- 1Global Centre for Environmental Remediation (GCER), College of Engineering, Science and Environment, The University of Newcastle, Callaghan, NSW, Australia
- 2Cooperative Research Centre for Contamination Assessment and Remediation of the Environment (CRC CARE), The University of Newcastle, Callaghan, NSW, Australia
- 3Department of Agriculture, Noakhali Science and Technology University (NSTU), Noakhali, Bangladesh
- 4Department of Soil Science, Bangladesh Agricultural University (BAU), Mymensingh, Bangladesh
- 5Institite of Medical and Biomedical Education, St George’s University of London, London, United Kingdom
Rice consumption is a major dietary source of Cd and poses a potential threat to human health. The aims of this study were to examine the influence of Fe and Cd application on yield and yield components, dynamics of Cd in pore water, translocation factors, daily dietary intake, and estimation of human health risks. A pot experiment was performed under glasshouse conditions where rice cultivars (Langi and Quest) were cultivated in two dissimilar soils under different levels of Cd (0, 1.0, and 3.0 mg kg−1) and Fe (0, 1.0, and 2.0 g kg−1). The results showed that variation in two rice cultivars in terms of yield and yield-related components was dose dependent. Cadmium concentration in soil pore water was decreased over time and increased with increasing Cd levels but decreased with Fe application. Translocation factors (TFs) from root to straw (TFroot-straw) or straw to husk (TFstraw-husk) were higher than root to grain (TFroot-grain) or straw to grain (TFstraw-grain). The Quest cultivar had 20% lower Cd than the Langi cultivar. Application of Fe at the rate of 1 and 2 g kg−1 soil reduced Cd by 23 and 46%, respectively. Average daily intake (ADI) of Cd exceeded the permissible limit (5.8 × 10−3 mg −1 kg−1 bw per week) when rice plant subjected 1 and 3 mg kg−1 Cd stress with or without Fe application. Results also indicated that ADI value was lower in the Quest cultivar as compared to the Langi cultivar. Estimation of human health risk revealed that the non-carcinogenic risks (HQ > 1) and carcinogenic risks (CR > 1.0 × 10−4) increased with increasing Cd levels in the soil. The application of Fe decreased the human health risks from rice consumption which is more pronounced in Fe 2.0 than in Fe1.0 treatments. The rice cultivar grown in soil-1 (pH 4.6) showed the highest health risks as compared to soil-2 (pH 6.6) and the Quest cultivar had lower health risks than the Langi cultivar.
Introduction
Cadmium (Cd) is considered as one of the mobile and highly toxic heavy metals that exists widely in the soil environment at very low concentrations, usually <0.5 mg kg−1 (Khan et al., 2017; Zhou et al., 2018). Human interventions such as mining and smelting activities, burning of fossil fuel, sewage sludge application, and phosphate fertilizer application result in the increase of Cd concentrations in the soil (Rizwan et al., 2016; Khan et al., 2017). Cd is a non-essential trace metal which at concentrations over a certain level can impair the yield and quality of crops (Kanu et al., 2017; Zhou et al., 2018). For example, Kanu et al. (2017) reported that 1, 2, and 3 mg kg−1 of Cd in soils decreased the grain yield by 34, 43, and 63% in a rice variety and decreased protein and amylose contents. Cd is readily taken up by roots of the rice plants cultivated in Cd polluted soil, and it is translocated to edible portions (Song et al., 2015). Cd accumulation is governed by Cd bioavailability in the soil and the inherent capacity of the crop plant (Grant et al., 2008; Roberts, 2014). Compared with other cereals, rice (Oryza sativa L.), which is the principal food for more than half of the world’s population, is highly efficient in Cd accumulation and transfer from soil to grain (Shahriar et al., 2020). A high concentration of Cd in cultivated land is a serious environmental issue as the toxicity of Cd can pose a threat to food security, impacting both yield and yield components of rice plants (Meharg et al., 2013; Herath et al., 2014). There are several studies reported that Cd toxicity retarded the growth and biomass of rice plants ( Ahsan et al., 2007; Li et al., 2012; Wang et al., 2014). Cd toxicity in rice plants reduced yield formation and yield components in terms of panicle numbers, grains per panicle, and filled grains per panicle (Kanu et al., 2017). It was found that biomass, plant height, and photosynthetic rate of rice plants were reduced under Cd stressful conditions (Rizwan et al., 2017). Some studies indicated that plant height, fresh weight, and chlorophyll content of rice plants were decreased when rice plants were exposed to Cd toxicity (Wu et al., 2014). Liu et al. (2007) demonstrated that Cd stress significantly reduced plant height, tillering capacity, leaf area, dry matter accumulation, and grain yield of rice plants. Feng et al. (2018) also showed that application of 1 and 5 mg kg−1 Cd to soil reduced plant biomass, plant height, and photosynthetic rate of plant with severe toxicity in case of 5 mg kg−1 treatment.
Iron (Fe) is an essential micronutrient for plant growth and development, and it functions as a co-factor of many enzymatic reactions in the plant (Liu et al., 2017). Generally, Fe contents in soils influenced Cd accumulation in the plant, restricting Cd in roots and inhibited its uptake and translocation to grains (Shao et al., 2008; Liu et al., 2010). Liu et al. (2008) suggested that enhanced Fe levels decreased Cd accumulation and alleviated Cd toxicity in rice plants. Hence, Fe nourishment was shown as an effective remediation technique to prevent Cd accumulation and Fe deficiency in rice plants when cultivated in Cd-contaminated soil (Sebastian and Prasad, 2016). Fe supplementation promoted the formation of Fe oxides/hydroxides in soil which have ample functional groups and large surface area with abundant reactive sites for binding Cd (Li et al., 2020). Thus, Fe oxides decreased Cd mobility and availability in soil due to the transformation of available Cd to Fe oxide-bounded Cd (Li et al., 2019). Besides, Fe plaque is precipitated on the root surface of rice as a result of oxidation of ferrous ion to ferric ion (Liu et al., 2008; Cheng et al., 2014; Sebastian and Prasad, 2016). Fe plaque increased Cd adsorption/co-precipitation on the root surface, thereby limiting Cd uptake and transfer in rice tissues (Cao et al., 2018; Zhang et al., 2019). In addition, Cd can be transported into rice via the Fe uptake pathway; thus, Fe competes for the same transporters (OsNRAMP1, OsNRAMP5, and OsIRT1) with Cd and restricts Cd uptake and translocation within rice plants (Takahashi et al., 2011; Ishimaru et al., 2012; Takahashi et al., 2014). The accumulation of Cd in rice plants is also affected by rice cultivars (Ye et al., 2012). There is a considerable variation in Cd uptake and accumulation among different rice genotypes (Arao and Ae, 2003). Different uptake and translocation of Cd in crops have been noted among the plant species and among the cultivars within the same species (Liu et al., 2007; Liu et al., 2010; Sebastian and Prasad, 2016).
Apart from the rice cultivar, the paddy soil type in terms of pH is also a crucial soil property influencing the bioaccumulation of Cd from soil to rice plant (Zhao et al., 2010; Ye et al., 2012). Generally, Cd accumulation in rice grain is significantly and negatively correlated with soil pH (Honma et al., 2016; Gu et al., 2018; Hussain et al., 2021a). The movement and bioaccumulation of Cd promoted the decrease in soil pH because protonation increased at low soil pH led to reducing the negative charge on the soil surface (Rajkumar et al., 2012; Gu et al., 2018; Zhao and Wang, 2020). In this case, Cd solubility and phytoaccumulation are enhanced via the transformation of Cd from stable fractions (Fe-Mn oxide bound and carbonate structures) to easily exchangeable fractions (mobile and bioavailable structures) (Li et al., 2017; Hussain et al., 2021a; Hussain et al., 2021b). With the increase in soil pH, the solubility and distribution of Cd decreased via increasing the complexation by adsorptive sites on the soil surfaces (Wang et al., 2019; Hussain et al., 2021a).
Elevated Cd concentration in edible portions of crop plants causes serious health hazards through food chain contamination leading to chronic exposure (Grant et al., 2008). Nowadays, dietary intake is recognized as a dominant pathway of Cd exposure to human beings (Zhou et al., 2015; Halim et al., 2020; Chen et al., 2021; Yan et al., 2021). For example, it was estimated that about 90% of the non-smoking general population was exposed to Cd through contaminated food consumption (Clemens et al., 2013; Chen et al., 2018b). Several toxicological studies have shown that Cd contamination is one of the serious environmental issues which disrupted food safety and threatened human health (Zhao et al., 2015; Chen et al., 2018b; Shahriar et al., 2020). Chronic exposure to Cd is responsible for a number of diseases in humans such as skeletal damage, cardiac failure, reproductive effects, hypertension, osteoporosis, anemia, damage to lungs, renal dysfunctions, and cancers (Akesson et al., 2014; Aoshima, 2016). The world’s first documented incidence of Cd poisoning was in Japan during the 1950s through the outbreak of “Itai-Itai disease” that occurred due to ingestion of Cd-contaminated rice cultivated in the Jinzu River basin of Toyama prefecture (Aoshima, 2016).
Rice consumption is considered to be the significant pathway of Cd exposure and contributed more than 50% of the total dietary intake of Cd (Song et al., 2017; Chen et al., 2021). It is of significance, particularly in the Asian subcontinent, including Bangladesh, India, China, Japan, Sri Lanka, and Pakistan, etc. (Meharg et al., 2013; Siddique et al., 2021). Thus, there is an urgent need to develop mitigation strategies to reduce Cd toxicity in rice plants and associated health risks from rice consumption. Health risk estimation of Cd exposure from rice intake is crucial as it provides important information on risk management and enables action required to minimize risk so that human health is protected. However, few studies have investigated the human health risk due to Cd intake from rice under the influence of Fe application while using different rice cultivars grown in different soil types. The present study was designed to investigate the Cd toxicity in two different rice cultivars grown in two contrasting soils of Australia spiked with different levels of Cd and Fe and estimation of associated human health risks. The overall objectives of this study are as follows: 1) the effect of Cd levels on yield components and yield of rice cultivars, 2) the exploration of Cd dynamics in soil pore water and translocation factors, and 3) the estimation of human health risks in terms of non-carcinogenic risks which is defined as the hazard quotient (HQ) and carcinogenic risks (CR).
Methodology
Preparation of Soils for Pot Study
Two types of soil (soil-1, low pH, and soil-2, neutral pH) were sampled at a depth of 0–15 cm from uncontaminated rice growing area for this pot study. Soil-1 was collected from the Leeton Field Station, while soil-2 was collected from Yanco Agricultural Institute, New South Wales (NSW), Australia. Approximately 1 ton of soils was collected from each site. Soils were collected from a single sampling point at 4 m × 4 m grid area. Collected soils samples were air-dried under the sun and unwanted materials, e.g., plant debris, stones, pebbles, etc., were cleaned. Each soil was homogenized and crushed to pass through a 4 mm mesh-sieve before being used to fill pots. A subsample (approx. 500 g soil) was further crushed and screened by a sieve (<2 mm mesh) and used for characterization of basic soil properties. Basic physico-chemical characteristics of the soil are summarized in Table 1. The pots used in this experiment were 9 L rectangular plastic pots (length 31 cm, width 22 cm, and height 18 cm) loaded with 6 kg of the test soil. The pot trial was set up in a greenhouse at the University of Newcastle, Australia. The day and night temperatures were adjusted to 28°C and 22°C with 85% RH (relative humidity) under ambient photoperiod (240–350 μmol m−2s−1). The entire experimental procedure is illustrated in Figure 1.
Preparation of Rice Cultivars
Two commonly grown Australian rice cultivars, the Langi rice cultivar—long grain and the Quest rice cultivar—medium grain, were secured from the Department of Primary Industries, NSW, Australia, and used as plant materials in this study. Sodium hypochlorite (NaOCl) solution (1% v/v) and Decon (0.5 ml) were used to sterilize the rice seeds for 20 min followed by 3–4 times washing with ultra-pure water (Lee et al., 2012). Sterilized rice seeds were allowed to germinate on a plastic tray with a bottom portion covered with the moistened paper towel in the a constant room temperature (30°C) of the laboratory. After 72 h, all sprouted rice seeds were then relocated to a seedling growing media and nurtured for 22 days. Healthy uniform size seedlings were chosen and pulled out with care and then transplanted into plastic pots. Each plastic pot was received two hills and two rice seedlings were placed in each hill. The rice plants are cultivated under flooded conditions of about 2–3 cm standing water layer above the soil surface during the whole growth period. Randomized complete block design (RCBD) was utilized to organize each pot.
Treatments and Fertilizer Application
The pot soils were spiked with three levels of Cd at the rates of 0 (Cd0), 1 (Cd1.0), and 3 (Cd3.0) mg Cd kg−1 soil (as cadmium nitrate: Cd(NO3)2.4H2O) and Fe at the rates of 0 (Fe0), 1 (Fe1.0) and 2 (Fe2.0) g Fe kg−1 soil (as ferrous sulfate: FeSO4.7H2O). The levels of Cd were selected based on the concentration usually found in the paddy field soil (Jinadasa, 1999; Machiwa, 2010; Satpathy et al., 2014; Honma et al., 2016; Liu et al., 2016; Duan et al., 2017). Ultra-pure water was used to dissolve Cd(NO3)2.4H2O and FeSO4.7H2O separately and properly mixed with the pot soils. The pot with spiked soils was equilibrated for 1 month, maintaining 80% water-holding capacity beforehand transferring rice seedlings. Therefore, the Cd and Fe combination has given a total of nine treatments which designated as Fe0Cd0, Fe0Cd1.0, Fe0Cd3.0, Fe1.0Cd0, Fe1.0Cd1.0, Fe1.0Cd3.0, Fe2.0Cd0, Fe2.0Cd1.0, and Fe2.0Cd3.0. The study consisted of three replications per treatment resulting in a total of 108 pots. Chemical fertilizer used in each pot soil and application rates per kg soil were N:200 mg, P:65 mg, K:160 mg, and S:75 mg in the form of urea (CO(NH2)2), triple superphosphate (Ca(H2PO4), muriate of potash (KCl), and gypsum (CaSO4.2H2O), respectively. All fertilizers except N were applied to the pot soil as basal dose before transplanting of rice seedlings. The N fertilizer addition was done at 7, 30, and 60 days after transplanting rice seedlings in 3 equal splits. Intercultural operations were performed as and when necessary throughout the entire growth period. The pot experiment started in April and continued up to August in 2018.
Collection of Soil Pore Water and Analysis
Soil pore water was collected four times at 10, 30, 60, and 110 days after the transplanting of rice seedlings into a plastic pot during the entire plant growth. Soil pore water was extracted using the Rhizon soil pore water sampler (Rhizosphere Research Products, Netherlands). The Rhizon sampler was vertically inserted into pot soil up to a depth of 10 cm from the soil surface near the center after puddling of pot soil but before transplanting of rice seedlings. 10 ml of soil pore water was collected using a syringe and filtered through a 0.45 um syringe filter into a prewashed plastic tube. The filtered soil pore water was acidified immediately with high purity nitric acid (7 M) to measure dissolved metals using ICP-MS, Agilent 7900, Japan.
Measurement of Yield and Its Components
At the mature stage, rice plants from each pot were harvested on August 22, 2018, using sharp stainless-steel scissors. After harvesting, whole rice plants were rinsed at least 3-4 times with deionized water followed by ultra-pure water and placed on a plastic sheet for sun drying. Yield-related components were categorized as the number of tillers per hill, plant height, panicle length, and filled grains per panicle from 5 individual rice plants which were randomly selected from each pot. Plant height and panicle length were recorded manually using measuring tape. The number of tillers per pot was recorded before harvesting rice plants. Filled grains were separated from each panicle and counted manually. The straw and grains weights were recorded with the help of electric balance. After oven drying, whole paddy rice was separated into rice grains and husk. Rice grains were finely ground and digested following the procedure described by Rahman et al. (2009). The concentration of Cd in rice grains was measured by ICP-MS (Agilent 7900, Japan). Rice flour-SRM1568b (NIST, United States) was used as standard reference materials for quality control. Blanks, duplicates, and continuing calibration verifications (CCVs) were also employed in each batch of Cd analysis. The observed value of Cd in rice flour was 20.43 ± 0.84 µg kg−1, which indicated 91% recovery of the certified value (22.4 ± 0.1.3 µg kg−1). The translocation factors (TFs) of Cd from root to straw (TFroot-straw), root to grain (TFroot-grain), straw to husk (TFstraw-husk), and straw to grain (TFstraw-grain) was measured by estimating Cd concentration in one part with respect to the other parts as described by Khaliq et al. (2019).
Estimation of Health Risk From Cd Exposure
In this study, the potential health risks from Cd contamination through consumption of rice was estimated by calculating the average daily intake (ADI), hazard quotient (HQ), and cancer risk (CR) using the following equations:
where
ADI = estimated daily intake of Cd from rice consumption (mg d−1) per BM (kg);
CCd = concentration of Cd in rice (mg kg−1);
IngR = ingestion rate of rice (0.432 kg d−1) for adults obtained from Islam et al. (2017);
BM = body mass (70 kg) (Pirsaheb et al., 2021);
EF = exposure frequency (365 days yr−1);
ED = exposure duration (70 years);
AT = average exposure time (365 days× ED = 25,550 days);
RfD = oral reference dose (1 × 10−3 mg kg−1 daily for Cd) as suggested by USEPA (IARC, 2012);
CSF = cancer slope factor (6.3 mg kg−1 per day) (Kusin et al., 2018; Pirsaheb et al., 2021).
In terms of non-carcinogenic risk, HQ > 1 denotes significant adverse human health effects due to the presence of a particular element in the diet, while HQ < 1 indicates no significant risk is anticipated (Zhuang et al., 2016; Abtahi et al., 2017; Antoniadis et al., 2019; Halder et al., 2020). In case of carcinogenic risk, if CR value surpassing 1.0 × 10−4 is considered as unacceptable whereas CR value below 1.0 × 10−6 is not considered to develop significant health effect, CR values from 1.0 × 10−6 to 1.0 × 10−4 are usually supposed to be an acceptable range (IARC, 2012).
Statistical Analysis
JMP Pro software version 14.2.0 software was utilized to perform all statistical analyses. Three-way full factorial analysis of variance (ANOVA) was applied to test the significance of the different treatments. To determine the comparison between variance, a student’s t-test was utilized and a significance test was done at the 0.05 level. GraphPad Prism Software (Version: 9.0.0) was used to generate all figures. Data from the statistical analysis were expressed as means ± standard error (SE) (n = 3).
Results and Discussion
Effect of Cd and Fe on Yield and Yield Components of Rice Cultivars
Yield and yield components of two rice cultivars were determined after harvesting of whole rice plant and results are demonstrated in Tables 2, 3. There was a wide variation in yield components and yield of two rice cultivars. In terms of performance, the Quest cultivar produced the highest number of tillers per hill, plant height, panicle length, number of filled grains per panicle, straw yield, and grain yield than the Langi cultivar (Table 2). From Table 2, it was revealed that the number of tiller per hill, filled grains per panicle, straw yield, and grain yield were decreased by 37,11, 30, and 32%, respectively, in soil 1 (pH 4.6) compared to soil 2 (pH 6.6). However, no changes were observed for plant height and panicle between soils 1 and 2. The results showed that soil pH significantly influenced the grain yield. Results indicated that variation in yield components and the yield of the rice cultivar were dose dependent. The difference between Cd1.0 and Cd3.0 was statistically identical except plant height and filled grains per panicle for Langi and filled grains per panicle for the Quest cultivar (Table 3). The values of yield components and the yield of the rice cultivar were decreased with the increasing Cd level in the soil (Table 3). Compared to control, the number of tillers per hill was reduced by 16.5 and 24.1% for the Langi cultivar while 12.3 and 19.9% for the Quest cultivar at 1 and 3 mg kg−1 Cd treatments. Similarly, plant height, panicle length, the number of filled grains per panicle, straw yield, and grain yield of the Langi cultivar was reduced by 3.9, 5.6, 8.7, 18.3, and 20.3%, respectively, at Cd1.0 while 9.5, 9.3, 17.2, 28.4, and 33.3%, respectively, at Cd3.0 treatment compared to the Cd control. On the other hand, the plant height, panicle length, number of filled grains per panicle, straw yield, and grain yield of the Quest cultivar were decreased by 3.5, 2.7, 6.2, 12.2, and 16.5%, respectively, at Cd1.0, while 5.5, 4.8, 14.8, 17.3, and 29.4%, respectively, at Cd3.0 treatment, compared to the Cd control. The difference in yield components and yield may be related to the inherent tolerance capacity of the rice cultivar to Cd stress and the toxic effect of external Cd was less in the Quest cultivar compared to the Langi cultivar. A decrease in yield and yield components of the rice cultivar through the application of exogenous Cd has been observed by Kanu et al. (2017), and the negative consequences were greatly varied depending on the rice cultivar and levels of Cd toxicity in the soil. Reduction of yield and yield-related components due to Cd toxicity was also reported by Herath et al. (2014). The application of Fe in the soil increased the number of tillers per hill, plant height, panicle length, number of filled grains per panicle, straw yield, and grain yield of the rice cultivar, and this was more pronounced in Fe1.0 than Fe2.0 compared to the control (Table 4). Also, in previous studies, it has been observed that biomass of straw and grain of rice plant was enhanced through the moderate Fe (1 g kg−1) application compared to high Fe exposure (2 g kg−1) to the soil (Liu et al., 2020). This is likely because moderate Fe supplementation can enhance rice growth and promote photosynthesis and a reverse situation is observed when a high Fe dose is applied (Pinto et al., 2016). Yield components and yields of rice cultivar were significantly (p <0 .0001) influenced by combined Fe and Cd supplementation into the soil (Table 5). The addition of 1 and 2 g kg−1 Fe significantly increased rice cultivar's yield components and yield compared to Fe0 with no Cd supply, but the increment was less in Fe2.0 treatment than the Fe1.0. Rice cultivar subjected to moderate Fe supply (1 g kg−1) combined with Cd still produced higher yield components and yield than higher Fe application (2 g kg−1). For example, the grain yield of the Langi cultivar was increased by 67.3 and 33.2% at Fe1.0 and Fe2.0, whereas the grain yield of Quest was increased by 60.1 and 25.9% at Fe1.0 and Fe2.0 treatment under the Cd1.0 addition. At Cd3.0, grain yield increased by 55.8 and 21.6% for the Langi cultivar and 54.7 and 23.4% for the Quest cultivar at 1 and 2 g kg−1 Fe application. Yield components and yield of both rice cultivars were slightly reduced with Cd3.0 application as compared with Cd1.0. Higher values of yield components and yield were observed at moderate Fe supply because moderate Fe supply reduced Cd toxicity in rice plants through enhancing growth, photosynthetic activity, stomatal conductance with no visual symptoms of Fe toxicity (Liu et al., 2010). On the other hand, high Fe supply combined with Cd stress reduced photosynthetic rate and deactivated antioxidative systems resulting in lower biomass and grain yield (Liu et al., 2020). So it is recommended to use suitable doses of Fe in the rice field to promote rice production and combat Cd toxicity in rice plants when cultivated in the Cd-contaminated soil.
Cd Dynamics in Soil Pore Water
There was a significant difference (p < 0.001) in soil pore water Cd concentration over time (Figure 2). The Cd concentration in soil pore water reached 70.75 μg L−1 by 10 days after transplanting (DAT) of rice seedlings and then gradually decreased to 24.48 μg L−1 before harvesting of rice plant (110 DAT) (Figure 2). Soil pore water sampled at the flowering stage (after 60 DAT) contained less Cd than initial samples. Our results are consistent with an earlier study conducted in Canada by Gao et al. (2011), showing that Cd concentration in soil pore water ranged from 20 to 80 μg L−1. Similar results of Cd in soil pore water were reported in previous studies (Meers et al., 2007; Beesley and Dickinson, 2009). The Cd concentration in soil pore water was significantly affected by Cd and Fe addition in soils (Figure 3). Compared to control, application of 1 and 3 mg kg−1 Cd in the soil increased Cd concentration in pore water by 48.3 and 76.1%, respectively, under Fe0 treatment. A recent study by Wang et al. (2021) showed that Cd contents in pore water of soil increased with the increasing level of Cd in the soil and ranged from 0.11 to 1.7 mg L−1 in the red soil and 0.06–1.3 mg L−1 in the yellow brown soil, respectively, when soil spiked with five levels of Cd (0, 1, 2, 2.5, 5.0, 7.5, and 10 mg kg−1 soil). At Cd1.0 treatment, the pore water Cd concentrations were decreased by 31.5 and 58.4% with the addition of 1 and 2 g kg−1 Fe, respectively, as compared to corresponding Fe control. Addition of 1 and 2 g kg−1 soil reduced pore water Cd concentrations by 23.7 and 43.6% under 3 mg kg−1 Cd supplementation, respectively, when compared with Fe control. The results of this study demonstrated that pore water Cd concentration decreased due to the addition of Fe to the soil. It was well accepted that application of Fe increased the formation of Fe oxides in soil pore water having large surface area along with more functional groups with abundant reactive sites to adsorb Cd, thereby reducing Cd concentration in pore water (Jiang et al., 2017; Zhu et al., 2018; Li et al., 2020). Besides, Fe plaque is deposited around the root surfaces with Fe addition that impede Cd uptake and accumulation through adsorption or co-precipitation, thereby decreasing pore water Cd, which reduced potential Cd transfer from root to rice grain (Wan et al., 2018; Deng et al., 2020).
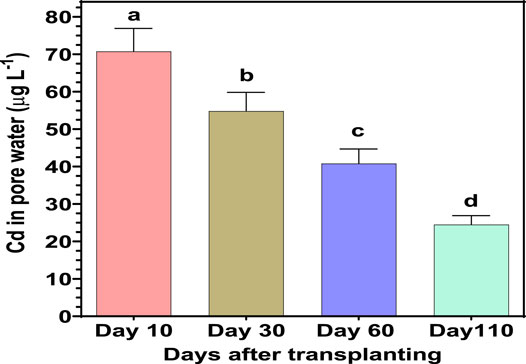
FIGURE 2. Reduction of total Cd concentration in pore water over time. Levels not connected by the same letter are significantly different (p < 0.001). Data are mean ± SE (standard error).
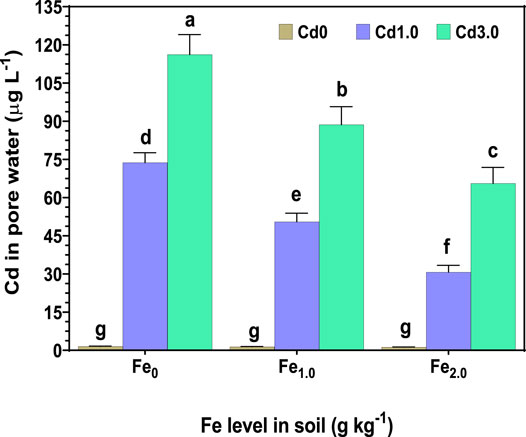
FIGURE 3. Concentration of Cd in pore water at different levels of supplied Fe and Cd in soil. Levels not connected by the same letter are significantly different (p < 0.001). Data are mean ± SE (standard error).
Translocation Factor
Translocation factors (TFs) of Cd from root to straw, root to grain, straw to husk, and straw to grain were determined and demonstrated in Figures 4, 5. TFs between the different parts of root-rice systems varied greatly and depending on the external Cd and Fe application and rice cultivar. TF of Cd from root to straw (TFroot-straw) was varied between 0.20–0.33, while from root to grain (TFroot-grain), the value lies between 0.01–0.02. TF from root to straw (TFroot-straw) was an order of magnitude higher than root to grain (TFroot-grain) (Figure 4), showing stronger accumulation ability of Cd by straw as compared to the translocation of Cd in rice grain. A similar finding was reported by Deng et al. (2020), who showed that the mean TF of Cd occurred in the order of TFroot-straw > TFroot-grain ranging between 0.38–0.46 for TFroot-straw and 0.07–0.08 for TFroot-grain, respectively. On the other hand, TF of Cd from straw to husk (TFstraw-husk) was varied between 0.12–0.26, while from straw to grain (TFstraw-grain), it ranged between 0.06–0.11. TF from straw to grain (TFstraw-grain) was lower than straw to husk (TFstraw-husk) (Figure 5), showing the inherent ability of rice plants to accumulate more Cd in the husk and less transfer to rice grain. These results were consistent with the outcomes from Deng et al. (2020), who observed that Cd translocation in terms of TFstraw-husk was higher than TFstraw-grain ranging between 0.20–0.24 for TFstraw-husk and 0.18–0.20 for TFstraw-grain, respectively. Similarly, Khaliq et al. (2019) also reported that the TF of Cd in rice grain was much lower as compared to the different parts of the rice plant. The translocation of Cd between different parts of the rice cultivar trended to increase with the increasing Cd level and decrease with increasing Fe concentration. With regard to the cultivar performance, the Quest cultivar had lower Cd translocation factors than Langi cultivars. Similar results were found by Kanu et al. (2017), who studied five rice cultivars and showed that the translocation factor for Xiangyxiangzhan and Guixiangzhan rice cultivars was minimal compared to Meixiangzhan, Basmati, and Nongxiang18 rice cultivars. The translocation factors of Cd into different parts of the rice plant for the Fe2.0 group were significantly lower than those for control and Fe1.0 under Cd1.0 and Cd3.0 treatments. The TF values were increased with the increasing Cd level which is supported by Kanu et al. (2017), who observed the highest TF values when rice plants are subjected to Cd treatment.
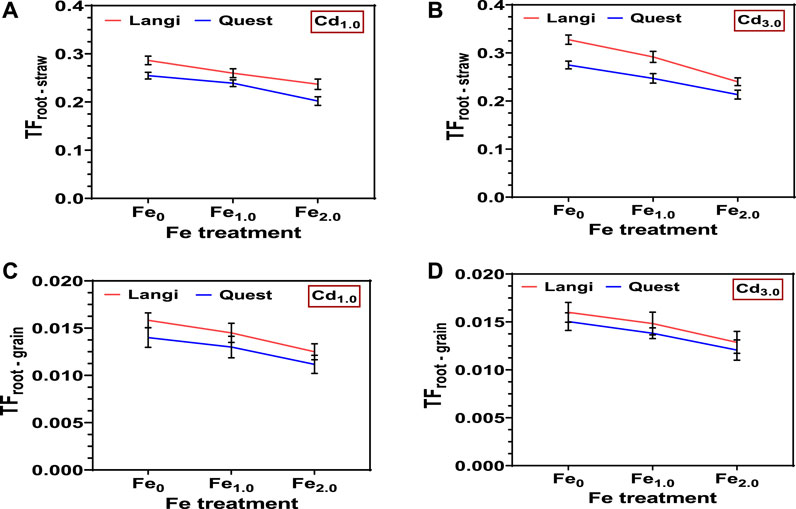
FIGURE 4. Effect of Fe on translocation factors of Cd from root to straw (A,B) and root to grain (C,D) between two Cd levels.
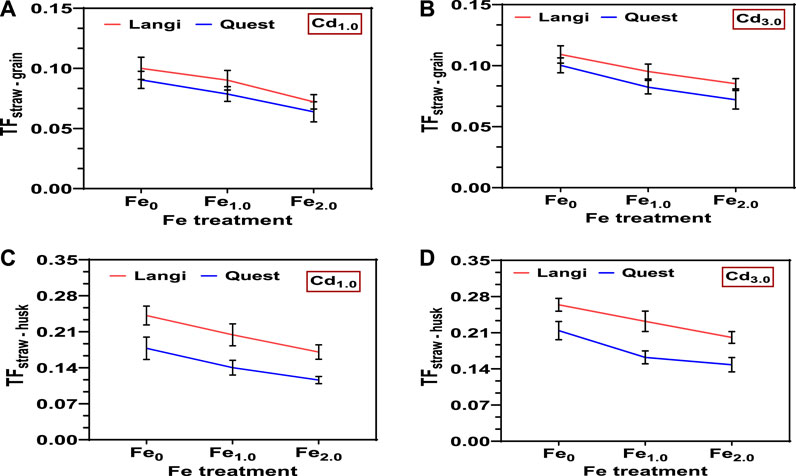
FIGURE 5. Effect of Fe on translocation factors of Cd from straw to grain (A,B) and straw to husk (C,D) between two Cd levels.
Cd Exposure From Rice and Health Risk
The Cd concentration in rice grain was demonstrated in Table 6. The grain Cd concentration ranged from 0.01 mg kg−1 to 1.92 mg kg−1 for all treatment combinations. The rice cultivar cultivated in soil-1 (low pH) had higher grain Cd concentration than soil-2 (high pH). The Cd concentration in two rice cultivars differed significantly (p < 0.001); 0.67 mg k g−1 for the Langi and 0.51 mg kg−1 for the Quest rice cultivar. The rice cultivar treated with Cd1.0 led to the lower grain Cd concentration while Cd3.0 resulted in higher Cd accumulation in rice grain. Fe supplementation significantly reduced the grain Cd accumulation (Table 6). Average daily intake (ADI) was calculated using Cd dataset of two rice cultivars grown in two dissimilar soils and presented in Table 6. In soil-1, the ADI of Cd from the rice grain of the Langi cultivar ranged from 0.84 × 10−3 to 11.83 × 10−3 (mg −1kg−1 bw) (weekly 5.88 × 10−3 to 82.8 × 10−3 (mg−1 kg−1 bw) while for the Quest cultivar, it ranged from 0.56 × 10−3 to 9.42 × 10−3 (mg−1 kg−1 bw) (weekly 3.92 × 10−3 to 65.94 × 10−3 (mg−1 kg−1 bw). For soil-2, the ADI value of the Langi cultivar lies between 0.20 × 10−3 to 3.85 × 10−3 (mg−1 kg−1 bw) (weekly 1.4 × 10−3 to 37.3 × 10−3 (mg−1 kg−1 bw) while for the Quest cultivar, it ranged from 0.08 × 10−3 to 3.85 × 10−3 (mg−1 kg−1 bw) (weekly 0.56 × 10−3 to 26.95 × 10−3 (mg−1 kg−1 bw). The ADI of Cd from rice grain of both rice cultivars was increased with the increasing Cd levels in the soil and the highest value was observed when no Fe was supplied to the soil. Our results also revealed that ADI value was higher for the Langi cultivar compared to the Quest cultivar. Toxicological Profile for Cadmium, Agency for Toxic Substances and Disease Registry (ATSDR) under US Department of Health and Human Services (ATSDR, 2012) and the European Food Safety Authority (EFSA, 2011) set weekly tolerable Cd intake limits of 0.7 and 2.5 × 10−3 mg−1 kg−1 bw, respectively. The joint FAO/WHO Expert Committee on Food Additives (JECFA) recommended the provisional tolerable weekly intake (PTWI) value of Cd as 5.8 × 10−3 mg−1 kg−1 bw, which is equivalent to 1 × 10−3 mg−1 kg−1 bw per day (PTDI) (JECFA, 2010). Table 6 showed that the daily intake of Cd from rice grain was below the provisional tolerable weekly intake (PTDI) when no exogenous Cd was supplied to the soil but much higher than PTDI when additional Cd was supplied to the soil. A recent investigation demonstrated that the average weekly intake of Cd from rice grain was 0.66 × 10−3 to 4.03 × 10−3 mg−1 kg−1bw, which was lower than this study (Shahriar et al., 2020). Similarly, Naseri et al. (2015) found an average weekly intake of Cd in different types of rice were greatly varied and ranged from 3.7 × 10−3 to 5.7 × 10−3 mg−1 kg−1 bw based on 110 g rice consumption per day. The higher value of ADI of Cd in this study possibly indicates the influence of exogenous Cd addition, dissimilar soil type, and variation in Cd uptake between the rice cultivars. In the present investigation, the bioaccessibility of Cd was not considered, which might be another reason for the higher ADI of Cd. The bioaccessibility of Cd in different rice brands varied from 17.4 to 79.7% (Sharafi et al., 2019). Another study showed that the bioaccessibility fraction of Cd ranged from 2.57 to 26.9% from rice consumption (Tefera et al., 2021). So, the average daily intake of Cd will be less if we consider the bioaccessibility fraction of Cd in the rice grain of two rice cultivars. Further investigation on the bioaccessibility fraction of Cd in rice grain should be conducted in order to ensure a more accurate Cd risk estimation.
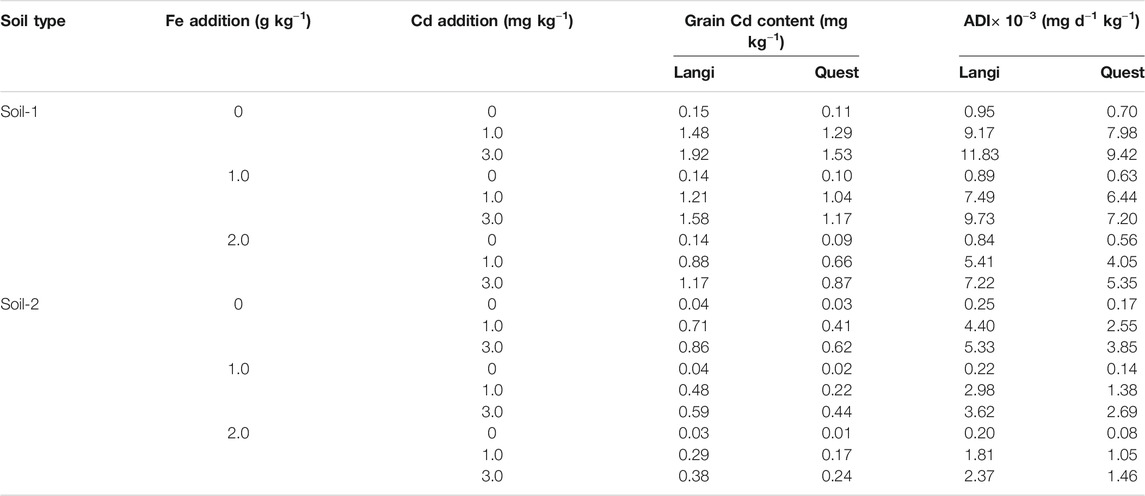
TABLE 6. Concentrations of Cd in grain when soils were treated with Cd and Fe and daily Cd exposure.
In this study, the non-carcinogenic risk (HQ) of Cd ranged from 0.84 to 11.83 for the Langi cultivar and 0.56 to 9.42 for the Quest cultivar in soil-1 while 0.20 to 5.33 for the Langi cultivar and 0.08 to 3.85 for the Quest cultivar in soil-2. The value of HQ was increased with increasing Cd contamination in both soils and decreased with the addition of Fe (Figures 6A,B). For example, the HQ value was 4.57 and 5.81 with Cd1.0 and Cd3.0 treatment, respectively, when 1 g kg−1 Fe was added to the soil while at 2.0 g kg−1 Fe supplementation, the value was 3.08 and 4.10 at Cd1.0 and Cd3.0 treatment, respectively. For both Cd treatments, the HQ values of two rice cultivars were exceeded the safe level (HQ > 1), indicating a risk that existed due to non-carcinogenic effects, which is supported by published reports (Rai et al., 2019; Pirsaheb et al., 2021). The results of this study are in good agreement with Chen et al. (2018a), who reported HQ value from rice consumption was higher than the threshold level and ranged between 2.35 and 4.17. Shahriar et al. (2020) also demonstrated that the HQ values were varied from 0.09 to 0.58 from rice intake in Bangladesh, which were much lower than this present study. According to USEPA, the permissible value of CR is 1.0 × 10−4 while the tolerable limit of CR for regulatory purposes ranged from 1.0 × 10−6 to 1.0 × 10−4 (IARC, 2012). The CR value of Cd exposure was shown in Figure 6 (C and D). It was observed that the CR value at all treatment combinations was higher than the permissible limit of 1.0 × 10−4, indicating a potential carcinogenic significant risk of Cd through consumption of rice. The CR value at Cd treatment without Fe application had the highest risk than with Fe application. The Langi cultivar had a higher potential health risk than the Quest cultivar. The results of this present study are in good agreement with the findings by Shahriar et al. (2020), who showed that the mean CR value of different rice brands was ranged from 2.10 × 10−3 to 8.70 × 10−3. In this study, the results of health risk estimation might be overestimated because the only exposure route of rice consumption was taken into account. The contribution of rice consumption to dietary intake of Cd differed remarkedly depending on gender, age, ingestion rate, and geographical area even within the same study area (Shahriar et al., 2020).
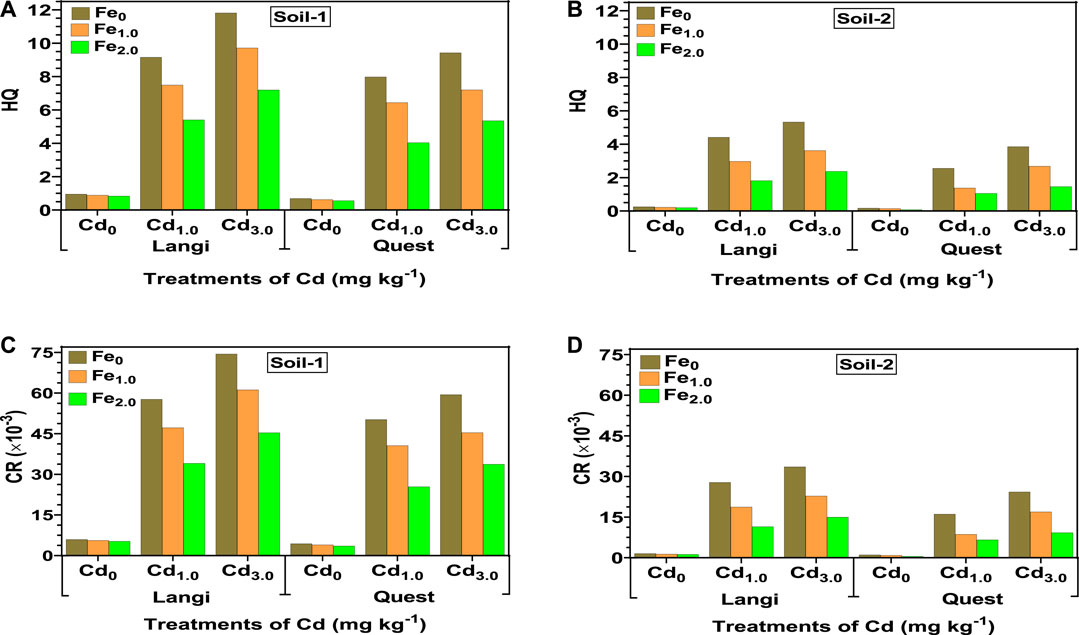
FIGURE 6. Human health risk estimation regarding hazard quotient (A,B) and cancer risk assessment (C,D).
Conclusion
The present study clearly showed that the effect of Cd and Fe addition significantly affected the yield and yield-related components, Cd dynamics in pore water, transfer factors, and associated health risks. Yield and yield-related components were found to be reduced with Cd addition and promoted with Fe application. Transfer factors and pore water Cd concentration were also found to decrease with increased Fe concentration. Differences existed in two rice cultivars in terms of yield and yield-related components, transfer factors, and pore water Cd dynamics. The higher values of HQ (>1) were observed for both Cd treatments indicated adverse health effects to the exposed population from Cd exposure and HQ < 1 was observed for the Cd control with or without Fe application. Based on CR assessment, all treatment combinations in this study pose potential health risks greater than the permissible limit 1.0 × 10−4, but risks were comparatively lower when no Cd was added to the soil. The Langi rice cultivar represented higher non-carcinogenic risks (HQ) and carcinogenic risks (CR) compared to the Quest rice cultivar. Therefore, it is necessary to perform the experiment at the field level to elucidate the molecular mechanism of Cd translocation along with the screening of the rice cultivar that accumulates less Cd. Future studies should also include the bioaccessibility of Cd to estimate human health effects in terms of non-carcinogenic risks (HQ) and carcinogenic risks (CR).
Data Availability Statement
The raw data supporting the conclusion of this article will be made available by the authors without undue reservation.
Author Contributions
ABS: conceptualization, designing and performing the experimentation, writing—original draft, formal analysis, statistical analysis, visualization, and data collection; MMR: project administrator, conceptualization, supervision, formal analysis, and writing—editing and reviewing; MRI: methodology, formal analysis, and writing—editing and reviewing; DM: writing—editing and reviewing; RN: supervision, writing—editing and reviewing.
Conflict of Interest
The authors declare that the research was conducted in the absence of any commercial or financial relationships that could be construed as a potential conflict of interest.
Publisher’s Note
All claims expressed in this article are solely those of the authors and do not necessarily represent those of their affiliated organizations, or those of the publisher, the editors and the reviewers. Any product that may be evaluated in this article, or claim that may be made by its manufacturer, is not guaranteed or endorsed by the publisher.
Acknowledgments
The first author gratefully acknowledges funding from the Australian Government Research Training Program Scholarship at The University of Newcastle, Australia. We also acknowledge the financial support from CRC CARE to conduct this research. We express many thanks to Md Harunur Rashid for providing technical support. We are grateful to Kim Colyvas, Manager of Statistical Consulting Unit, School of Mathematical and Physical Sciences, University of Newcastle, for providing statistical support. The Australian Rice Partnership that includes Sunrice, AgriFutures, and NSW DPI are acknowledged for allowing the rice germplasm to be used in this experiment. NSW DPI provided the rice seed under the MTA that is covered by the Australian Rice Partnership.
References
Abtahi, M., Fakhri, Y., Oliveri Conti, G., Keramati, H., Zandsalimi, Y., Bahmani, Z., et al. (2017). Heavy Metals (As, Cr, Pb, Cd and Ni) Concentrations in Rice (Oryza Sativa) From Iran and Associated Risk Assessment: a Systematic Review. Toxin Rev. 36, 331–341. doi:10.1080/15569543.2017.1354307
Akesson, A., Barregard, L., Bergdahl, I. A., Nordberg, G. F., Nordberg, M., and Skerfving, S. (2014). Non-Renal Effects and the Risk Assessment of Environmental Cadmium Exposure. Environ. Health Perspect. 122, 431–438. doi:10.1289/ehp.1307110
Antoniadis, V., Golia, E. E., Liu, Y.-T., Wang, S.-L., Shaheen, S. M., and Rinklebe, J. (2019). Soil and maize Contamination by Trace Elements and Associated Health Risk Assessment in the Industrial Area of Volos, Greece. Environ. Int. 124, 79–88. doi:10.1016/j.envint.2018.12.053
Ahsan, N., Lee, S.-H., Lee, D.-G., Lee, H., Lee, S. W., Bahk, J. D., et al. (2007). Physiological and Protein Profiles Alternation of Germinating Rice Seedlings Exposed to Acute Cadmium Toxicity. C. R. Biol. 330, 735–746.
Aoshima, K. (2016). Itai-Itai Disease: Renal Tubular Osteomalacia Induced by Environmental Exposure to Cadmium-Historical Review and Perspectives. Soil Sci. Plant Nutr. 62, 319–326. doi:10.1080/00380768.2016.1159116
Arao, T., and Ae, N. (2003). Genotypic Variations in Cadmium Levels of Rice Grain. Soil Sci. Plant Nutr. 49, 473–479.
ATSDR (2012). Toxicological Profile for Cadmium, Agency for Toxic Substances and Disease Registry (ATSDR) Toxicological Profiles. Atlanta (GA): Agency for Toxic Substances and Disease Registry (US).
Beesley, L., and Dickinson, N. (2009). Carbon and Trace Element Mobility in an Urban Soil Amended With Green Waste Compost. J. Soils Sediments. 10, 215–222. doi:10.1007/s11368-009-0112-y
Cao, Z.-Z., Qin, M.-L., Lin, X.-Y., Zhu, Z.-W., and Chen, M.-X. (2018). Sulfur Supply Reduces Cadmium Uptake and Translocation in rice Grains (Oryza Sativa L.) by Enhancing Iron Plaque Formation, Cadmium Chelation and Vacuolar Sequestration. Environ. Pollut. 238, 76–84. doi:10.1016/j.envpol.2018.02.083
Chen, H.-P., Wang, P., Chang, J.-D., Kopittke, P. M., and Zhao, F.-J. (2021). Producing Cd-Safe Rice Grains in Moderately and Seriously Cd-Contaminated Paddy Soils. Chemosphere 267, 128893. doi:10.1016/j.chemosphere.2020.128893
Chen, H., Yang, X., Wang, P., Wang, Z., Li, M., and Zhao, F.-J. (2018a). Dietary Cadmium Intake From Rice and Vegetables and Potential Health Risk: A Case Study in Xiangtan, Southern China. Sci. Total Environ. 639, 271–277. doi:10.1016/j.scitotenv.2018.05.050
Chen, H., Zhang, W., Yang, X., Wang, P., McGrath, S. P., and Zhao, F.-J. (2018b). Effective Methods to Reduce Cadmium Accumulation in Rice Grain. Chemosphere 207, 699–707. doi:10.1016/j.chemosphere.2018.05.143
Cheng, H., Wang, M., Wong, M. H., and Ye, Z. (2014). Does Radial Oxygen Loss and Iron Plaque Formation on Roots Alter Cd and Pb Uptake and Distribution in Rice Plant Tissues? Plant Soil. 375, 137–148. doi:10.1007/s11104-013-1945-0
Clemens, S., Aarts, M. G. M., Thomine, S., and Verbruggen, N. (2013). Plant Science: the Key to Preventing Slow Cadmium Poisoning. Trends Plant Sci. 18, 92–99. doi:10.1016/j.tplants.2012.08.003
Deng, X., Yang, Y., Zeng, H., Chen, Y., and Zeng, Q. (2020). Variations in Iron Plaque, Root Morphology and Metal Bioavailability Response to Seedling Establishment Methods and Their Impacts on Cd and Pb Accumulation and Translocation in Rice (Oryza Sativa L.). J. Hazard. Mater. 384, 121343. doi:10.1016/j.jhazmat.2019.121343
Duan, G., Shao, G., Tang, Z., Chen, H., Wang, B., Tang, Z., et al. (2017). Genotypic and Environmental Variations in Grain Cadmium and Arsenic Concentrations Among a Panel of High Yielding Rice Cultivars. Rice 10, 9. doi:10.1186/s12284-017-0149-2
EFSA (2011). EFSA Panel on Contaminants in the Food Chain. Statement on Tolerable Weekly Intake for Cadmium. EFSA J. 9, 1975. doi:10.2903/j.efsa.2011.1975
Feng, X., Liu, W., Sehar, S., Zheng, W., Zhang, G., and Wu, F. (2018). Application of Sulfur Fertilizer Reduces Cadmium Accumulation and Toxicity in Tobacco Seedlings (Nicotiana Tabacum). Plant Growth Regul. 85, 165–170. doi:10.1007/s10725-018-0368-6
Gao, X., Flaten, D. N., Tenuta, M., Grimmett, M. G., Gawalko, E. J., and Grant, C. A. (2011). Soil Solution Dynamics and Plant Uptake of Cadmium and Zinc by Durum Wheat Following Phosphate Fertilization. Plant Soil. 338, 423–434. doi:10.1007/s11104-010-0556-2
Grant, C. A., Clarke, J. M., Duguid, S., and Chaney, R. L. (2008). Selection and Breeding of Plant Cultivars to Minimize Cadmium Accumulation. Sci. Total Environ. 390, 301–310. doi:10.1016/j.scitotenv.2007.10.038
Gu, Q., Yang, Z., Yu, T., Yang, Q., Hou, Q., and Zhang, Q. (2018). From Soil to Rice - a Typical Study of Transfer and Bioaccumulation of Heavy Metals in China. Acta Agriculturae Scand. Section B - Soil Plant Sci. 68, 631–642. doi:10.1080/09064710.2018.1455218
Halder, D., Saha, J. K., and Biswas, A. (2020). Accumulation of Essential and Non-essential Trace Elements in rice Grain: Possible Health Impacts on Rice Consumers in West Bengal, India. Sci. Total Environ. 706, 135944. doi:10.1016/j.scitotenv.2019.135944
Halim, M. A., Rahman, M. M., Megharaj, M., and Naidu, R. (2020). Cadmium Immobilization in the Rhizosphere and Plant Cellular Detoxification: Role of Plant-Growth-Promoting Rhizobacteria as a Sustainable Solution. J. Agric. Food Chem. 68, 13497–13529. doi:10.1021/acs.jafc.0c04579
Herath, H., Bandara, D., Weerasinghe, P., Iqbal, M., and Wijayawardhana, H. (2014). Effect of Cadmium on Growth Parameters and Plant Accumulation in Different Rice (Oryza sativa L.) Varieties in Sri Lanka. Trop. Agric. Res. 25, 532–542.
Honma, T., Ohba, H., Kaneko-Kadokura, A., Makino, T., Nakamura, K., and Katou, H. (2016). Optimal Soil EH, PH, and Water Management for Simultaneously Minimizing Arsenic and Cadmium Concentrations in Rice Grains. Environ. Sci. Technol. 50, 4178–4185. doi:10.1021/acs.est.5b05424
Hussain, B., Ashraf, M. N., Shafeeq-ur-Rahman, R., Abbas, A., Li, J., and Farooq, M. (2021a). Cadmium Stress in Paddy Fields: Effects of Soil Conditions and Remediation Strategies. Sci. Total Environ. 754, 142188. doi:10.1016/j.scitotenv.2020.142188
Hussain, B., Umer, M. J., Li, J., Ma, Y., Abbas, Y., Ashraf, M. N., et al. (2021b). Strategies for Reducing Cadmium Accumulation in Rice Grains. J. Clean. Prod. 286, 125557. doi:10.1016/j.jclepro.2020.125557
IARC (2012). Cadmium and Cadmium compounds. International Agency for Reseach on Cancer (IARC) Monograph on the Evaluation of Carcinogenic Risks to Humans. Review of Human Carcinogens: Arsenic, Metals, Fibre and Dusts. 100c.
Ishimaru, Y., Bashir, K., Nakanishi, H., and Nishizawa, N. K. (2012). OsNRAMP5, a Major Player for Constitutive Iron and Manganese Uptake in rice. Plant Signaling Behav. 7, 763–766. doi:10.4161/psb.20510
Islam, S., Rahman, M. M., Islam, M. R., and Naidu, R. (2017). Geographical Variation and Age-Related Dietary Exposure to Arsenic in rice From Bangladesh. Sci. Total Environ. 601-602, 122–131. doi:10.1016/j.scitotenv.2017.05.184
JECFA (2010). Joint FAO/WHO Expert Committee on Food Additives, Seventy-Third Meeting, Geneva, 8-17 June 2010. Summary and Conclusions. JECFA/73/SC. Geneva: Food and Agriculture Organization of the United Nations, WHO.
Jiang, J., Dai, Z., Sun, R., Zhao, Z., Dong, Y., Hong, Z., et al. (2017). Evaluation of Ferrolysis in Arsenate Adsorption on the Paddy Soil Derived from an Oxisol. Chemosphere 179, 232–241. doi:10.1016/j.chemosphere.2017.03.115
Jinadasa, N., Milham, P., Hawkins, C., Cornish, P., William, P., Kaldor, C., et al. (1999). Cadmium Levels in Soils and Vegetables of the Greater Sydney Region. Austrália, Rural Industries Research and Development Corporation.
Kanu, A. S., Ashraf, U., Mo, Z., Fuseini, I., Mansaray, L. R., Duan, M., et al. (2017). Cadmium Uptake and Distribution in Fragrant Rice Genotypes and Related Consequences on Yield and Grain Quality Traits. J. Chem. 2017, 1405878. doi:10.1155/2017/1405878
Khaliq, M. A., James, B., Chen, Y. H., Ahmed Saqib, H. S., Li, H. H., Jayasuriya, P., et al. (2019). Uptake, Translocation, and Accumulation of Cd and its Interaction With Mineral Nutrients (Fe, Zn, Ni, Ca, Mg) in Upland Rice. Chemosphere 215, 916–924. doi:10.1016/j.chemosphere.2018.10.077
Khan, M. A., Khan, S., and Khan, A. (2017). Soil Contamination With Cadmium, Consequences and Remediation Using Organic Amendments. Sci. Total Environ. 601-602, 1591–1605. doi:10.1016/j.scitotenv.2017.06.030
Kusin, F. M., Azani, N. N. M., Hasan, S. N. M. S., and Sulong, N. A. (2018). Distribution of Heavy Metals and Metalloid in Surface Sediments of Heavily-Mined Area for Bauxite Ore in Pengerang, Malaysia and Associated Risk Assessment. Catena 165, 454–464. doi:10.1016/j.catena.2018.02.029
Lee, C.-H., Hsieh, Y.-C., Lin, T.-H., and Lee, D.-Y. (2012). Iron Plaque Formation and its Effect on Arsenic Uptake by Different Genotypes of Paddy rice. Plant Soil. 363, 231–241. doi:10.1007/s11104-012-1308-2
Li, B., Wang, X., Qi, X., Huang, L., and Ye, Z. (2012). Identification of Rice Cultivars With Low Brown Rice Mixed Cadmium and Lead Contents and Their Interactions With the Micronutrients Iron, Zinc, Nickel and Manganese. J. Environ. Sci. 24, 1790–1798.
Li, H., Luo, N., Li, Y. W., Cai, Q. Y., Li, H. Y., Mo, C. H., et al. (2017). Cadmium in Rice: Transport Mechanisms, Influencing Factors, and Minimizing Measures. Environ. Pollut. 224, 622–630. doi:10.1016/j.envpol.2017.01.087
Li, S., Chen, S., Wang, M., Lei, X., Zheng, H., Sun, X., et al. (2020). Iron Fractions Responsible for the Variation of Cd Bioavailability in Paddy Soil Under Variable pe+pH Conditions. Chemosphere 251, 126355. doi:10.1016/j.chemosphere.2020.126355
Li, X.-c., Yang, Z.-z., Zhang, C., Wei, J.-j., Zhang, H.-q., Li, Z.-h., et al. (2019). Effects of Different Crystalline Iron Oxides on Immobilization and Bioavailability of Cd in Contaminated Sediment. Chem. Eng. J. 373, 307–317. doi:10.1016/j.cej.2019.05.015
Liu, H. J., Zhang, J. L., Christie, P., and Zhang, F. S. (2010). Influence of Iron Fertilization on Cadmium Uptake by Rice Seedlings Irrigated with Cadmium Solution. Commun. Soil Sci. Plant Anal. 41, 584–594. doi:10.1080/00103620903531169
Liu, H., Yang, L., Li, N., Zhou, C., Feng, H., Yang, J., et al. (2020). Cadmium Toxicity Reduction in Rice (Oryza Sativa L.) through Iron Addition During Primary Reaction of Photosynthesis. Ecotoxicology Environ. Saf. 200, 110746. doi:10.1016/j.ecoenv.2020.110746
Liu, H., Zhang, C., Wang, J., Zhou, C., Feng, H., Mahajan, M. D., et al. (2017). Influence and Interaction of Iron and Cadmium on Photosynthesis and Antioxidative Enzymes in Two rice Cultivars. Chemosphere 171, 240–247. doi:10.1016/j.chemosphere.2016.12.081
Liu, H., Zhang, J., Christie, P., and Zhang, F. (2008). Influence of Iron Plaque on Uptake and Accumulation of Cd by Rice (Oryza Sativa L.) Seedlings Grown in Soil. Sci. Total Environ. 394, 361–368. doi:10.1016/j.scitotenv.2008.02.004
Liu, J., Cai, G., Qian, M., Wang, D., Xu, J., Yang, J., et al. (2007). Effect of Cd on the Growth, Dry Matter Accumulation and Grain Yield of Different rice Cultivars. J. Sci. Food Agric. 87, 1088–1095. doi:10.1002/jsfa.2816
Liu, X., Tian, G., Jiang, D., Zhang, C., and Kong, L. (2016). Cadmium (Cd) Distribution and Contamination in Chinese Paddy Soils on National Scale. Environ. Sci. Pollut. Res. 23, 17941–17952. doi:10.1007/s11356-016-6968-7
Machiwa, J. F. (2010). Heavy Metal Levels in Paddy Soils and rice (Oryza Sativa (L)) from Wetlands of Lake Victoria Basin, Tanzania. Tanzania J. Sci. 36, 59–72.
Meers, E., Du Laing, G., Unamuno, V., Ruttens, A., Vangronsveld, J., Tack, F. M. G., et al. (2007). Comparison of Cadmium Extractability From Soils by Commonly Used Single Extraction Protocols. Geoderma 141, 247–259. doi:10.1016/j.geoderma.2007.06.002
Meharg, A. A., Norton, G., Deacon, C., Williams, P., Adomako, E. E., Price, A., et al. (2013). Variation in rice Cadmium Related to Human Exposure. Environ. Sci. Technol. 47, 5613–5618. doi:10.1021/es400521h
Naseri, M., Vazirzadeh, A., Kazemi, R., and Zaheri, F. (2015). Concentration of Some Heavy Metals in Rice Types Available in Shiraz Market and Human Health Risk Assessment. Food Chem. 175, 243–248. doi:10.1016/j.foodchem.2014.11.109
Pinto, S. d. S., Souza, A. E. d., Oliva, M. A., and Pereira, E. G. (2016). Oxidative Damage and Photosynthetic Impairment in Tropical Rice Cultivars Upon Exposure to Excess Iron. Sci. Agric. (Piracicaba, Braz.) 73, 217–226. doi:10.1590/0103-9016-2015-0288
Pirsaheb, M., Hadei, M., and Sharafi, K. (2021). Human Health Risk Assessment by Monte Carlo Simulation Method for Heavy Metals of Commonly Consumed Cereals in Iran- Uncertainty and Sensitivity Analysis. J. Food Compost. Anal. 96, 103697. doi:10.1016/j.jfca.2020.103697
Rahman, M. M., Owens, G., and Naidu, R. (2009). Arsenic Levels in rice Grain and Assessment of Daily Dietary Intake of Arsenic From Rice in Arsenic-Contaminated Regions of Bangladesh-Implications to Groundwater Irrigation. Environ. Geochem. Health. 31, 179–187. doi:10.1007/s10653-008-9238-x
Rai, P. K., Lee, S. S., Zhang, M., Tsang, Y. F., and Kim, K.-H. (2019). Heavy Metals in Food Crops: Health Risks, Fate, Mechanisms, and Management. Environ. Int. 125, 365–385. doi:10.1016/j.envint.2019.01.067
Rajkumar, M., Sandhya, S., Prasad, M. N. V., and Freitas, H. (2012). Perspectives of Plant-Associated Microbes in Heavy Metal Phytoremediation. Biotechnol. Adv. 30, 1562–1574. doi:10.1016/j.biotechadv.2012.04.011
Rizwan, M., Ali, S., Abbas, T., Adrees, M., Zia-Ur-Rehman, M., Ibrahim, M., et al. (2017). Residual Effects of Biochar on Growth, Photosynthesis and Cadmium Uptake in Rice (Oryza Sativa L.) under Cd Stress With Different Water Conditions. J. Environ. Manage. 206, 676–683. doi:10.1016/j.jenvman.2017.10.035
Rizwan, M., Ali, S., Adrees, M., Rizvi, H., Zia-Ur-Rehman, M., Hannan, F., et al. (2016). Cadmium Stress in Rice: Toxic Effects, Tolerance Mechanisms, and Management: a Critical Review. Environ. Sci. Pollut. Res. 23, 17859–17879. doi:10.1007/s11356-016-6436-4
Roberts, T. L. (2014). Cadmium and Phosphorous Fertilizers: The Issues and the Science. Proced. Eng. 83, 52–59. doi:10.1016/j.proeng.2014.09.012
Satpathy, D., Reddy, M. V., and Dhal, S. P. (2014). Risk Assessment of Heavy Metals Contamination in Paddy Soil, Plants, and Grains (Oryza Sativa L.) at the East Coast of India. Biomed. Res. Int. 2014, 545473. doi:10.1155/2014/545473
Sebastian, A., and Prasad, M. N. V. (2016). Iron Plaque Decreases Cadmium Accumulation inOryza sativaL. And Serves as a Source of Iron. Plant Biol. J. 18, 1008–1015. doi:10.1111/plb.12484
Shahriar, S., Rahman, M. M., and Naidu, R. (2020). Geographical Variation of Cadmium in Commercial Rice Brands in Bangladesh: Human Health Risk Assessment. Sci. Total Environ. 716, 137049. doi:10.1016/j.scitotenv.2020.137049
Shao, G., Chen, M., Wang, D., Xu, C., Mou, R., Cao, Z., et al. (2008). Using Iron Fertilizer to Control Cd Accumulation in Rice Plants: A New Promising Technology. Sci. China Ser. C-life Sci. 51, 245–253. doi:10.1007/s11427-008-0031-y
Sharafi, K., Nodehi, R. N., Mahvi, A. H., Pirsaheb, M., Nazmara, S., Mahmoudi, B., et al. (2019). Bioaccessibility Analysis of Toxic Metals in Consumed Rice Through an In Vitro Human Digestion Model - Comparison of Calculated Human Health Risk From Raw, Cooked and Digested Rice. Food Chem. 299, 125126. doi:10.1016/j.foodchem.2019.125126
Siddique, A. B., Rahman, M. M., Islam, M. R., and Naidu, R. (2021). Varietal Variation and Formation of Iron Plaques on Cadmium Accumulation in Rice Seedling. Environ. Adv. 5, 100075. doi:10.1016/j.envadv.2021.100075
Song, W.-e., Chen, S.-b., Liu, J.-f., Chen, L., Song, N.-n., Li, N., et al. (2015). Variation of Cd Concentration in Various Rice Cultivars and Derivation of Cadmium Toxicity Thresholds for Paddy Soil by Species-Sensitivity Distribution. J. Integr. Agric. 14, 1845–1854. doi:10.1016/s2095-3119(14)60926-6
Song, Y., Wang, Y., Mao, W., Sui, H., Yong, L., Yang, D., et al. (2017). Dietary Cadmium Exposure Assessment Among the Chinese Population. PLos One. 12, e0177978. doi:10.1371/journal.pone.0177978
Takahashi, R., Ishimaru, Y., Nakanishi, H., and Nishizawa, N. K. (2011). Role of the Iron Transporter OsNRAMP1 in Cadmium Uptake and Accumulation in Rice. Plant Signaling Behav. 6, 1813–1816. doi:10.4161/psb.6.11.17587
Takahashi, R., Ishimaru, Y., Shimo, H., Bashir, K., Senoura, T., Sugimoto, K., et al. (2014). From Laboratory to Field: OsNRAMP5-Knockdown Rice is a Promising Candidate for Cd Phytoremediation in Paddy Fields. Plos One. 9, e98816. doi:10.1371/journal.pone.0098816
Tefera, W., Tang, L., Lu, L., Xie, R., Seifu, W., and Tian, S. (2021). Rice Cultivars Significantly Mitigate Cadmium Accumulation in Grains and its Bioaccessibility and Toxicity in Human HL-7702 Cells. Environ. Pollut. 272, 116020. doi:10.1016/j.envpol.2020.116020
Wan, Y., Camara, A. Y., Yu, Y., Wang, Q., Guo, T., Zhu, L., et al. (2018). Cadmium Dynamics in Soil Pore Water and Uptake by Rice: Influences of Soil-Applied Selenite With Different Water Managements. Environ. Pollut. 240, 523–533. doi:10.1016/j.envpol.2018.04.044
Wang, P., Chen, H., Kopittke, P. M., and Zhao, F.-J. (2019). Cadmium Contamination in Agricultural Soils of China and the Impact on Food Safety. Environ. Pollut. 249, 1038–1048. doi:10.1016/j.envpol.2019.03.063
Wang, Y., Jiang, X., Li, K., Wu, M., Zhang, R., Zhang, L., et al. (2014). Photosynthetic Responses of Oryza sativa L. Seedlings to Cadmium Stress: Physiological, Biochemical and Ultrastructural Analyses. Biometals 27, 389–401.
Wang, Y.-m., Liu, Q., Li, M., Yuan, X.-y., Uchimiya, M., Wang, S.-w., et al. (2021). Rhizospheric Pore-Water Content Predicts the Biochar-Attenuated Accumulation, Translocation, and Toxicity of Cadmium to Lettuce. Ecotoxicology Environ. Saf. 208, 111675. doi:10.1016/j.ecoenv.2020.111675
Wu, M., Wang, P.-Y., Sun, L.-G., Zhang, J.-J., Yu, J., Wang, Y.-W., et al. (2014). Alleviation of Cadmium Toxicity by Cerium in Rice Seedlings is Related to Improved Photosynthesis, Elevated Antioxidant Enzymes and Decreased Oxidative Stress. Plant Growth Regul. 74, 251–260. doi:10.1007/s10725-014-9916-x
Yan, J., Fischel, M., Chen, H., Siebecker, M. G., Wang, P., Zhao, F.-J., et al. (2021). Cadmium Speciation and Release Kinetics in a Paddy Soil as Affected by Soil Amendments and Flooding-Draining Cycle. Environ. Pollut. 268, 115944. doi:10.1016/j.envpol.2020.115944
Ye, X., Ma, Y., and Sun, B. (2012). Influence of Soil Type and Genotype on Cd Bioavailability and Uptake by Rice and Implications for Food Safety. J. Environ. Sci. 24, 1647–1654. doi:10.1016/s1001-0742(11)60982-0
Zhang, Q., Chen, H., Xu, C., Zhu, H., and Zhu, Q. (2019). Heavy Metal Uptake in Rice is Regulated by pH-dependent Iron Plaque Formation and the Expression of the Metal Transporter Genes. Environ. Exp. Bot. 162, 392–398. doi:10.1016/j.envexpbot.2019.03.004
Zhao, F.-J., Ma, Y., Zhu, Y.-G., Tang, Z., and McGrath, S. P. (2015). Soil Contamination in China: Current Status and Mitigation Strategies. Environ. Sci. Technol. 49, 750–759. doi:10.1021/es5047099
Zhao, F.-J., and Wang, P. (2020). Arsenic and Cadmium Accumulation in Rice and Mitigation Strategies. Plant Soil. 446, 1–21. doi:10.1007/s11104-019-04374-6
Zhao, K., Liu, X., Xu, J., and Selim, H. M. (2010). Heavy Metal Contaminations in a Soil-Rice System: Identification of Spatial Dependence in Relation to Soil Properties of Paddy fields. J. Hazard. Mater. 181, 778–787. doi:10.1016/j.jhazmat.2010.05.081
Zhou, H., Zeng, M., Zhou, X., Liao, B.-H., Peng, P.-Q., Hu, M., et al. (2015). Heavy Metal Translocation and Accumulation in Iron Plaques and Plant Tissues for 32 Hybrid rice (Oryza Sativa L.) Cultivars. Plant Soil. 386, 317–329. doi:10.1007/s11104-014-2268-5
Zhou, H., Zhu, W., Yang, W.-T., Gu, J.-F., Gao, Z.-X., Chen, L.-W., et al. (2018). Cadmium Uptake, Accumulation, and Remobilization in Iron Plaque and Rice Tissues at Different Growth Stages. Ecotoxicology Environ. Saf. 152, 91–97. doi:10.1016/j.ecoenv.2018.01.031
Zhu, B., Liao, Q., Zhao, X., Gu, X., and Gu, C. (2018). A Multi-Surface Model to Predict Cd Phytoavailability to Wheat (Triticum aestivum L.). Sci. Total Environ. 630, 1374–1380. doi:10.1016/j.scitotenv.2018.03.002
Keywords: cadmium, iron, rice cultivar, translocation factor, health risk
Citation: Siddique AB, Rahman MM, Islam MR, Mondal D and Naidu R (2021) Response of Iron and Cadmium on Yield and Yield Components of Rice and Translocation in Grain: Health Risk Estimation. Front. Environ. Sci. 9:716770. doi: 10.3389/fenvs.2021.716770
Received: 29 May 2021; Accepted: 12 July 2021;
Published: 17 August 2021.
Edited by:
Zhi Wang, Innovation Academy for Precision Measurement Science and Technology (CAS), ChinaReviewed by:
An Liu, Shenzhen University, ChinaNaga Raju Maddela, Technical University of Manabi, Ecuador
Copyright © 2021 Siddique, Rahman, Islam, Mondal and Naidu. This is an open-access article distributed under the terms of the Creative Commons Attribution License (CC BY). The use, distribution or reproduction in other forums is permitted, provided the original author(s) and the copyright owner(s) are credited and that the original publication in this journal is cited, in accordance with accepted academic practice. No use, distribution or reproduction is permitted which does not comply with these terms.
*Correspondence: Mohammad Mahmudur Rahman, bWFobXVkLnJhaG1hbkBuZXdjYXN0bGUuZWR1LmF1; Ravi Naidu, cmF2aS5uYWlkdUBuZXdjYXN0bGUuZWR1LmF1