- 1Department of Water Protection Engineering and Environmental Microbiology, Faculty of Geoengineering, University of Warmia and Mazury in Olsztyn, Olsztyn, Poland
- 2Department of Environmental Biotechnology, Faculty of Geoengineering, University of Warmia and Mazury in Olsztyn, Olsztyn, Poland
- 3Microbiology Unit, Institute for Ecology of Industrial Areas, Katowice, Poland
- 4Faculty of Organization and Management, Silesian University of Technology, Gliwice, Poland
The diversity of beta-lactam antibiotic resistance genes, with particular emphasis on carbapenemase genes, during the treatment process at two wastewater treatment plants (WWTPs) with different levels of hospital wastewater inflow was investigated using high-throughput sequencing. An additional aspect of the study was to determine the taxonomic diversity of microorganisms in the studied samples. The obtained results suggest that bacteria of the Fusobacteriaceae family, not associated to date with this phenomenon, may be involved in the spread of antibiotic resistance in the environment. In samples from both wastewater treatment plants, the dominant beta-lactamase genes included blaOXA, blaGES, blaBEL, blaCfxA, and blaTEM. It is worth noting that the blaKPC and blaNDM genes were only found in untreated municipal wastewater with a higher hospital wastewater content. Moreover, an increase in the abundance of the blaIMP gene after the biological treatment stage in the studied treatment plants was found. In wastewater characterized by a higher proportion of hospital wastewater, 94 correlations were observed, while in wastewater with its lower proportion, 41 correlations were noted. Considering the above, the current research indicates that the inflow of hospital wastewater contributes to the spread of antibiotic resistance in the aquatic environment.
Introduction
Antibiotics belonging to the beta-lactam group are used in human medicine in the greatest quantities (Fernandez et al., 2017). In its 2018 report, the World Health Organization (WHO) stated that beta-lactam antibiotics are the most commonly used antibiotics worldwide (WHO, 2018). The WHO Collaborating Centre classifies beta-lactam antibiotics into two groups: 1) beta-lactam antibacterials, 2) penicillins and other beta-lactam antibacterials, including carbapenems. Antibiotic consumption is determined using the Defined Daily Dose (DDD) unit per 1,000 inhabitants per day. In a 2020 report, the European Centre for Disease Prevention and Control (ECDC) determined the average antibacterial consumption to be 19.4 DDD per 1,000 inhabitants per day in Europe. The European country with the highest consumption of antibiotics from the above-mentioned groups was Greece. The Netherlands had the lowest consumption, with Poland ranked 11th among European countries (ECDC, 2020; Buta et al., 2021).
Given the very high use of beta-lactam antibiotics, genes conferring resistance to this group of antibiotics have begun to pose a hazard to public health due to their widespread occurrence. Beta-lactam resistance genes can be classified and divided into four classes (the Ambler classification) (Bush, 2018). Classes A, C, and D include beta-lactamases with a serine active center, while class B includes enzymes with auxiliary divalent zinc cofactor (Bonomo, 2017). Penicillinase, first described in 1940, belonged to class C and is now known as AmpC present in Escherichia coli (Abraham and Chain, 1940). The literature mostly mentions the beta-lactamases originating from microorganisms possessing AmpC, including MIR, DHA, ACC, MOX, EBC, and FOX types, with the CMY-2 subtype being the most widespread one of them (Nikonorow et al., 2013; Chaturvedi et al., 2020). Many of the class A and D beta-lactamases are classified as extended-spectrum beta-lactamases (ESBLs) such as TEM, SHV, and CTX-M which are among the most abundant ESBLs worldwide. Other, less commonly found ESBLs belonging to the GES, PER, and VEB types, have also been described (Dallenne et al., 2010). Ghafourian et al. (2015) reported that over 100 TEM-type beta-lactamases were determined, of which only TEM-1 and TEM-2 did not encode ESBLs. Moreover, the presence of a wide range of SHV and CTX-M beta-lactamase variants was confirmed. The above-mentioned beta-lactamases (TEM, SHV, and CTX-M) have been identified in many representatives of the Enterobacteriaceae family (Escherichia coli, Klebsiella pneumoniae), and in bacteria of the Pseudomonas and Acinetobacter genera. It is noteworthy that while the above-mentioned microorganisms are associated with the natural environment (river water) or wastewater, they can also cause infections in hospitals. Of all the beta-lactamase classes, carbapenem antibiotic-hydrolyzing beta-lactamases (very important to public health) are currently found in three of them, i.e., classes A, B, and D. The most commonly discussed class A carbapenemase-encoding genes include KPC and GES types; for class B—NDM, IMP, and VIM types; for class D, these include OXA-23, -24, -51, -58, and OXA-48-related subtypes. Frequently described variants of carbapenemase-encoding gene types in municipal wastewater, hospital wastewater and river water include NDM-1, NDM-5, NDM-7, KPC-2, VIM-1, VIM-2, GES-5, and OXA-48 (Yang et al., 2016; Nasri et al., 2017; Mathys et al., 2019).
A particular hazard to human health and life is posed by Gram-negative bacteria carrying beta-lactamase genes, which are capable of rapidly acquiring subsequent antibiotic resistance determinants (Khurana et al., 2017). Currently, the greatest threat to hospital patients’ health is presented by ESBL-encoding genes conferring resistance to penicillins as well as first-, second-, and third-generation cephalosporins and aztreonam (Sonda et al., 2016), and genes encoding carbapenemases hydrolyzing carbapenem antibiotics. Both enzyme groups are produced by bacteria of the Enterobacteriaceae family and other Gram-negative bacteria (Khan et al., 2017). The hospital outbreaks triggered by the above-mentioned microorganisms are bacterial infections which are very difficult to treat (Müller et al., 2016). The presence of ESBL-producing bacteria was found on various usable hospital surfaces (Muzslay et al., 2017). Rapid dissemination of these antimicrobial-resistant bacteria, coupled with the unavailability of effective antibiotics, contributed to the warning issued by the WHO of the “post-antimicrobial era” in which the world will be afflicted by widespread infections (Nwafia et al., 2019; Matar et al., 2020).
In recent years, numerous studies have demonstrated the presence of antibiotic-resistant bacteria (ARB), antibiotic resistance genes (ARGs), and unmetabolized antibiotics in hospital or municipal wastewater, as well as the possible release of these pollutants into the natural environment (Röderová et al., 2016). In hospital wastewater, both ESBL-encoding genes (blaTEM, blaSHV, blaCTX-M) (Adelowo et al., 2018; Haller et al., 2018; Banjo et al., 2020) and carbapenemase-encoding genes (blaKPC, blaVIM, blaIMP, blaOXA-48, blaNDM) (Nasri et al., 2017; Jin et al., 2018; Cahill et al., 2019) were detected. The literature indicates that hospital wastewater, as compared to municipal wastewater, features a significantly higher diversity and abundance of ARGs, including ESBL-, and carbapenemase-encoding genes (Hassoun-Kheir et al., 2020).
Since wastewater treatment plants employing conventional treatment methods do not completely reduce antibiotic-resistant bacteria and drug resistance genes found in the inflowing wastewater (Osińska et al., 2020; Zieliński et al., 2020), more knowledge is needed on the fate of ARGs originating from hospital wastewater during the treatment process. It is also necessary to determine the role played by hospital wastewater inflowing to WWTP in the spread of antibiotic resistance genes in the environment. Therefore, this study aimed to determine the diversity of beta-lactam resistance genes, with particular emphasis on carbapenemase genes, during the treatment process at two WWTPs receiving different levels of hospital wastewater inflow. The metagenomic analysis employed in the study enabled the determination of the taxonomic diversity of microorganisms in the studied samples.
Materials and Methods
Sampling Sites
Samples for analysis were collected from two WWTPs with different levels of hospital wastewater inflow relative to the total amount of wastewater reaching the treatment facilities. The first WWTP is located in the region of Warmia and Mazury (WM-WWTP), and the second WWTP is located in the region of Silesia (S-WWTP) in Poland (Europe).
Although both WWTPs deploy mechanical and biological wastewater treatment systems, additional phosphorus chemical removal technology was implemented in S-WWTP. WM-WWTP processes wastewater from the capital city of the region of Warmia and Mazury (Northern Poland) and four surrounding municipalities and it has an average daily processing capacity of 35,000 m3. The plant receives wastewater from four hospitals, which accounts for 2% of the total treated wastewater. WM-WWTP has a population equivalent (PE) of 260,361, and treated wastewater is characterized by a chemical oxygen demand (COD) of 49 mg/L and biological oxygen demand (BOD) of 6 mg/L. The processing technology involves mechanical treatment systems with automated screens for separating large solids, two horizontal sand filters and two sedimentation tanks. The biological treatment system is composed of phosphate and nitrogen removal chambers and five multifunctional bioreactors equipped with four Passavant aerating rotors each and secondary sedimentation tanks. Sewage sludge is inactivated in closed and open digestion chambers and it is dehydrated by a mechanical filter press. Processed wastewater is discharged to a river (Kowalska et al., 2014).
S-WWTP (Southern Poland) receives 32,731 m3 of wastewater each day and has a PE of 403,138. Hospital wastewater accounts for 0.4% of the wastewater processed by S-WWTP. Wastewater entering the plant via trunk collectors is pumped to a pre-treatment line composed of hook screens, belt conveyors, and a manual screen for the separation of large solids. Pretreated wastewater is then passed through horizontal sand filters. Filtered wastewater undergoes biological treatment in the C-TECH reactor. In the first stage, phosphorus is precipitated chemically with an iron coagulant. Wastewater is then directed to the reactor where it is treated in a repeated series of aeration, sedimentation, and decantation processes. The last stage of biological treatment involves phosphorus removal, denitrification, and nitrification. The generated sewage sludge is neutralized in anaerobic digestion chambers and it is dehydrated in a high-speed decanter centrifuge and a belt filter press (RCGW, 2020). Schematic diagrams of the analyzed WWTPs are presented in Supplementary Figures S1, S2 in the supplementary materials.
Sample Collection
Samples of wastewater and sewage sludge were collected in June (2018) (summer), November (2018) (autumn), and March (2019) (spring). Wastewater and sewage sludge were sampled from different stages of treatment in both WWTPs. The sampling sites are described in detail in Supplementary Table S1; Supplementary Figures S1, S2.
Samples of wastewater were collected into sterile 500 ml bottles (SIMAX) and sewage sludge samples were collected using 100 ml sterile plastic tubes. Wastewater was sampled in triplicate at hourly intervals for 24 h to obtain representative samples and the collected hourly samples were pooled into a composite sample. Samples of sewage sludge were also collected in triplicate to create complex samples. The samples were transported to the laboratory at a temperature of 4°C.
DNA Extraction and Sequencing
Metagenomic DNA was extracted from wastewater using the Power Water (MoBio Laboratories Inc., CA, United States) kit and from sewage sludge using the Power Soil kit (MoBio Laboratories Inc., CA, United States) following the manufacturer’s instructions. Samples were quantified by the picogreen method using Victor 3 fluorometry and their quality and quantity were assessed. Illumina TruSeq DNA PCR-Free libraries were prepared manually following the manufacturer’s protocol TruSeq DNA PCR-Free Sample Preparation Guide, Part #15036187 Rev. D (Illumina, San Diego, CA, United States). The libraries were tested using LightCycle qPCR and the size distribution was assessed by an Agilent Technologies 2100 Bioanalyzer using a DNA 1000 chip and sequenced on a NovaSeq6000 in an S4 flowcell lane using a 2 × 150 bp configuration.
Bioinformatic Analysis
Forty-five samples from three seasons were used for metagenomics analysis. Fastq sequences were initially quality filtered, including adaptor-trimming using Trimmomatic v 0.39 (Bolger et al., 2014) with default parameters. Paired-end sequences were merged using PANDAseq (Masella et al., 2012). Reads that software could not merge were excluded from further analyses. The data sequences then underwent quality control using the fastx toolkit 0.0.14-5 (http://hannonlab.cshl.edu/fastx_toolkit/) with the fastq_quality_filter tool. Data filtration excluded all reads from analyses that did not reach a minimum of 80% of bases set at a quality score of q = 20. Using Seqtk (https://github.com/lh3/seqtk), fastq files were converted to fasta files for the BLAST alignment.
Subsequently, metagenomic sequences were analyzed by a BLASTx- type search against the Comprehensive Antibiotic Resistance Database (CARD) (McArthur et al., 2013) (https://card.mcmaster.ca; October 2019 3.0.7). Samples were parsed and filtered to collect the top hits for each sample considering positive-hit reads with an E value ≤ 1 × 10−10 (Escudeiro et al., 2019); amino acid identity ≥90% (Casaburi et al., 2019) and alignment length ≥25 amino acids (Kristiansson et al., 2011; Zhang et al., 2011; Yang et al., 2013; Su et al., 2017).
Database of integrons and insertion sequences was created by downloading DNA sequences from the INTEGRALL (https://integrall.bio.ua.pt). Sequences belonging to integrons or IS-like sequences were identified by aligning the sequences against sequences in the database using BLASTn with E value ≤ 1 × 10−10 (Tian et al., 2016). A read was annotated to be a resistance gene if its BLASTn hit for the alignment against the INTEGRALL database had a ≥90% nucleotide read identity for ≥100 bp.
Sequencing results in the form of FASTQ files were uploaded to the MetaGenome Rapid Annotation Subsystems Technology (MG-RAST) server for analysis (6). Each file underwent quality control (QC), which included quality filtering (removing sequences with ≥5 ambiguous base pairs), and length filtering (removing sequences with a length ≥2 standard deviations from the mean). The UCLUST algorithm was used to cluster the identified rRNA sequences. A representative sequence of each cluster was used for taxonomic identification based on the Greengenes reference database. The metagenome data are deposited in the NCBI Sequence Read Archive (SRA) under accessions NCBI SRA SRP286056.
For further analyses, the results obtained from samples collected from three research seasons (summer, autumn, and spring) were averaged.
Statistical Analysis
To investigate the co-occurrence patterns of microbial community and resistome, correlation matrixes were constructed by calculating all pairwise Spearman rank correlations. A correlation between any two items was considered statistically robust if the Spearman correlation coefficient (ρ) was >0.7 and the p value was <0.01 (Barberán et al., 2012). The resulting correlation matrixes were translated into an association network using Gephi 0.9.1 (Bastian et al., 2009).
Results
Overview of Metagenomic Analysis
The study used Illumina deep sequencing to identify ARGs, mobile genetic elements (MGEs), and the taxonomic structure in samples of wastewater and sewage sludge. The sequencing enabled generating >250 Gb of raw data from 15 samples and a number of reads ranging from 94.8 to 129.5 million (Supplementary Table S2). The databases identified the occurrence of 1,906 ARGs and class 1, 2, and 3 integron integrase genes. Antibiotic resistance genes from the beta-lactamase group accounted for 67.3% of genes in samples from WM-WWTP and for 62.7% of genes from S-WWTP. The study focused mainly on carbapenem resistance genes, i.e., the blaGES, blaIMP, blaKPC, blaNDM, blaVIM gene types and blaOXA-23, blaOXA-24, blaOXA-48, and blaOXA-58 gene subtypes. Only subtypes conferring resistance to carbapenems (GES-11, -14, -18, -2, -20, -4, -5, -6) were selected from the blaGES type gene group (Naas et al., 2016; Bonomo, 2017).
Taxonomic Structure
In the studied samples, 22 phyla from the Bacteria domain (82%) and three phyla from the Archaea domain (12%) were identified. Moreover, the occurrence of 17 classes from four phyla (Proteobacteria, Firmicutes, Bacteroidetes, and Euryarchaeota) was determined and attention was paid to three families because of the association of these families with beta-lactamases and frequent causation of nosocomial infections (Enterobacteriaceae, Moraxellaceae, and Fusobacteriaceae) (Supplementary Figures S2–S4). The data concerning microorganism diversity, averaged for three seasons (including standard deviations) at particular sampling sites, are presented in Supplementary materials (Supplementary Tables S3–S8).
Statistical analysis demonstrated an absence of statistically significant differences between the occurrence of specific microorganism phyla, classes, or families in wastewater at subsequent stages of the treatment system (p > 0.05) (Supplementary Tables S8–S10). Of all the phyla found in wastewater from both wastewater treatment plants, Proteobacteria, Bacteroidetes, and Firmicutes (38.5 and 39.6%, 31 and 29%, 9.1 and 14.9%, respectively in WM-WWTP and S-WWTP) were dominant (Supplementary Figure S3). At each stage of wastewater treatment, in both wastewater treatment plants, the microorganisms belonging to the Proteobacteria phylum were most abundant. A change in the proportion of these microorganisms between the inflowing and treated wastewater (52.5%:51%, and 48.4%:35.6%, percentage distribution in WM1:WM5 and S1:S5, respectively) was noted (Supplementary Figure S3). Moreover, a reduction in the number of the most abundant phyla of microorganisms during the wastewater treatment process amounted to 75.5%/87.8% for Proteobacteria, 58.1%/57.6% for Bacteroidetes, and 91.5%/94.1% for Firmicutes, in WM-WWTP/S-WWTP, respectively. The results indicate a reduction in the count of bacteria from the Firmicutes phylum in wastewater from both treatment plants, following the process of treatment using activated sludge (WM4/S3) when comparing WM1/S1 to WM4/S3 (23.6%/26.6%:3.4%/4.3%). In addition, the C-TECH reactor used in the S-WWTP decreased the count of these microorganisms with lower efficiency, when comparing the percentage occurrence of the Firmicutes phylum in wastewater from reactor S4 (14.8%) to the activated sludge chamber S3 (4.3%) (Supplementary Figure S3).
For the Euryarchaeota phylum (Archaea), the authors observed an increase in these microorganism counts up to 12% in the sample of sewage sludge (WM6) and leachate from sewage sludge (S6) for both studied wastewater treatment plants (Supplementary Figure S1). A deeper taxonomic analysis of this phylum determined the dominant proportion of the Methanomicrobia class in samples from these sites, amounting to 17 and 12%, respectively (Supplementary Figure S4).
Selected microorganism families (Enterobacteriaceae, Moraxellaceae, and Fusobacteriaceae) occurred more abundantly in samples from WM-WWTP than in the samples from S-WWTP and the Moraxellaceae family had the highest count (in wastewater from both treatment plants). In the samples of inflowing wastewater and treated wastewater from both treatment plants, the reduction in the count of microorganisms belonging to the mentioned families amounted to 85–99%.
Abundance of ARGs and MEs
In the studied samples, the presence of beta-lactam antibiotic resistance genes was detected, i.e. 1,283 genes encoding beta-lactamases were assigned to 58 types in samples from WM-WWTP and 1,195 genes represented 52 types in samples from S-WWTP (Supplementary Figures S6, S7). The particular numbers of gene copies are expressed in the “ppm” unit which represents “gene copy per 1 million reads.”
In samples from both wastewater treatment plants, the dominant beta-lactamase gene types included blaOXA, blaGES, blaBEL, blaCfxA, and blaTEM. In samples from the WM-WWTP, these genes occurred in the following ranges: 0.064–1.415 ppm, 0.008–2.358 ppm, 0.014–0.388 ppm, 0.008–0.98 ppm, and 0.008–0.758 ppm, while in samples from S-WWTP, these genes occurred in the following ranges: 0.041–1.765 ppm, 0.008–1.224 ppm, 0.019–0.425 ppm, 0.01–1.458 ppm, and 0.02–0.559 ppm, respectively (Supplementary Tables S12, S13). The difference in the number of gene copies determined in wastewater samples between the experimental treatment plants was not statistically significant (p > 0.05) (Supplementary Table S14). In samples from both treatment plants, the most abundant carbapenem resistance genes included the blaGES type and the blaOXA-23, blaOXA-24, and blaOXA-48 subtypes (Figure 1).
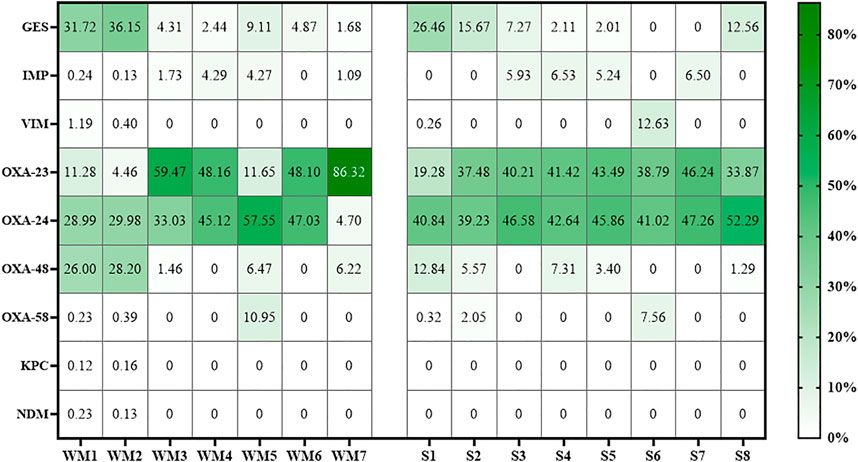
FIGURE 1. Percentage distribution of carbapenemase genes in samples from both WWTPs. Abbreviations: WM, wastewater treatment plant from the Warmia and Mazury region; S, wastewater treatment plant from the Silesia region.
No statistically significant differences were found in the abundance of the studied beta-lactam resistance genes between raw wastewater (WM1/S1) and treated wastewater (WM5/S5) (p > 0.05) (Supplementary Table S15). However, during the treatment process, a percentage increase was observed in the number of gene types occurring in the highest amounts in wastewater (blaOXA, blaBEL, blaCfxA, and blaTEM) (Supplementary Figures S6, S7).
Even though both studied wastewater treatment plants are based on the activated sludge technology, differences were found in the occurrence of certain beta-lactamases in wastewater samples from activated sludge chambers. Based on the percentage occurrence, an increase in the number of bla genes in samples after the treatment stage using activated sludge (WM4/S3) was noted for samples from a site with the inflowing wastewater (WM1/S1) (blaOXA 2.7-fold, blaBEL 4.5-fold in WM-WWTP, and blaTEM 2.9-fold, blaBEL 5.7-fold in S-WWTP) (Supplementary Figures S6, S7). It is worth noting the difference in the number of genes in samples from S-WWTP after the treatment site in the activated sludge chamber (S3) and after the C-TECH reactor (S4). The use of the C-TECH reactor reduced the number of genes in the effluent (Supplementary Figures S6, S7).
The processes employed at the initial treatment stage (WM1/WM2/S1/S2) enabled a great reduction in the abundance of carbapenemase genes from the inflowing wastewater. The blaNDM and blaKPC genes, which were only present in the wastewater inflowing to WM-WWTP, were eliminated from the wastewater after the mechanical treatment stage. For the blaIMP gene, in both treatment plants, the biological treatment system increased the amount of this gene (0.24:4.27% in wastewater from WM-WWTP, and 0.00:5.24% in wastewater from S-WWTP, WM1:WM5, and S1:S5, respectively) (Figure 1).
The occurrence of three mobile genetic elements, i.e., intI1, intI2, and intI3, was determined in the studied samples. Among these elements, in each studied sample from both treatment plants, intI1 constituted the majority (>98%) (Supplementary Figure S8). In samples from the WM-WWTP, integrase genes occurred in the following ranges: 328.59–2911.29 ppm, 0.36–34.4 ppm, and 2.41–10.02 ppm, while in samples from S-WWTP, they were detected in the following ranges: 166.59–2883.91 ppm, 0.02–29.87 ppm, and 0.09–2.46 ppm, for intI1, intI2, and intI3, respectively (Supplementary Tables S16, S17).
Correlation Analysis of ARGs, MGEs and Taxonomic Structure
Spearman’s rank statistical test demonstrated a higher number of statistically significant correlations (p < 0.01) between integrase genes and beta-lactam resistance genes in samples from WM-WWTP than in samples from S-WWTP (Figures 2, 3). In wastewater from the treatment plant receiving a higher percentage of hospital wastewater in the total volume of wastewater under treatment (the Warmia and Mazury region), statistically significant correlations were determined between the abundance of antibiotic resistance genes and intI genes, amounting to 38 for intI1; 33 for intI2 and 23 for intI3, while in the treatment plant with a lower percentage of hospital wastewater (the Silesia region)—it amounted to 22, 16, and 3 for intI1, intI2, and intI3, respectively. In samples from WM-WWTP, the presence of strong, positive, statistically significant correlations was observed between the abundance of the three studied integrase gene types and carbapenemase genes blaGES, blaVIM, blaOXA-24, blaOXA-48, blaOXA-58. The respective correlation coefficients were 0.57–0.74, 0.56–0.75, 0.64–0.79, 0.58–0.81, 0.58–0.7. In contrast, in samples from S-WWTP, the abundance of intI1 and intI2 genes correlated less strongly with the genes blaGES, blaOXA-23, blaOXA-24, blaOXA-48 than in samples from WM-WWTP (0.52–0.59, 0.5–0.64, 0.5–0.62, 0.58–0.62, respectively).
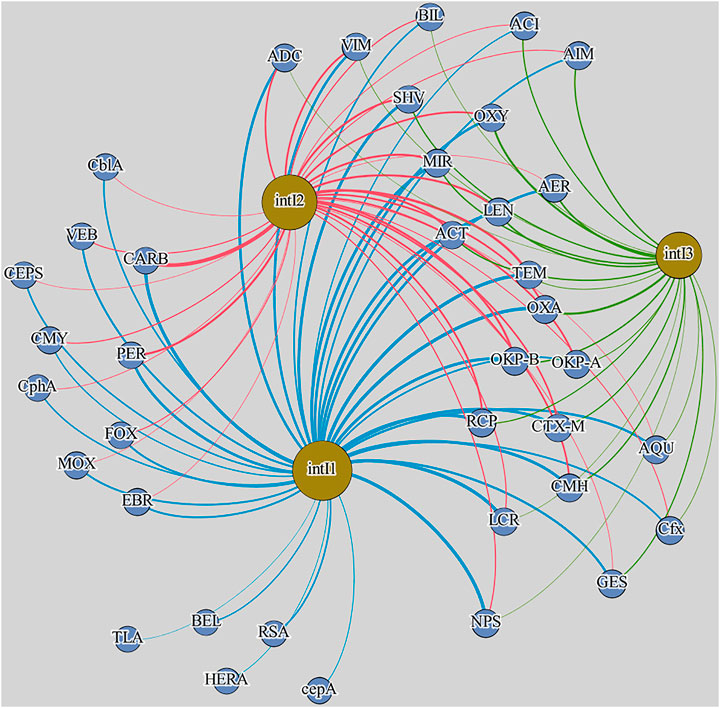
FIGURE 2. Network analysis of integrase and beta-lactamase genes in samples from WM-WWTP. All correlations in the diagram are statistically significant (p < 0.01). Edges: blue—intI1:beta-lactamase gene correlations, yellow—intI2:beta-lactamase gene correlations, pink—intI3:beta-lactamase gene correlations; Nodes: yellow—integron integrase genes, blue—beta-lactamase genes. WM-WWTP stands for the wastewater treatment plant from the Warmia and Mazury region.
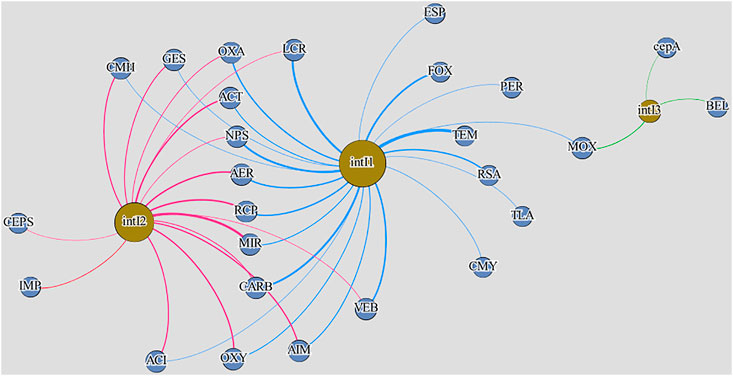
FIGURE 3. Network analysis of integrase and beta-lactamase genes in samples from S-WWTP. All correlations in the diagram are statistically significant (p < 0.01). Edges: blue—intI1:beta-lactamase gene correlations, yellow—intI2:beta-lactamase gene correlations, pink—intI3:beta-lactamase gene correlations; Nodes: yellow—integron integrase genes, blue—beta-lactamase genes. S-WWTP stands for the wastewater treatment plant from the Silesia region.
In samples from WM-WWTP, the abundance of the identified bacterial phyla, Fusobacteria and Proteobacteria, correlated strongly with class 1 integron integrase genes (0.56 and 0.45), class 2 integron integrase genes (0.53 and 0.45), and class 3 integron integrase genes (0.44 and 0.31). However, all of the above-mentioned correlations were statistically insignificant except for the correlation between the count of bacteria from the Fusobacteria phylum and the intI1 gene.
In samples from S-WWTP, only the number of class 1 and 2 intI genes correlated strongly with the number of microorganism phyla. The abundance of genes intI1 correlated strongly with the abundance of two phyla, Fusobacteria (0.59), and Proteobacteria (0.58), while the abundance of intI2 correlated strongly with the abundance of up to 10 phyla, i.e., Firmicutes (0.79), Euryarchaeota (0.72), Actinobacteria (0.7), Tenericutes (0.63), Bacteroidetes (0.61), Synergistetes (0.59), Spirochaetes (0.55), Crenarchaeota (0.54), Cyanobacteria (0.52), and Fusobacteria (0.5).
Samples from the treatment plant in the Silesia region were characterized by a higher number of statistically significant correlations between the abundance of microorganism phyla and carbapenem resistance genes. In samples from WM-WWTP, this only included the phylum of Fusobacteria, while in samples from S-WWTP, these phyla included Fusobacteria, Firmicutes, Bacteroidetes, Proteobacteria, and Dictyoglomi.
An analysis of Spearman’s correlations between the count of selected microorganism families and the abundance of integrase and carbapenemase genes determined a higher number of significant positive correlations between variables in samples from WM-WWTP (22 correlations) compared to samples from S-WWTP (19 correlations). In samples from both treatment plants, the abundance of intI1 and intI2 genes correlated significantly with the abundance of the families of Enterobacteriaceae, Moraxellaceae, and Fusobacteriaceae, however, only in samples from WM-WWTP can the emergence of significant correlations between the numbers of intI3 gene and Fusobacteriaceae (0.44) and Moraxellaceae (0.51) be observed. In samples from both treatment plants, the abundance of Fusobacteriaceae and Moraxellaceae correlated strongly with the number of carbapenem resistance genes (Supplementary Figures S9, S10).
Discussion
The taxonomic structure of the studied samples collected from the wastewater treatment plant was typical of samples of this type (Ye et al., 2016; Guo et al., 2017). The study results indicate the dominant proportion of bacteria from the Proteobacteria, Firmicutes, and Bacteroidetes phyla in the taxonomic structure of the studied samples. The literature confirms that bacteria from the Proteobacteria phylum are dominant in wastewater samples, even though the species belonging to this phylum account for a small percentage of the human gastrointestinal biota (Bäumlisberger et al., 2015). Microorganisms from the Firmicutes phylum, along with Bacteroidetes, are also among those most commonly found in wastewater samples (Singh et al., 2012; Wang et al., 2014). In the presented study, bacteria from the Firmicutes phylum occurred in higher numbers at the initial stages of the treatment process and their percentage in wastewater decreased over the subsequent stages of the treatment process. Bacteria belonging to the human gastrointestinal biota, including the Firmicutes phylum, often shape the taxonomic structure of inflowing wastewater, while at the later stages of treatment, their percentage is effectively reduced (Cai et al., 2014).
For an in-depth taxonomic analysis, the Enterobacteriaceae and Moraxellaceae families representing the Proteobacteria phylum were selected. These families include species carrying carbapenem resistance genes and causing outbreaks of nosocomial infections, such as carbapenem-resistant Enterobacteriaceae (CRE), and carbapenem-resistant Acinteobacter baumannii (CRAB). Currently, these species pose one of the greatest hazards to public health. Infections caused by these organisms are often linked to high morbidity and mortality (Grundmann et al., 2017; Iovleva and Doi, 2017; Logan and Weinstein, 2017; Isler et al., 2018; David et al., 2019; Hamidian and Nigro, 2019). Moreover, the study focused on microorganisms from the Fusobacteriaceae family since an analysis of the obtained study results indicated that these microorganisms may be a significant factor in harboring antibiotic resistance genes in the environment.
The study found that a greater diversity of beta-lactam resistance gene types was found in wastewater inflowing to WM-WWTP (which contained a higher percentage of hospital wastewater) than in samples from S-WWTP. The literature confirms that the inflow of hospital wastewater may induce a greater diversity of antibiotic resistance genes, including among beta-lactam antibiotic resistance gene types (Buelow et al., 2018; Khan et al., 2019). Researchers commonly describe hospital wastewater as a source of a wide spectrum of antibiotic-resistant bacteria and antibiotic resistance genes (Chagas et al., 2011; Korzeniewska and Harnisz, 2013; Hocquet et al., 2016; Rowe et al., 2017). The literature also identified a greater amount of drug resistance genes in wastewater originating from hospitals than in exclusively municipal wastewater by comparing the occurrence of beta-lactam antibiotic resistance genes (Zagui et al., 2020). The genes occurring most abundantly in samples from both treatment plants included blaTEM and blaOXA. Numerous literature reports confirm the presence of these genes in environmental samples (river water, wastewater) at high concentrations (Amador et al., 2015; Zieliński et al., 2019; Wang et al., 2020; Chaturvedi et al., 2021).
Based on the research results presented in this study, it was found that one of the carbapenem resistance genes, occurring in abundance in wastewater in both studied treatment plants, was blaGES. The literature indicates the occurrence of carbapenemase-resistance subtypes of blaGES in both wastewater (Conte et al., 2017; Wang et al., 2020) and bacterial strains isolated from wastewater (Kim, 2016; Araújo et al., 2021). As regards another carbapenemase-encoding gene (blaIMP), a significant increase in its amount was found in wastewater from both treatment plants after the biological treatment stage, which contributed to an increase in its occurrence in treated wastewater. At the stage of wastewater treatment in the activated sludge chamber, an increase in the number of antibiotic resistance genes can take place, which is most likely due to the high density of bacterial cells and the induced horizontal transfer of genes between microorganisms (Jiao et al., 2017). Jendrzejewska (2018) described a case of an increase in the amount of carbapenem resistance genes (blaGES and blaPER) at the biological treatment stage. Makowska et al. (2020) also indicated an increase in the concentration of genes such as blaKPC or blaNDM in wastewater after treatment using the biological method. Moreover, Wang et al. (2015) compared various activated sludge-based wastewater treatment systems and noted that an aeration tank with activated sludge induced an increase in the concentration of antibiotic resistance genes, which may pose a threat to the natural environment. Metagenomic analysis studies identified an activated sludge tank as a hotspot of various ARGs, independent of wastewater source, or treatment processes (Liu et al., 2019). Moreover, the literature describes the occurrence of carbapenem resistance genes in river water receiving treated wastewater (Chouchani et al., 2013; Khan et al., 2019). It is noteworthy that the genes blaNDM and blaKPC were present in the wastewater inflowing to WM-WWTP. Both genes are characteristic of the bacteria strongly associated with outbreaks of nosocomial infections, such as Klebsiella pneumoniae (Nasri et al., 2017; Teixeira et al., 2020) or Acinetobacter baumanii (Goudarzi et al., 2019). Lamba et al. (2017) concluded that the concentration of carbapenem-resistant bacteria of the Enterobacteriaceae family and the blaNDM gene was nine orders of magnitude higher in hospital wastewater than in local municipal wastewater.
Beta-lactamase and carbapenemase encoding genes are usually located on plasmids (the structure of which often includes integrons) or in the bacterial chromosome (Papp-Wallace et al., 2016; Perez et al., 2016; Bonomo, 2017). The literature identifies the presence of beta-lactam resistance genes from all Ambler classes in integron gene cassettes in environmental samples such as treated wastewater, sewage sludge, or soil (Gatica et al., 2016; Böhm et al., 2020). Despite sequence differences in the integrase gene, it was described that the same gene cassettes could be found in class 1 and 2 and class 1 and 3 integrons (Carattoli, 2001). In 1995, the occurrence of the same gene cassette carrying the blaIMP gene in class 3 integron, which was initially determined in class 1 integron, was described for the first time (Arakawa et al., 1995). In addition, the literature reports on the possibility of the occurrence of gene cassette rearrangement between class 1 integrons and class 3 integrons in an environment with a rich microbiome and resistome, such as wastewater (Stalder et al., 2012; Amos et al., 2015).
The metagenomic analysis conducted in this study shows the dominant proportion of the class 1 integron integrase gene in samples from both wastewater treatment plants. Mobile genetic elements such as intI1 genes are effective tools for bacterial adaptation and play a significant role in the transmission of antibiotic resistance. Previous studies have noted that anthropogenic environmental changes lead to the promotion of the abundance of integrase genes, particularly the intI1 gene (Stalder et al., 2012). The differences in the amounts of integrase genes demonstrated in this study, have also been confirmed in the literature (Guo et al., 2017; Piotrowska et al., 2017; Wang et al., 2017; An et al., 2018). Integrase genes are found in higher concentrations in wastewater of hospital origin (Timraz et al., 2017; Wang et al., 2018) compared to municipal wastewater (Di Cesare et al., 2016), which may explain the occurrence of the studied intI in higher amounts in samples from WM-WWTP.
Based on the analysis of links between the studied variables (beta-lactam resistance genes and genes encoding the integrase enzyme), more significant positive correlations were found in samples from the wastewater treatment plant receiving a higher load of hospital wastewater (WM-WWTP). The obtained study results suggest the increasing importance of the intI3 gene in antibiotic resistance gene dissemination in samples from wastewater treatment plants that receive hospital wastewater in addition to municipal wastewater. Positive, significant correlations were found between the amount of intI3 with an abundance of blaVIM and blaGES genes. In their study, Lamba et al. (2017) also described significant correlations between the abundance of beta-lactamase genes (blaCTX, blaNDM, blaTEM, and blaOXA) and the number of class 1 and 3 integrons, while their study results show that the abundance of class 2 integrons did not significantly correlate with the number of resistance genes. Simo Tchuinte et al. (2016) also highlight the role of class 3 integron in the spread of the antibiotic resistance phenomenon while describing the occurrence of this mobile genetic element harboring oxacillinase genes in effluent hospital wastewater. Shibata et al. (2003) found the occurrence of intI3 gene in clinical strains carrying genes from the metallo-β-lactamase group. Moreover, class 3 integrons may be involved in the spread of resistance both under clinical conditions and in the natural environment and may participate in the exchange of antibiotic resistance genes between these two environments (Barraud et al., 2013).
Wastewater constitutes a rich reservoir of bacteria and genes. The numerous links between them are well described (Yuan et al., 2018; Ju et al., 2019). In this study, the occurrence of the dominant Proteobacteria phylum was strongly related to the abundance of integrase- and carbapenemase-encoding genes. However, in the samples from WM-WWTP which received a major volume of hospital wastewater, highly statistically significant correlations were found between the occurrence of the Fusobacteria phylum and the abundance of carbapenemase genes, despite the occurrence of these bacteria in small amounts. Bacteria belonging to this phylum are mostly part of the natural human oral microbiota (McInnes et al., 2020). The literature reports on the occurrence of strong correlations between this phylum and the genes encoding resistance to aminoglycosides, beta-lactams, and antibiotics from the group of macrolides, lincosamides, and group B streptogramins (MLSB) (Zhou et al., 2018) or genes from the oxacillinase group (Ambler class D) (Qiu et al., 2019). To the best of the authors’ knowledge, to date there have been no reports in the available literature on the important role of this phylum in spreading the antibiotic resistance problem in wastewater. This study, however, confirms the role of Fusobacteria in the spread of ARGs, including the carbapenemase-encoding genes of importance to public health.
Conclusion
The employed metagenomic analysis determined the hospital wastewater inflowing to WWTP as a probable factor leading not only to an increase in the number of carbapenemase-encoding genes but also to an extension of the diversity of resistance gene types in wastewater. In wastewater with a higher percentage of hospital wastewater, as compared to wastewater with its lower percentage, blaKPC and blaNDM genes, associated with dangerous hospital outbreaks, were detected. Wastewater with a higher load of hospital wastewater was characterized by a greater number of statistically significant correlations between an abundance of specific beta-lactamase gene types and an abundance of integrase gene types compared to wastewater with a lower percentage of hospital wastewater. Microorganisms belonging to the families of Moraxellaceae (such genera as Acinetobacter sp. and Moraxella sp.) and Fusobacteriaceae (which are strongly linked to the described mobile genetic elements and carbapenem resistance genes) may be a significant factor in the spread of resistance genes. The obtained results provide new information on the possible involvement of bacteria of the Fusobacteriaceae family in the spread of antibiotic resistance in the environment. It is also worth highlighting the increasing importance of intI3 gene interactions between antibiotic resistance genes and bacteria in wastewater with a higher percentage of hospital wastewater. Considering the above, the results of this study show the widespread occurrence of antibiotic resistance genes (including very clinically important carbapenem resistance genes) in municipal wastewater with hospital wastewater inflow. Moreover, this paper describes how the studied wastewater may contribute to the spread of those pollutants in the aquatic environment irrespective of the treatment system.
Data Availability Statement
The metagenome data used in submitted paper #738158, are deposited in the NCBI Sequence Read Archive (SRA) under accessions NCBI SRA BioProject: PRJNA666519—https://www.ncbi.nlm.nih.gov/bioproject/PRJNA6665196.
Author Contributions
Conceptualization, SC, MH, EK, GP; Methodology, SC, MH, EK, GP; Soft-ware, JH, TD; Validation, JH; Formal Analysis, JH, SC; Data Curation, JH, SC, TD, ŁJ; Writing—Original Draft Preparation, JH; Writing—Review and Editing, SC, MH, EK, GP; Visualization, JH; Supervision, MH; Funding Acquisition, MH, GP.
Funding
This study was supported by grant No. 2017/M/NZ9/00071 from the Polish National Science Centre. JH has received a scholarship from the Interdisciplinary Doctoral Program in Bioeconomy (POWR.03.02.00-00-I034/16 00) funded by the European Social Fund.
Conflict of Interest
The authors declare that the research was conducted in the absence of any commercial or financial relationships that could be construed as a potential conflict of interest.
Publisher’s Note
All claims expressed in this article are solely those of the authors and do not necessarily represent those of their affiliated organizations, or those of the publisher, the editors and the reviewers. Any product that may be evaluated in this article, or claim that may be made by its manufacturer, is not guaranteed or endorsed by the publisher.
Supplementary Material
The Supplementary Material for this article can be found online at: https://www.frontiersin.org/articles/10.3389/fenvs.2021.738158/full#supplementary-material
References
Abraham, E. P., and Chain, E. (1940). An Enzyme from Bacteria Able to Destroy Penicillin. Nature 146, 837. doi:10.1038/146837a0
Adelowo, O. O., Caucci, S., Banjo, O. A., Nnanna, O. C., Awotipe, E. O., Peters, F. B., et al. (2018). Extended Spectrum Beta-Lactamase (ESBL)-Producing Bacteria Isolated from Hospital Wastewaters, Rivers and Aquaculture Sources in Nigeria. Environ. Sci. Pollut. Res. 25, 2744–2755. doi:10.1007/s11356-017-0686-7
Amador, P. P., Fernandes, R. M., Prudêncio, M. C., Barreto, M. P., and Duarte, I. M. (2015). Antibiotic Resistance in Wastewater: Occurrence and Fate ofEnterobacteriaceaeproducers of Class A and Class C β-lactamases. J. Environ. Sci. Health A 50, 26–39. doi:10.1080/10934529.2015.964602
Amos, G. C., Gozzard, E., Carter, C. E., Mead, A., Bowes, M. J., Hawkey, P. M., et al. (2015). Validated Predictive Modelling of the Environmental Resistome. ISME J. 9, 1467–1476. doi:10.1038/ismej.2014.237
An, X.-L., Chen, Q.-L., Zhu, D., Zhu, Y.-G., Gillings, M. R., and Su, J.-Q. (2018). Impact of Wastewater Treatment on the Prevalence of Integrons and the Genetic Diversity of Integron Gene Cassettes. Appl. Environ. Microbiol. 84, e02766–17. doi:10.1128/AEM.02766-17
Arakawa, Y., Murakami, M., Suzuki, K., Ito, H., Wacharotayankun, R., Ohsuka, S., et al. (1995). A Novel Integron-Like Element Carrying the Metallo-Beta-Lactamase Gene blaIMP. Antimicrob. Agents Chemother. 39, 1612–1615. doi:10.1128/AAC.39.7.1612
Araújo, S., Sousa, M., Tacão, M., Baraúna, R. A., Silva, A., Ramos, R., et al. (2021). Carbapenem-Resistant Bacteria over a Wastewater Treatment Process: Carbapenem-Resistant Enterobacteriaceae in Untreated Wastewater and Intrinsically-Resistant Bacteria in Final Effluent. Sci. Total Environ. 782, 146892. doi:10.1016/j.scitotenv.2021.146892
Banjo, O. A., Adekanmbi, A. O., and Oyelade, A. A. (2020). Occurrence of CTX-M, SHV and TEM β-Lactamase Genes in Extended Spectrum Beta-Lactamase (ESBL)- Producing Bacteria Recovered from Wastewater of a Privately-Owned Hospital in Nigeria and a Hand-Dug Well within its Vicinity. Gene Rep. 21, 100970. doi:10.1016/j.genrep.2020.100970
Barberán, A., Bates, S. T., Casamayor, E. O., and Fierer, N. (2012). Using Network Analysis to Explore Co-Occurrence Patterns in Soil Microbial Communities. ISME J. 6, 343–351. doi:10.1038/ismej.2011.119
Barraud, O., Casellas, M., Dagot, C., and Ploy, M.-C. (2013). An Antibiotic-Resistant Class 3 Integron in an Enterobacter cloacae Isolate from Hospital Effluent. Clin. Microbiol. Infect. 19, E306–E308. doi:10.1111/1469-0691.12186
Bastian, M., Heymann, S., and Jacomy, M. (2009). “Gephi: An Open Source Software for Exploring and Manipulating Networks,” in AAAI Conference on Weblogs and Social Media (ICWSM09), San Jose, California, May 17–20, 2009, 361–362. doi:10.1136/qshc.2004.010033
Bäumlisberger, M., Youssar, L., Schilhabel, M. B., and Jonas, D. (2015). Influence of a Non-Hospital Medical Care Facility on Antimicrobial Resistance in Wastewater. PLoS One 10, e0122635. doi:10.1371/journal.pone.0122635
Böhm, M.-E., Razavi, M., Flach, C.-F., and Larsson, D. G. J. (2020). A Novel, Integron-Regulated, Class C β-Lactamase. Antibiotics 9, 123. doi:10.3390/antibiotics9030123
Bolger, A. M., Lohse, M., and Usadel, B. (2014). Trimmomatic: A Flexible Trimmer for Illumina Sequence Data. Bioinformatics 30, 2114–2120. doi:10.1093/bioinformatics/btu170
Bonomo, R. A. (2017). β-Lactamases: A Focus on Current Challenges. Cold Spring Harb. Perspect. Med. 7, a025239. doi:10.1101/cshperspect.a025239
Buelow, E., Bayjanov, J. R., Majoor, E., Willems, R. J., Bonten, M. J., Schmitt, H., et al. (2018). Limited Influence of Hospital Wastewater on the Microbiome and Resistome of Wastewater in a Community Sewerage System. FEMS Microbiol. Ecol. 94, fiy087. doi:10.1093/femsec/fiy087
Bush, K. (2018). Past and Present Perspectives on β-Lactamases. Antimicrob. Agents Chemother. 62, e01076–18. doi:10.1128/AAC.01076-18
Buta, M., Hubeny, J., Zieliński, W., Harnisz, M., and Korzeniewska, E. (2021). Sewage Sludge in Agriculture - the Effects of Selected Chemical Pollutants and Emerging Genetic Resistance Determinants on the Quality of Soil and Crops - A Review. Ecotoxicology Environ. Saf. 214, 112070. doi:10.1016/j.ecoenv.2021.112070
Cahill, N., O'Connor, L., Mahon, B., Varley, Á., McGrath, E., Ryan, P., et al. (2019). Hospital Effluent: A Reservoir for Carbapenemase-Producing Enterobacterales? Sci. Total Environ. 672, 618–624. doi:10.1016/j.scitotenv.2019.03.428
Cai, L., Ju, F., and Zhang, T. (2014). Tracking Human Sewage Microbiome in a Municipal Wastewater Treatment Plant. Appl. Microbiol. Biotechnol. 98, 3317–3326. doi:10.1007/s00253-013-5402-z
Carattoli, A. (2001). Importance of Integrons in the Diffusion of Resistance. Vet. Res. 32, 243–259. doi:10.1051/vetres:2001122
Casaburi, G., Duar, R. M., Vance, D. P., Mitchell, R., Contreras, L., Frese, S. A., et al. (2019). Early-Life Gut Microbiome Modulation Reduces the Abundance of Antibiotic-Resistant Bacteria. Antimicrob. Resist. Infect. Control. 8, 131. doi:10.1186/s13756-019-0583-6
Chagas, T. P. G., Seki, L. M., Cury, J. C., Oliveira, J. A. L., Dávila, A. M. R., Silva, D. M., et al. (2011). Multiresistance, Beta-Lactamase-Encoding Genes and Bacterial Diversity in Hospital Wastewater in Rio de Janeiro, Brazil. J. Appl. Microbiol. 111, 572–581. doi:10.1111/j.1365-2672.2011.05072.x
Chaturvedi, P., Chaurasia, D., Pandey, A., and Gupta, P. (2020). Co-Occurrence of Multidrug Resistance, β-lactamase and Plasmid Mediated AmpC Genes in Bacteria Isolated from River Ganga, Northern India. Environ. Pollut. 267, 115502. doi:10.1016/j.envpol.2020.115502
Chaturvedi, P., Singh, A., Chowdhary, P., Pandey, A., and Gupta, P. (2021). Occurrence of Emerging Sulfonamide Resistance (Sul1 and Sul2) Associated with Mobile Integrons-Integrase (intI1 and intI2) in Riverine Systems. Sci. Total Environ. 751, 142217. doi:10.1016/j.scitotenv.2020.142217
Chouchani, C., Marrakchi, R., Henriques, I., and Correia, A. (2013). Occurrence of IMP-8, IMP-10, and IMP-13 Metallo-β-Lactamases Located on Class 1 Integrons and Other Extended-Spectrum β-lactamases in Bacterial Isolates from Tunisian Rivers. Scand. J. Infect. Dis. 45, 95–103. doi:10.3109/00365548.2012.717712
Conte, D., Palmeiro, J. K., da Silva Nogueira, K., de Lima, T. M. R., Cardoso, M. A., Pontarolo, R., et al. (2017). Characterization of CTX-M Enzymes, Quinolone Resistance Determinants, and Antimicrobial Residues from Hospital Sewage, Wastewater Treatment Plant, and River Water. Ecotoxicology Environ. Saf. 136, 62–69. doi:10.1016/j.ecoenv.2016.10.031
Dallenne, C., Da Costa, A., Decré, D., Favier, C., and Arlet, G. (2010). Development of a Set of Multiplex PCR Assays for the Detection of Genes Encoding Important β-lactamases in Enterobacteriaceae. J. Antimicrob. Chemother. 65, 490–495. doi:10.1093/jac/dkp498
David, S., Reuter, S., Reuter, S., Harris, S. R., Glasner, C., Feltwell, T., et al. (2019). Epidemic of Carbapenem-Resistant Klebsiella pneumoniae in Europe Is Driven by Nosocomial Spread. Nat. Microbiol. 4, 1919–1929. doi:10.1038/s41564-019-0492-8
Di Cesare, A., Eckert, E. M., D'Urso, S., Bertoni, R., Gillan, D. C., Wattiez, R., et al. (2016). Co-occurrence of Integrase 1, Antibiotic and Heavy Metal Resistance Genes in Municipal Wastewater Treatment Plants. Water Res. 94, 208–214. doi:10.1016/j.watres.2016.02.049
European Centre for Disease Prevention and Control (2020). Antimicrobial Consumption in the EU/EEA – Annual Epidemiological Report 2019 Stockholm: ECDC, 1–25.
Escudeiro, P., Pothier, J., Dionisio, F., and Nogueira, T. (2019). Antibiotic Resistance Gene Diversity and Virulence Gene Diversity Are Correlated in Human Gut and Environmental Microbiomes. mSphere 4, e00135-19. doi:10.1128/mSphere.00135-19
Fernandez, T. D., Mayorga, C., Salas, M., Barrionuevo, E., Posadas, T., Ariza, A., et al. (2017). Evolution of Diagnostic Approaches in Betalactam Hypersensitivity. Expert Rev. Clin. Pharmacol. 10, 671–683. doi:10.1080/17512433.2017.1313110
Gatica, J., Tripathi, V., Green, S., Manaia, C. M., Berendonk, T., Cacace, D., et al. (2016). High Throughput Analysis of Integron Gene Cassettes in Wastewater Environments. Environ. Sci. Technol. 50, 11825–11836. doi:10.1021/acs.est.6b03188
Ghafourian, S., Sadeghifard, N., Soheili, S., and Sekawi, Z. (2015). Extended Spectrum Beta-Lactamases: Definition, Classification and Epidemiology. Curr. Issues Mol. Biol. 17, 11–22. doi:10.21775/cimb.017.011
Goudarzi, H., Mirsamadi, E. S., Ghalavand, Z., Hakemi Vala, M., Mirjalali, H., and Hashemi, A. (2019). Rapid Detection and Molecular Survey of blaVIM, blaIMP and blaNDM Genes Among Clinical Isolates of Acinetobacter Baumannii Using New Multiplex Real-Time PCR and Melting Curve Analysis. BMC Microbiol. 19, 122. doi:10.1186/s12866-019-1510-y
Grundmann, H., Glasner, C., Albiger, B., Aanensen, D. M., Tomlinson, C. T., Andrasević, A. T., et al. (2017). Occurrence of Carbapenemase-Producing Klebsiella pneumoniae and Escherichia coli in the European Survey of Carbapenemase-Producing Enterobacteriaceae (EuSCAPE): A Prospective, Multinational Study. Lancet Infect. Dis. 17, 153–163. doi:10.1016/S1473-3099(16)30257-2
Guo, J., Li, J., Chen, H., Bond, P. L., and Yuan, Z. (2017). Metagenomic Analysis Reveals Wastewater Treatment Plants as Hotspots of Antibiotic Resistance Genes and Mobile Genetic Elements. Water Res. 123, 468–478. doi:10.1016/j.watres.2017.07.002
Haller, L., Chen, H., Ng, C., Le, T. H., Koh, T. H., Barkham, T., et al. (2018). Occurrence and Characteristics of Extended-Spectrum β-lactamase- and Carbapenemase- Producing Bacteria from Hospital Effluents in Singapore. Sci. Total Environ. 615, 1119–1125. doi:10.1016/j.scitotenv.2017.09.217
Hamidian, M., and Nigro, S. J. (2019). Emergence, Molecular Mechanisms and Global Spread of Carbapenem-Resistant Acinetobacter Baumannii. Microb. Genomics 5, e000306. doi:10.1099/mgen.0.000306
Hassoun-Kheir, N., Stabholz, Y., Kreft, J.-U., de la Cruz, R., Romalde, J. L., Nesme, J., et al. (2020). Comparison of Antibiotic-Resistant Bacteria and Antibiotic Resistance Genes Abundance in Hospital and Community Wastewater: A Systematic Review. Sci. Total Environ. 743, 140804. doi:10.1016/j.scitotenv.2020.140804
Hocquet, D., Muller, A., and Bertrand, X. (2016). What Happens in Hospitals Does Not Stay in Hospitals: Antibiotic-Resistant Bacteria in Hospital Wastewater Systems. J. Hosp. Infect. 93, 395–402. doi:10.1016/j.jhin.2016.01.010
Iovleva, A., and Doi, Y. (2017). Carbapenem-Resistant Enterobacteriaceae. Clin. Lab. Med. 37, 303–315. doi:10.1016/j.cll.2017.01.005
Isler, B., Doi, Y., Bonomo, R. A., and Paterson, D. L. (2018). New Treatment Options against Carbapenem-Resistant Acinetobacter Baumannii Infections. Antimicrob. Agents Chemother. 63, e01110–18. doi:10.1128/AAC.01110-18
Jendrzejewska, N. (2018). Antibiotic Resistance of Bacteria and Incidence of Carbapenamase-Coding Genes blaPERand blaGES in Isolates from Wastewater Treatment Plants. World Sci. News 93, 43–52.
Jiao, Y.-N., Chen, H., Gao, R.-X., Zhu, Y.-G., and Rensing, C. (2017). Organic Compounds Stimulate Horizontal Transfer of Antibiotic Resistance Genes in Mixed Wastewater Treatment Systems. Chemosphere 184, 53–61. doi:10.1016/j.chemosphere.2017.05.149
Jin, L., Wang, R., Wang, X., Wang, Q., Zhang, Y., Yin, Y., et al. (2018). Emergence of Mcr-1 and Carbapenemase Genes in Hospital Sewage Water in Beijing, China. J. Antimicrob. Chemother. 73, 84–87. doi:10.1093/jac/dkx355
Ju, F., Beck, K., Yin, X., Maccagnan, A., McArdell, C. S., Singer, H. P., et al. (2019). Wastewater Treatment Plant Resistomes Are Shaped by Bacterial Composition, Genetic Exchange, and Upregulated Expression in the Effluent Microbiomes. ISME J. 13, 346–360. doi:10.1038/s41396-018-0277-8
Khan, A. U., Maryam, L., and Zarrilli, R. (2017). Structure, Genetics and Worldwide Spread of New Delhi Metallo-β-Lactamase (NDM): A Threat to Public Health. BMC Microbiol. 17, 101. doi:10.1186/s12866-017-1012-8
Khan, F. A., Söderquist, B., and Jass, J. (2019). Prevalence and Diversity of Antibiotic Resistance Genes in Swedish Aquatic Environments Impacted by Household and Hospital Wastewater. Front. Microbiol. 10, 688. doi:10.3389/fmicb.2019.00688
Khurana, S., Mathur, P., Kapil, A., Valsan, C., and Behera, B. (2017). Molecular Epidemiology of Beta-Lactamase Producing Nosocomial Gram-Negative Pathogens from North and South Indian Hospitals. J. Med. Microbiol. 66, 999–1004. doi:10.1099/jmm.0.000513
Kim, H. C. (2016). Isolation of Carbapenemase Producing Enterobacteriaceae in the Greater Toronto Area ’S Sewage Treatment Plants and Surface Waters , and Their Comparison to Clinical CPE from Toronto by.
Korzeniewska, E., and Harnisz, M. (2013). Beta-Lactamase-Producing Enterobacteriaceae in Hospital Effluents. J. Environ. Manage. 123, 1–7. doi:10.1016/j.jenvman.2013.03.024
Kowalska, E., Paturej, E., and Zielińska, M. (2014). Use of Lecane Rotifers for Limiting Thiothrix Filamentous Bacteria in Bulking Activated Sludge in a Dairy Wastewater Treatment Plant. Arch. Biol. Sci. Belgra 66, 1371–1378. doi:10.2298/ABS1404371K
Kristiansson, E., Fick, J., Janzon, A., Grabic, R., Rutgersson, C., Weijdegård, B., et al. (2011). Pyrosequencing of Antibiotic-Contaminated River Sediments Reveals High Levels of Resistance and Gene Transfer Elements. PLoS One 6, e17038. doi:10.1371/journal.pone.0017038
Lamba, M., Graham, D. W., and Ahammad, S. Z. (2017). Hospital Wastewater Releases of Carbapenem-Resistance Pathogens and Genes in Urban India. Environ. Sci. Technol. 51, 13906–13912. doi:10.1021/acs.est.7b03380
Liu, Z., Klümper, U., Liu, Y., Yang, Y., Wei, Q., Lin, J.-G., et al. (2019). Metagenomic and Metatranscriptomic Analyses Reveal Activity and Hosts of Antibiotic Resistance Genes in Activated Sludge. Environ. Int. 129, 208–220. doi:10.1016/j.envint.2019.05.036
Logan, L. K., and Weinstein, R. A. (2017). The Epidemiology of Carbapenem-Resistant Enterobacteriaceae: The Impact and Evolution of a Global Menace. J. Infect. Dis. 215, S28–S36. doi:10.1093/infdis/jiw282
Makowska, N., Philips, A., Dabert, M., Nowis, K., Trzebny, A., Koczura, R., et al. (2020). Metagenomic Analysis of β-lactamase and Carbapenemase Genes in the Wastewater Resistome. Water Res. 170, 115277. doi:10.1016/j.watres.2019.115277
Masella, A. P., Bartram, A. K., Truszkowski, J. M., Brown, D. G., and Neufeld, J. D. (2012). PANDAseq: Paired-End Assembler for Illumina Sequences. BMC Bioinformatics 13, 31. doi:10.1186/1471-2105-13-31
Matar, G. M., Andremont, A., and Bazzi, W. (2020). Editorial: Combating Antimicrobial Resistance - A One Health Approach. Front. Cel. Infect. Microbiol. 9, 458. doi:10.3389/fcimb.2019.00458
Mathys, D. A., Mollenkopf, D. F., Feicht, S. M., Adams, R. J., Albers, A. L., Stuever, D. M., et al. (2019). Carbapenemase-Producing Enterobacteriaceae and Aeromonas Spp. Present in Wastewater Treatment Plant Effluent and Nearby Surface Waters in the US. PLoS One 14, e0218650. doi:10.1371/journal.pone.0218650
McArthur, A. G., Waglechner, N., Nizam, F., Yan, A., Azad, M. A., Baylay, A. J., et al. (2013). The Comprehensive Antibiotic Resistance Database. Antimicrob. Agents Chemother. 57, 3348–3357. doi:10.1128/AAC.00419-13
McInnes, R. S., McCallum, G. E., Lamberte, L. E., and van Schaik, W. (2020). Horizontal Transfer of Antibiotic Resistance Genes in the Human Gut Microbiome. Curr. Opin. Microbiol. 53, 35–43. doi:10.1016/j.mib.2020.02.002
Müller, V., Karami, N., Nyberg, L. K., Pichler, C., Torche Pedreschi, P. C., Quaderi, S., et al. (2016). Rapid Tracing of Resistance Plasmids in a Nosocomial Outbreak Using Optical DNA Mapping. ACS Infect. Dis. 2, 322–328. doi:10.1021/acsinfecdis.6b00017
Muzslay, M., Moore, G., Alhussaini, N., and Wilson, A. P. R. (2017). ESBL-Producing Gram-Negative Organisms in the Healthcare Environment as a Source of Genetic Material for Resistance in Human Infections. J. Hosp. Infect. 95, 59–64. doi:10.1016/j.jhin.2016.09.009
Naas, T., Dortet, L., and Iorga, B. I. (2016). Structural and Functional Aspects of Class A Carbapenemases. Curr. Drug Targets 17, 1006–1028. doi:10.2174/1389450117666160310144501
Nasri, E., Subirats, J., Sànchez-Melsió, A., Mansour, H. B., Borrego, C. M., and Balcázar, J. L. (2017). Abundance of Carbapenemase Genes (blaKPC, blaNDM and blaOXA-48) in Wastewater Effluents from Tunisian Hospitals. Environ. Pollut. 229, 371–374. doi:10.1016/j.envpol.2017.05.095
Nikonorow, E., Baraniak, A., and Gniadkowski, M. (2013). Oporność Bakterii Z Rodziny Enterobacteriaceae Na Antybiotyki β-laktamowe Wyniķajaca Z Wytwarzanla β-laktamaz. Postep. Mikrobiol. 52, 261–271.
Nwafia, I. N., Ohanu, M. E., Ebede, S. O., and Ozumba, U. C. (2019). Molecular Detection and Antibiotic Resistance Pattern of Extended-Spectrum Beta-Lactamase Producing Escherichia C in a Tertiary Hospital in Enugu, Nigeria. Ann. Clin. Microbiol. Antimicrob. 18, 41. doi:10.1186/s12941-019-0342-9
Osińska, A., Korzeniewska, E., Harnisz, M., Felis, E., Bajkacz, S., Jachimowicz, P., et al. (2020). Small-Scale Wastewater Treatment Plants as a Source of the Dissemination of Antibiotic Resistance Genes in the Aquatic Environment. J. Hazard. Mater. 381, 121221. doi:10.1016/j.jhazmat.2019.121221
Papp-Wallace, K. M., Becka, S. A., Taracila, M. A., Winkler, M. L., Gatta, J. A., Rholl, D. A., et al. (2016). Exposing a β-Lactamase "Twist": the Mechanistic Basis for the High Level of Ceftazidime Resistance in the C69F Variant of the Burkholderia Pseudomallei PenI β-Lactamase. Antimicrob. Agents Chemother. 60, 777–788. doi:10.1128/AAC.02073-15
Perez, F., El Chakhtoura, N. G., Papp-Wallace, K. M., Wilson, B. M., and Bonomo, R. A. (2016). Treatment Options for Infections Caused by Carbapenem-resistantEnterobacteriaceae: Can We Apply "Precision Medicine" to Antimicrobial Chemotherapy? Expert Opin. Pharmacother. 17, 761–781. doi:10.1517/14656566.2016.1145658
Piotrowska, M., Przygodzińska, D., Matyjewicz, K., and Popowska, M. (2017). Occurrence and Variety of β-Lactamase Genes Among Aeromonas Spp. Isolated from Urban Wastewater Treatment Plant. Front. Microbiol. 8, 863. doi:10.3389/fmicb.2017.00863
Qiu, W., Sun, J., Fang, M., Luo, S., Tian, Y., Dong, P., et al. (2019). Occurrence of Antibiotics in the Main Rivers of Shenzhen, China: Association with Antibiotic Resistance Genes and Microbial Community. Sci. Total Environ. 653, 334–341. doi:10.1016/j.scitotenv.2018.10.398
Röderová, M., Sedláková, M. H., Pudová, V., Hricová, K., Silová, R., Imwensi, P. E., et al. (2016). Occurrence of Bacteria Producing Broad-Spectrum Beta-Lactamases and Qnr Genes in Hospital and Urban Wastewater Samples. New Microbiol. 39, 124–133.
Rowe, W. P. M., Baker-Austin, C., Verner-Jeffreys, D. W., Ryan, J. J., Micallef, C., Maskell, D. J., et al. (2017). Overexpression of Antibiotic Resistance Genes in Hospital Effluents Over Time. J. Antimicrob. Chemother. 72, 1617–1623. doi:10.1093/jac/dkx017
Shibata, N., Doi, Y., Yamane, K., Yagi, T., Kurokawa, H., Shibayama, K., et al. (2003). PCR Typing of Genetic Determinants for Metallo-β-Lactamases and Integrases Carried by Gram-Negative Bacteria Isolated in Japan, with Focus on the Class 3 Integron. J. Clin. Microbiol. 41, 5407–5413. doi:10.1128/JCM.41.12.5407-5413.2003
Simo Tchuinte, P. L., Stalder, T., Venditti, S., Ngandjio, A., Dagot, C., Ploy, M.-C., et al. (2016). Characterisation of Class 3 Integrons with Oxacillinase Gene Cassettes in Hospital Sewage and Sludge Samples from France and Luxembourg. Int. J. Antimicrob. Agents 48, 431–434. doi:10.1016/j.ijantimicag.2016.06.018
Singh, K. M., Jakhesara, S. J., Koringa, P. G., Rank, D. N., and Joshi, C. G. (2012). Metagenomic Analysis of Virulence-Associated and Antibiotic Resistance Genes of Microbes in Rumen of Indian Buffalo (Bubalus Bubalis). Gene 507, 146–151. doi:10.1016/j.gene.2012.07.037
Sonda, T., Kumburu, H., van Zwetselaar, M., Alifrangis, M., Lund, O., Kibiki, G., et al. (2016). Meta-Analysis of Proportion Estimates of Extended-Spectrum-Beta-Lactamase-Producing Enterobacteriaceae in East Africa Hospitals. Antimicrob. Resist. Infect. Control. 5, 18. doi:10.1186/s13756-016-0117-4
Stalder, T., Barraud, O., Casellas, M., Dagot, C., and Ploy, M.-C. (2012). Integron Involvement in Environmental Spread of Antibiotic Resistance. Front. Microbio. 3, 119. doi:10.3389/fmicb.2012.00119
Su, J.-Q., An, X.-L., Li, B., Chen, Q.-L., Gillings, M. R., Chen, H., et al. (2017). Metagenomics of Urban Sewage Identifies an Extensively Shared Antibiotic Resistome in China. Microbiome 5, 84. doi:10.1186/s40168-017-0298-y
Teixeira, P., Tacão, M., Pureza, L., Gonçalves, J., Silva, A., Cruz-Schneider, M. P., et al. (2020). Occurrence of Carbapenemase-Producing Enterobacteriaceae in a Portuguese River: blaNDM, blaKPC and blaGES Among the Detected Genes. Environ. Pollut. 260, 113913. doi:10.1016/j.envpol.2020.113913
Tian, Z., Zhang, Y., Yu, B., and Yang, M. (2016). Changes of Resistome, Mobilome and Potential Hosts of Antibiotic Resistance Genes during the Transformation of Anaerobic Digestion from Mesophilic to Thermophilic. Water Res. 98, 261–269. doi:10.1016/j.watres.2016.04.031
Timraz, K., Xiong, Y., Al Qarni, H., and Hong, P.-Y. (2017). Removal of Bacterial Cells, Antibiotic Resistance Genes and Integrase Genes by On-Site Hospital Wastewater Treatment Plants: Surveillance of Treated Hospital Effluent Quality. Environ. Sci. Water Res. Technol. 3, 293–303. doi:10.1039/C6EW00322B
Wang, J., Chu, L., Wojnárovits, L., and Takács, E. (2020). Occurrence and Fate of Antibiotics, Antibiotic Resistant Genes (ARGs) and Antibiotic Resistant Bacteria (ARB) in Municipal Wastewater Treatment Plant: An Overview. Sci. Total Environ. 744, 140997. doi:10.1016/j.scitotenv.2020.140997
Wang, J., Mao, D., Mu, Q., and Luo, Y. (2015). Fate and Proliferation of Typical Antibiotic Resistance Genes in Five Full-Scale Pharmaceutical Wastewater Treatment Plants. Sci. Total Environ. 526, 366–373. doi:10.1016/j.scitotenv.2015.05.046
Wang, M., Shen, W., Yan, L., Wang, X.-H., and Xu, H. (2017). Stepwise Impact of Urban Wastewater Treatment on the Bacterial Community Structure, Antibiotic Contents, and Prevalence of Antimicrobial Resistance. Environ. Pollut. 231, 1578–1585. doi:10.1016/j.envpol.2017.09.055
Wang, Q., Wang, P., and Yang, Q. (2018). Occurrence and Diversity of Antibiotic Resistance in Untreated Hospital Wastewater. Sci. Total Environ. 621, 990–999. doi:10.1016/j.scitotenv.2017.10.128
Wang, Z. H., Yang, J. Q., Zhang, D. J., Zhou, J., Zhang, C. D., Su, X. R., et al. (2014). Composition and Structure of Microbial Communities Associated with Different Domestic Sewage Outfalls. Genet. Mol. Res. 13, 7542–7552. doi:10.4238/2014.September.12.21
WHO (2018). WHO Report on Surveillance of Antibiotic Consumption. Available at: https://apps.who.int/iris/bitstream/handle/10665/277359/9789241514880-eng.pdf (Accessed February 12, 2021).
Yang, F., Mao, D., Zhou, H., and Luo, Y. (2016). Prevalence and Fate of Carbapenemase Genes in a Wastewater Treatment Plant in Northern China. PLoS One 11, e0156383. doi:10.1371/journal.pone.0156383
Yang, Y., Li, B., Ju, F., and Zhang, T. (2013). Exploring Variation of Antibiotic Resistance Genes in Activated Sludge over a Four-Year Period through a Metagenomic Approach. Environ. Sci. Technol. 47, 10197–10205. doi:10.1021/es4017365
Ye, J., Song, Z., Wang, L., and Zhu, J. (2016). Metagenomic Analysis of Microbiota Structure Evolution in Phytoremediation of a Swine Lagoon Wastewater. Bioresour. Technol. 219, 439–444. doi:10.1016/j.biortech.2016.08.013
Yuan, Q.-B., Zhai, Y.-F., Mao, B.-Y., and Hu, N. (2018). Antibiotic Resistance Genes and intI1 Prevalence in a Swine Wastewater Treatment Plant and Correlation with Metal Resistance, Bacterial Community and Wastewater Parameters. Ecotoxicology Environ. Saf. 161, 251–259. doi:10.1016/j.ecoenv.2018.05.049
Zagui, G. S., de Andrade, L. N., Moreira, N. C., Silva, T. V., Machado, G. P., da Costa Darini, A. L., et al. (2020). Gram-Negative Bacteria Carrying β-lactamase Encoding Genes in Hospital and Urban Wastewater in Brazil. Environ. Monit. Assess. 192, 376. doi:10.1007/s10661-020-08319-w
Zhang, T., Zhang, X.-X., and Ye, L. (2011). Plasmid Metagenome Reveals High Levels of Antibiotic Resistance Genes and Mobile Genetic Elements in Activated Sludge. PLoS One 6, e26041. doi:10.1371/journal.pone.0026041
Zhou, Z.-C., Feng, W.-Q., Han, Y., Zheng, J., Chen, T., Wei, Y.-Y., et al. (2018). Prevalence and Transmission of Antibiotic Resistance and Microbiota between Humans and Water Environments. Environ. Int. 121, 1155–1161. doi:10.1016/j.envint.2018.10.032
Zieliński, W., Buta, M., Hubeny, J., Korzeniewska, E., Harnisz, M., Nowrotek, M., et al. (2019). Prevalence of Beta Lactamases Genes in Sewage and Sludge Treated in Mechanical-Biological Wastewater Treatment Plants. J. Ecol. Eng. 20, 80–86. doi:10.12911/22998993/112506
Keywords: wastewater, hospital wastewater, antibiotic resistance genes, environmental resistome, beta-lactamases, carbapenemases
Citation: Hubeny J, Ciesielski S, Harnisz M, Korzeniewska E, Dulski T, Jałowiecki Ł and Płaza G (2021) Impact of Hospital Wastewater on the Occurrence and Diversity of Beta-Lactamase Genes During Wastewater Treatment with an Emphasis on Carbapenemase Genes: A Metagenomic Approach. Front. Environ. Sci. 9:738158. doi: 10.3389/fenvs.2021.738158
Received: 08 July 2021; Accepted: 06 October 2021;
Published: 22 October 2021.
Edited by:
Timothy Sibanda, University of Namibia, NamibiaReviewed by:
Shuying Li, Zhejiang University, ChinaDambudzo Mbendana, Agricultural Research Council of South Africa (ARC-SA), South Africa
Copyright © 2021 Hubeny, Ciesielski, Harnisz, Korzeniewska, Dulski, Jałowiecki and Płaza. This is an open-access article distributed under the terms of the Creative Commons Attribution License (CC BY). The use, distribution or reproduction in other forums is permitted, provided the original author(s) and the copyright owner(s) are credited and that the original publication in this journal is cited, in accordance with accepted academic practice. No use, distribution or reproduction is permitted which does not comply with these terms.
*Correspondence: Monika Harnisz, bW9uaWthLmhhcm5pc3pAdXdtLmVkdS5wbA==, bW9uaWthaEB1d20uZWR1LnBs