- 1Teagasc, Environmental Research Centre, Wexford, Ireland
- 2Teagasc, Crops Research Centre, Carlow, Ireland
- 3UCD Earth Institute, Dublin, Ireland
- 4UCD School of Biology of Environmental Science, Dublin, Ireland
Winter oilseed rape is traditionally established via plough-based soil cultivation and conventional sowing methods. Whilst there is potential to adopt lower cost, and less intensive establishment systems, the impact of these on greenhouse gas emissions have not been evaluated. To address this, field experiments were conducted in 2014/2015 and 2015/2016 to investigate the effects of 1) crop establishment method and 2) sowing method on soil greenhouse gas emissions from a winter oilseed rape crop grown in Ireland. Soil carbon dioxide, nitrous oxide and methane emission measurements were carried out using the static chamber method. Yield (t seed ha−1) and the yield-scaled global warming potential (kg CO2-eq. kg−1 seed) were also determined for each management practice. During crop establishment, conventional tillage induced an initially rapid loss of carbon dioxide (2.34 g C m−2 hr−1) compared to strip tillage (0.94 g C m−2 hr−1) or minimum tillage (0.16 g C m−2 hr−1) (p < 0.05), although this decreased to background values within a few hours. In the crop establishment trial, the cumulative greenhouse gas emissions were, apart from methane, unaffected by tillage management when sown at a conventional (125 mm) or wide (600 mm) row spacing. In the sowing method trial, cumulative carbon dioxide emissions were also 21% higher when plants were sown at 10 seeds m−2 compared to 60 seeds m−2 (p < 0.05). Row spacing width (125 and 750 mm) and variety (conventional and semi-dwarf) were found to have little effect on greenhouse gas emissions and differences in seed yield between the sowing treatments were small. Overall, management practices had no consistent effect on soil greenhouse gas emissions and modifications in seed yield per plant countered differences in planting density.
Introduction
Atmospheric concentrations of the greenhouse gases (GHG) carbon dioxide (CO2), nitrous oxide (N2O) and methane (CH4) continue to rise globally due to anthropogenic activities. Agriculture is responsible for approximately 12% of global GHG emissions with livestock systems, soil cultivation, rice production and crop residue management making the more significant contributions (Ciais et al., 2014). In terms of land use impacts, croplands make one of the major contributions to agricultural GHG emissions through various farming and management activities. Field operations such as soil tillage, sowing, fertilizer addition and chemical treatment that are normally required to maximize plant productivity and yield can have significant impacts on GHG emissions through perturbations in the carbon (C), nitrogen (N) and water dynamics of these ecosystem (Bondeau et al., 2007; Osborne et al., 2010). Mitigation of GHG emissions from agricultural sources involves measures that aim to increase soil organic carbon (SOC) sequestration, reduce GHG emission rates, or both, through improved management practices (Smith et al., 2007; Minasny et al., 2017; Ogle et al., 2019). Cropland management practices that involve the adoption of less intensive soil cultivation and agronomic interventions that improve agricultural productivity may can lead to reductions in GHG emissions and determine whether these ecosystems function as sinks or sources of C (Ceschia et al., 2010).
Soil CO2 is produced by autotrophic and heterotrophic processes. Autotrophic respiration is derived from roots and/or the metabolism of photosynthetic substrates released by roots (Högberg and Read, 2006) whereas heterotrophic respiration is associated with the microbial decomposition of SOC or root exudates (Trumbore, 2000). Agriculture accounts for ∼60% of the global anthropogenic N2O emissions primarily due to increased N-fertilizer use (Smith, 2017), with croplands accounting for at least 80% these emissions (Tian et al., 2019). Nitrous oxide production in soils arise from nitrification; the conversion of ammonium (NH4+) to nitrite (NO2−) and subsequently nitrate (NO3−) in aerobic soils, and denitrification, the sequential reduction of NO3− to gaseous N2O or N2 in anaerobic soils (Butterbach-Bahl et al., 2013). The soil-atmosphere exchange of CH4 is largely governed by the balance between net CH4 production by methanogens and net CH4 oxidation/uptake by methanotrophs (Serrano-Silva et al., 2014). Although upland arable soils are generally aerobic and considered CH4 sinks, intensive soil management practices have reduced the capacity of many soils to oxidise CH4 (Suwanwaree and Robertson, 2005). Land management practices thus often govern whether cropland soils are net sinks or sources of GHGs (Ceschia et al., 2010).
Tillage and sowing are management practices that influence crop establishment, plant growth, nutrient uptake, canopy/soil microclimate and yield (Sharratt and McWilliams, 2005; Malhi et al., 2006; Soane et al., 2012), and subsequently impact on C and N and the GHG balance in croplands (Ceschia et al., 2010; Moors et al., 2010). Conventional tillage (CT) encompasses soil inversion, and crop residue incorporation, facilitating seedbed preparation for the succeeding crop. However, CT practices result in the mechanical disruption of soil aggregates and the release of protected organic C from soil organic matter (SOM) resulting in enhanced CO2 emissions. Altering the turnover rate of SOM can directly impact C sequestration and the emissions of CO2, N2O and CH4 (Six et al., 2004; Abdalla et al., 2013; Abdalla et al., 2016; Shakoor et al., 2021). Agricultural practices that minimize or reduce tillage operations can increase soil aggregate formation potentially retaining ∼30% of crop residues on the soil surface increasing nutrient availability and reducing soil erosion (CTIC, 2004). These practices include minimum tillage (MT), involving shallow cultivation to a depth of 5–10 cm, and strip tillage (ST), combining cultivated strips (25 cm depth) with direct drilling whilst leaving the inter-row spaces unaffected, have been promoted as alternative management practices to CT (Davies and Finney, 2002; Morris et al., 2010) that could reduce GHG emissions and increase C sequestration. In combination with these approaches crop residues may also be retained on the soil surface to protect the C in soil aggregates. The argument being that these non-inversion tillage systems will reduce the exposure and subsequent oxidation of SOC and lower GHG emissions.
In addition to CO2, soil tillage can also affect the emissions of N2O and CH4. Both N2O and CH4 have global warming potentials (GWP) that are 265 and 28 times higher than CO2, respectively (Myhre et al., 2013). In some cases, reductions in CO2 emissions that have been attributed to a particular tillage management may be off-set by increases in the emissions of N2O (Six et al., 2004). The rate of N2O production is controlled by factors that are affected by tillage intensity, such as the soil water-filled pore space (WFPS), soil organic C availability and temperature (Butterbach-Bahl et al., 2013). The reported effects of RT operations on N2O emissions are equivocal, showing either increases (Ball et al., 1999; Shakoor et al., 2021), decreases (Kessavalou et al., 1998; Chatskikh and Olesen, 2007; Ussiri et al., 2009) or similar N2O emissions (Abdalla et al., 2010; O’Neill et al., 2020).
Methane fluxes are regulated by factors such as soil moisture, temperature, oxygen availability, SOM content and C and N availability (Jacinthe and Lal, 2005). Therefore, tillage practices, through their effects on soil physicochemical properties, can influence net CH4 emissions (Hütsch, 1998). Well-aerated soils with higher oxygen availability that facilitate CH4 diffusion to methanotrophic sites tend to have higher rates of CH4 uptake than poorly drained soils that restrict CH4 oxidation (Prajapati and Jacinthe, 2014). Soils may also differ in their capacity to oxidise methane, however, the mechanism(s) involved is not fully understood (Lang et al., 2020).
Oilseed rape (Brassica napus L.; OSR) is the third most important oil crop in the world after oil palm and soybean (FAOSTAT, 2021). The winter OSR variety is predominantly cultivated in temperate climatic regions of Europe, where the mean yield of winter and spring OSR varieties (2.7 t ha−1) consistently exceed the global average (2.1 t ha−1) (FAOSTAT, 2021). In Ireland, WOSR is cultivated as a break crop in cereal production systems on ∼10,000 ha annually (Zahoor et al., 2015; CSO, 2020) and traditionally established via CT sowing operations (Forristal and Murphy, 2010). Compared to CT practices, the crop area under reduced tillage in Ireland is small at 40,000 ha (Meade and Mullins, 2005) although there is increasing interest by growers in the adoption of RT approaches for crop establishment for environmental and economic reasons.
Several studies have quantified GHG emissions from WOSR and/or canola systems. Management practices varied considerably between these experiments spanning 3 decades of research where the soil was cultivated by CT, RT or NT practices or the crops were established using a wide range of row spacing’s and seed rates (Table 1; Walter et al., 2015 and references therein). Another confounding factor in previous studies is that the chambers used for GHG emission measurements were either placed in the inter-row space between plant rows or enclosed both the plant row and inter-row areas. This could complicate an assessment of the impact of different management practices as the presence of plants could directly or indirectly have an impact on GHG emissions. Given the broad range of management practices used it is also difficult to generalize about the reason(s) for any observed differences in GHG emissions.
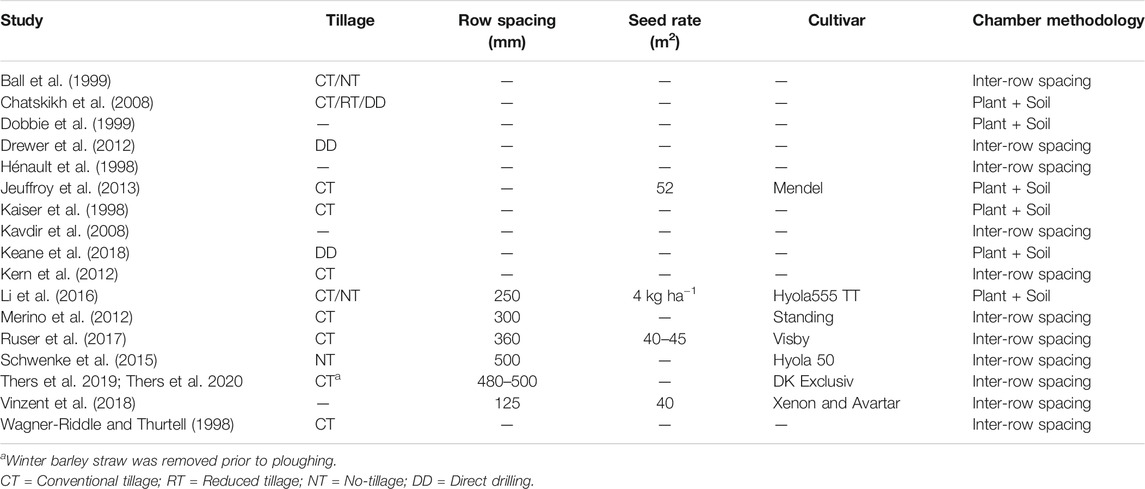
TABLE 1. Management practices and GHG emission measurement methodologies as described in previous studies of OSR.
Other agronomic management practices, such as row spacing and seed rate, are often optimized by growers to encourage rapid plant emergence, canopy closure and development from sowing to harvest. Crop row spacing and plant density influence canopy architecture, which affects solar radiation interception, and the utilization of water and nutrients. Increasing row width and/or reducing the seed rate may expose surface soils to increases in solar radiation, precipitation and wind, resulting in microclimate-related modifications in soil temperature and moisture that influence GHG emissions.
Although there are some studies that have examined the link between WOSR yields, tillage practice, row spacing or seed rate (Christian and Bacon, 1990; Vann et al., 2016) the link between cultivation practices, GHG emissions and yield has not yet been examined. The objectives of this study were to examine the effects of: 1) non-inversion tillage and row spacing, 2) row spacing and seed rate, using two WOSR cultivars, on CO2, N2O and CH4 emissions, compared to the conventional management practice typically used on Irish farms (CT; 125 mm row spacing, 60 seeds m−2). Given the importance of agronomic practices that can contribute to GHG mitigation whilst having no yield penalty we also assess the effects on GWP per unit of yield (i.e., the yield-scaled GWP) in different WOSR cultivation systems.
Materials and Methods
Experiment 1: Crop Cultivation
The different cultivation treatments (“Exp. 1”) were established in the Hockey field at Knockbeg (52°51′42″N, 6°56′28″W) around 5 km from the Teagasc Crops Research Facility in 2014/2015 (Figure 1B). The soil at this site is a sandy loam-to-loam texture (Conry, 1987) and before cultivation had been under a permanent pasture for at least 10 years. Since the 1990s the site has been under CT with continuous crop cultivation (van Groenigen et al., 2011).
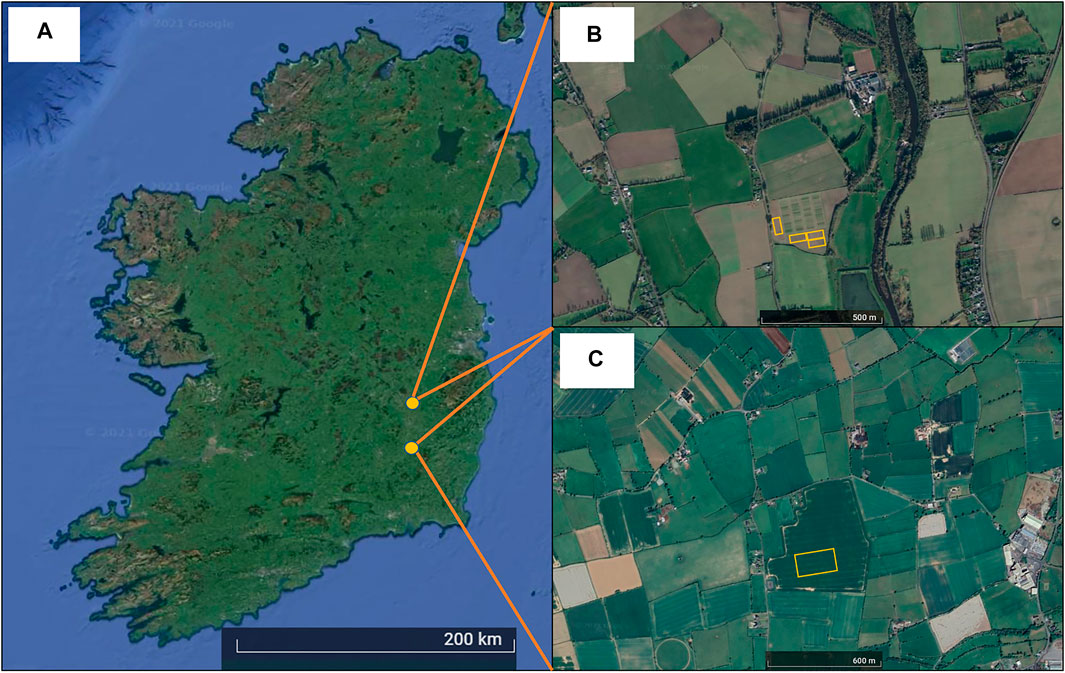
FIGURE 1. Geographical overview of Ireland (A) and the locations of the study sites in (B) Knockbeg, Co. Laois and (C) Goresbridge, Co. Kilkenny, with the surrounding agricultural land uses (Google Earth; created on 31st July 2021). Orange lines indicate the location of the experimental trial plots.
Winter oilseed rape (Brassica napus L. cv. Compass) was cultivated using CT, strip tillage (ST) and MT. The CT treatment consisted of a primary cultivation, soil inversion to a depth of 200–250 mm, with a 5-fin mouldboard plough followed by one pass of a roller to consolidate the soil after ploughing. Secondary cultivation (power harrowing) was performed immediately prior to sowing the seed. The ST treatment is a modified non-inversion tillage technique where strips of soil (200 mm) are cultivated to the conventional plough depth (200–250 mm) whilst the inter-row spacing between plants is left uncultivated with the previous crop residue retained on the soil surface (He-va Sub-Tiller, Denmark). The MT treatment consisted of non-inversion soil cultivation to a depth of 100–125 mm using a stubble cultivator with tines spaced 300 mm apart followed by leveling discs and a cage roller (Horsch, Terrano FX3, United States). A dynamic 3 m cultivator drill (Vaderstad, Rapid 300S, Sweden) capable of working in a range of seedbed types delivered seed through individual hydraulic metering units to a disc coulter.
Plants were sown at a rate of 60 seeds m−2 at two row spacing’s, 125 and 600 mm, giving a total of five treatments as there was no 125 mm spacing for the ST treatment. The treatments (25 m × 5 m) were laid out in a randomised block design with four replications. Calcium ammonium nitrate (CAN) was applied in two split applications of 76 kg N ha−1 (March 16, 2015) and 150 kg N ha−1 (April 1, 2015). Management details are listed in the Supplementary Table S1 of the Supplementary Information. The crop was harvested on the August 8, 2015.
Experiment 2: Sowing Treatments
The sowing treatments (“Exp. 2”) were established in a privately-owned field in Goresbridge, Co. Kilkenny (52°38′8″N, 7°0′43″W) in 2015/2016 (Figure 1C). The soil is a loam texture and has been under continuous wheat cultivation for >10 years. The field was cultivated by deep MT (200–250 mm) (Horsch, Terrano Simba, United States) on the August 22, 2015 and conventional (cv. Compass: C) and semi-dwarf (cv. Troy: T) varieties of WOSR were sown on the August 31, 2015. Plants were sown at four row spacing’s (125, 250, 500 and 750 mm) and four seeding rates (10, 15, 30 and 60 seeds m−2). The experiment was a laid out in a randomised split-plot design with four replications. The main plots (variety) were divided into sub-plots (30 m × 5 m) each containing a combination of different row spacings and seed rates. For logistical reasons, only eight treatments that considered the row spacing/seed rate “extremes” were examined in this study: 1) C125/10, 2) C125/60 (control), 3) C750/10, 4) C750/60, 5) T125/10, 6) T125/60, 7) T750/10 and 8) T750/60. Nitrogen fertiliser (188 kg N ha−1) was supplied in three split applications: 53 kg ha−1 ammonium sulphate nitrate, 80 and 55 kg N ha−1 (both CAN). Fungicide, insecticide and herbicide were applied throughout the season and the crop was harvested on the July 29, 2016. Further management details are listed in Supplementary Table S1 of the Supplementary Information. Soil chemical properties for each site are described in Table 2.
Soil CO2, N2O and CH4 Emission Measurements
Circular stainless-steel collars (225 mm ∅, area = 0.03 m2) with rubber gaskets were inserted into the inter-row spacing (100 mm depth) of all cultivation treatments. Soil CO2 emission measurements were carried out using an infrared gas analyzer (EGM-4, PP Systems, United Kingdom) by slowly sealing an unvented chamber (0.0034 m3) onto a rubber O-ring gasket lining the collar. The enclosure time was 120 s with readings taken every 20 s. After sampling, the chamber was removed, and the CO2 concentration was allowed to equilibrate before placement on the next collar. Gas accumulation was linear within the headspace and measurements with R2 > 0.8 were retained for analysis (Widén and Lindroth, 2003). Additionally, in Exp. 2, four PVC Collars (160 mm ∅) were inserted into bare soil which had plants removed adjacent to the main experimental plots to estimate soil basal/heterotrophic respiration (Rh).
Emissions of N2O and CH4 were measured using the manual closed chamber method (Chadwick et al., 2014). Accumulation of N2O within the chamber headspace, measured at four time points over 1 hour (T0, T20, T40, T60), was determined to be linear on 84% of occasions (O’Neill et al., 2020). The unvented chambers (above) were sealed onto the collars for an enclosure period of 40 min (T40) and sampling carried out between 9.00 and 13.00 h (Barton et al., 2015). The chambers were covered with aluminium foil to prevent solar radiation induced temperature changes inside the headspace during the enclosure period.
For gas sampling, headspace air was withdrawn through a stopcock fitted to the chamber vent using a 20 ml polypropylene syringe (BD Plastipak, Spain). The chamber headspace was mixed by flushing air slowly with the syringe plunger twice prior to the withdrawal of the gas sample. Using a hypodermic needle, samples were immediately transferred into pre-evacuated 7 ml glass exetainers (Sigma-Aldrich, United Kingdom) fitted with double wadded septa (Labco, High Wycombe, United Kingdom). The exetainers were injected with a 12 ml sample to create an overpressure and prevent back diffusion of ambient air during storage. Four ambient air samples were also taken near ground level before and after each sampling occasion to obtain a surrogate time zero (T0) sample for each chamber (Chadwick et al., 2014; Charteris et al., 2020). Sampling frequency was increased during the period of fertilizer application: four times per week for 2 weeks, then twice a week for 2 weeks, then once a week until harvest. Additional samples were taken before or after precipitation events, which are known to stimulate denitrification (Butterbach-Bahl et al., 2013).
Analysis of N2O and CH4 concentrations were carried out with a Gas Chromatograph with a 63Ni electron capture detector (ECD) at 60°C with a flame ionization detector (FID) at 300°C (Bruker Scion 456, Germany). Samples were injected into the GC using a Combi-PAL auto-sampler (CTC Analytics AG, Switzerland). Results were expressed in parts per million by volume (ppmv).
Daily GHG emissions were calculated using the Eq. 1:
where ∆C/∆t is the rate of change of CO2 (To to T2; IRGA) N2O and CH4 concentration (T0 to T40; GC), where ∆C is the change in concentration of the gas in the headspace volume (ppmv or ppbv), ∆t is the enclosure time period (minutes), MW is the molar mass of CO2-C (12 g), N2O-N (28 g) or CH4-C (12 g), P is the atmospheric pressure at the time of sampling (Pa), R is the gas constant (8.314 J mol−1 K−1), T is the air temperature at the time of sampling (K), V is the headspace volume within the chamber (m3) and A is the area covered by the base (m2). Cumulative GHG emissions (±SE) were calculated by trapezoidal integration of the daily means.
The global warming potential (GWP) was calculated for each management practice by converting N2O and CH4 emissions to CO2 equivalent emissions (CO2-eq.) for a 100 years time horizon. The radiative forcing potential used relative to CO2 was 265 for N2O and 28 for CH4 (Myhre et al., 2013). Yield-scaled GWP was calculated by dividing the CO2-equivalent emissions by the seed yield (harvested at 9% moisture) and expressed in units of kg CO2-eq. kg−1. The contribution of CO2 to GWP was excluded based on: 1) the assumption that the soil CO2-C efflux was largely off-set by high rates of net primary productivity (C input) and biomass removal at harvest (C export) (Smith et al., 2007) and 2) the absence of accurate crop residue (straw and root C) data to quantify the annual change in SOC (Mosier et al., 2006).
Soil and Climatic Measurements
Soil mineral N (NH4+ and NO3−) concentrations were determined during the spring-summer growth period. Soil cores (0–10 cm depth) were taken weekly after fertilization for 1 month and every 3–4 weeks thereafter until harvest. Soils were initially stored at 4°C and extracted either on the same day of collection, or within 24 h, using 2 M KCL at a ratio of 5:1 (v:w) water: soil (Maynard et al., 1993). The NH4+ and NO3− concentrations of the extract were analyzed with an Aquakem 600 discrete analyzer (Thermo Fisher Scientific, United States).
Soil volumetric moisture content (%) (GS3, Decagon Devices, United States) and soil temperature (oC; Exp. 2 only) (ELE International, Bedfordshire, United Kingdom) were measured (50–100 mm depth) in the inter-row spacing adjacent to the collars. Water-filled pore space (WFPS) was calculated using Eq. 2:
where Bd is the bulk density measured at the soil surface (70 mm depth) and Pd is particle density estimated at 2.65 g cm−3 (Linn and Doran, 1984).
Climatic measurements of mean air temperature (oC), atmospheric pressure (Pa), rainfall (mm) and soil temperature (oC) were taken from the Met Eireann automated weather station at Oakpark, Co. Carlow, Ireland, which was located 5 km from the Exp. 1 and 30 km from the Exp. 2 sites.
Statistical Analysis
Statistical analysis was conducted using SAS 9.4 (Cary, NY, United States) and R software (R 3.6.1, R Core Team, 2019). Normality and homogeneity of variance were checked using histograms and residual graphs. Where necessary, log or square root transformations (y or y + constant) were applied to data to achieve homogeneity of variance. For Exp. 1, the PROC GLIMMIX procedure in SAS was used to test for differences between the treatments. Tillage (CT, MT, and ST) and row spacing (125 and 600 mm) were the main effects. To account for the missing treatment (i.e., ST125) in the model, additional parameters were created, each with two levels: a “Nested Group” (Factors = CT + MT; Single = ST) and a “Variable Group” (1 = CT125, 2 = all others). The _residual_ option was used in the RANDOM statement. Significant pairwise differences were determined according to the simulate post-hoc test (p < 0.05). For Exp. 2, a linear-mixed effects model (lmer) in the “lme4” (Bates et al., 2012) and “lmerTest” packages (Kuznetsova et al., 2017). Variety (cvs. Compass and Troy), row spacing (125 and 750 mm) and seed rate (10 m−2 and 60 m−2) were set as the main effects with block and block x variety set as the random effects. Model effects were examined using the emmeans function (Lenth, 2019) where ANOVA and pairwise comparisons (normal and back-transformed data) were examined by the Tukey method at a significance level of p < 0.05. Plots were made using the “ggplot2” package in R (Wickham, 2016).
Results
Crop Cultivation
Meteorological data was similar for both sites during the gas measurement periods (Figure 2). The mean air temperatures were 10.9°C (2.9–19.9°C) and 11.0°C (3.6–19.8°C) for Exp. 1 and Exp. 2, respectively. Cumulative rainfall was similar from the period of 27th February to 22nd July each year in Exp. 1 (261 mm) and Exp. 2 (265 mm), representing 28 and 37% of the annual rainfall, respectively. During the growing seasons, the wettest months for Exp. 1 and Exp. 2 were May (90 mm) and April (64 mm) whilst the driest months were April (26 mm) and July (24 mm), respectively.
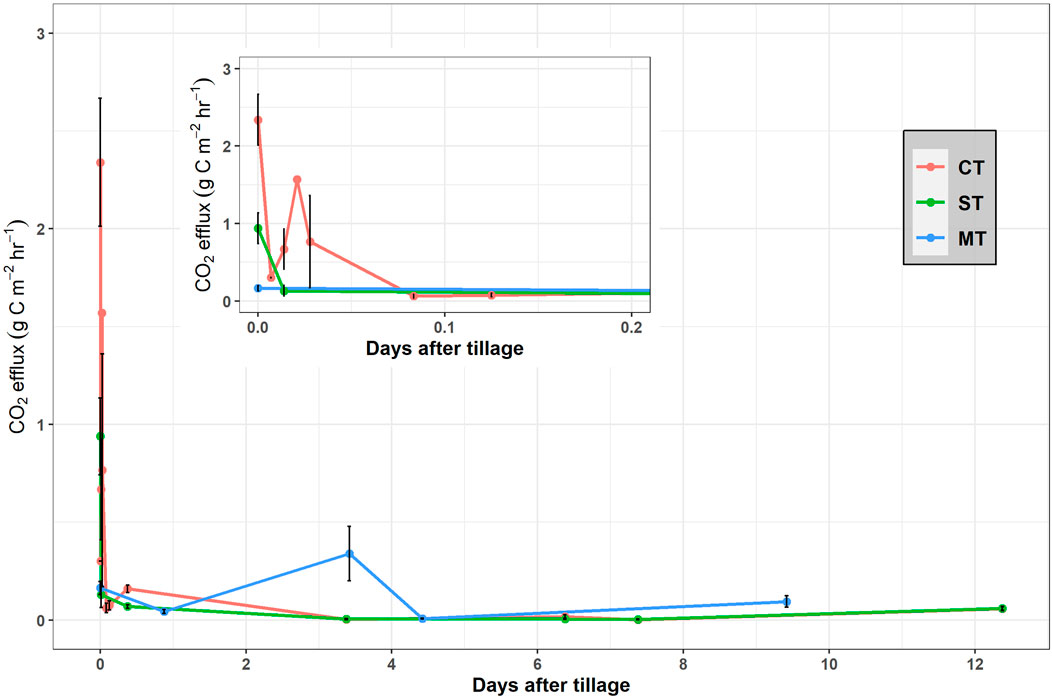
FIGURE 2. Daily air temperature (blue line) and rainfall (grey bars) recorded from the automated weather station at Oakpark covering the 2-year period from sowing in Exp. 1 to harvest in Exp. 2 (August 2014–August 2016). The shaded red areas indicate the gas measurement periods in Exp. 1 (17/03/2015 – 21/07/2015) and Exp. 2 (27/02/2016 – 22/07/2021).
A rapid loss of CO2 was observed after the implementation of CT (maximum recorded value of 2.34 g C m−2 hr−1) at a rate 2.5 times that of ST (maximum recorded value of 0.94 g C m−2 hr−1) and 14.6 times that of MT (maximum recorded value of 0.16 g C m−2 hr−1) (Figure 3). These decreased rapidly to 0.16 g C m−2 hr−1 (CT) and 0.07 g C m−2 hr−1 (ST) after the tillage events and then remained largely stable for the rest of the measurement period. Excluding the anomalously high value found in the MT treatment after 3 days, the daily soil CO2 emissions ranged from 0.04 to 1.38 g C m−2 h−1 across all treatments during the 12 days period.
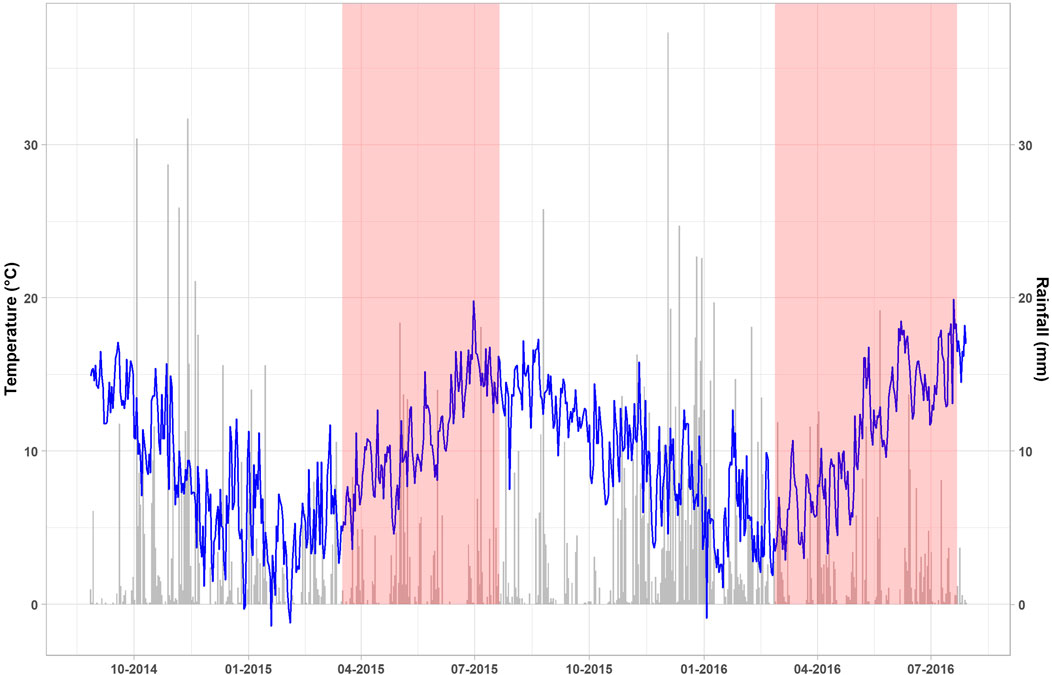
FIGURE 3. Soil CO2 efflux after the initial soil cultivation event for conventional tillage (CT), strip tillage (ST) and minimum tillage (MT) over a 12-day period. The inset plot illustrates the soil CO2 efflux of CT, ST and MT up to 5 h after the initial soil cultivation.
Daily soil CO2 emissions where generally higher during the early part of the growing season and showed two peaks, each occurring around 7 days after the first and second N fertilizer applications, respectively (Figure 4A). Soil CO2 emissions tended to be greater in the CT125 system with maximum daily emissions of 4.0 and 4.2 g C m−2 d−1. The emissions of CO2 converged towards similar values in all systems by mid-April until June, where the mean CO2 loss was 1.16 ± 0.07 g C m−2 d−1. Higher CO2 emissions were generally observed between 32 and 70% WFPS, with a tendency for lower emissions under both drier (25% WFPS) and wetter (85% WFPS) conditions. Cumulative CO2 emissions were not significantly affected by the crop cultivation treatment, with values ranging from 1,083 to 1,683 kg C ha−1, although higher CO2 emissions were generally found in the 125 mm row spacing treatment (Table 3).
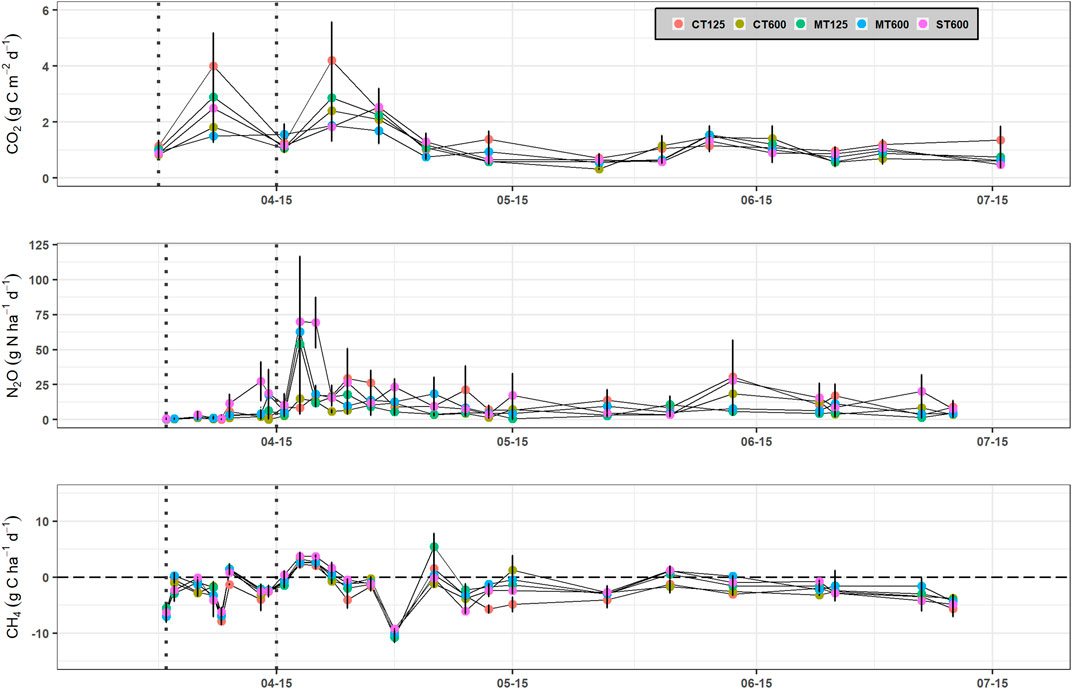
FIGURE 4. Soil CO2, N2O and CH4 emissions for each crop establishment system in Exp. 1: conventional tillage at 125 (CT125) and 600 mm (CT600) row spacing, minimum tillage at 125 (MT125) and 600 mm (MT600) row spacing, and strip tillage at 600 mm row spacing (ST600). Vertical dashed lines indicate fertilizer application and vertical lines on each data point represent the standard error of the means.
Slight increases in N2O were observed in the ST600 system (11.6–27.3 g N ha−1 d−1) ca. 10 days after the first fertilization (Figure 4B). The highest N2O emissions were observed in the MT125 (53.8 g N ha−1 d−1), MT600 (62.8 g N ha−1 d−1) and ST600 (70.0 g N ha−1 d−1) treatments 4 days after the second N application and a second peak occurred in the ST600 (69.3 g N ha−1 d−1) treatment 2 days later. These were observed at 72–79% WFPS when the soil temperature was >10°C (Supplementary Information, Supplementary Figure S1), however, the majority of the N2O emissions occurred across a wide range of WFPS (25–90%) (Figure 4B). Maximum N2O emissions were lower in the CT125 and CT600 treatments, with values of 30.5 and 18.5 g N ha−1 d−1, respectively, occurring 58 days after fertilization. There was no significant effect of tillage or row spacing on the cumulative N2O emissions, with values ranging from 0.81 to 2.05 kg N ha−1 (Table 3).
Daily CH4 emissions displayed similar temporal patterns and ranged from −10.7 to 5.4 g C ha−1 d−1 (Figure 4C). Net CH4 emissions preceded by significant uptake of CH4 were observed after the second N application. With some exceptions, net CH4 uptake was generally sustained until June. For the cumulative CH4 uptake values, a significant tillage x nested effect (p < 0.05) was observed with a 55% increase in uptake in the CT (−0.34 ± 0.03 kg C ha−1) compared to the MT systems (−0.22 ± 0.03 kg C ha−1), with the total CH4 uptake in the ST treatment midway between the CT and MT treatments (Table 3).
Sowing Treatments
Daily GHG emissions for the two WOSR varieties are illustrated in Figure 5. Transient fluctuations in soil CO2 emissions were found during the season, ranging from 0.11 to 3.20 g C m−2 d−1 (Figure 5A), with no clear seasonal trend. Nitrogen fertilizer application tended to initially suppress CO2 emissions, which was followed by increases in CO2 emissions 1–2 weeks later. Soil CO2 emissions increased temporarily in the 125/10 and 750/10 plots in June, however, little variation existed between sowing treatments during the main growing season.
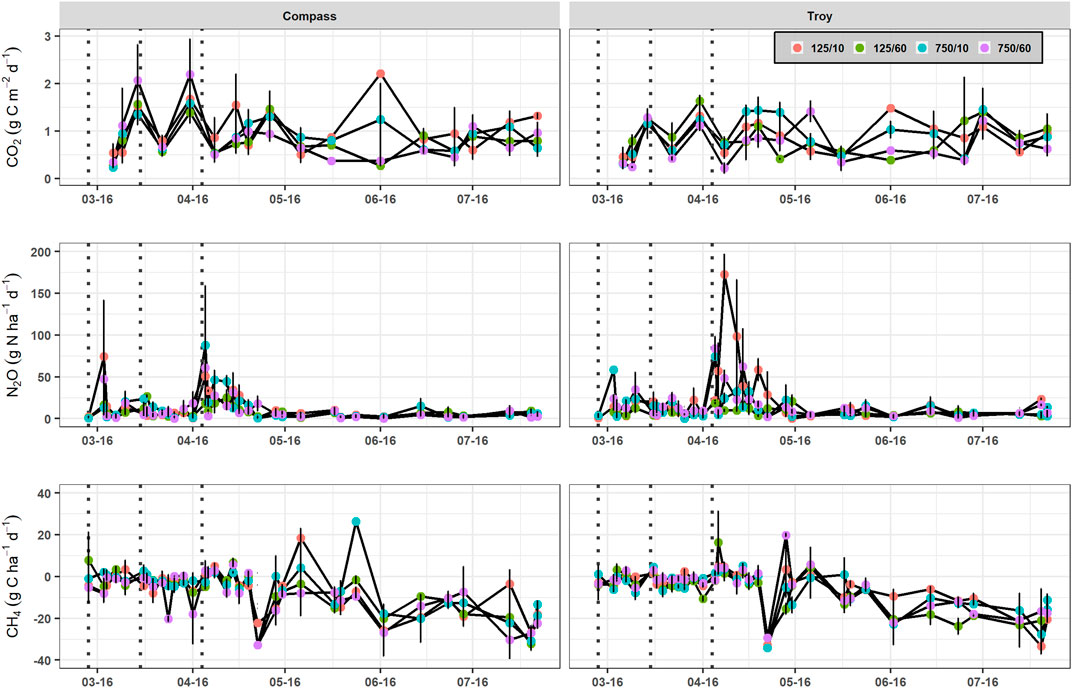
FIGURE 5. Soil CO2, N2O and CH4 emissions for each sowing method treatment in Exp. 2: the conventional (“cv. Compass”) and semi-dwarf (“cv. Troy”) biomass varieties sown at 125 mm or 750 mm row spacing with 10 or 60 seeds m−2. Vertical dashed lines indicate fertilizer application and the vertical lines on each data point represent standard error of the means.
Daily soil N2O emissions were clearly associated with N fertilizer applications (Figure 5B). Higher N2O emissions were found from the cv. Troy plots particularly the 125/10 treatment, and were also observed for soil temperatures >10°C and WFPS values approaching 80% (Supplementary Information, Supplementary Figure S1). Nitrous oxide emissions fell to background rates around 1 month after the final N application.
Daily soil CH4 emissions ranged from −38.2 to 26.3 g C ha−1 d−1 across all sowing treatments (Figure 5C). Low net CH4 uptake rates occurred after mineral N was applied to the soils. The sharp increase in CH4 uptake in April coincided with a decrease in soil NH4+ and NO3− concentrations in late-April (Supplementary Information, Supplementary Figures S2, S3). Several treatments associated with the Compass and Troy cultivars displayed transient net CH4 emission peaks but, in general, there was a consistent trend of increasing net CH4 uptake towards the summer months. The highest net CH4 uptake rates occurred within a narrow temperature range of 13–15°C but a broader range (26–45%) of WFPS. Combining the data from Exp. 1 and Exp. 2, WFPS explained around one quarter of the variance (R2 = 0.29) in the CH4 emissions (Supplementary Information, Supplementary Figure S4). Neither the cumulative N2O nor CH4 emissions were affected by row spacing, seed rate or variety (Table 4).
Yield and Yield-Scaled GWP
Seed yields were unaffected by crop cultivation treatment, canopy management or sowing method (Tables 3, 4). Yields averaged 4.98 t seed ha−1 in Exp. 1 and 4.56 t seed ha−1 in Exp. 2. Yield-scaled GWP values ranged from 0.06 to 0.16 kg CO2-eq. kg−1 in Exp. 1 and 0.05–0.19 kg CO2-eq. kg−1 in Exp. 2. In the latter experiment, the mean yield-scaled GWP of cv. Troy (0.12 kg CO2-eq. kg−1) was double that of the cv. Compass sown plots (0.06 kg CO2-eq. kg−1) (p < 0.05).
Discussion
Crop Management and GHG Emissions
In this study, short-term CO2 emissions after tillage operations were characterized by an initially rapid increase followed by a fast exponential decline (Figure 3). The CT treatment resulted in a maximum CO2 efflux rate ca. 2.5 times that of ST and 14.6 times that of MT which is consistent with an effect of gaseous diffusion due to soil disturbance (Jackson et al., 2003; La Scala et al., 2006; Reicosky and Archer, 2007; Morell et al., 2010). The large loss of CO2 from the MT plot (0.34 g C m−2 hr−1 or 8.15 g C m−2 d−1) ca. 3.5 days after tillage coincided with a rainfall event, a phenomenon often called the “Birch Effect” (Gebremichael et al., 2019). Increased organic matter mineralization after MT in combination with favorable soil moisture and temperature may have led to the relatively larger efflux of CO2 compared to CT and ST (Franzluebbers et al., 1995; Jabro et al., 2008). Overall, however tillage had very little impact on short-term CO2 emissions.
Soil CO2 emissions during spring-summer were unaffected by variations in tillage intensity (Table 3 and Table 4) in line with earlier studies that reported no significant effects of RT systems on CO2 emissions (Kessavalou et al., 1998; Chatskikh et al., 2008; Abdalla et al., 2014). Tillage management can affect soil CO2 emissions through its influence on soil moisture, soil temperature and soil organic C accumulation (Buyanovsky et al., 1986; Hendrix et al., 1988; Franzluebbers et al., 1995; Fortin et al., 1996). In RT systems this is often associated with the retention of crop residues that lower soil temperatures and increase soil moisture content (Chen and McKyes, 1993). However, daily soil temperatures and WFPS values varied little between treatments indicating that the effect of contrasting tillage regimes on residue retention had little effect on CO2 emissions. In this study, the highest soil CO2 emissions occurred during the spring period of the growing season suggesting that phenology, through its effects on root respiration processes and/or the rhizodeposition of labile C, contributed to CO2 production (Rood et al., 1984; Whipps, 1990; Franzluebbers et al., 1995; Rochette and Flanagan, 1997; Jans et al., 2010). In OSR, the highest values for leaf area index were reached close to flowering, which is consistent with the higher soil CO2 emissions observed in March and April across all experiments (except cv. Troy in Exp. 2). Crops allocate, on average, 21% of their photosynthetically fixed C to their roots of which a smaller proportion of this C is released to the soil in root exudates (Pausch and Kuzykayov, 2018). The absence of variation between tillage management may reflect either a low supply of labile C belowground or labile C substrate was not limiting CO2 production.
Tillage management had little impact on N2O emissions in this study (Table 2 and Table 3) in line with earlier work examining CT and RT systems (Abdalla et al., 2010; Liu et al., 2016). Peak N2O emissions that were found in the MT and ST but not in the CT treatments coincided with a higher WFPS (>70%) and higher temperatures (>10°C) as noted in previous studies (Adviento‐Borbe et al., 2007; Abdalla et al., 2010; Žurovec et al., 2017; O’Neill et al., 2020). This indicates that denitrification is the main source of N2O production and the N2O emissions are largely dependent on the extent of soil anaerobiosis. A lower WFPS and an associated increase in soil oxygenation may explain the absence of any impact of fertilization on N2O emissions in the CT systems (Figure 4B).
Cumulative CH4 uptake was ca. 55% greater in the CT compared to the MT treatments (p < 0.05, Table 2). Plaza-Bonilla et al. (2014) also reported a significantly higher cumulative uptake of CH4 in CT (2.69 kg C ha−1) compared to NT (1.16 kg C ha−1) under Mediterranean dryland conditions (p < 0.05). The authors suggested that the greater CH4 oxidation found under CT might be explained by the short duration of NT (3 years) and the possible lack of differences in soil pore structure and methanotrophic communities between CT/NT plots. Reduced tillage practices can however result in a more porous and stable soil structure that facilitates CH4 diffusion (Ball et al., 1997; Hütsch, 1998; Ussiri et al., 2009) and the long-term implementation of RT (>40 years) has been shown to restore the CH4 oxidation capacity of arable soil (Ussiri et al., 2009; Jacinthe et al., 2014). Although the reason(s) for the observed differences between tillage treatments in this study are not clear, it could be explained by both the enhanced diffusion of atmospheric CH4 into the soil and the greater CH4 oxidation rates under CT as the soils became drier in summer.
Soil WFPS had the largest effect on CH4 emissions and explained approximately one quarter of the variability in the daily CH4 emissions (Supplementary Information, Supplementary Figure S4). Higher soil water content inhibits the diffusive transport of CH4 and oxygen to active microbial sites, consequently reducing CH4 uptake in arable soils (Flessa et al., 1995; Drewer et al., 2012). The results of this study are consistent with the recent review by Cowan et al. (2020) who found soil volumetric water content as the strongest predictor of CH4 emissions across agricultural soils in the United Kingdom and Ireland (R2 < 0.1). This indicates that soil water content and its impact on diffusion processes may override the effect of tillage management in regulating CH4 exchange in arable soils.
Row spacing was found to have no significant effect on GHG emissions. In OSR, row spacing influences plant height, branching, leaf area, pod number and the overall canopy architecture. Plants sown in narrow rows reach canopy closure quicker than wide row cropping systems and thus, it was hypothesised that row spacing would influence factors such as root distribution, root C input, water use, N uptake, light interception and soil temperature, and directly or indirectly influence GHG emissions (Sharratt and McWilliams, 2005). Zapata et al. (2021) reported higher CO2 emissions from soybean compared to wheat (wide vs. narrow row spacing) due to prolonged exposure of surface soil to direct sunlight and high intensity rainfall. The absence of a row spacing effect in this study may be due to comparable above-ground vegetative growth rates and rapid canopy closure, irrespective of plant stand structure, thereby limiting the potential microclimatic effects of row spacing on GHG emissions.
Seed rate was a significant factor affecting GHG emissions in Exp. 2 (Table 3). Mean cumulative CO2 emissions (Compass and Troy) were 21% higher in the 10 seeds m−2 compared to the 60 seeds m−2 treatments (p < 0.05). Seed rate determines the plant density and the canopy architecture and, like row spacing, will influence light interception, water and nutrient use and soil microclimate. For OSR crops, Roques and Berry (2016) found that high seed rate plots accelerated flowering and earlier maturation whereas plots with low seed rates remain greener for longer. The longer a plant retains its leaves, or delays leaf senescence, the greater the photosynthetic capacity of the stand and the amount of labile C that is released via root exudates to the rhizosphere. Soil CO2 emissions in the bare soil plots (Rh) also tended to be higher during pod development in June (data not shown). This result points to a parallel increase in the rate of OM decomposition in the cropped soils caused by a reduction in shading and increases in soil temperature in the 10 seeds m−2 treatments. An increase in belowground OM inputs during vegetative growth and/or decomposition of native SOM at maturity may have both contributed to the greater soil CO2 emissions.
Variation in Yield and GWP Across Different Management Practices
Despite the use of a wide range of a management practices (tillage intensity, row spacing, seed rate and variety), seed yield was unaffected (Table 2 and Table 3). These results support earlier studies that found no effect of tillage, row spacing and seed rate on final seed yields (Degenhardt and Kondra, 1981; Christensen and Drabble, 1984; Christian and Bacon, 1990; Bonari et al., 1995; Vann et al., 2016; Wynne et al., 2020).
Although seed yield and yield components are determined by plant density (Leach et al., 1999; Diepenbrock, 2000; Rathke et al., 2006; Kuai et al., 2015), individual OSR plants can counter variations in plant density by increasing yield per plant (Diepenbrock, 2000), so that the seed yield is not compromised by sowing technique.
The two varieties also produced similar seed yields (Table 3). The shorter height of the cv. Troy plants had no detrimental impact on yield components and many of the commercially available semi-dwarf genotypes produce similar yields to conventional varieties (Sieling and Kage, 2008). Miersch et al. (2016) noted that semi-dwarf varieties yielded higher than the conventional types when N is the limiting resource. The non-limiting soil mineral N concentrations in this study (0–10 cm) may have resulted in the similar yields, although semi-dwarf varieties, such as cv Troy, may also perform better in low N input systems compared to conventional varieties. Semi-dwarf varieties may also be favoured by farmers for their higher lodging resistance plus easier harvesting and pesticide applications (Sieling and Kage, 2008).
Yield-scaled GWP values were unaffected by crop management practice. In Exp. 2, cv. Troy had a yield scaled GWP that was double that of cv. Compass (p < 0.05). Cumulative N2O emissions did not significantly differ between treatments, however, the greater GWP was attributed to N-fertilizer applied in excess in the 10 seeds m−2 cv. Troy plots, as indicated by the large N2O emission peaks after the third N application (Figure 5). Sowing at a higher seed rate may circumvent the high GWP of cv. Troy but puts the variety at an inferior position to cv. Compass insofar as low seed rates can achieve similar yields to conventional sowing without adversely affecting GWP.
Our yield-scaled GWP values 0.05–0.16 kg CO2-eq. kg−1 are within the range of those for crops reported in the literature. Linquist et al. (2012) calculated a yield-scaled GWP of 0.166 for wheat and 0.185 kg CO2-eq. kg−1 for maize in their meta-analysis of 57 cropland sites which included rice 0.657 kg CO2-eq. kg−1. Rajaniemi et al. (2011) found slightly higher yield-scaled GWP values for barley, oats, wheat and rye (0.57, 0.57, 0.59 and 0.87 kg CO2-eq. kg−1). Gan et al. (2012) calculated a farm-scale carbon footprint of 0.281–0.317 kg CO2-eq. kg−1 for barley succeeding oilseed rape. These studies, however, included the GWP of additional upstream sources such as fuel emissions, production and use of fertilizers and seed production.
The short measurement campaign (6-months) may pose some limitations for the longer-term interpretation of results. We did not take gas measurements after tillage in Exp. 2 meaning we may have missed any transient emissions of CO2, N2O or CH4, whilst the low temporal frequency of measurements in the winter/spring of Exp. 1 could have introduced large errors in the estimation of the cumulative GHG emissions when determined by linear interpolation. To reduce any bias, only the higher temporal frequency measurements made during spring were considered as representative of yield scaled GWP. Annual GWP values are also required to cover the fallow and post-tillage periods as these can be a large source of GHG emissions from croplands (Linquist et al., 2012). Including all factors leading to CO2 emissions on-farm (tillage, herbicide, pesticide, fertilization) as well as upstream activities (harvest, fertilizer manufacturing, other chemical inputs, transport) (Sainju, 2016) would provide a more complete assessment of the GHG balance of WOSR production systems.
Conclusion
Overall, management practice had a minimal effect on GHG emissions, crop yield and yield scaled GWP. Soil tillage and row spacing had no significant impact on CO2 and N2O emissions. Consequently, the management approach used for WOSR cropping systems can be tailored to the particular conditions and economic factors. The low seeding rate resulted in higher CO2 emissions irrespective of WOSR variety, whilst the yield-scaled GWP of cv. Troy was, on average, higher than that of the cv. Compass. Sowing the semi-dwarf variety at low seeding rates may therefore increase the risk of higher C and/or N emissions from OSR systems. The overall findings indicate that considerable flexibility is possible in the way that OSR crops are established, grown and managed according to local/regional conditions and economics, without compromising yield or GHG emissions. Longer-term, high temporal resolution measurements are required to examine whether the observed GHG and GWP values can be attributed more to management or climatic effects.
Data Availability Statement
The datasets presented in this article are not readily available because The data analyzed in this study is subject to the following licenses/restrictions: confidential. Requests to access the datasets should be directed to Macdara O’Neill, bWFjZGFyYS5vbmVpbGxAdGVhZ2FzYy5pZQ==.
Author Contributions
MO’N, GL, PF, and BO conceived of and designed the experiments. MO performed the experimental work and gaseous emission measurements. MO’N, GL, PF, and BO wrote the manuscript.
Funding
This work was supported through the Teagasc Walsh Fellowship Scheme awarded to MO’N (RMIS 6363).
Conflict of Interest
The authors declare that the research was conducted in the absence of any commercial or financial relationships that could be construed as a potential conflict of interest.
Publisher’s Note
All claims expressed in this article are solely those of the authors and do not necessarily represent those of their affiliated organizations, or those of the publisher, the editors and the reviewers. Any product that may be evaluated in this article, or claim that may be made by its manufacturer, is not guaranteed or endorsed by the publisher.
Acknowledgments
The authors would like to thank the staff at Teagasc Oakpark and Johnstown Castle for their technical support and assistance with the experimental setup, fieldwork and laboratory analysis. We would also like to thank the reviewers for their valuable input to the manuscript.
Supplementary Material
The Supplementary Material for this article can be found online at: https://www.frontiersin.org/articles/10.3389/fenvs.2021.716636/full#supplementary-material
References
Abdalla, K., Chivenge, P., Ciais, P., and Chaplot, V. (2016). No-tillage lessens soil CO2 emissions the most under arid and sandy soil conditions: results from a meta-analysis. Biogeosciences 13 (12), 3619–3633. doi:10.5194/bgd-12-15495-201510.5194/bg-13-3619-2016
Abdalla, M., Hastings, A., Helmy, M., Prescher, A., Osborne, B., Lanigan, G., et al. (2014). Assessing the combined use of reduced tillage and cover crops for mitigating greenhouse gas emissions from arable ecosystem. Geoderma 223-225, 9–20. doi:10.1016/j.geoderma.2014.01.030
Abdalla, M., Jones, M., Ambus, P., and Williams, M. (2010). Emissions of nitrous oxide from Irish arable soils: effects of tillage and reduced N input. Nutr. Cycl. Agroecosyst. 86 (1), 53–65. doi:10.1007/s10705-009-9273-8
Abdalla, M., Osborne, B., Lanigan, G., Forristal, D., Williams, M., Smith, P., et al. (2013). Conservation tillage systems: a review of its consequences for greenhouse gas emissions. Soil Use Manage 29 (2), 199–209. doi:10.1111/sum.12030
Adviento-borbe, M. A. A., Haddix, M. L., Binder, D. L., Walters, D. T., and Dobermann, A. (2007). Soil greenhouse gas fluxes and global warming potential in four high-yielding maize systems. Glob. Change Biol. 13 (9), 1972–1988. doi:10.1111/j.1365-2486.2007.01421.x
Ball, B. C., Dobbie, K. E., Parker, J. P., and Smith, K. A. (1997). The influence of gas transport and porosity on methane oxidation in soils. J. Geophys. Res. 102 (D19), 23301–23308. doi:10.1029/97JD00870
Ball, B. C., Parker, J. P., and Scott, A. (1999). Soil and residue management effects on cropping conditions and nitrous oxide fluxes under controlled traffic in Scotland. Soil Tillage Res. 52 (3-4), 191–201. doi:10.1016/S0167-1987(99)00081-1
Barton, L., Wolf, B., Rowlings, D., Scheer, C., Kiese, R., Grace, P., et al. (2015). Sampling frequency affects estimates of annual nitrous oxide fluxes. Sci. Rep. 5, 15912. doi:10.1038/srep15912
Bates, D., Maechler, M., Bolker, B., Walker, S., Christensen, R. H. B., Singmann, H., and Scheipl, F. (2012). Package ‘lme4’. Vienna, Austria: CRAN. R Foundation for Statistical Computing.
Bonari, E., Mazzoncini, M., and Peruzzi, A. (1995). Effects of conventional and minimum tillage on winter oilseed rape (Brassica napus L.) in a sandy soil. Soil Tillage Res. 33 (2), 91–108. doi:10.1016/0167-1987(94)00440-P
Bondeau, A., Smith, P. C., Zaehle, S., Schaphoff, S., Lucht, W., Cramer, W., et al. (2007). Modelling the role of agriculture for the 20th century global terrestrial carbon balance. Glob. Change Biol. 13 (3), 679–706. doi:10.1111/j.1365-2486.2006.01305.x
Butterbach-Bahl, K., Baggs, E. M., Dannenmann, M., Kiese, R., and Zechmeister-Boltenstern, S. (2013). Nitrous oxide emissions from soils: how well do we understand the processes and their controls? Phil. Trans. R. Soc. B 368 (1621), 20130122. doi:10.1098/rstb.2013.0122
Buyanovsky, G. A., Wagner, G. H., and Gantzer, C. J. (1986). Soil respiration in a winter wheat ecosystem. Soil Sci. Soc. America J. 50 (2), 338–344. doi:10.2136/sssaj1986.03615995005000020017x
Central Statistics Office (CSO) (2020). Area, yield and production of crops 2020. Retrieved from https://www.cso.ie/en/statistics/agriculture/areayieldandproductionofcrops/.
Ceschia, E., Béziat, P., Dejoux, J. F., Aubinet, M., Bernhofer, C., Bodson, B., et al. (2010). Management effects on net ecosystem carbon and GHG budgets at European crop sites. Agric. Ecosyst. Environ. 139 (3), 363–383. doi:10.1016/j.agee.2010.09.020
Chadwick, D. R., Cardenas, L., Misselbrook, T. H., Smith, K. A., Rees, R. M., Watson, C. J., et al. (2014). Optimizing chamber methods for measuring nitrous oxide emissions from plot-based agricultural experiments. Eur. J. Soil Sci. 65 (2), 295–307. doi:10.1111/ejss.12117
Charteris, A. F., Chadwick, D. R., Thorman, R. E., Vallejo, A., Klein, C. A. M., Rochette, P., et al. (2020). Global Research Alliance N 2 O chamber methodology guidelines: Recommendations for deployment and accounting for sources of variability. J. Environ. Qual. 49 (5), 1092–1109. doi:10.1002/jeq2.20126
Chatskikh, D., and Olesen, J. E. (2007). Soil Tillage Enhanced CO2 and N2O Emissions from Loamy Sand Soil Under Spring Barley. Soil Till. Res. 97 (1), 5–18.
Chatskikh, D., Olesen, J. E., Hansen, E. M., Elsgaard, L., and Petersen, B. M. (2008). Effects of reduced tillage on net greenhouse gas fluxes from loamy sand soil under winter crops in Denmark. Agric. Ecosyst. Environ. 128 (1-2), 117–126. doi:10.1016/j.agee.2008.05.010
Chen, Y., and McKyes, E. (1993). Reflectance of light from the soil surface in relation to tillage practices, crop residues and the growth of corn. Soil Tillage Res. 26 (2), 99–114. doi:10.1016/0167-1987(93)90037-p
Christensen, J. V., and Drabble, J. C. (1984). Effect of row spacing and seeding rate on rapeseed yield in northwest Alberta. Can. J. Plant Sci. 64 (4), 1011–1013. doi:10.4141/cjps84-137
Christian, D. G., and Bacon, E. T. G. (1990). A long-term comparison of ploughing, tine cultivation and direct drilling on the growth and yield of winter cereals and oilseed rape on clayey and silty soils. Soil Tillage Res. 18 (4), 311–331. doi:10.1016/0167-1987(90)90117-V
Ciais, P., Sabine, C., Bala, G., Bopp, L., Brovkin, V., Canadell, J., et al. (2014). “Carbon and other biogeochemical cycles,” in Climate change 2013: the physical science basis. Contribution of Working Group I to the Fifth Assessment Report of the Intergovernmental Panel on Climate Change. Editors T. F. Stocker, D. Qin, G.‐ K. Plattner, M. Tignor, S. K. Allen, J. Boschung, A. Nauels, Y. Xia, V. Bex, and P. M. Midgley (Cambridge, UK: Cambridge University Press), 465–570.
Conry, M. J. (1987). Soils of Co. Laois. National Soil Survey of Ireland, Soil Survey Bulletin No. 41. Dublin, Ireland: An Foras Talúntais.
Cowan, N., Maire, J., Krol, D., Cloy, J. M., Hargreaves, P., Murphy, R., et al. (2020). Agricultural soils: A sink or source of methane across the British Isles? Eur. J. Soil Sci. 72, 1842–1862. doi:10.1111/ejss.13075
CTIC (2004). National Survey of Conservation Tillage Practices. West Lafayette: Conservation Technology Information Center., IN. http://www.ctic.purdue.edu/CTIC/CRM.html.
Davies, D. B., and Finney, J. B. (2002). “Reduced cultivations for cereals: research, development and advisory needs under changing economic circumstances,” in Home Grown Cereals Authority Review, 48. Available from: http://cereals.adhb.org.uk/media/288079/rr48.
Degenhardt, D. F., and Kondra, Z. P. (1981). The influence of seeding date and seeding rate on seed yield and yield components of five genotypes of Brassica napus. Can. J. Plant Sci. 61 (2), 175–183. doi:10.4141/cjps81-027
Diepenbrock, W. (2000). Yield analysis of winter oilseed rape (Brassica napus L.): a review. Field Crops Res. 67 (1), 35–49. doi:10.1016/S0378-4290(00)00082-4
Dobbie, K. E., McTaggart, I. P., and Smith, K. A. (1999). Nitrous oxide emissions from intensive agricultural systems: variations between crops and seasons, key driving variables, and mean emission factors. J. Geophys. Res. 104 (D21), 26891–26899. doi:10.1029/1999JD900378
Drewer, J., Finch, J. W., Lloyd, C. R., Baggs, E. M., and Skiba, U. (2012). How do soil emissions of N2O, CH4 and CO2 from perennial bioenergy crops differ from arable annual crops? Glob. Change Biol. Bioenergy 4 (4), 408–419. doi:10.1111/j.1757-1707.2011.01136.x
Flessa, H., Dörsch, P., and Beese, F. (1995). Seasonal variation of N2O and CH4fluxes in differently managed arable soils in southern Germany. J. Geophys. Res. 100 (D11), 23115–23124. doi:10.1029/95JD02270
Food and Agricultural Organization of the United Nations Corporate Statistical Database (FAOSTAT) (2021). Crops. Retrieved from https://www.fao.org/faostat/en/#data/QC.
Forristal, P., and Murphy, K. (2010). Crop establishment systems for winter oilseed rape. Adv. Anim. Biosciences 1 (1), 9. doi:10.1017/s2040470010001524
Fortin, M.-C., Rochette, P., and Pattey, E. (1996). Soil Carbon Dioxide Fluxes from Conventional and No-Tillage Small-Grain Cropping Systems. Soil Sci. Soc. America J. 60 (5), 1541–1547. doi:10.2136/sssaj1996.03615995006000050036x
Franzluebbers, A. J., Hons, F. M., and Zuberer, D. A. (1995). Tillage-induced seasonal changes in soil physical properties affecting soil CO2 evolution under intensive cropping. Soil Tillage Res. 34 (1), 41–60. doi:10.1016/0167-1987(94)00450-S
Gan, Y., Liang, C., Huang, G., Malhi, S. S., Brandt, S. A., and Katepa-Mupondwa, F. (2012). Carbon footprint of canola and mustard is a function of the rate of N fertilizer. Int. J. Life Cycle Assess. 17 (1), 58–68. doi:10.1007/s11367-011-0337-z
Gebremichael, A., Orr, P. J., and Osborne, B. (2019). The impact of wetting intensity on soil CO2 emissions from a coastal grassland ecosystem. Geoderma 343, 86–96. doi:10.1016/j.geoderma.2019.02.016
Hénault, C., Devis, X., Lucas, J. L., and Germon, J. C. (1998). Influence of different agricultural practices (type of crop, form of N-fertilizer) on soil nitrous oxide emissions. Biol. Fertil. Soils 27 (3), 299–306. doi:10.1007/s003740050437
Hendrix, P., Han, C., and Groffman, P. (1988). Soil respiration in conventional and no-tillage agroecosystems under different winter cover crop rotations. Soil Tillage Res. 12 (2), 135–148. doi:10.1016/0167-1987(88)90037-2
Högberg, P., and Read, D. (2006). Towards a more plant physiological perspective on soil ecology. Trends Ecol. Evol. 21 (10), 548–554. doi:10.1016/j.tree.2006.06.004
Hütsch, B. W. (1998). Tillage and land use effects on methane oxidation rates and their vertical profiles in soil. Biol. Fertil. Soils 27 (3), 284–292. doi:10.1007/s003740050435
Jabro, J. D., Sainju, U., Stevens, W. B., and Evans, R. G. (2008). Carbon dioxide flux as affected by tillage and irrigation in soil converted from perennial forages to annual crops. J. Environ. Manag. 88 (4), 1478–1484. doi:10.1016/j.jenvman.2007.07.012
Jacinthe, P.-A., Dick, W. A., Lal, R., Shrestha, R. K., and Bilen, S. (2014). Effects of no-till duration on the methane oxidation capacity of Alfisols. Biol. Fertil. Soils 50 (3), 477–486. doi:10.1007/s00374-013-0866-7
Jacinthe, P.-A., and Lal, R. (2005). Labile carbon and methane uptake as affected by tillage intensity in a Mollisol. Soil Tillage Res. 80 (1-2), 35–45. doi:10.1016/j.still.2004.02.018
Jackson, L. E., Calderon, F. J., Steenwerth, K. L., Scow, K. M., and Rolston, D. E. (2003). Responses of soil microbial processes and community structure to tillage events and implications for soil quality. Geoderma 114 (3-4), 305–317. doi:10.1016/S0016-7061(03)00046-6
Jans, W. W. P., Jacobs, C. M. J., Kruijt, B., Elbers, J. A., Barendse, S., and Moors, E. J. (2010). Carbon exchange of a maize (Zea mays L.) crop: Influence of phenology. Agric. Ecosyst. Environ. 139 (3), 316–324. doi:10.1016/j.agee.2010.06.008
Jeuffroy, M. H., Baranger, E., Carrouée, B., de Chezelles, E., Gosme, M., Hénault, C., et al. (2013). Nitrous oxide emissions from crop rotations including wheat, oilseed rape and dry peas. Biogeosciences 10 (3), 1787–1797. doi:10.5194/bg-10-1787-2013
Kaiser, E.-A., Kohrs, K., Kücke, M., Schnug, E., Heinemeyer, O., and Munch, J. C. (1998). Nitrous oxide release from arable soil: importance of N-fertilization, crops and temporal variation. Soil Biol. Biochem. 30 (12), 1553–1563. doi:10.1016/S0038-0717(98)00036-4
Keane, B. J., Ineson, P., Vallack, H. W., Blei, E., Bentley, M., Howarth, S., et al. (2018). Greenhouse gas emissions from the energy crop oilseed rape (Brassica napus ); the role of photosynthetically active radiation in diurnal N2 O flux variation. GCB Bioenergy 10 (5), 306–319. doi:10.1111/gcbb.12491
Kern, J., Hellebrand, H. J., Gömmel, M., Ammon, C., and Berg, W. (2012). Effects of climatic factors and soil management on the methane flux in soils from annual and perennial energy crops. Biol. Fertil. Soils 48 (1), 1–8. doi:10.1007/s00374-011-0603-z
Kessavalou, A., Mosier, A. R., Doran, J. W., Drijber, R. A., Lyon, D. J., and Heinemeyer, O. (1998). Fluxes of Carbon Dioxide, Nitrous Oxide, and Methane in Grass Sod and Winter Wheat‐Fallow Tillage Management. J. Environ. Qual. 27 (5), 1094–1104. doi:10.2134/jeq1998.00472425002700050015x
Kuai, J., Sun, Y., Zuo, Q., Huang, H., Liao, Q., Wu, C., et al. (2015). The yield of mechanically harvested rapeseed (Brassica napus L.) can be increased by optimum plant density and row spacing. Sci. Rep. 5, 18835. doi:10.1038/srep18835
Kuznetsova, A., Brockhoff, P. B., and Christensen, R. H. (2017). lmerTest package: tests in linear mixed effects models. J. Stat. Softw. 82 (13), 1–26. doi:10.18637/jss.v082.i13
La Scala, N., Bolonhezi, D., and Pereira, G. T. (2006). Short-term soil CO2 emission after conventional and reduced tillage of a no-till sugar cane area in southern Brazil. Soil Tillage Res. 91 (1-2), 244–248. doi:10.1016/j.still.2005.11.012
Lang, R., Goldberg, S. D., Blagodatsky, S., Piepho, H.-P., Hoyt, A. M., Harrison, R. D., et al. (2020). Mechanism of methane uptake in profiles of tropical soils converted from forest to rubber plantations. Soil Biol. Biochem. 145, 107796. doi:10.1016/j.soilbio.2020.107796
Leach, J. E., Stevenson, H. J., Rainbow, A. J., and Mullen, L. A. (1999). Effects of high plant populations on the growth and yield of winter oilseed rape (Brassica napus). J. Agric. Sci. 132 (2), 173–180. doi:10.1017/S0021859698006091
Lenth, R. (2019). emmeans: Estimated Marginal Means, aka Least-Squares Means. R package version 1.3.2. Available online at: https://CRAN.R-project.org/package=emmeans.
Li, G. D., Conyers, M. K., Schwenke, G. D., Hayes, R. C., Liu, D. L., Lowrie, A. J., et al. (2016). Tillage does not increase nitrous oxide emissions under dryland canola (Brassica napus L.) in a semiarid environment of south-eastern Australia. Soil Res. 54 (5), 512–522. doi:10.1071/SR15289
Linn, D. M., and Doran, J. W. (1984). Effect of Water-Filled Pore Space on Carbon Dioxide and Nitrous Oxide Production in Tilled and Nontilled Soils. Soil Sci. Soc. America J. 48 (6), 1267–1272. doi:10.2136/sssaj1984.03615995004800060013x
Linquist, B., Groenigen, K. J., Adviento-Borbe, M. A., Pittelkow, C., and Kessel, C. (2012). An agronomic assessment of greenhouse gas emissions from major cereal crops. Glob. Change Biol. 18 (1), 194–209. doi:10.1111/j.1365-2486.2011.02502.x
Malhi, S. S., Lemke, R., Wang, Z. H., and Chhabra, B. S. (2006). Tillage, nitrogen and crop residue effects on crop yield, nutrient uptake, soil quality, and greenhouse gas emissions. Soil Tillage Res. 90 (1-2), 171–183. doi:10.1016/j.still.2005.09.001
Maynard, D. G., Kalra, Y. P., and Crumbaugh, J. A. (1993). Nitrate and exchangeable ammonium nitrogen. Soil Sampl. Methods Anal. 1, 25–33. doi:10.1080/1065657x.1993.10757875
Meade, C. V., and Mullins, E. D. (2005). “Gm Crop Cultivation in Ireland: Ecological and Economic Considerations,” in Biology and Environment: Proceedings of the Royal Irish Academy (Ireland: Royal Irish Academy), 105, 33–52. doi:10.3318/bioe.2005.105.1.33
Merino, P., Artetxe, A., Castellón, A., Menéndez, S., Aizpurua, A., and Estavillo, J. M. (2012). Warming potential of N2O emissions from rapeseed crop in Northern Spain. Soil Tillage Res. 123, 29–34. doi:10.1016/j.still.2012.03.005
Miersch, S., Gertz, A., Breuer, F., Schierholt, A., and Becker, H. C. (2016). Influence of the Semi-dwarf Growth Type on Seed Yield and Agronomic Parameters at Low and High Nitrogen Fertilization in Winter Oilseed Rape. Crop Sci. 56 (4), 1573–1585. doi:10.2135/cropsci2015.09.0554
Minasny, B., Malone, B. P., McBratney, A. B., Angers, D. A., Arrouays, D., Chambers, A., et al. (2017). Soil carbon 4 per mille. Geoderma 292, 59–86. doi:10.1016/j.geoderma.2017.01.002
Moors, E. J., Jacobs, C., Jans, W., Supit, I., Kutsch, W. L., Bernhofer, C., et al. (2010). Variability in carbon exchange of European croplands. Agric. Ecosyst. Environ. 139 (3), 325–335. doi:10.1016/j.agee.2010.04.013
Morell, F. J., Álvaro-Fuentes, J., Lampurlanés, J., and Cantero-Martínez, C. (2010). Soil CO2 fluxes following tillage and rainfall events in a semiarid Mediterranean agroecosystem: effects of tillage systems and nitrogen fertilization. Agric. Ecosyst. Environ. 139 (1-2), 167–173. doi:10.1016/j.agee.2010.07.015
Morris, N. L., Miller, P. C. H., J.H.Orson, J. H., and Froud-Williams, R. J. (2010). The adoption of non-inversion tillage systems in the United Kingdom and the agronomic impact on soil, crops and the environment-A review. Soil Tillage Res. 108 (1-2), 1–15. doi:10.1016/j.still.2010.03.004
Mosier, A. R., Halvorson, A. D., Reule, C. A., and Liu, X. J. (2006). Net global warming potential and greenhouse gas intensity in irrigated cropping systems in northeastern Colorado. J. Environ. Qual. 35 (4), 1584–1598. doi:10.2134/jeq2005.0232
Myhre, G., Shindell, D., Bréon, F. M., Collins, W., Fuglestvedt, J., Huang, J., and Zhang, H. (2013). “Climate change 2013: the physical science basis,” in Contribution of Working Group I to the Fifth Assessment Report of the Intergovernmental Panel on Climate Change. Editors M. Tignor, S. K. Allen, J. Boschung, A. Nauels, Y. Xia, V. Bex, and P. M. Midgley (Cambridge, United Kingdom and New York, NY, USA: Cambridge University Press).
Ogle, S. M., Alsaker, C., BaldockBernoux, J. M., Bernoux, M., Breidt, F. J., McConkey, B., et al. (2019). Climate and Soil Characteristics Determine where No-Till Management Can Store Carbon in Soils and Mitigate Greenhouse Gas Emissions. Sci. Rep. 9, 11665. doi:10.1038/s41598-019-47861-7
O’Neill, M., Gallego-Lorenzo, L., Lanigan, G. J., Forristal, P. D., and Osborne, B. A. (2020). Assessment of nitrous oxide emission factors for arable and grassland ecosystems. J. Integr. Environ. Sci. 17, 165–185. doi:10.1080/1943815X.2020.1825227
Osborne, B., Saunders, M., Walmsley, D., Jones, M., and Smith, P. (2010). Key questions and uncertainties associated with the assessment of the cropland greenhouse gas balance. Agric. Ecosyst. Environ. 139 (3), 293–301. doi:10.1016/j.agee.2010.05.009
Pausch, J., and Kuzyakov, Y. (2018). Carbon input by roots into the soil: quantification of rhizodeposition from root to ecosystem scale. Glob. Change Biol. 24 (1), 1–12. doi:10.1111/gcb.13850
Plaza-Bonilla, D., Cantero-Martínez, C., Bareche, J., Arrúe, J. L., and Álvaro-Fuentes, J. (2014). Soil Carbon Dioxide and Methane Fluxes as Affected by Tillage and N Fertilization in Dryland Conditions. Plant Soil 381 (1), 111–130.
Prajapati, P., and Jacinthe, P. A. (2014). Methane oxidation kinetics and diffusivity in soils under conventional tillage and long-term no-till. Geoderma 230-231, 161–170. doi:10.1016/j.geoderma.2014.04.013
Pribyl, D. W. (2010). A critical review of the conventional SOC to SOM conversion factor. Geoderma 156 (3-4), 75–83. doi:10.1016/j.geoderma.2010.02.003
Rajaniemi, M., Mikkola, H., and Ahokas, J. (2011). Greenhouse gas emissions from oats, barley, wheat and rye production. Agron. Res. 9, 189–195.
Rathke, G., Behrens, T., and Diepenbrock, W. (2006). Integrated nitrogen management strategies to improve seed yield, oil content and nitrogen efficiency of winter oilseed rape (Brassica napus L.): a review. Agric. Ecosyst. Environ. 117 (2-3), 80–108. doi:10.1016/j.agee.2006.04.006
Reicosky, D. C., and Archer, D. W. (2007). Moldboard plow tillage depth and short-term carbon dioxide release. Soil Tillage Res. 94 (1), 109–121. doi:10.1016/j.still.2006.07.004
Rochette, P., and Flanagan, L. B. (1997). Quantifying Rhizosphere Respiration in a Corn Crop Under Field Conditions. Soil Sci. Soc. Am. J. 61 (2), 466–474.
Rood, S. B., Major, D. J., and Charnetski, W. A. (1984). Seasonal changes in 14CO2 assimilation and 14C translocation in oilseed rape. Field Crops Res. 8, 341–348. doi:10.1016/0378-4290(84)90081-9
Roques, S. E., and Berry, P. M. (2016). The yield response of oilseed rape to plant population density. J. Agric. Sci. 154 (2), 305–320. doi:10.1017/S0021859614001373
Ruser, R., Fuß, R., Andres, M., Hegewald, H., Kesenheimer, K., Köbke, S., et al. (2017). Nitrous oxide emissions from winter oilseed rape cultivation. Agric. Ecosyst. Environ. 249, 57–69. doi:10.1016/j.agee.2017.07.039
Sainju, U. M. (2016). A global meta-analysis on the impact of management practices on net global warming potential and greenhouse gas intensity from cropland soils. PloS one 11 (2), e0148527. doi:10.1371/journal.pone.0148527
Schwenke, G. D., Herridge, D. F., Scheer, C., Rowlings, D. W., Haigh, B. M., and McMullen, K. G. (2015). Soil N2O emissions under N2-fixing legumes and N-fertilised canola: a reappraisal of emissions factor calculations. Agric. Ecosyst. Environ. 202, 232–242. doi:10.1016/j.agee.2015.01.017
Serrano-Silva, N., Sarria-guzmán, Y., Dendooven, L., and Luna-Guido, M. (2014). Methanogenesis and methanotrophy in soil: a review. Pedosphere 24 (3), 291–307. doi:10.1016/S1002-0160(14)60016-3
Shakoor, A., Shahbaz, M., Farooq, T. H., Sahar, N. E., Shahzad, S. M., Altaf, M. M., et al. (2021). A global meta-analysis of greenhouse gases emission and crop yield under no-tillage as compared to conventional tillage. Sci. Total Environ. 750, 142299. doi:10.1016/j.scitotenv.2020.142299
Sharratt, B. S., and McWilliams, D. A. (2005). Microclimatic and Rooting Characteristics of Narrow-Row versus Conventional-Row Corn. Agron. J. 97 (4), 1129–1135. doi:10.2134/agronj2004.0292
Sieling, K., and Kage, H. (2008). The potential of semi-dwarf oilseed rape genotypes to reduce the risk of N leaching. J. Agric. Sci., 146(1), 77–84. doi:10.1017/S0021859607007472
Six, J., Ogle, S. M., Jay Breidt, F., Conant, R. T., Mosier, A. R., and Paustian, K. (2004). The potential to mitigate global warming with no-tillage management is only realized when practised in the long term. Glob. Change Biol. 10 (2), 155–160. doi:10.1111/j.1529-8817.2003.00730.x
Smith, K. A. (2017). Changing views of nitrous oxide emissions from agricultural soil: key controlling processes and assessment at different spatial scales. Eur. J. Soil Sci. 68 (2), 137–155. doi:10.1111/ejss.12409
Smith, P., Martino, Z., and Cai, D. (2007). “Agriculture,” in Climate change 2007: Mitigation. Contribution of Working Group III to the Fourth Assessment Report of the Intergovernmental Panel on Climate Change. Editors B. Netz, O. R. Davidson, P. R. Bosch, R. Dave, and L. A) Meyer (Cambridge, UK and New York, NY, USA: Cambridge University Press), 497–540.
Soane, B. D., Ball, B. C., Arvidsson, J., Basch, G., Moreno, F., and Roger-Estrade, J. (2012). No-till in northern, western and south-western Europe: A review of problems and opportunities for crop production and the environment. Soil Tillage Res. 118, 66–87. doi:10.1016/j.still.2011.10.015
Suwanwaree, P., and Robertson, G. P. (2005). Methane Oxidation in Forest, Successional, and No-till Agricultural Ecosystems. Soil Sci. Soc. Am. J. 69 (6), 1722–1729. doi:10.2136/sssaj2004.0223
Thers, H., Abalos, D., Dörsch, P., and Elsgaard, L. (2020). Nitrous oxide emissions from oilseed rape cultivation were unaffected by flash pyrolysis biochar of different type, rate and field ageing. Sci. Total Environ. 724, 138140. doi:10.1016/j.scitotenv.2020.138140
Thers, H., Petersen, S. O., and Elsgaard, L. (2019). DMPP reduced nitrification, but not annual N2O emissions from mineral fertilizer applied to oilseed rape on a sandy loam soil. GCB Bioenergy 11 (12), 1396–1407. doi:10.1111/gcbb.12642
Tian, H., Yang, J., Xu, R., Lu, C., Canadell, J. G., Davidson, E. A., et al. (2019). Global soil nitrous oxide emissions since the preindustrial era estimated by an ensemble of terrestrial biosphere models: Magnitude, attribution, and uncertainty. Glob. Change Biol. 25 (2), 640–659. doi:10.1111/gcb.14514
Trumbore, S. (2000). Age of soil organic matter and soil respiration: radiocarbon constraints on belowground C dynamics. Ecol. Appl. 10 (2), 399–411. doi:10.1890/1051-0761(2000)010[0399:AOSOMA]2.0.CO;2
Ussiri, D. A. N., Lal, R., and Jarecki, M. K. (2009). Nitrous oxide and methane emissions from long-term tillage under a continuous corn cropping system in Ohio. Soil Tillage Res. 104 (2), 247–255. doi:10.1016/j.still.2009.03.001
van Groenigen, K. J., Hastings, A., Forristal, D., Roth, B., Jones, M., and Smith, P. (2011). Soil C storage as affected by tillage and straw management: An assessment using field measurements and model predictions. Agric. Ecosyst. Environ. 140 (1-2), 218–225. doi:10.1016/j.agee.2010.12.008
Vann, R. A., Reberg-Horton, S. C., and Brinton, C. M. (2016). Row spacing and seeding rate effects on canola population, weed competition, and yield in winter organic canola production. Agron. J. 108 (6), 2425–2432. doi:10.2134/agronj2016.02.0097
Vinzent, B., Fuss, R., Maidl, F.-X., and Hülsbergen, K.-J. (2018). N 2 O emissions and nitrogen dynamics of winter rapeseed fertilized with different N forms and a nitrification inhibitor. Agric. Ecosyst. Environ. 259, 86–97. doi:10.1016/j.agee.2018.02.028
Wagner-Riddle, C., and Thurtell, G. W. (1998). Nitrous oxide emissions from agricultural fields during winter and spring thaw as affected by management practices. Nutrient Cycling in Agroecosystems 52 (2), 151–163. doi:10.1023/A:1009788411566
Walter, K., Don, A., Fuß, R., Kern, J., Drewer, J., and Flessa, H. (2015). Direct Nitrous Oxide Emissions from Oilseed Rape Cropping–A Meta‐Analysis. Gcb Bioener. 7 (6), 1260–1271.
Whipps, J. M. (1990). “Carbon economy,” in The Rhizosphere. Editor J. M. Lynch (Chichester: Wiley), 59–97.
Widén, B., and Lindroth, A. (2003). A Calibration System for Soil Carbon Dioxide-Efflux Measurement Chambers. Soil Sci. Soc. Am. J. 67 (1), 327–334. doi:10.2136/sssaj2003.3270
Wynne, K., Neely, C. B., Adams, C., Kimura, E., DeLaune, P. B., Hathcoat, D., et al. (2020). Testing row spacing and planting rate for fall‐planted spring canola in the southern United States. Agron.j. 112 (3), 1952–1962. doi:10.1002/agj2.20201
Zapata, D., Rajan, N., Mowrer, J., Casey, K., Schnell, R., and Hons, F. (2021). Long-term tillage effect on with-in season variations in soil conditions and respiration from dryland winter wheat and soybean cropping systems. Sci. Rep. 11 (1), 1–10. doi:10.1038/s41598-021-80979-1
Žurovec, O., Sitaula, B. K., Čustović, H., Žurovec, J., and Dörsch, P. (2017). Effects of tillage practice on soil structure, N2O emissions and economics in cereal production under current socio-economic conditions in central Bosnia and Herzegovina. PloS one 12 (11), e0187681. doi:10.1371/journal.pone.0187681
Keywords: crop management, tillage, row spacing, seed rate, variety, GHG (greenhouse gases), oilseed rape
Citation: O’Neill M, Lanigan GJ, Forristal PD and Osborne BA (2021) Greenhouse Gas Emissions and Crop Yields From Winter Oilseed Rape Cropping Systems are Unaffected by Management Practices. Front. Environ. Sci. 9:716636. doi: 10.3389/fenvs.2021.716636
Received: 02 June 2021; Accepted: 19 August 2021;
Published: 15 September 2021.
Edited by:
Amit Kumar, Nanjing University of Information Science and Technology, ChinaReviewed by:
Munesh Kumar, Hemwati Nandan Bahuguna Garhwal University, IndiaWanfa Wang, Tianjin University, China
Copyright © 2021 O’Neill, Lanigan, Forristal and Osborne. This is an open-access article distributed under the terms of the Creative Commons Attribution License (CC BY). The use, distribution or reproduction in other forums is permitted, provided the original author(s) and the copyright owner(s) are credited and that the original publication in this journal is cited, in accordance with accepted academic practice. No use, distribution or reproduction is permitted which does not comply with these terms.
*Correspondence: M. O’Neill, bWFjZGFyYS5vbmVpbGxAdGVhZ2FzYy5pZQ==