- 1Universidade Católica Portuguesa (Universidade Católica Portuguesa, CBQF - Centro de Biotecnologia e Química Fina – Laboratório Associado, Escola Superior de Biotecnologia, Porto, Portugal
- 2CEB—Centre of Biological Engineering, Universidade do Minho, Braga, Portugal
Aerobic granular sludge (AGS) processes are among the most robust wastewater treatments. One of their greatest advantages is related to the granules multi-layered structure, which creates a protective barrier against organic shock loads and variable wastewater composition, particularly attractive for the treatment of industrial wastewater. However, when treating a wastewater with variable and complex composition, the difficulty in identifying factors that most affect a specific biological process increases. In this study, the effect of organic loading rate (OLR), namely carbon content, on nitrification in an AGS process treating fish canning wastewater was investigated. Besides process performance, also biomass structural changes, and microbial community composition were analysed. Reactor operation lasted for 107 days and was divided in three phases during which different OLR and C/N ratios were applied. A higher OLR was applied during the first two phases (ca. 1.1 and 1.5 kg COD m−3 day−1, respectively) compared to the third phase (between 0.12 and 0.78 kg COD m−3 day−1) and the C/N ratios also varied (ca. 4.4, 7.8, and 2.9, respectively). Throughout the operation, COD concentration in the outlet was lower than 100 mg O2 L−1. Nitrification was inhibited during the second phase and recovered afterwards. Principal component analysis (PCA) of quantitative image analysis (QIA) and performance data allowed to distinguish process changes over the three operational phases. During the first two phases, the decrease in the biomass robustness occurred, but recovered during the last phase, indicating that the high content of organic matter had possibly an effect on the aerobic granules structural characteristics. The composition of the AGS microbiome did not change substantially after the end of the higher OLR periods. The main microbial diversity shifts were mostly associated to adaptation to higher or lower carbon availability. Bacteria and inferred enzymes associated to nitrogen and phosphorous removal were identified. Chryseobacterium, a bacterium with high metabolic versatility, was able to adapt to the organic shock load, becoming dominant over operation. Despite the variable composition of the fish canning wastewater, carbon was identified as the main driver for nitrification inhibition, while promoting changes in the physical characteristics and on the microbial community of granules.
Introduction
Aerobic granular sludge (AGS) processes are improved wastewater treatment systems, with lower footprint and energy requirements, which are becoming the best alternatives to conventional wastewater treatment (Guo et al., 2020). After being explored and widely applied in urban wastewater treatment plants, the next challenge is to spread the application of AGS to the treatment of different types of industrial wastewater. Wastewater produced by industries can be very complex, variable, presenting higher carbon and nitrogen concentrations, as well as salt and toxic compounds (Amorim et al., 2017), which often affect the biological treatment. However, this negative effect is expected to occur to a lower extent in AGS processes, since the granules structure creates a protective environment for the different microbial groups present within the biomass (Cai et al., 2021). The inhibition effects stimulated by toxic compounds, mostly present in chemical industry wastewater have been extensively studied (Moreira et al., 2015; Ramos et al., 2017; Cai et al., 2021). On the other hand, food industry wastewater, which might not necessarily contain toxic compounds, is often characterized by a complex composition, a high content of carbon and nutrients, and salts (Cristóvão et al., 2015; Carrera et al., 2019). Salt levels higher than 20 g L−1 can negatively affect the AGS process performance (Bassin et al., 2011; Pronk et al., 2014). Aspects associated to the effect of wastewater strength, such as organic loading rate (OLR), as well as the effect of different C/N ratios on the AGS systems performance have been addressed (Luo et al., 2014; Hamza et al., 2019; Pishgar et al., 2019; Wang et al., 2019). However, most of these studies used synthetic wastewater.
Nitrogen and phosphorous removal have been considered the most sensitive processes to wastewater composition, due to the higher susceptibility of associated bacteria (De Kreuk et al., 2010; Luo et al., 2014; Hamza et al., 2019). Nitrification is a two-step autotrophic process, performed by different groups of slow-growing bacteria: ammonia oxidizing bacteria (AOB) and nitrite oxidizing bacteria (NOB). During denitrification, nitrate can be reduced to nitrogen-gas, in the presence of a carbon source. Simultaneous nitrification and denitrification (SND) is expected to occur within the granule (De Kreuk et al., 2005). However, to achieve this, both processes need to meet favourable conditions, mostly related to the absence/presence of carbon source, which should be diffusible, and to the amount of dissolved oxygen concentration in the bulk liquid (Layer et al., 2020). Nevertheless, nitrification must be firstly assured for achieving nitrogen removal. Both AOB and NOB are sensitive to different operational factors, such as temperature, dissolved oxygen, pH, solids retention time (SRT), but also to free ammonia and nitrite accumulation (Tang and Chen, 2015). However, the composition of the wastewater, namely in terms of carbon and nitrogen is also relevant for AOB and NOB activity (Carrera et al., 2004; de Sousa Rollemberg et al., 2018). Therefore, for optimizing the treatment of industrial wastewater, it is highly relevant to understand the link between wastewater composition and nitrification. AGS systems performance depends on the relationship established between bacteria responsible for nutrient removal and a wide variety of other bacterial groups with metabolic functions associated to organic carbon degradation, namely regarding extracellular polymeric substances (EPS) production, hydrolysis, carbon uptake and storage, and xenobiotics degradation (Szabó et al., 2017; Xia et al., 2018). Wastewater composition can affect aerobic granules stability, by disturbing the dynamics of this complex microbial community, and consequently its structure and morphology. Therefore, it is also crucial to understand how changes in microbial community composition and in physical properties of the granules can enlighten on AGS process stability.
The main objective of this study was to identify the main industrial wastewater constituents that drive the nitrification process, when treating industrial wastewater, while relating process stability with microbial composition, predicted metabolic function and structural characteristics of the granules. Fish canning wastewater with different OLR and C/N ratio fed to an AGS process was the basis to improve our knowledge on the application of such processes to the treatment of industrial wastewater.
Materials and Methods
Aerobic Granular Sludge Setup and Operation
A lab-scale sequencing batch reactor (SBR) (2.5 L working volume) was operated in four successive treatments of 6 h-cycles per day, each comprising 60 min of feeding, 292 min of aeration, 3 min of settling and 5 min of effluent withdrawal, according to Paulo et al. (2021). The AGS from a reactor located in Frielas wastewater treatment plant (WWTP), Lisbon (Portugal), was mixed with AGS from a previous study (Paulo et al., 2021). The reactor was fed with the wastewater provided by a fish canning plant (A POVEIRA, S.A., Póvoa de Varzim, Portugal), collected after solids and fat removal. The facility separates the brine water from the remaining water streams. Therefore, the effluent produced at the plant and used in this study did not present a very high salinity. The AGS-SBR operation was divided into three phases, over 107 days (Table 1), each corresponding to a different batch of wastewater collected at the plant, in order to investigate on the effect of wastewater composition changes on the AGS process. The pH of the AGS process was monitored at the sampling days, but not controlled.
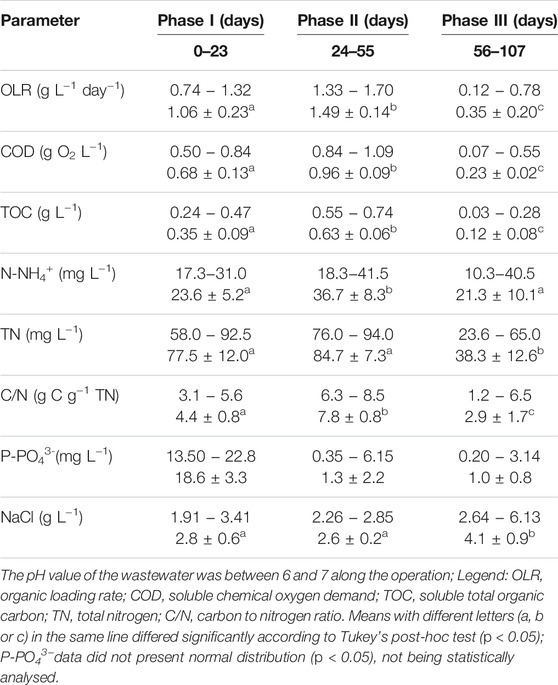
TABLE 1. Fish canning wastewater characterization throughout the reactor operation; minimum and maximum values and average ±standard deviation values are presented.
Analytical Methods
COD/BOD5 ratio of samples of the pre-treated wastewater was evaluated in accordance with standard methods (APHA, 1998). Carbon, nitrogen, and phosphorous removal by the AGS process were evaluated by analysing the composition of the influent (wastewater), of the reactor bulk after anaerobic feeding and of the effluent discharged (outlet). For that, suspended solids were removed from the collected samples with syringe nylon membrane filters (0.45 µm pore-size). The total organic carbon (TOC) was measured for selected wastewater samples using a Total Organic Carbon Analyzer (Shimadzu, Japan). The COD was analyzed in accordance with standard methods (APHA, 1998). Photometric test kits (Spectroquant®, Merck Millipore, United States) were used for determining the concentration of total nitrogen, ammonia, nitrate, nitrite, phosphorous, and chloride, according to the manufacturer’s instructions. NaCl content was indirectly quantified by measuring chloride content (Supplementary Figure S1). Total suspended solids (TSS) and volatile suspended solids (VSS) concentrations in the outlet were determined in accordance with standard methods (APHA, 1998). The pH of wastewater was checked periodically, while the pH in the outlet was measured every sampling day. The possibility of free ammonia accumulation at the end of the process was checked by using the equation proposed by Anthonisen et al. (1976), using the pH value and the NH4+-N concentration at the outlet.
Extracellular Polymeric Substances Extraction and Biochemical Characterization
The AGS samples were collected from the bioreactor at specific time points of reactor operation (day 0, day 55 and day 107). After sieving the AGS biomass, the EPS were extracted from the granules by using a sodium carbonate (Na2CO3) aqueous solution, heat and constant mixing, following the procedure described by Felz et al. (2016). The EPS biochemical characterization was performed using colorimetric methods for the quantification of proteins (Lowry et al., 1951), carbohydrates (Dubois et al., 1956) and humic acids like substances (Frølund et al., 1995).
Quantitative Image Analysis and Principal Component Analysis
Duplicate AGS biomass samples were collected along reactor operation and preserved according to Paulo et al. (2021). Structural characteristics of granules were followed by quantitative image analysis (QIA), using a sample volume of 2 ml and with a total magnification of ×15 QIA evaluated size and morphological descriptors including the equivalent diameter (Deq), number (%Nb), convexity (Conv), robustness (Rob) and roundness (round) of the granules, according to Ramos et al. (2017). The Conv represents the roughness of the granules border, the Rob combines the granule fulfilling ability and the border’s roughness and the Round represents the space fulfilling ability. Two size classes were considered for the granules based on the Deq: intermediate granules (IG) (Deq between 0.15 and 1.5 mm) and large granules (LG) (Deq above 1.5 mm).
The PCA was performed for the main QIA structural parameters of the aerobic granules (Supplementary Table S1) with the AGS-SBR physicochemical parameters, using Matlab 9.2 (The Mathworks, Natick MA, United States). The main objective of this analysis was to establish the relationships between the physicochemical parameters and granules structural properties during the treatment of industrial wastewater. Data used for the PCA analysis are presented as Supplementary Material (Supplementary Table S2 and Supplementary Table S3).
Aerobic Granular Sludge Microbiome Analysis
Genomic DNA Extraction
The AGS biomass samples were collected from the reactor during the aeration period, at the end and/or during each operational phase. Granules were aseptically crushed using a pottering tube and a pestle. UltraClean Microbial DNA Isolation Kit (Qiagen, Germany) was used for extracting the genomic DNA of crushed biomass, according to manufacturer’s instructions. The DNA concentration was measured by fluorimetry using Qubit (Thermo Fisher Scientific, United States) and the extracted DNA was stored at −20°C for further utilization.
Next-Generation Sequencing and Data Analysis
The NGS was performed using the previously extracted DNA. DNA amplification, libraries preparation, sequencing to bioinformatics data analysis were performed at GATC-Eurofins (Konstanz, Germany). Paired-end sequencing based on 16S rRNA phylogenetic gene was conducted using two primers (357F-TACGGGAGGCAGCAG, (Turner et al., 1999); 800R-CCAGGGTATCTAATCC, (Kisand et al., 2002) which cover V3-V4 hypervariable region (Illumina MiSeq platform). The microbiome analysis and profiling was performed according to procedures described in Paulo et al. (2021).The raw sequence data obtained for the AGS biomass samples was deposited in Sequence Read Archive (SRA) from NCBI database, associated to the BioProject with accession number PRJNA742749.
Microbial Metabolic Function Prediction
The Piphillin software (Iwai et al., 2016) was used for performing an in silico analysis of the AGS microbiome, by obtaining a putative metabolic functional profile of the biomass present during AGS reactor operation. Operational Taxonomic Unit (OTU) sequences and abundances, given by the 16S rRNA sequencing, were analysed and matched against the Kyoto Encyclopedia of Genes and Genomes (KEGG; http://www.genome.jp/kegg/) database of phylogenetically referenced prokaryotic genomes, for predicting the profile of functional categories at different levels. Piphillin software was also used for inferring the presence and relative abundance of enzymes involved in nitrification, denitrification, and phosphorous removal processes, commonly found in AGS biomass. For this, the results obtained from the 16S rRNA sequencing were compared against the BioCyc database (https://biocyc.org/). An identity cut-off of 97% of each OTU was used for all predictions.
Statistical Analysis
Differences between EPS characterization results (e.g., components concentrations, PN/PS ratio and total EPS) and also between different parameters characterizing the fish canning wastewater fed during the three operational phases (e.g., OLR, COD, P-PO43−, N-NH4+, TN, TOC, C/N ratio, and NaCl) were statistically analysed by one-way ANOVA. Significant differences between the means were determined by Tukey’s post-hoc test using the SPSS program (SPSS Inc., Chicago, IL Version 24.0). ANOVA and Tukey’s post-hoc test results are presented as Supplementary Material (Supplementary Table S4, Supplementary Table S5, Supplementary Table S6, and Supplementary Table S7).
Results
Reactor Performance
The wastewater presented a COD/BOD5 ratio between 2 and 2.5, confirming its biodegradability. Three operational phases were defined according to variations in the wastewater composition, mainly in organic matter, NaCl, phosphorous and nitrogen content (Table 1). A higher OLR was applied during the two first operational phases (I and II), while wastewater with lower organic matter content but more variable in composition was fed during phase III (Figure 1B). The carbon content (calculated as the ratio between TOC and COD) present in the organic matter (measured as COD) was similar in phases I and III, ca. 51–52%. During phase II, the carbon content was higher (ca. 66%). The ratio between the content in carbon and nitrogen was different between operational phases, being higher during phase II (ca. 8). The wastewater fed during phase III presented the lowest C/N ratio (ca. 3), despite periods of higher variation. OLR, COD, TOC concentrations in the wastewater, as well as respective C/N ratios, were found to be significantly different between the three operational phases. These differences did not affect COD removal, with COD content in the outlet always below the discharge limit of 125 mg O2 L−1 (maximum of 92 mg O2 L−1) (Figure 1A). However, during phases I and II, the COD concentration at the end of the anaerobic feeding period was often higher than 200 mg O2 L−1, being later consumed during the aerobic period of the treatment cycle.
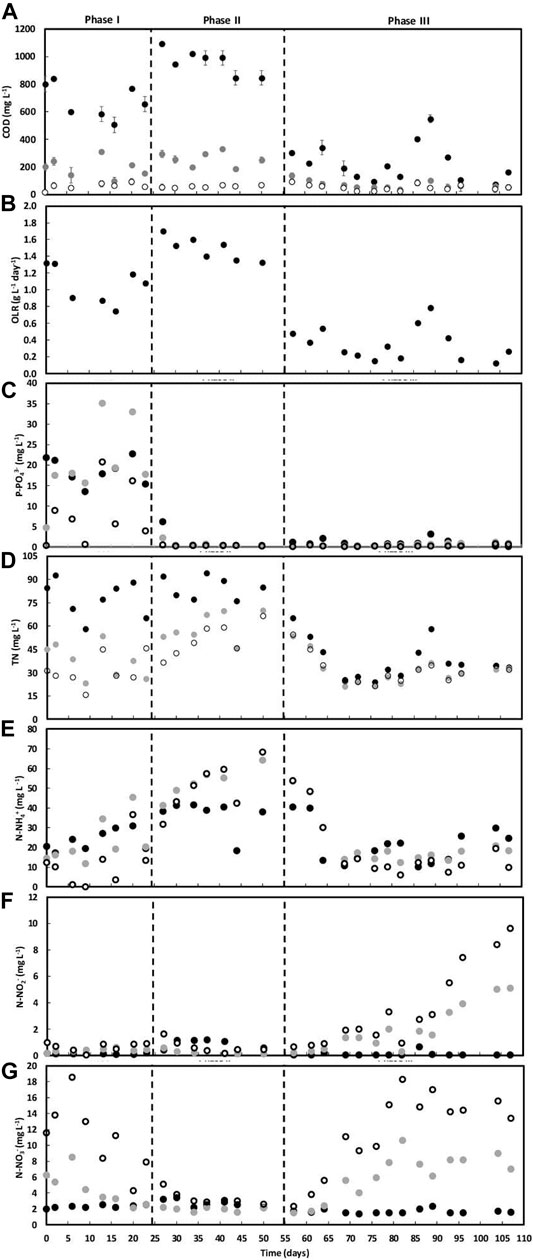
FIGURE 1. COD (A), OLR (B), phosphate (C), TN (D), ammonium (E), nitrite (F), and nitrate (G) concentrations along AGS-SBR operation. Concentration in the wastewater (●), in the reactor bulk liquid after anaerobic feeding (●) and outlet (○) are shown.
Phosphate concentration in the wastewater was also very different between operational phases, being higher during phase I (Table 1; Figure 1C), which was characterized by an unstable P-removal. Afterwards, the wastewater presented a very low phosphate concentration, reducing the need for phosphate removal.
Total nitrogen (TN) concentration in the wastewater was not significantly different between phases I and II, being reduced to half during phase III (Table 1). TN in the outlet was often lower than that observed at the end of anaerobic feeding period, mostly during phases I and II (Figure 1D). During phase III, no variation between the TN values measured before and after the aerobic period was observed.
The maximum and minimum ammonium concentration values fed to the AGS reactor occurred during phases II and III, respectively, in agreement with the OLR applied during these operational periods (Figure 1E). While ammonium concentration in wastewater was similar in phases I and III, it was significantly different from that in phase II. Ammonium removal was the most unstable process during reactor operation, being dynamic during phase I, completely inhibited during phase II and resumed during phase III. Nitrate was the main product of nitrification during phase I (Figure 1G) and nitrite was only quantified at higher extent in the beginning of phase III (Figure 1F), accumulating with nitrate at the end of the aerated period.
The NaCl concentration presented similar average values during phases I and II (2.8 ± 0.6 and 2.6 ± 0.2 g L−1, respectively) and, despite the decrease in OLR observed during phase III, NaCl concentration increased to 4.1 ± 0.9 g L−1 (Supplementary Figure S1). In fact, statistical analysis corroborated that NaCl content in phases I and II were significantly different from that of phase III.
After reactor start-up, the period between days 5 and 45 (phases I and II) presented a higher TSS concentration in the outlet (385 ± 105 mg TSS L−1), reducing to half (159 ± 63 mg TSS L−1) from day 50 until the end of the operation (Figure 2). The biomass bed height was initially mainly composed of granular biomass, but during phase II, it gradually increased due to the formation of suspended biomass (Figure 2). Nevertheless, the suspended biomass fraction in the AGS reactor decreased during phase III, being no longer observed from day 82 onwards.
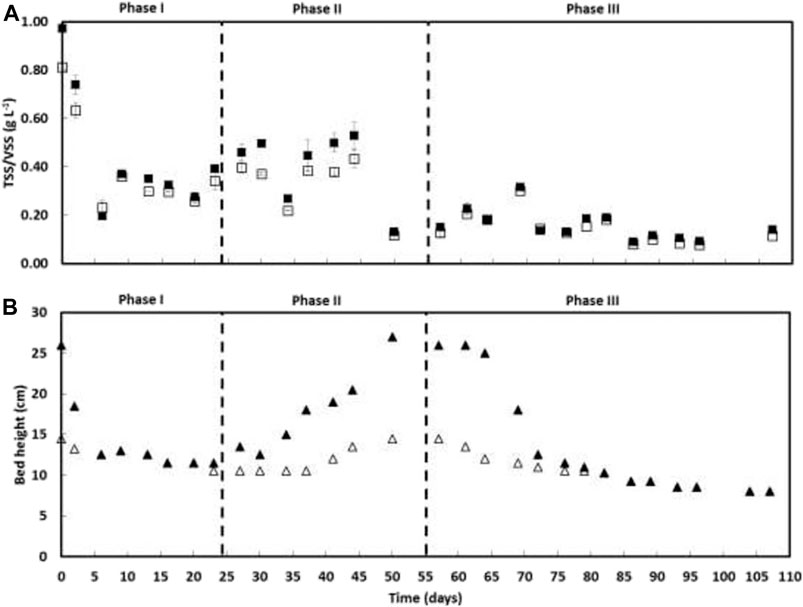
FIGURE 2. TSS (■) and VSS (□) concentrations in the outlet (A) and the biomass bed height (cm) (B) along AGS-SBR operation; The granular biomass (∆) and granular plus suspended biomass bed height (▲) are shown.
Extracellular Polymeric Substances Biochemical Characterization
Throughout the operational period, the EPS extracted from the AGS biomass presented different composition in terms of carbohydrates, proteins, and humic acids concentrations (Table 2). While protein content was not significantly different (p > 0.05), the carbohydrates and humic acids concentrations varied significantly on the different sampling days, as well as PN/PS ratio and total EPS (p < 0.05). In fact, the carbohydrate concentration in the EPS was significantly different in all samples while the humic acids content was very similar on days 55 and 107 (end of phases II and III, respectively), diverging significantly from that on day 0 (beginning of phase I). The PN/PS ratio was, therefore, significantly different between samples, while the total EPS was significantly different between day 0 and the end of phase II. Overall, results indicate that proteins were present in the EPS in a higher and more stable concentration compared to the other components. During AGS operation, humic acids concentration decreased while carbohydrates content presented an opposite tendency, contributing to different final concentration of EPS and PN/PS ratios.

TABLE 2. Biochemical characterization of the EPS extracted from the AGS biomass (average value ±standard deviation).
Principal Component Analysis
A PCA was performed with the ensemble of physicochemical parameters and granules structural properties, obtained during the treatment of fish canning wastewater by the AGS process (Figure 3). The analysis revealed the formation of three clusters, with cluster I being mostly composed by samples from phase I and the first day of phase III, cluster II gathering samples from phase II, and a more defined cluster III, with most of the samples from phase III (Figure 3A).
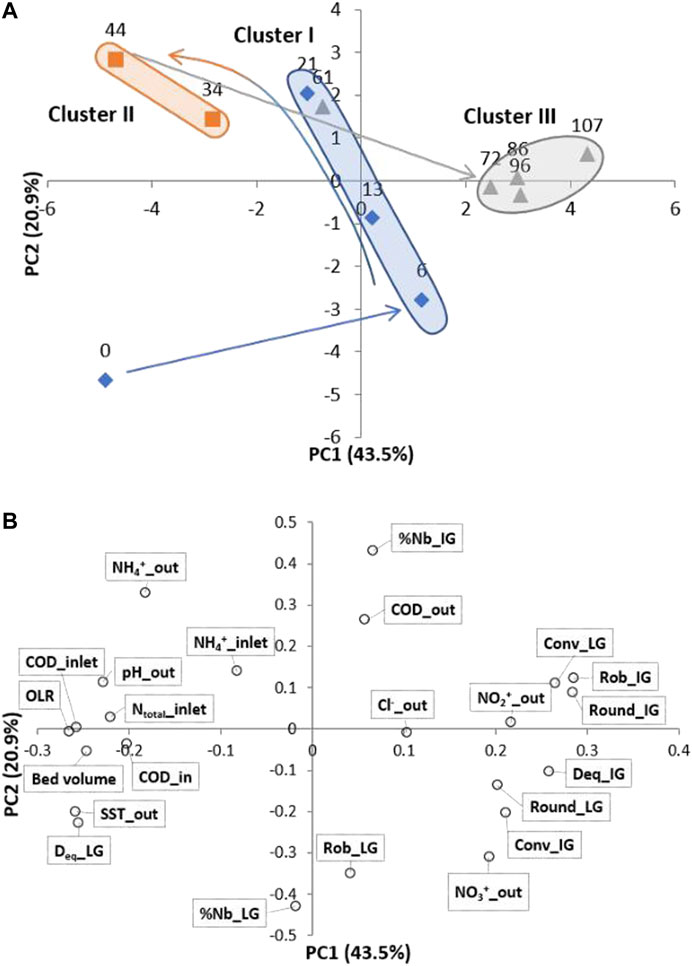
FIGURE 3. PCA with the main QIA data and physicochemical parameters from operational days corresponding to: phase I (day 0, day 6, day 13, day 21) (◊),phase II (day 34, day 44) (■) and phase III (day 61, day 72, d86, day 96, day 107) (▲) of the AGS process; (A) Operational periods and (B) QIA and physicochemical data. Note: PC1 and PC2 explain 43.5 and 20.9% of the dataset variance, respectively.
The evolution from day 0 until day 6 is associated to the adaptation of the AGS process to the fish canning wastewater, during reactor start up, positively influenced by morphological parameters from intermediate and large granules (i.e., Rob_IG, Round_IG, and Conv_LG) (Supplementary Figure S2). The elongated shape of cluster I is associated to a distribution mainly on the PC2 axis, initially influenced by the nitrate concentration at the outlet (Figure 3B) and the number percentage of large granules (%Nb_LG), and evolving towards a positive PC2 influenced by the number percentage of intermediate granules (%Nb_IG) and ammonium concentration at the outlet. During phase I, the AGS process started by producing nitrate as the main product of nitrification. However, the evolution of the cluster I towards the negative PC1 axis indicates a gradual increase of the influence of the wastewater composition (OLR and COD) (Figure 3B) on the system and a negative impact on the nitrification efficiency. The formation of a second cluster (cluster II), in the same quadrant of the last sample of phase I with a more negative PC1 value, indicates that the composition of the wastewater, namely OLR, and COD (Figure 3B), became determinant for the PCA trajectory throughout phase II. In the top left quadrant, it is possible to observe that ammonia concentration in the inlet and outlet are placed opposite to the bottom right quadrant, associated to nitrate concentration in the outlet. Moreover, the COD in the wastewater and the applied OLR are situated opposite to the granules morphological and size parameters (Rob_IG and LG, Round_IG and LG, Conv_IG and LG, and Deq_IG) (Figure 3B). On the other hand, cluster III shifted to a positive PC1 combined with a decrease of PC2, associated to nitrate and nitrite outlet concentrations, both products resulting from nitrification recovery (Figure 3B). During phase III, the importance of morphological and size parameters (namely Rob_IG and LG, Round_IG, Conv_IG and LG, Deq_IG and LG and %Nb_LG) for explaining dataset variance became more relevant (Supplementary Figure S2).
The PCA analysis evidenced a close relationship of the OLR in the wastewater, bed height and TSS at the effluent (Figure 3B). This clearly demonstrates that an increase of the organic loading led to an increase of the bed height and TSS at the effluent, causing therefore negative effects on the granule’s morphology. Hence, this PCA showed that PC1 was strongly affected by the granule’s morphological parameters and OLR.
Microbial Community Analysis
Bacterial Phyla, Classes and Families
Proteobacteria and Bacteroidetes represented more than 91% of the total bacterial relative abundance in AGS biomass throughout the operation (Figure 4A). Proteobacteria were dominant in the biomass microbial community at the beginning of the operation (day 0: Proteobacteria—60%; Bacteroidetes—31%), but lost this position to Bacteroidetes during phase II (day 44: Proteobacteria—36%; Bacteroidetes—60%). Actinobacteria (≤3%) and Nitrospirae (≤1.5%) were detected over the entire operation period, while Gemmatimonadetes increased up to 3.2% by the end of the operation.
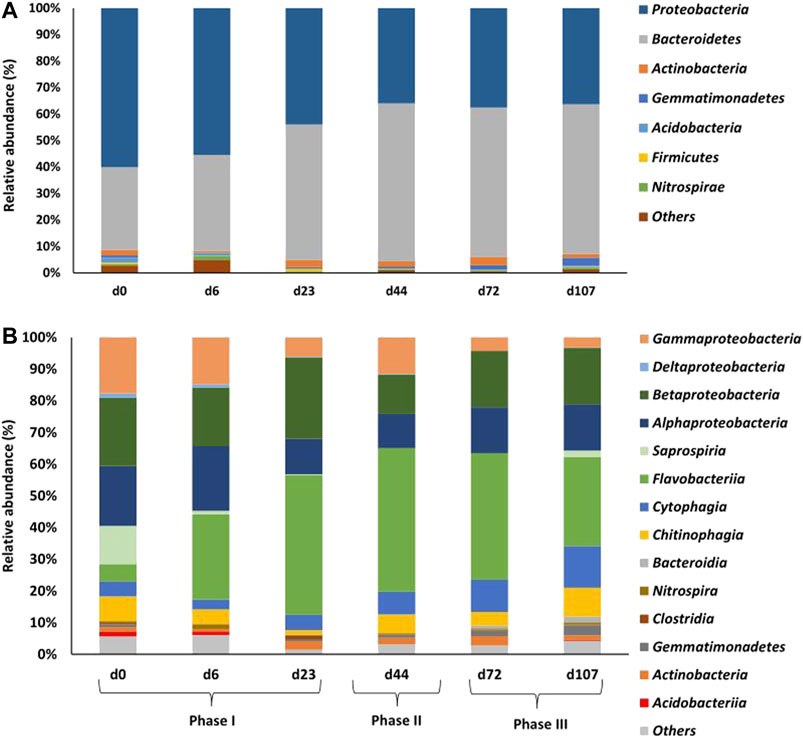
FIGURE 4. Relative abundance of bacterial phyla (A) and classes (B) in AGS biomass throughout the different operational days and corresponding phases.
The diversity of bacterial classes was higher at the beginning of reactor operation, decreasing over time (Figure 4B). Within Proteobacteria, Gammaproteobacteria suffered a decrease in relative abundance and Alphaproteobacteria reduced slightly along the process. Despite the greater variation in relative abundance observed at the end of phases I and II, Betaproteobacteria presented similar relative abundance at the beginning and end of operation (day 0–21.6%; day 6–18.4%; day 23–25.6%; day 44–12.4%; day 72–17.8%; day 107–17.8%). Among Bacteroidetes, Flavobacteriia became the most abundant class at the end of phase I, keeping the dominance until the end of operation. Chitinophagia kept their relative abundance while Cytophagia increased during phases II and III. Saprospiria relative abundance rapidly decreased during the first week of operation. Except Gemmatimonadetes and Actinobacteria, which reached up to 3% in relative abundance, the remaining bacterial classes (Nitrospira, Clostridia, Acidobacteriia) did not reach more than 1.5% in the AGS microbial community.
The Haliscomenobacteraceae family was dominant in the inoculum (day 0–11%; day 107–2%), losing its relevance to the Flavobacteriaceae family (day 0–5%; day 107–28%), which became the most abundant family until the end of the study (Supplementary Figure S3). Despite this shift, many bacterial families present in the inoculum persisted in the process, some keeping a somewhat similar relative abundance (Caulobacteraceae and Rhodobacteraceae—between 2 and 6.5%), others increasing their presence (Chitinophagaceae, to 9%; Cytophagaceae, to 7%; and Gemmatimonadaceae, to 3%), while many decreased their relative abundance (Comamonadaceae, to 3.5%; Sphingomonadaceae, to 3.2%, Candidatus Competibacteraceae, to 0.6% and Methylococcaceae, to 0%) at the end of phase III.
Bacterial Genera
Several identified bacterial genera (total of 22) were shared among AGS samples, representing between 58 (day 0—inoculum) and 83% (day 44—phase II) of the total relative abundance present in the AGS biomass.
Among Bacteroidetes members, Chryseobacterium, Taibaiella, Mariniflexile, Flavobacterium, Terrimonas, Chryseolinea and Leadbetterella were present in the core microbiome and together represented between 29 and 58% of the overall microbial community. Phenylobacterium, Asprobacter, Paracoccus, Novosphingobium, Acidovorax, Xenophilus, Thauera, and Plasticicumulans were identified within the Proteobacteria phylum (ca. 19–31%). Dermatophilus (Actinobacteria), Fusibacter (Firmicutes), and Nitrospira (Nitrospirae) were also identified in the core microbiome, contributing for 0.1–2% of the total community.
Figure 5 shows shifts of the most abundant bacterial genera (including also the ones absent in the core microbiome). Many bacteria kept their relative abundance throughout the process (Phenylobacterium, Thauera, Terrimonas, Paracoccus), while others, mainly Chryseobacterium, increased their dominance from phase I until the end. While Mariniflexile and Flavobacterium gradually increased, Haliscomenobacter decreased during reactor start-up, and Novosphingobium and Taibaiela decreased gradually their relative abundance along the process. Phase II conditions were possibly detrimental to certain bacterial genera, not detected at the end of this phase (Haliscomenobacter, Acinetobacter, Aquabacterium, and Ralstonia). Xenophilus relative abundance increased along phase I until the end of phase II, reducing its relative abundance thereafter. During phase III, the relative abundance of Methylocaldum and Plasticicumulans have declined while other bacterial genera proliferated (Chrysolinea, Ralstonia, Brevundimonas, and Gemmatimonas).
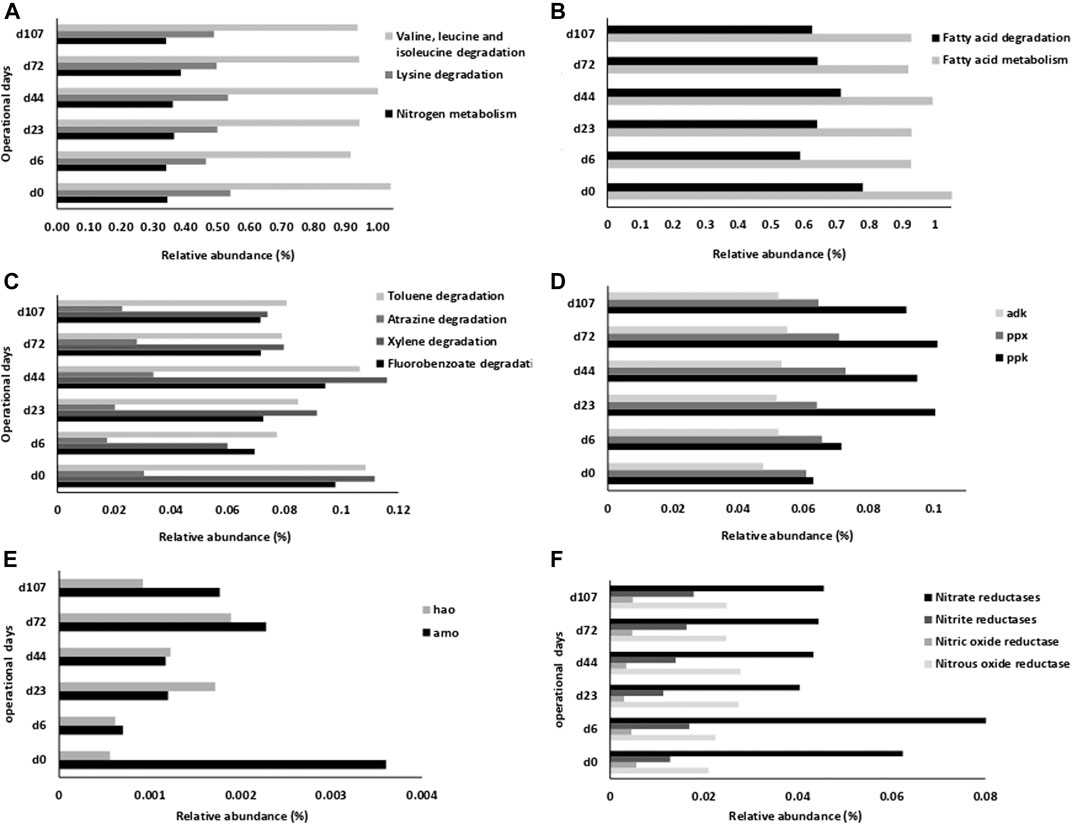
FIGURE 5. Heatmap presenting the evolution in dominant bacterial genera in the AGS biomass along the reactor operational phases, considering the first twelve dominant bacterial genera from each biomass sample.
Nitrifiers and Phosphate-Accumulating Organisms
Nitrifying bacteria were identified in several AGS samples, indicating the persistence of such group in the AGS. Nitrosomonas (AOB) were identified in the inoculum (0.3%), after 6 days of operation (0.8%) and at the end of phase III (1.3%) while Nitrospira (NOB) was detected in all AGS samples (0.55%, 1.46%, 0.45%, 0.39%, 0.40%, 0.70% of relative abundance, for days 0, 6, 23, 44, 72 and 107, respectively). Bacteria associated to phosphorous removal were detected throughout the process. Candidatus Accumulibacter was identified in the inoculum (0.6%), while Tetrasphaera were detected in all operational phases (between 0.1 and 1% of relative abundance). Gemmatimonas, a putative PAO, was identified in the AGS biomass from the end of phase I onwards, reaching its highest abundance at the end of phase III (2.7%) (data not shown).
Microbial Metabolic Function Prediction
Functional Genes Prediction
The in silico analysis of the AGS microbiome allowed predicting 358 functional encoding genes, with 226 (92% of total relative abundance) associated to prokaryotic cells and 132 genes (8% of total relative abundance) encoding for functions found in eukaryotic cells (e.g., human diseases and organismal systems), the latter not relevant and thus not included in further analysis. Considering all sampling days, the prokaryotic genes represented an average number of 213 ± 2, with 206 genes common to all. In general, the AGS presented a similar relative abundance of the main functional categories: the most abundant were associated to global and overview pathways (37.1 ± 0.2% of total relative abundance), followed by amino acid and carbohydrate metabolisms (8.0 ± 0.1% and 8.2 ± 0.1%, respectively) and environmental and genetic information processing and cellular processes (6.0 ± 0.3% and 5.7 ± 0.2%, respectively) (Supplementary Figure S4). Regarding specific functional categories, xenobiotics and lipid metabolism accounted for 3.0 ± 0.3% and 2.4 ± 0.1%, % of relative abundance, respectively. The presence of similar abundance of general and specific functional categories of genes along the process indicates that the AGS microbiome however dynamic, is stable at the functional level, independently of the bacterial genera dominating each operational phase.
The analysis of specific functional subcategories has shown differences between AGS samples along the process. The relative abundance of genes predicted for nitrogen metabolism slightly increased during the operational phases with higher OLR (days 23 and 44) (from 0.34 to 0.37%), but the highest predicted value (0.39%) was detected latter on phase III (day 72) (Figure 6A). Genes for the degradation of the amino acids lysine and valine, leucine and isoleucine were predicted with a higher relative abundance on days 0 and 44 (inoculum and phase II, respectively) (Figure 6A). The same pattern was observed for genes associated to fatty acid metabolism and fatty acid degradation (Figure 6B), for different xenobiotic compounds degradation (Figure 6C) and for bacterial biofilm formation (data not shown).
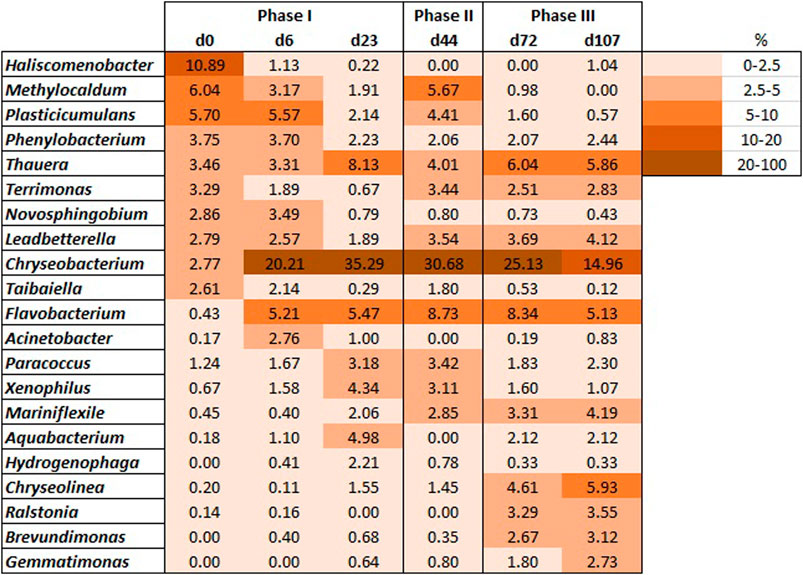
FIGURE 6. Relative abundance of genes predicted for degradation of specific amino acids and nitrogen metabolism (A), fatty acid degradation and metabolism (B), and xenobiotics degradation [toluene, atrazine, xylene, and fluorobenzoate) (C)]; Relative abundance of enzymes predicted for anaerobic phosphate release (adk, ppx) and aerobic phosphate accumulation (ppk) (D), for nitrification (amo and hao) (E) and for denitrification (F).
Prediction of Enzymes Associated With Nutrient Removal
The nitrification process requires the action of ammonia monooxygenase (amo), which catalyzes the first reaction of ammonia oxidation to nitrite, and of hydroxylamine dehydrogenase (hao), able to catalyze the second reaction in ammonia oxidation to nitrite. Both amo and hao enzymes were predicted in biomass during the entire process (Figure 6E). The amo enzyme was identified with much higher relative abundance in the inoculum (day 0), compared to the remaining operational days. The relative abundance of the amo enzyme decreased at the beginning of operation, recovering latter to higher relative abundance on days 72 and 107. On the other hand, the hao enzyme was predicted with lower relative abundance in the inoculum. Its relative abundance tended to increase after day 23 until the end of the assay, but this enzyme relative abundance was somewhat variable.
Denitrification requires the activity of nitrate, nitrite, nitric oxide, and nitrous oxide reductases. These enzymes have been predicted in the analysed AGS samples, namely different assimilatory and periplasmic nitrate reductases (Figure 6F). Nitrite, nitric, and nitrous oxide reductases were also identified in the biomass samples. The potential for nitrate reduction, by different nitrate reductases, was higher in the inoculum and initial days (day 0–0.062%; d6—0.081%), reducing their relative abundance to stable relative abundance values throughout the process (0.043 ± 0.002%). Nitrite, nitric oxide, and nitrous oxide reductases were predicted with similar relative abundance, for all AGS samples (0.015 ± 0.003%, 0.004 ± 0.001%, and 0.025 ± 0.003%, respectively).
Enzymes responsible for phosphate removal were also predicted. The anaerobic phosphate release is performed by the enzymes adelynate kinase (adk) and exopolyphosphatase (ppx), while the aerobic phosphate uptake is performed by a polyphosphate kinase (ppk). The adk enzyme was predicted with similar relative abundance in all AGS samples (between 0.048 and 0.055%) (Figure 6D) whereas the enzyme ppx slightly increased in relative abundance between days 23 and 72. The greatest variation for enzymes related to phosphate removal was detected for the enzyme ppk which presented a higher increase after reactor start-up.
Discussion
The AGS process was able to treat fish canning wastewater with variable content of organic matter and other components in its composition. The treatment of wastewater with higher organic load disturbed the nitrification process which was able to promptly recover (between 5 and 10 days) after decreasing the organic load and C/N ratio in wastewater. The correlation between biomass physical characteristics and physicochemical variables obtained from reactor performance indicated a greater biomass stability during the period of nitrification recovery. The AGS process was robust and able to rapidly reach and keep a stable functional microbial community despite treating variable wastewater composition, corroborated by the prediction of different functional genes and enzymes, which were identified throughout the process, indicating metabolic stability.
Despite the smaller difference between the OLRs applied during phases I and II compared to that in phase III (1.06 ± 0.23, 1.49 ± 0.14, 0.35 ± 0.20 g L−1 day−1, respectively), complete nitrification inhibition occurred during phase II. In fact, the wastewater fed during these two former operational phases presented different carbon and nitrogen content as the C/N ratio of phase II was twice higher than that on phase I. A previous study has shown that, together with the nitrogen load, the organic load present in the fish canning wastewater can indirectly influence nutrient removal efficiency, mainly nitrification (Paulo et al., 2021). However, besides the OLR, the C/N ratio can also disturb ammonia oxidation (Tang and Chen, 2015). Carrera et al. (2004) have studied the effect of COD/N on a biological nutrient removal (BNR) process and found out that the COD/N ratio increase (between 0.7 and 3.4) decreased the nitrification rate almost in 80%, while promoting the heterotrophic activity. The structural stability of the aerobic granules has also been associated to the presence of nitrifying bacteria, which can be regulated by the COD/N ratio of the wastewater (Liu et al., 2004). These observations are related to the fact that nitrifiers can be outcompeted by heterotrophic microorganisms, which uptake the excess of carbon while consuming the available oxygen (Mosquera-Corral et al., 2005; Pronk et al., 2015; Layer et al., 2019). The overgrowth of suspended biomass observed during phase II was probably the direct consequence of an increase in carbon concentration in wastewater that was not completely consumed during the anaerobic feeding period. The growth of suspended biomass due to the increased organic matter content in the wastewater, also associated to the presence of less diffusible carbon sources in complex wastewater, often occur in AGS processes treating industrial wastewater (Pronk et al., 2015; Carrera et al., 2019; Layer et al., 2019). In this way, nitrifiers were indirectly affected by the wastewater composition, which created unfavorable conditions for their activity. The lower OLR and C/N ratio applied afterwards were again more amenable to nitrifiers, associated to the absence of extra carbon during the aerobic period. Once the accumulated suspended biomass was washed out from the AGS reactor (Figures 2A,B), the competition for oxygen was reduced, enabling nitrification restart, which was not affected by its temporary inhibition. This is corroborated not only by the identification of Nitrosomonas (AOB) at the beginning and end of the process, but as well by the presence of Nitrospira (NOB) throughout the whole operational period. The robustness of the AGS process was confirmed mostly by the stability of NOB in the system, once these bacteria have a higher sensitivity to environmental changes than AOB (de Sousa Rollemberg et al., 2018; Liu et al., 2019). Besides this, the presence of amo and hao enzymes was predicted for all operational phases. After a first decrease, possibly due to the initial biomass adaptation, both enzymes were predicted with higher relative abundance at the end of phases I and II. An additional increase on these enzymes relative abundance was observed during phase III, which could be associated with nitrification recovery. This was also corroborated by the increase of relative abundance of genes involved in the nitrogen metabolism observed at the beginning of phase III.
The PCA analysis of QIA and physicochemical parameters indicated that changes in the AGS process were influenced by different variables throughout the operational phases. Samples were initially gathered in two disperse clusters, corresponding to the first two operational phases, but formed a denser cluster during the last phase. PCA results indicated that the AGS system was initially affected by reactor operational conditions and type of wastewater, which caused changes in the structure of the granules, mainly on the large ones. A gradual adaptation of the AGS to the wastewater was pointed out by the closer relationship obtained between biomass changes and the high organic matter content in the wastewater (end of phase II). During the last phase of operation, a greater similarity between the morphological characteristics (Rob, Round and Conv) of the granules was observed, probably due to the higher process stability as a lower OLR and C/N were applied, also an indication of robustness of the AGS system. Phase II was characterized by a closer relationship between OLR and, necessarily, COD in the wastewater, as well as with ammonia in the wastewater and in the outlet, in agreement with the nitrification disruption. The periods of higher nitrification activity were close related with the nitrate concentration in the outlet. The adaptation of granular biomass possibly led to a decrease of larger granules (LG) during phases I and II, which resulted in the predominance of intermediate granules (IG) during phase III. Overall, PCA results supported the fact that a high content in organic matter had possibly an effect on the aerobic granules physical properties, which were able to reach a higher stability during periods of lower carbon content in wastewater.
Despite the structural changes of the AGS biomass, the variation of wastewater composition was not found to markedly influence the microbial community composition. Similar to PCA results, the major difference was identified at the beginning of the operation, when the AGS microbiome presented a higher bacterial diversity. The adaptation to reactor operation and wastewater composition might have led to a lower bacterial diversity in AGS, but many bacterial genera were kept from the beginning until the end of operation. Thus, a stable core microbiome was present in AGS. While some bacteria were kept with similar relative abundance until the end of operation, some have suffered changes in their abundance. Although the carbon content of the wastewater might have been the key stressor for those changes, a stable carbon removal performance was kept along the process. In fact, the excess of carbon present at the beginning of the aerobic period during both phases I and II did not affect COD removal, which was kept stable, producing effluents with COD levels under the discharge limits. The ability of AGS processes for COD removal from fish canning wastewater has been previously observed (Corsino et al., 2016; Carrera et al., 2019; Paulo et al., 2021) At the basis of this ability is the fact that several metabolic functions occurring in an AGS process contributed for carbon removal, namely by assimilation, carbon uptake and storage (EPS and polyhydroxyalkanoates (PHA) production), denitrification and aerobic respiration (Szabó et al., 2017). Most of the microorganisms able to perform the referred processes are fast-growing bacteria, which combine several metabolic abilities, sharing functional redundancy and therefore, can easily proliferate and thus dominate the microbial community composition. While slow-growing bacteria, mostly associated with nutrient removal, are usually present in lower numbers and are, therefore, less represented within the AGS microbial population, a greater diversity of heterotrophs has been identified in different AGS processes (de Sousa Rollemberg et al., 2018; Winkler et al., 2018). Chryseobacterium, dominant in the AGS biomass, is a bacterium with this diverse metabolic versatility. This bacterium is an OHO (ordinary heterotrophic organism), with proteolytic activity, able to produce storage polymers, and has been recognized as a putative EPS producer (Szabó et al., 2017; de Sousa Rollemberg et al., 2018; Winkler et al., 2018; Hugo et al., 2019). The auto-aggregation index of Chryseobacterium was found to increase with COD concentration up to 2000 mg L−1, higher than the one quantified for Acinetobacter (Adav et al., 2010). Other bacteria identified in this study also combine different metabolic functions. Flavobacterium, also an OHO, has been appointed as a putative EPS producer, with hydrolytic activity (de Sousa Rollemberg et al., 2018; Winkler et al., 2018; Xia et al., 2018). Paracoccus, point out as a putative EPS producer with ability to store polymers and to denitrify (Szabó et al., 2017; Pishgar et al., 2019). Acinetobacter, an OHO able to perform heterotrophic nitrification as well as denitrification, under low nutrient conditions, is a putative EPS producer (Hamza et al., 2018; Winkler et al., 2018). Thauera, a putative EPS producer, also presents the ability to denitrify (Szabó et al., 2017; Winkler et al., 2018). Hydrogenophaga and Ralstonia are heterotrophic bacteria known for their ability to denitrify (Dalsing et al., 2015; Yingrui Liu et al., 2019). Aquabacterium, firstly isolated from biofilm formed in the drinking water system, is an OHO with ability to survive in environments with low carbon availability (Kalmbach et al., 1999). The outgrowth of Chryseobacterium might have led to the decrease of several OHO, such as Aquabacterium but also Haliscomenobacter, Acinetobacter and Ralstonia, no longer detected at the end of phase II. Their low numbers compared to the higher relative abundance of other OHO, might explain why these were not detected in the AGS biomass during this operational phase. Results indicate that Chyseobacterium was the OHO better adapted to the higher OLR, but possibly also to the composition of fish canning wastewater, being able not only to grow fast at the expense of proteins, but also to aggregate and be retained in the granules.
Regarding denitrification, results indicate that the potential for complete nitrogen removal was present in the AGS biomass even in the absence of optimal operational conditions and/or of extra carbon source, due to the versatility and diversity of denitrifying bacteria. Besides the identification of several bacterial genera as denitrifiers, enzymes associated to denitrification process were predicted from the AGS microbiome. Denitrification presented better conditions for its occurrence during phase I, due to a higher carbon availability. However, ammonia assimilation might have occurred during phases I and II, explaining the difference between TN values at the beginning and end of the aeration period. The balance for TN values as well as the nitrification performance indicate that no denitrification nor ammonia assimilation have occurred during phase III. The occurrence of simultaneous nitrification and denitrification in AGS processes requires optimal operational conditions, due to limitations associated to substrate availability and diffusion in the granules and to dissolved oxygen concentration (Manuel Layer et al., 2020). Real wastewater can be composed by less diffusible substrates, undermining the denitrification process. If, on one hand, wastewater with lower carbon content can lead to a more stable process performance, by promoting higher granules structural stability (Peyong et al., 2012), also protecting the nitrification process, on the other hand it can limit denitrification (Manuel Layer et al., 2020). Another important functional ability related to heterotrophic metabolism is the fact that OHO can obtain carbon and energy from simple and complex organic compounds, such as xenobiotics. Therefore, the higher carbon concentration present in the wastewater might have also increased the presence of bacteria with ability to degrade chemical compounds, as indicated by the higher number of genes predicted for xenobiotics degradation during the phase with higher OLR and C/N ratio. While OHO belonging to the Bacteroidetes phylum increased, Proteobacteria were possibly more affected by the higher OLR and C/N ratio. Amino acids and fatty acids are expected to be present in the fish canning wastewater. Also, the abundance of predicted genes for the metabolism of these groups of compounds increased during phase II, possibly due to their higher concentration in the wastewater.
Also detected in the AGS biomass, Taibaiella, Leadbetterella, Terrimonas, and Chryseolinea are associated to the hydrolysis and degradation of organic compounds (Abdullah et al., 2013; Szabó et al., 2017; Xu et al., 2018; Layer et al., 2020). Mariniflexile, a bacterium mostly found in saline water, might have been already present in the inoculum, a biomass previously adapted to fish canning wastewater. Brevundimonas is an aerobic oligotrophic bacteria, which can establish bacteria-algal associations, being previously identified in AGS biomass (Wang et al., 2020; Zou et al., 2021). The increase in relative abundance of several bacterial genera at the end of operation, namely of Ralstonia, Brevundimonas, Gemmatimonas, Aquabacterium, and Chryseolinea, might be related with a greater ability to survive in conditions with lower organic matter and nutrients availability.
A part of the carbon present in the wastewater is used by bacteria for EPS production, which is required for granules formation and for keeping their stability and integrity, while protecting bacteria from stress conditions (Szabó et al., 2017; Xia et al., 2018). The EPS are composed by a mixture of macromolecules resulting from microbial secretion, cell lysis or due to compounds present in the medium, being mainly composed by proteins, polysaccharides, humic-like substances, and nucleic acids (More et al., 2014; Ding et al., 2015). Results show that the biochemical composition of the EPS present in the AGS biomass changed throughout time, mostly regarding carbohydrates and humic acids content. Similar to bacterial metabolism, EPS bacterial production is regulated by the external environment. Therefore, several operational factors can influence EPS bacterial production in an AGS process, namely pH, shear stress, temperature, as well as the composition of the wastewater, also considering the C/N ratio (More et al., 2014). At the beginning of the operation, the AGS biomass presented higher EPS content, which decreased during operation. This higher total EPS content was mostly related to the content in humic acids of the inoculated biomass. Humic acids are not secreted by bacteria, and are believed to be either taken up from the environment (More et al., 2014) or resultant from substrate hydrolysis (Liu et al., 2021). The composition of the fish canning wastewater might not contribute to their presence in the EPS composition, possibly leading to its decrease in time. The carbohydrates content in EPS was more variable, presenting a significantly higher concentration during the phases with higher OLR. The higher carbon content present in the wastewater might result in higher amount of carbohydrates in the EPS similarly to what was observed in previous studies (Liu et al., 2021). This is in agreement with the results obtained, since the PN/PS ratio increased again at the end of phase III. Thauera, Paracoccus, Flavobacterium, Chryseobacterium, and Phenylobacterium (putative EPS producers) composed ca. 12% of the total relative abundance found in the inoculum, but rapidly increased up to 34% during the start-up, mainly due to the high relative abundance of Chryseobacterium. The highest amount of these bacteria was identified at the end of phase II (ca. 54%), gradually decreasing until 31% by the end of operation. This is also corroborated by the higher relative abundance of genes predicted for biofilm formation, associated to EPS bacterial production (Wu et al., 2019), when both OLR and C/N ratio were higher. Extra carbon can enhance biofilm production (Wu et al., 2019) and might also enhance the growth of EPS producing bacteria, which are heterotrophic bacteria.
Despite occurring at lower extent, phosphate removal also took place in the AGS process. The low amount of phosphate present in the wastewater, mostly during phases II and III, decreased the need for its removal. However, microbiome analysis results indicate that AGS biomass never lost the ability to remove phosphate. Apart from the identification of several PAOs in the AGS biomass, the prediction of enzymes associated to anaerobic phosphate release and aerobic phosphate uptake, indicate the persistence of such microorganisms in the microbiome.
Conclusion
The AGS process can be affected by the content in carbon present in food industry wastewater, by changing the nitrification process performance. Nitrification inhibition was quickly reverted when a lower OLR and C/N ratio were applied, indicating that a low OLR (below 1 g L−1 day−1) and possibly also a C/N ratio closer to 3 favor the carbon and ammonium removal from fish canning wastewater. This was corroborated by PCA analysis, which allowed identifying a more stable and robust system dynamics under this condition. Despite the variable composition of this type of wastewater, it was possible to indicate the main factor affecting the nitrification performance: this process inhibition was not a direct consequence of the presence of extra organic carbon in the wastewater but mostly due to the metabolic competition switch created by it. Therefore, the overgrowth of heterotrophic bacteria should be controlled to avoid the competition of fast growing bacteria with autotrophs, namely nitrifiers. On the other hand, the maximum salt concentration (6 g L−1) was not found to affect nitrification recovery. Microbiome analysis, together with metabolic function and enzymes prediction corroborated this hypothesis, by indicating the conservation of functional diversity required for carbon and nutrient removal. The stability of the microbial community composition was shown by a resilient core microbiome, dominated by Cryseobacterium, an EPS producer, with ability to grow and possibly also to aggregate better at higher OLR conditions. Changes in the relative abundance of specific bacteria, functional groups and enzymes, possibly resulted from an adaptation to the wastewater, demonstrating the microbial adaptability. Overall, there is a strong indication that the AGS biomass can keep a wide diversity of bacteria and, therefore, metabolic functions which allow the removal processes to adapt and recover once the conditions are again favorable.
Data Availability Statement
The raw sequence data obtained for the AGS biomass samples was deposited in Sequence Read Archive (SRA) from NCBI database, associated to the BioProject with accession number PRJNA742749.
Author Contributions
AP: Investigation, Formal analysis, Writing—original draft. CA: Conceptualization, Writing—review and editing, Funding acquisition. JC: Software, Formal analysis, Writing-review and editing. DM: Software, Writing—review and editing. EF: Supervision, Writing—review and editing. PC: Conceptualization, Supervision, Writing—review and editing.
Funding
This work was supported by National Funds from FCT—Fundação para a Ciência e a Tecnologia, through the project GReAT-PTDC/BTA-BTA/29970/2017 (POCI-01-0145-FEDER-029970).
Conflict of Interest
The authors declare that the research was conducted in the absence of any commercial or financial relationships that could be construed as a potential conflict of interest.
Publisher’s Note
All claims expressed in this article are solely those of the authors and do not necessarily represent those of their affiliated organizations, or those of the publisher, the editors and the reviewers. Any product that may be evaluated in this article, or claim that may be made by its manufacturer, is not guaranteed or endorsed by the publisher.
Acknowledgments
We would like to thank the scientific collaboration of CBQF under the FCT project UIDB/50016/2020 and of CEB under the FCT project UIDB/04469/2020. We would like also to thank “A Poveira S.A.” (Póvoa de Varzim, Portugal) for providing the fish canning wastewater.
Supplementary Material
The Supplementary Material for this article can be found online at: https://www.frontiersin.org/articles/10.3389/fenvs.2021.735607/full#supplementary-material
References
Abdullah, N., Yuzir, A., Curtis, T. P., Yahya, A., and Ujang, Z. (2013). Characterization of Aerobic Granular Sludge Treating High Strength Agro-Based Wastewater at Different Volumetric Loadings. Bioresour. Technology 127 (2013), 181–187. doi:10.1016/j.biortech.2012.09.047
Adav, S. S., Lee, D. J., and Lai, J. Y. (2010). Potential Cause of Aerobic Granular Sludge Breakdown at High Organic Loading Rates. Appl. Microbiol. Biotechnol. 85 (5), 1601–1610. doi:10.1007/s00253-009-2317-9
Amorim, C. L., Duque, A. F., Moreira, I. S., van Loosdrecht, M. C. M., and Castro, P. M. L. (2017). Technologies for the Treatment and Recovery of Nutrients from Industrial Wastewater. In Á. Val del Río, J. L. Campos Gómez, and A. Mosquera Corral (Eds.), Technologies for the Treatment and Recovery of Nutrients from Industrial Wastewater. doi:10.4018/978-1-5225-1037-6
Anthonisen, A. C., Loehr, R. C., Prakasam, T. B., and Srinath, E. G. (1976). Inhibition of Nitrification by Ammonia and Nitrous Acid. J. Water Pollut. Control. Fed. 48 (5), 835–852.
APHA (1998). Standard Methods for the Examination of Water and Wastewater. Washington, DC: American Public Health Association.
Bassin, J. P., Pronk, M., Muyzer, G., Kleerebezem, R., Dezotti, M., and van Loosdrecht, M. C. M. (2011). Effect of Elevated Salt Concentrations on the Aerobic Granular Sludge Process: Linking Microbial Activity with Microbial Community Structure. Appl. Environ. Microbiol. 77 (22), 7942–7953. doi:10.1128/AEM.05016-11
Cai, F., Lei, L., Li, Y., and Chen, Y. (2021). A Review of Aerobic Granular Sludge (AGS) Treating Recalcitrant Wastewater: Refractory Organics Removal Mechanism, Application and prospect. Sci. Total Environ. 782, 146852. doi:10.1016/j.scitotenv.2021.146852
Carrera, J., Vicent, T., and Lafuente, J. (2004). Effect of Influent COD/N Ratio on Biological Nitrogen Removal (BNR) from High-Strength Ammonium Industrial Wastewater. Process Biochem. 39 (12), 2035–2041. doi:10.1016/j.procbio.2003.10.005
Carrera, P., Campo, R., Méndez, R., Di Bella, G., Campos, J. L., Mosquera-Corral, A., et al. (2019). Does the Feeding Strategy Enhance the Aerobic Granular Sludge Stability Treating saline Effluents? Chemosphere 226, 865–873. doi:10.1016/j.chemosphere.2019.03.127
Corsino, S. F., Capodici, M., Morici, C., Torregrossa, M., and Viviani, G. (2016). Simultaneous Nitritation-Denitritation for the Treatment of High-Strength Nitrogen in Hypersaline Wastewater by Aerobic Granular Sludge. Water Res. 88, 329–336. doi:10.1016/j.watres.2015.10.041
Cristóvão, R. O., Botelho, C. M., Martins, R. J. E., Loureiro, J. M., and Boaventura, R. A. R. (2015). Fish Canning Industry Wastewater Treatment for Water Reuse - A Case Study. J. Clean. Prod. 87 (1), 603–612. doi:10.1016/j.jclepro.2014.10.076
Dalsing, B. L., Truchon, A. N., Gonzalez-Orta, E. T., Milling, A. S., and Allen, C. (2015). Ralstonia Solanacearum Uses Inorganic Nitrogen Metabolism for Virulence, ATP Production, and Detoxification in the Oxygen-Limited Host Xylem Environment. MBio 6 (2), 1–13. doi:10.1128/mBio.02471-14
De Kreuk, M. K., Heijnen, J. J., and Van Loosdrecht, M. C. M. (2005). Simultaneous COD, Nitrogen, and Phosphate Removal by Aerobic Granular Sludge. Biotechnol. Bioeng. 90 (6), 761–769. doi:10.1002/bit.20470
De Kreuk, M. K., Kishida, N., Tsuneda, S., and van Loosdrecht, M. C. M. (2010). Behavior of Polymeric Substrates in an Aerobic Granular Sludge System. Water Res. 44 (20), 5929–5938. doi:10.1016/j.watres.2010.07.033
de Sousa Rollemberg, S. L., Mendes Barros, A. R., Milen Firmino, P. I., and Bezerra dos Santos, A. (2018). Aerobic Granular Sludge: Cultivation Parameters and Removal Mechanisms. Bioresour. Technology 270 (September), 678–688. doi:10.1016/j.biortech.2018.08.130
Ding, Z., Bourven, I., Guibaud, G., van Hullebusch, E. D., Panico, A., Pirozzi, F., et al. (2015). Role of Extracellular Polymeric Substances (EPS) Production in Bioaggregation: Application to Wastewater Treatment. Appl. Microbiol. Biotechnol. 99 (23), 9883–9905. doi:10.1007/s00253-015-6964-8
Dubois, M., Gilles, K. A., Hamilton, J. K., Rebers, P. A., and Smith, F. (1956). Colorimetric Method for Determination of Sugars and Related Substances. Anal. Chem. 28 (3), 350–356. doi:10.1080/09670262.2012.70222510.1021/ac60111a017
Felz, S., Al-Zuhairy, S., Aarstad, O. A., van Loosdrecht, M. C. M., and Lin, Y. M. (2016). Extraction of Structural Extracellular Polymeric Substances from Aerobic Granular Sludge. JoVE 2016 (115), 1–8. doi:10.3791/54534
Fr/olund, B., Griebe, T., and Nielsen, P. H. (1995). Enzymatic Activity in the Activated-Sludge Floc Matrix. Appl. Microbiol. Biotechnol. 43 (4), 755–761. doi:10.1007/BF00164784
Guo, H., van Lier, J. B., and de Kreuk, M. (2020). Digestibility of Waste Aerobic Granular Sludge from a Full-Scale Municipal Wastewater Treatment System. Water Res. 173, 115617–7. doi:10.1016/j.watres.2020.115617
Hamza, R. A., Sheng, Z., Iorhemen, O. T., Zaghloul, M. S., and Tay, J. H. (2018). Impact of Food-To-Microorganisms Ratio on the Stability of Aerobic Granular Sludge Treating High-Strength Organic Wastewater. Water Res. 147, 287–298. doi:10.1016/j.watres.2018.09.061
Hamza, R. A., Zaghloul, M. S., Iorhemen, O. T., Sheng, Z., and Tay, J. H. (2019). Optimization of Organics to Nutrients (COD:N:P) Ratio for Aerobic Granular Sludge Treating High-Strength Organic Wastewater. Sci. Total Environ. 650, 3168–3179. doi:10.1016/j.scitotenv.2018.10.026
Hugo, C., Bernardet, J. F., Nicholson, A., and Kämpfer, P. (2019). “Chryseobacterium,” in Bergey’s Manual of Systematics of Archaea and Bacteria, 1–107. doi:10.1002/9781118960608.gbm00301.pub2
Iwai, S., Weinmaier, T., Schmidt, B. L., Albertson, D. G., Poloso, N. J., Dabbagh, K., et al. (2016). Piphillin: Improved Prediction of Metagenomic Content by Direct Inference from Human Microbiomes. PLoS ONE 11 (11), e0166104–18. doi:10.1371/journal.pone.0166104
Kalmbach, S., Manz, W., Wecke, J., and Szewzyk, U. (1999). Aquabacterium Gen. nov., with Description of Aquabacterium Citratiphilum Sp. nov., Aquabacterium Parvum Sp. Nov. And Aquabacterium Commune Sp. nov., Three In Situ Dominant Bacterial Species from the Berlin Drinking Water System. Int. J. Syst. Bacteriol. 49 (2), 769–777. doi:10.1099/00207713-49-2-769
Kisand, V., Cuadros, R., and Wikner, J. (2002). Phylogeny of Culturable Estuarine Bacteria Catabolizing Riverine Organic Matter in the Northern Baltic Sea. Appl. Environ. Microbiol. 68 (1), 379–388. doi:10.1128/AEM.68.1.379-388.2002
Layer, M., Adler, A., Reynaert, E., Hernandez, A., Pagni, M., Morgenroth, E., et al. (2019). Organic Substrate Diffusibility Governs Microbial Community Composition, Nutrient Removal Performance and Kinetics of Granulation of Aerobic Granular Sludge. Water Res. X 4, 100033. doi:10.1016/j.wroa.2019.100033
Layer, M., Villodres, M. G., Hernandez, A., Reynaert, E., Morgenroth, E., and Derlon, N. (2020). Limited Simultaneous Nitrification-Denitrification (SND) in Aerobic Granular Sludge Systems Treating Municipal Wastewater: Mechanisms and Practical Implications. Water Res. X. 7, 100048. doi:10.1016/j.wroa.2020.100048
Liu, X., Liu, J., Deng, D., Li, R., Guo, C., Ma, J., et al. (2021). Investigation of Extracellular Polymeric Substances (EPS) in Four Types of Sludge: Factors Influencing EPS Properties and Sludge Granulation. J. Water Process Eng., 40(December, 101924 2020). doi:10.1016/j.jwpe.2021.101924
Liu, Y., Wei, D., Xu, W., Feng, R., Du, B., and Wei, Q. (2019). Nitrogen Removal in a Combined Aerobic Granular Sludge and Solid-phase Biological Denitrification System: System Evaluation and Community Structure. Bioresour. Technology 288 (May), 121504. doi:10.1016/j.biortech.2019.121504
Liu, Y., Yang, S.-F., and Tay, J.-H. (2004). Improved Stability of Aerobic Granules by Selecting Slow-Growing Nitrifying Bacteria. J. Biotechnol. 108 (2), 161–169. doi:10.1016/j.jbiotec.2003.11.008
Lowry, O. H., Rosebrough, N. J., Randall, R. J., and Lewis, A. (1951). Protein Measurement with the Folin Phenol Reagent. J. Biol. Chem. 193 (2), 265–275. doi:10.1016/s0021-9258(19)52451-6
Luo, J., Hao, T., Wei, L., Mackey, H. R., Lin, Z., and Chen, G.-H. (2014). Impact of Influent COD/N Ratio on Disintegration of Aerobic Granular Sludge. Water Res. 62, 127–135. doi:10.1016/j.watres.2014.05.037
More, T. T., Yadav, J. S. S., Yan, S., Tyagi, R. D., and Surampalli, R. Y. (2014). Extracellular Polymeric Substances of Bacteria and Their Potential Environmental Applications. J. Environ. Manage. 144 (February), 1–25. doi:10.1016/j.jenvman.2014.05.0102015
Moreira, I. S., Amorim, C. L., Ribeiro, A. R., Mesquita, R. B. R., Rangel, A. O. S. S., van Loosdrecht, M. C. M., et al. (2015). Removal of Fluoxetine and its Effects in the Performance of an Aerobic Granular Sludge Sequential Batch Reactor. J. Hazard. Mater. 287, 93–101. doi:10.1016/j.jhazmat.2015.01.020
Mosqueracorral, A., Dekreuk, M., Heijnen, J., and Vanloosdrecht, M. (2005). Effects of Oxygen Concentration on N-Removal in an Aerobic Granular Sludge Reactor. Water Res. 39 (12), 2676–2686. doi:10.1016/j.watres.2005.04.065
Paulo, A. M. S., Amorim, C. L., Costa, J., Mesquita, D. P., Ferreira, E. C., and Castro, P. M. L. (2021). Long-term Stability of a Non-adapted Aerobic Granular Sludge Process Treating Fish Canning Wastewater Associated to EPS Producers in the Core Microbiome. Sci. Total Environ. 756, 144007. doi:10.1016/j.scitotenv.2020.144007
Peyong, Y. N., Zhou, Y., Abdullah, A. Z., and Vadivelu, V. (2012). The Effect of Organic Loading Rates and Nitrogenous Compounds on the Aerobic Granules Developed Using Low Strength Wastewater. Biochem. Eng. J. 67, 52–59. doi:10.1016/j.bej.2012.05.009
Pishgar, R., Dominic, J. A., Sheng, Z., and Tay, J. H. (2019). Influence of Operation Mode and Wastewater Strength on Aerobic Granulation at Pilot Scale: Startup Period, Granular Sludge Characteristics, and Effluent Quality. Water Res. 160, 81–96. doi:10.1016/j.watres.2019.05.026
Pronk, M., Abbas, B., Al-zuhairy, S. H. K., Kraan, R., Kleerebezem, R., and van Loosdrecht, M. C. M. (2015). Effect and Behaviour of Different Substrates in Relation to the Formation of Aerobic Granular Sludge. Appl. Microbiol. Biotechnol. 99 (12), 5257–5268. doi:10.1007/s00253-014-6358-3
Pronk, M., Bassin, J. P., De Kreuk, M. K., Kleerebezem, R., and Van Loosdrecht, M. C. M. (2014). Evaluating the Main and Side Effects of High Salinity on Aerobic Granular Sludge. Appl. Microbiol. Biotechnol. 98 (3), 1339–1348. doi:10.1007/s00253-013-4912-z
Ramos, C., Amorim, C. L., Mesquita, D. P., Ferreira, E. C., Carrera, J., and Castro, P. M. L. (2017). Simultaneous Partial Nitrification and 2-fluorophenol Biodegradation with Aerobic Granular Biomass: Reactor Performance and Microbial Communities. Bioresour. Technology 238, 232–240. doi:10.1016/j.biortech.2017.03.173
Szabó, E., Liébana, R., Hermansson, M., Modin, O., Persson, F., and Wilén, B.-M. (2017). Microbial Population Dynamics and Ecosystem Functions of Anoxic/aerobic Granular Sludge in Sequencing Batch Reactors Operated at Different Organic Loading Rates. Front. Microbiol. 8 (MAY), 1–14. doi:10.3389/fmicb.2017.00770
Tang, H. L., and Chen, H. (2015). Nitrification at Full-Scale Municipal Wastewater Treatment Plants: Evaluation of Inhibition and Bioaugmentation of Nitrifiers. Bioresour. Technology 190, 76–81. doi:10.1016/j.biortech.2015.04.063
Turner, S., Pryer, K. M., Miao, V. P. W., and Palmer, J. D. (1999). Investigating Deep Phylogenetic Relationships Among Cyanobacteria and Plastids by Small Subunit rRNA Sequence Analysis. J. Eukaryot. Microbiol. 46 (4), 327–338. doi:10.1111/j.1550-7408.1999.tb04612.x
Wang, J., Liu, Q., Wu, B., Hu, H., Dong, D., Yin, J., et al. (2020). Effect of Salinity on Mature Wastewater Treatment Biofilm Microbial Community Assembly and Metabolite Characteristics. Sci. Total Environ. 711, 134437. doi:10.1016/j.scitotenv.2019.134437
Wang, X., Chen, Z., Shen, J., Zhao, X., and Kang, J. (2019). Impact of Carbon to Nitrogen Ratio on the Performance of Aerobic Granular Reactor and Microbial Population Dynamics during Aerobic Sludge Granulation. Bioresour. Technology 271 (September 2018), 258–265. doi:10.1016/j.biortech.2018.09.119
Winkler, M.-K. H., Meunier, C., Henriet, O., Mahillon, J., Suárez-Ojeda, M. E., Del Moro, G., et al. (2018). An Integrative Review of Granular Sludge for the Biological Removal of Nutrients and Recalcitrant Organic Matter from Wastewater. Chem. Eng. J. 336 (July), 489–502. doi:10.1016/j.cej.2017.12.0262017
Wu, Y., Cai, P., Jing, X., Niu, X., Ji, D., Ashry, N. M., et al. (2019). Soil Biofilm Formation Enhances Microbial Community Diversity and Metabolic Activity. Environment International Environ. Int. 132(June), 105116. doi:10.1016/j.envint.2019.105116
Xia, J., Ye, L., Ren, H., and Zhang, X.-X. (2018). Microbial Community Structure and Function in Aerobic Granular Sludge. Appl. Microbiol. Biotechnol. 102 (9), 3967–3979. doi:10.1007/s00253-018-8905-9
Xu, J., He, J., Wang, M., and Li, L. (2018). Cultivation and Stable Operation of Aerobic Granular Sludge at Low Temperature by Sieving Out the Batt-like Sludge. Chemosphere 211, 1219–1227. doi:10.1016/j.chemosphere.2018.08.018
Keywords: aerobic granular sludge, food industry wastewater, organic load, carbon, nitrification, quantitative image analysis, microbiome, functional populations
Citation: Paulo AMS, Amorim CL, Costa J, Mesquita DP, Ferreira EC and Castro PML (2021) High Carbon Load in Food Processing Industrial Wastewater is a Driver for Metabolic Competition in Aerobic Granular Sludge. Front. Environ. Sci. 9:735607. doi: 10.3389/fenvs.2021.735607
Received: 02 July 2021; Accepted: 26 August 2021;
Published: 29 September 2021.
Edited by:
Michael F Cohen, Sonoma State University, United StatesReviewed by:
Hasnida Harun, Universiti Tun Hussein Onn Malaysia, MalaysiaGopala Krishna Gvt, PSNA College of Engineering and Technology, India
Copyright © 2021 Paulo, Amorim, Costa, Mesquita, Ferreira and Castro. This is an open-access article distributed under the terms of the Creative Commons Attribution License (CC BY). The use, distribution or reproduction in other forums is permitted, provided the original author(s) and the copyright owner(s) are credited and that the original publication in this journal is cited, in accordance with accepted academic practice. No use, distribution or reproduction is permitted which does not comply with these terms.
*Correspondence: Ana M. S. Paulo, apaulo@ucp.pt