:10.1046/j.1365-2427.1997.00275.x
Guo, F., Bunn, S. E., Brett, M. T., Fry, B., Hager, H., Ouyang, X., et al. (2018). Feeding Strategies for the Acquisition of High‐quality Food Sources in Stream Macroinvertebrates: Collecting, Integrating, and Mixed Feeding. Limnol. Oceanogr. 63, 1964–1978. doi:10.1002/lno.10818
He, X., and Wang, W.-X. (2020). Allocation and Stoichiometric Regulation of Phosphorus in a Freshwater Zooplankton under Limited Conditions: Implication for Nutrient Cycling. Sci. Total Environ. 728, 138795. doi:10.1016/j.scitotenv.2020.138795
Heisler, J., Glibert, P. M., Burkholder, J. M., Anderson, D. M., Cochlan, W., Dennison, W. C., et al. (2008). Eutrophication and Harmful Algal Blooms: A Scientific Consensus. Harmful Algae 8, 3–13. doi:10.1016/j.hal.2008.08.006
Hessen, D. O., and Leu, E. (2006). Trophic Transfer and Trophic Modification of Fatty Acids in High Arctic Lakes. Freshw. Biol. 51, 1987–1998. doi:10.1111/j.1365-2427.2006.01619.x
Ikeda, T., Hirakawa, K., and Shiga, N. (2002). Production, Metabolism and Production/biomass (P/B) Ratio of Metridia pacifica (Crustacea; Copepoda) in Toyama Bay, Southern Japan Sea. Plankton Biol. Ecol. 49, 58–65.
Ikeda, T., and Shiga, N. (1999). Production, Metabolism and Production/biomass (P/B) Ratio of Themisto Japonica (Crustacea: Amphipoda) in Toyama Bay, Southern Japan Sea. J. Plankton Res. 21, 299–308. doi:10.1093/plankt/21.2.299
Jardine, T. D., Galloway, A. W. E., and Kainz, M. J. (2020). Unlocking the Power of Fatty Acids as Dietary Tracers and Metabolic Signals in Fishes and Aquatic Invertebrates. Phil. Trans. R. Soc. B 375, 20190639. doi:10.1098/rstb.2019.0639
Kabeya, N., Fonseca, M. M., Ferrier, D. E. K., Navarro, J. C., Bay, L. K., Francis, D. S., et al. (2018). Genes for De Novo Biosynthesis of omega-3 Polyunsaturated Fatty Acids Are Widespread in Animals. Sci. Adv. 4, eaar6849. doi:10.1126/sciadv.aar6849
Kainz, M., Arts, M. T., and Mazumder, A. (2004). Essential Fatty Acids in the Planktonic Food Web and Their Ecological Role for Higher Trophic Levels. Limnol. Oceanogr. 49, 1784–1793. doi:10.4319/lo.2004.49.5.1784
Karpowicz, M., and Ejsmont-Karabin, J. (2021). Diversity and Structure of Pelagic Zooplankton (Crustacea, Rotifera) in NE Poland. Water 13, 456. doi:10.3390/w13040456
Karpowicz, M., and Ejsmont-Karabin, J. (2017). Effect of Metalimnetic Gradient on Phytoplankton and Zooplankton (Rotifera, Crustacea) Communities in Different Trophic Conditions. Environ. Monit. Assess. 189, 367. doi:10.1007/s10661-017-6055-7
Karpowicz, M., Ejsmont-Karabin, J., Kozłowska, J., Feniova, I., and Dzialowski, A. R. (2020). Zooplankton Community Responses to Oxygen Stress. Water 12, 706. doi:10.3390/w12030706
Karpowicz, M., Feniova, I., Gladyshev, M. I., Ejsmont‐Karabin, J., Górniak, A., Sushchik, N. N., et al. (2021). Transfer Efficiency of Carbon, Nutrients, and Polyunsaturated Fatty Acids in Planktonic Food Webs under Different Environmental Conditions. Ecol. Evol. 11, 8201–8214. doi:10.1002/ece3.7651
Karpowicz, M., Feniova, I., Gladyshev, M. I., Ejsmont-Karabin, J., Górniak, A., Zieliński, P., et al. (2019). The Stoichiometric Ratios (C:N:P) in a Pelagic Food Web under Experimental Conditions. Limnologica 77, 125690. doi:10.1016/j.limno.2019.125690
Kring, S. A., Figary, S. E., Boyer, G. L., Watson, S. B., and Twiss, M. R. (2014). Rapid In Situ Measures of Phytoplankton Communities Using the Bbe FluoroProbe: Evaluation of Spectral Calibration, Instrument Intercompatibility, and Performance Range. Can. J. Fish. Aquat. Sci. 71, 1087–1095. doi:10.1139/cjfas-2013-0599
Lacroix, G., Lescher-Moutoué, F., and Bertolo, A. (1999). Biomass and Production of Plankton in Shallow and Deep Lakes : Are There General Patterns ?. Ann. Limnol. - Int. J. Lim. 35, 111–122. doi:10.1051/limn/1999016
Lands, W. E. M. (2009). “Human Life: Caught in the Food Web,” in Lipids in Aquatic Ecosystems. Editors M. T. Arts, M. Kainz, and M. T. Brett (New York: Springer), 327–354. doi:10.1007/978-0-387-89366-2_14
Lau, D. C. P., Vrede, T., Pickova, J., and Goedkoop, W. (2012). Fatty Acid Composition of Consumers in Boreal Lakes - Variation across Species, Space and Time. Freshw. Biol. 57, 24–38. doi:10.1111/j.1365-2427.2011.02690.x
Makhutova, O. N., Protasov, A. A., Gladyshev, M. I., Sylaieva, A. A., Sushchik, N. N., Morozovskaya, I. A., et al. (2013). Feeding Spectra of Bivalve Mollusks Unio and Dreissena from Kanevskoe Reservoir, Ukraine: Are They Food Competitors or Not?. Zool. Stud. 52, 56. doi:10.1186/1810-522x-52-56
Murphy, J., and Riley, J. P. (1962). A Modified Single Solution Method for the Determination of Phosphate in Natural Waters. Analytica Chim. Acta 27, 31–36. doi:10.1016/S0003-2670(00)88444-5
Napolitano, G. E. (1999). “Fatty Acids as Trophic and Chemical Markers in Freshwater Ecosystems,” in Lipids in Freshwater Ecosystems. Editors M. T. ArtsandB, and C. Wainman (New York: Springer), 21–44. doi:10.1007/978-1-4612-0547-0_3
Persson, J., and Vrede, T. (2006). Polyunsaturated Fatty Acids in Zooplankton: Variation Due to Taxonomy and Trophic Position. Freshw. Biol. 51, 887–900. doi:10.1111/j.1365-2427.2006.01540.x
Petrosyan, V. G. (2014). The Integrated Database Management System and the Statistical Analysis of Biological Data. Moscow: Biosystem office. Russian Federal Service for Intellectual Property. Certificate 2014663194, Date of registration - 18.12.2014, Available at: http://www1.fips.ru/fips_servl/fips_servlet?DB=EVM&DocNumber=2014663194&TypeFile=html.
Prater, C., Wagner, N. D., and Frost, P. C. (2018). Seasonal Effects of Food Quality and Temperature on Body Stoichiometry, Biochemistry, and Biomass Production in Daphnia Populations. Limnol. Oceanogr. 63, 1727–1740. doi:10.1002/lno.10803
R Core Team (2017). R: A Language and Environment for Statistical Computing. Vienna, Austria: R Foundation for Statistical Computing. Available at: https://www.R-project.org/.
Ravet, J. L., Brett, M. T., and Arhonditsis, G. B. (2010). The Effects of Seston Lipids on Zooplankton Fatty Acid Composition in Lake Washington, Washington, USA. Ecology 91, 180–190. doi:10.1890/08-2037.1
Robin, J. H. (1995). Effect of Diets Containing γ-Linolenic Acid on N-6 Highly Unsaturated Fatty Acid Content of Rotifer (Brachionus plicatilis). Hydrobiologia 313-314, 185–190. doi:10.1007/BF00025949
Sargent, J. R., Tocher, D. R., and Bell, J. G. (2002). “The Lipids,” in Fish Nutrition. Editors J. E. Halver, and R. W. Hardy 3rd edn (San Diego: Academic Press), 181–257.
Schoo, K. L., Malzahn, A. M., Krause, E., and Boersma, M. (2013). Increased Carbon Dioxide Availability Alters Phytoplankton Stoichiometry and Affects Carbon Cycling and Growth of a marine Planktonic Herbivore. Mar. Biol. 160, 2145–2155. doi:10.1007/s00227-012-2121-4
Semenchenko, V. P., Razlutskij, V. I., Feniova, I. Y., and Aibulatov, D. N. (2007). Biotic Relations Affecting Species Structure in Zooplankton Communities. Hydrobiologia 579, 219–231. doi:10.1007/s10750-006-0411-x
Sinicyna, O. O., and Zdanowski, B. (2007). Development of the Zebra Mussel, Dreissena polymorpha (Pall.), Population in a Heated Lakes Ecosystem. II. Life Strategy. Arch. Pol. Fish. 15, 387–400.
Smyntek, P. M., Teece, M. A., Schulz, K. L., and Storch, A. J. (2008). Taxonomic Differences in the Essential Fatty Acid Composition of Groups of Freshwater Zooplankton Relate to Reproductive Demands and Generation Time. Freshw. Biol. 53, 1768–1782. doi:10.1111/j.1365-2427.2008.02001.x
Sterner, R. W., and Schulz, K. L. (1998). Zooplankton Nutrition: Recent Progress and a Reality Check. Aquat. Ecol. 32, 261–279. doi:10.1023/a:1009949400573
Stockwell, J. D., and Johannsson, O. E. (1997). Temperature-dependent Allometric Models to Estimate Zooplankton Production in Temperate Freshwater Lakes. Can. J. Fish. Aquat. Sci. 54, 2350–2360. doi:10.1139/f97-141
Taipale, S. J., Vuorio, K., Brett, M. T., Peltomaa, E., Hiltunen, M., and Kankaala, P. (2016). Lake Zooplankton δ 13 C Values Are Strongly Correlated with the δ 13 C Values of Distinct Phytoplankton Taxa. Ecosphere 7, e01392. doi:10.1002/ecs2.1392
Twining, C. W., Taipale, S. J., Ruess, L., Bec, A., Martin-Creuzburg, D., and Kainz, M. J. (2020). Stable Isotopes of Fatty Acids: Current and Future Perspectives for Advancing Trophic Ecology. Phil. Trans. R. Soc. B 375, 20190641. doi:10.1098/rstb.2019.0641
Urabe, J., and Watanabe, Y. (1992). Possibility of N or P Limitation for Planktonic Cladocerans: an Experimental Test. Limnol. Oceanogr. 37, 244–251. doi:10.4319/lo.1992.37.2.0244
Uttaro, A. (2006). Biosynthesis of Polyunsaturated Fatty Acids in Lower Eukaryotes. IUBMB Life (International Union Biochem. Mol. Biol. Life) 58, 563–571. doi:10.1080/15216540600920899
Vanni, M. J., and Layne, C. D. (1997). Nutrient Recycling and Herbivory as Mechanisms in the "Top-Down" Effect of Fish on Algae in Lakes. Ecology 78, 21–40. doi:10.1890/0012-9658(1997)078[0021:nraham]2.0.co;2
Vizcaíno-Ochoa, V., Lazo, J. P., Barón-Sevilla, B., and Drawbridge, M. A. (2010). The Effect of Dietary Docosahexaenoic Acid (DHA) on Growth, Survival and Pigmentation of California Halibut Paralichthys californicus Larvae (Ayres, 1810). Aquaculture 302, 228–234. doi:10.1016/j.aquaculture.2010.02.022
von Elert, E. (2002). Determination of Limiting Polyunsaturated Fatty Acids in Daphnia Galeata Using a New Method to Enrich Food Algae with Single Fatty Acids. Limnol. Oceanogr. 47, 1764–1773. doi:10.4319/lo.2002.47.6.1764
Wacker, A., and von Elert, E. (2001). Polyunsaturated Fatty Acids: Evidence for Non-substitutable Biochemical Resources in Daphnia Galeata. Ecology 82, 2507–2520. doi:10.1890/0012-9658(2001)082[2507:PFAEFN]2.0.CO;2
Wagner, N. D., Lankadurai, B. P., Simpson, M. J., Simpson, A. J., and Frost, P. C. (2015). Metabolomic Differentiation of Nutritional Stress in an Aquatic Invertebrate. Physiol. Biochem. Zool. 88, 43–52. doi:10.1086/679637
Wilson, A. E. (2003). Effects of Zebra Mussels on Phytoplankton and Ciliates: a Field Mesocosm experiment. J. Plankton Res. 25, 905–915. doi:10.1093/plankt/25.8.905
Wojtal-Frankiewicz, A., and Frankiewicz, P. (2011). The Impact of Pelagic (Daphnia Longispina) and Benthic (Dreissena polymorpha) Filter Feeders on Chlorophyll and Nutrient Concentration. Limnologica 41, 191–200. doi:10.1016/j.limno.2010.09.001
Keywords: fish, zebra mussels, nitrogen, phosphorus, food quality
Citation: Feniova I, Karpowicz M, Gladyshev M, Sushchik N, Petrosyan V, Sakharova E and Dzialowski A (2021) Effects of Macrobiota on the Transfer Efficiency of Essential Elements and Fatty Acids From Phytoplankton to Zooplankton Under Eutrophic Conditions. Front. Environ. Sci. 9:739014. doi: 10.3389/fenvs.2021.739014
Received: 09 July 2021; Accepted: 20 August 2021;
Published: 12 October 2021.
Edited by:
Xiufeng Zhang, Jinan University, ChinaReviewed by:
Jeyaraj Antony Johnson, Wildlife Institute of India, IndiaQinghui Huang, Tongji University, China
Copyright © 2021 Feniova, Karpowicz, Gladyshev, Sushchik, Petrosyan, Sakharova and Dzialowski. This is an open-access article distributed under the terms of the Creative Commons Attribution License (CC BY). The use, distribution or reproduction in other forums is permitted, provided the original author(s) and the copyright owner(s) are credited and that the original publication in this journal is cited, in accordance with accepted academic practice. No use, distribution or reproduction is permitted which does not comply with these terms.
*Correspondence: Irina Yu Feniova, ZmVuaW92YUBtYWlsLnJ1