- 1Dead Sea and Arava Science Center, Jerusalem, Israel
- 2Ben-Gurion University of the Negev, Eilat Campus, Israel
- 3Deutsche Gesellschaft für Internationale Zusammenarbeit (GIZ), Bonn, Germany
- 4Department of Agriculture and Forest Sciences (DAFNE), University of Tuscia, Viterbo, Italy
Soil salinization and sodification are common processes that particularly characterize drylands. These processes can be attributed either to natural conditions or anthropogenic activities. While natural causes include factors such as climate, lithology, topography, and pedology, human causes are mostly related to agricultural land-use, and specifically, to irrigated agriculture. The objective of this study was to thoroughly review this topic, while highlighting the major challenges and related opportunities. Over time, the extent of saline, sodic, and saline-sodic croplands has increased, resulting in accelerated land degradation and desertification, decreased agricultural productivity, and consequently jeopardizing environmental and food security. Mapping and monitoring saline soils is an important management tool, aimed at determining the extent and severity of salinization processes. Recent developments in advanced remote sensing methods have improved the efficacy of mapping and monitoring saline soils. Knowledge on prevention, mitigation, and recovery of soil salinity and sodicity has substantially grown over time. This knowledge includes advanced measures for salt flushing and leaching, water-saving irrigation technologies, precision fertilizer systems, chemical restoration, organic and microbial remediation, and phytoremediation of affected lands. Of a particular interest is the development of forestry-related means, with afforestation, reforestation, agroforestry, and silvopasture practices for the recovery of salt-affected soils. The forecasted expansion of drylands and aggravated drying of existing drylands due to climatic change emphasize the importance of this topic.
Introduction
Salinity and sodicity of soils can be caused by either natural or anthropogenic factors. While natural salinization and sodification processes occur regardless of anthropogenic activities, agriculture, specifically irrigated cropping systems, accelerates these processes without a doubt (Ayers and Westcot, 1985; Ghassemi et al., 1995; Forkutsa et al., 2009; Litalien and Zeeb, 2020). Over time, salinization and sodification of lands put environmental sustainability and agricultural crop productivity at risk (Jamil et al., 2011; Shrivastava and Rajesh, 2015; Singh, 2015; Ivushkin et al., 2019). Soil salinization and sodification processes mostly characterize dryland regions (Brown et al., 1982; Artzy and Hillel, 1988; Ghassemi et al., 1995; Cuevas et al., 2019 (Figure 1). Yet climatic changes, with forecasted increasing temperatures and growing frequency and magnitude of droughts in moister climatic regions, alongside with the aggravated drying and expansion of the world’s drylands (Cook et al., 2014; Huang et al., 2017a), make soil salinization and sodification a global challenge.
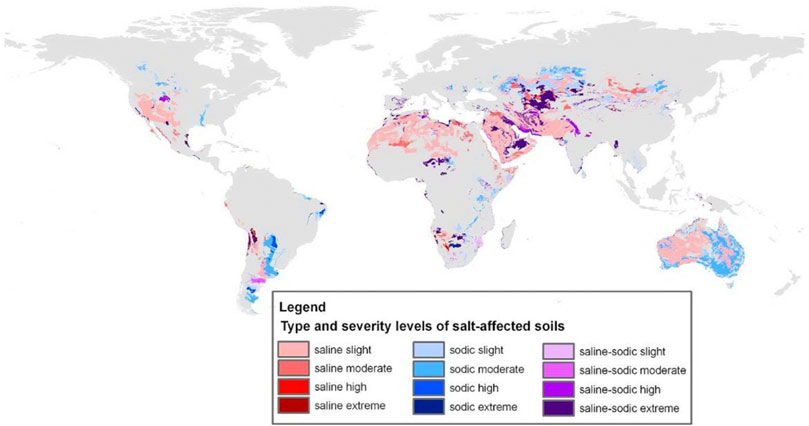
FIGURE 1. Global distribution of saline, sodic, and saline-sodic soils. Source: Encyclopedia of the environment. https://www.encyclopedie-environnement.org/en/zoom/land-salinization/.
Soil salinity refers to the presence of water-soluble salts, including sodium (Na+), potassium (K+), chloride (Cl−), and sulphate (SO42-). Some ions, such as K+ and SO42-, also act as plant nutrients, while Na+ and Cl− are not considered plant nutrients. Therefore, soil salinity often focusses on Na+ and Cl−. Sodicity refers to an excess of Na+ among the exchangeable cations in the soil solution (Qadir et al., 2007). Salinity and sodicity impact plant growth directly through the effects on water uptake by plants, nutrient availability for plants, and by imposing plant toxicity (Ayers and Westcot, 1985; Litalien and Zeeb, 2020), and indirectly, through the deterioration of soil physical conditions (Driessen et al., 2001).
The movement of water from the soil into the roots, through the stem into the leaves, and finally to the atmosphere, is mainly driven by a decreasing water potential (ψ) along this track. When water transpires from a plant’s leaves to the atmosphere, both the plant’s water content and water potential decrease. This results in movement of water through the plant tissues towards the leaves. When water potential in the root cells is lower than that of the soil’s rhizospherous zone, this water is replenished by water that the plant takes from the soil. The presence of water-soluble salts in the soil increases the osmotic potential, and hence, reduces the water potential of the soil, bringing it closer to the water potential in the plant roots. Therefore, water uptake by the roots slows down, causing drought stress for the plant. To some extent, plant roots are able to actively pump water from the soil and transport it further upwards. However, this process is energy-intensive and lowers the growth rate of plants (Ayers and Westcot, 1985).
Plant macro- and micro-nutrients, such as the cations K+, calcium (Ca2+), magnesium (Mg2+) and ammonium (NH4+), and the anions nitrate (NO3−), phosphate (PO43-) and SO42-, are absorbed through carrier proteins, which are located in the outer cell membranes of root epidermal cells. Among these carrier proteins, most are able to channel different cations or anions through the cell membrane. However, a limited number of carrier proteins is specified for one particular ion (Reid and Hayes, 2003). In the less specific carriers, nutrients compete among each other, as well as with other ions, for transport into the root cells. Regarding salinity and sodicity, Na+ competes with other cations, particularly with NH4+ and K+, while Cl− competes with NO3−. In essence, the presence of Na+ decreases the uptake of NH4+ and other cations, while the presence of Cl− decreases the absorption of NO3− from the soil’s exchange complex (Reid and Hayes, 2003).
The Cl− is not absorbed in the soil, and therefore, it moves with the soil-water and is easily absorbed by plants. Within the plant, Cl− is transported with the sapflow to the leaves, where it increases the osmotic potential of the sap and reduces water availability in the leaf tissue for plant metabolism. Symptoms of Cl− toxicity are leaf burn and dry leaf tissue. At the same time, Na+ ions are absorbed by the soil, and therefore, are not absorbed as readily as Cl− ions. Also, Na+ competes with other cations for absorption by plants. Therefore, high concentrations of K+, Ca2+, and Mg2+ reduce Na+ uptake by the plants. However, if Na+ concentration exceeds the plant specific threshold in the leaf tissue, the effects and symptoms of Na+ toxicity are the same as for Cl− toxicity (Ayers and Westcot, 1985).
Plants have several mechanisms to cope with excess Na+ and Cl−, all of which require additional energy, and therefore, adversely impact plant growth (Litalien and Zeeb, 2020). The most common mechanism is the prevention of salt uptake into the plant, by enzymes in the root cell membranes that constantly pump Na+ and Cl− back to the soil (Munns et al., 2019). Another mechanism is the secretion of salts through glands on the leaf. Although the plant absorbs salt, potentially impacting plant metabolism, salt secretion controls its concentration. An additional mechanism is the accumulation of salts in the plants’ vacuoles. This mechanism requires steady activity of enzymes that pump the salts against a concentration gradient in the vacuole (Litalien and Zeeb, 2020).
Soil sodicity, or the soil’s imbalance with regards to Na+ is expressed through the sodium adsorption ratio (SAR: Eq. 1) or the exchangeable sodium percentage (ESP: Eq. 2) (Qadir et al., 2007):
where: c–concentration (mmolc/l); Na–sodium; Ca–calcium; Mg–magnesium.
where: eNa+—exchangeable sodium (mmolc/kg); CEC–cation exchange capacity (mmolc/kg).
Soils with an ESP of 15 and more (which corresponds to SAR ≈13) are considered sodic (Driessen et al., 2001; Qadir et al., 2007). Sodic soils often have a pH higher than 8.5 and up to 11 (Table 1), imposing alkaline conditions that are toxic for plants. These conditions are caused by the presence of bicarbonate (HCO3−), from evaporation of water containing HCO3−, or from biological reduction of SO42- under water-saturated conditions.
Sodic soils contain dispersed clay materials, which clog soil pores so that infiltration and aeration of the root zone is hampered. The processes that cause clay dispersion are explained in full details in Qadir et al. (2007). In essence, divalent cations bind the negatively charged clay compounds stronger than monovalent cations. Cations are bound to the negatively charged clay surfaces, while at the same time and according to a concentration gradient, the cations tend to diffuse into the soil solution. This results in a positively charged cloud of cations around the clay compounds. If these clouds are large, neighboring clay compounds are driven away from each other, resulting in swelling or increased dispersion. Monovalent cations, in particular Na+, build larger positively-charged clouds than divalent cations. Na+ carries a larger hydrate shell than K+, which enhances clay dispersion.
The objective of this study was to thoroughly review the topic and implications of soil salinity and sodicity in drylands. In the following sections, we review selected aspects related to these processes, which are relevant for land managers and policy makers. These aspects include: natural causes (primary salinity or sodicity); anthropogenic causes (secondary salinity or sodicity); mapping techniques of saline lands; and the prevention, mitigation, and restoration of saline, sodic, and saline-sodic soils.
Natural Causes
Primary salinization and sodification are the processes of salt and sodium accumulation due to natural causes, namely mineralogy of the parent material, topography, and water table quality. Both sodium and other ions are products of primary mineral weathering. The weathering process releases soluble cations and anions, of which the most common cations are Ca2+, K+, Mg2+, and Na+, and the most common anions are HCO3−, Cl−, and SO42-. Certain minerals and rocks are more susceptible to chemical weathering than others, and release cations and anions more easily (Sverdrup and Warfvinge, 1988).
In humid climates, cations and anions are generally leached from the soil system and transported by water movement to low-lying landforms or groundwater aquifers (Zinck and Metternicht, 2009). In arid, semi-arid, and sub-humid climates, such cations tend to remain in the soil exchangeable complex, or to precipitate as secondary minerals when the ionic concentration in the solution reaches saturation of a certain salt. The less soluble salts, calcium carbonate (CaCO3), gypsum (CaSO4.2H2O), and magnesite (MgCO3), can easily precipitate under arid and semi-arid conditions. The result is a relative increase in the proportion of Na+ ions in solution, and, consequently, a replacement of some exchangeable Ca2+ and Mg2+ by Na+ on the exchange complex (Bui, 2017). This process increases ESP values, and leads to soil sodification. In arid conditions, where high evaporation rates tend to concentrate the water solution, salts that are more soluble than gypsum may precipitate in the soil. These salts include sodium carbonates (trona, nahcolite, thermonatrite), sodium sulphates (e.g., thenardite), magnesium sulphates (e.g., epsomite), potassium chloride (sylvite), magnesium chlorides (e.g., bischofite), and the most soluble and common salt, sodium chloride (NaCl, halite). Salts that are more soluble than gypsum are called ‘soluble salts’ and encompass the diagnostic minerals that define saline soils (IUSS Working Group WRB, 2015). Soils that have a high concentration of these soluble salts at some time in the year, either at the surface or at a certain depth, are classified as “solonchacks” according to WRB international classification (IUSS Working Group WRB, 2015). The presence of abundant Na-bearing minerals in the parent rocks, namely amphiboles (e.g. glaucophane, riebeckite, pargasite, arfvedsonite), Na-pyroxenes (e.g. augite, aegirina), and Na-plagioclases (e.g. albite, oligoclase, andesine) contribute to the high release of Na+ anions and then to sodification and/or salinization processes (Monteiro et al., 2012). The recharge and throughflow on sloping lands and discharge at lower topographic positions also contribute to land sodification (Fitzpatrick et al., 1994).
Some lithologies release relatively high quantities of cations and anions also in non-dryland regions. For example, sedimentary rocks deposited in lagoon or marine environments may include evaporite strata associated with shales and marls. Weathering of evaporite strata highly increases the content of dissolved salts in the circulant water. Often, such geological formations of evaporites are relatively plastic and tend to form domes through the tectonic process of diapirism. Usually, the formations of such evaporites occur on higher landscape positions, making them a source of salts for the surrounding landscapes (Zinck and Metternicht, 2009).
In drylands, salt-rich groundwater bodies downslope of recharge areas, called “saline seeps” (Brown et al., 1982) (Figure 2), elevate soil salinity enough to inhibit vegetation growth. The United States Department of Agriculture (USDA) describes several types of saline seeps, based on different geological, morphological, and hydrological contexts, including “slope change seep,” “soil texture change seep,” “geologic outcrop seep,” and “hydrostatic pressure seep” (Brown et al., 1982). Alluvial plains and wetlands in arid and semiarid regions are often very sensitive to primary (and secondary) salinization as they accumulate overland water flow due to their low relative elevation. The processes of salinization in these landforms are driven by the dynamics between ground- and surface-water. During dry periods, low-salinity groundwater has beneficial effects because its discharge can replace evaporating surface water, maintaining moderate salinity in the alluvial plain or wetland. On the contrary, when groundwater is saline and the water table level rises because of land-use change or river regulations, the impact on ground surface salinity is detrimental (Jolly et al., 2008). In extreme cases, salt marshes or highly saline discharge playas may form. Yet, where groundwater is absent or very deep, such as in the case of recharge playas, salinization processes are negated. While agricultural land-use is impossible in discharge playas, it can be successful in recharge playas (Stavi et al., 2017).
Another natural source of soil salinity and sodicity in arid and semi-arid regions is linked to volcanic activity, and specifically, to wind-blown ashes rich in sodium-rich minerals such as plagioclases and Na-pyroxenes (Rodríguez-Rodríguez et al., 1993). Secondary volcanic activities, like fumaroles and thermal springs, are sources of chlorine and sulfur components that increase the salinity of groundwater and soils (Di Liberto et al., 2002).
Coastal areas in arid and semi-arid climates are at high risk of primary soil salinization. Salts transported by winds directly from the sea surface through marine spray can be deposited on the inland ground surface. This is a common primary salinity process in oceanic islands, such as Hawaii (Whipkey et al., 2000). Aeolian salt deposition can affect areas several kilometers from the coastline. For example, in the Western Australia Wheatbelt, Pannell (2001) reported on considerable salt deposition (20–200 kg ha−1 year−1) by wind and rainfall at considerable distances from the coastline. Another type of primary salinity in coastal areas subjected to tides is the intrusion of saline water into rivers and groundwater. Specifically, the movement of high salinity backwater from the river delta inland is responsible to this process. The major cause of the backwater effect is the rise in sea level. Therefore, due to global warming, the extent of this process is expected to increase in the future. An example for such processes is evident in the Ganges, Brahmaputra, and the Meghna delta in Bangladesh (Mahmuduzzaman et al., 2014). This issue is relevant to many other large river deltas such as Mississippi, Orinoco, Niger, Indus, Mekong, and Yellow River, all of which have a huge impact on food and water security at local and regional levels (Rahman et al., 2019).
Antropogenic Causes
Secondary soil salinization is attributed either to water logging or to irrigation without proper leaching and drainage. These conditions leave dissolved salts in the soil profile or on the ground surface. Over time, if salts are not washed out, they will accumulate up to levels which impede plant growth (Ayers and Westcot, 1985) (Table 2). Secondary sodification is mostly attributed to the continuous use of sodic water for irrigation, resulting in the formation of dense clay-sodic layers in the sub-surface, which impede hydraulic conductivity of the soil profile (Shahid et al., 2018b).
Already in ancient times, secondary soil salinization affected irrigated agriculture, and resulted in the collapse of civilizations, such as in the case of Mesopotamia (Artzy and Hillel, 1988). Obviously, oases with a history of hundreds or thousands of years of irrigation avoided salinization because salts were washed out of their soils. Most of these oases are located in the forelands of mountains, such as the oases that are dotted around the Tarim Basin in China, or the Ferghana Valley in Central Asia. The oases, located on slightly inclined lands, allow some of the irrigation water to percolate through the soil profile, transporting the salts into the groundwater, which drains into drainage ditches or directly into the river further downstream. This process removes drainage water from the oasis area. The associated salt load is often transported to a terminal lake or wetland downstream of the oasis, forming salt-swamps, salt-lakes, or saltpans, often associated with sodic salts. Where drainage water or groundwater from irrigated lands drains into rivers, the salt concentration in the river water increases downstream. In these events, the use of this water for irrigation in downstream croplands accelerates the risk of soil salinization and sodification (Forkutsa et al., 2009). Salinity thresholds of irrigation water of different qualities are detailed in Table 3.
Large parts of the world’s irrigated lands are located in flat river plains, with very low or no inclination. Under such circumstances, it is difficult to enable drainage that adequately lowers the groundwater level and allows water percolation that sufficiently washes the salts from the soil profile. Under such topographical conditions, salinity is further aggravated by the gradually rising groundwater level. In these cases, capillary rise reaches the soil surface, depositing salts from the groundwater, and aggravating the severity of soil salinization and potential sodification (Forkutsa et al., 2009). Obviously, high volume irrigation methods, such as flood irrigation and furrow irrigation, aggravating the risk of soil salinization due to rising groundwater levels (Burt and Isbell, 2005). Yet, soil salinization may also be caused by water-saving irrigation methods, such as drip or sprinkler irrigation, which must be coupled with regular leaching (Stavi, 2020).
Secondary soil salinization and sodification have become urgent challenges in drylands, being a major driver of land degradation, adversely affecting agricultural production and food security. In the 1990s, 20% of irrigated lands across the world were affected by salinization, with Egypt and Iran having 33 and 29%, respectively, of their irrigated lands being affected. In absolute numbers, the largest areas of salt-affected irrigated lands were in India and China, with 7.0 and 6.7 million ha, corresponding respectively to 17 and 15% of their cropland area (Ghassemi et al., 1995). During the past 30 years, global soil salinization has been increasing, as shown by Ivushkin et al. (2019), who mapped global saline lands through remote sensing. According to this study, the world’s saline land (including both primary and secondary salinization) was 915.5 million ha in 1986 and 1,069.3 million ha in 2016. Other studies showed that globally, 33% of all irrigated lands and 20% of all croplands are affected by salinization (Thenkabail, 2010; Shrivastava and Rajesh, 2015; Singh, 2015). It is expected that by 2050, 50% of the global cropland areas will be affected by salinization of varying degrees (Jamil et al., 2011), with the consequent extending of sodic soils as well (Qadir et al., 2007). A contemporary report on the global status of human-made salt-affected soils will soon be released (http://www.fao.org/global-soil-partnership/areas-of-work/soil-salinity/en/).
Salinization of agricultural land significantly reduces economic benefits, as shown by Welle and Mauter (2017) for California, where salinization reduced overall agricultural revenues by 7.9%. Globally, Wichelns and Qadir (2014) calculated the annual loss of agricultural crop yields to be 27.3 million USD, due to reduced productivity of salinized lands or due to outmigration of local populations from salt-affected regions.
Under secondary salinity conditions, plant nutrient management needs to counteract the nutrient imbalance caused by excess Na+ and Cl−, while not straining the ability of plants to uptake soil-water. In a review study, Hu and Schmidhalter (2005) showed that Na+ competes with other cations, mainly NH4+ and K+, for exchange places in the soil and for carrying molecules that transport nutrients through the plant cell membrane into the root. Therefore, the application of NH4+ and K+ helps to address the plants’ demand for nutrients, and reduces the absorption of Na+. The application of Ca2+ and Mg2+ acts in a similar way, and also helps to preserve the soil structure, as Ca2+ and Mg2+ are more likely to displace Na+ from the exchange places in the soil than K+ or NH4+. With regards to anions, Cl− competes with NO3−. Therefore, the application of NO3− helps satisfy the plants’ demand for N, while reducing the absorption of Cl−. One way or another, fertilizer must be applied carefully, as fertilizers are water-soluble salts themselves, and therefore may increase the osmotic potential of soil, negatively affecting the ability of plants to uptake soil-water. Furthermore, excess Ca2+ may immobilize phosphate (Hu and Schmidhalter, 2005).
Sodification deteriorates the soil structure, resulting in the decrease in infiltration of water and penetration of air into the soil profile (Qadir et al., 2007). This limits the availability of water and oxygen for plants, as well as for the entire soil-food web (European Communities, 2009). In addition, very high Na+ contents may have negative effects on plant nutrition (Alexandre et al., 2018). Therefore, management of sodic lands needs to address these soil physical constraints.
Techniques for Soil Salinity Mapping and Monitoring
Calculating Soil Salinity
To plan effective restoration strategies and combat land degradation in the face of future climate change scenarios, the spatiotemporal distribution and likelihood of reoccurrence of salt-affected soils must be known (Hassani et al., 2020). Visual indications of soil salinization include a white salt crust or salt stains on the ground surface, fluffy soil surface, patchy or lack of seed germination, leaf burn and reduced plant vigor, naturally growing halophytes, worsening of affected areas after rainfalls, and waterlogging (Shahid et al., 2018a). Yet, mapping and monitoring of soil salinity at wide scales is challenging due to the dynamic feature of this property, which is highly variable in space and time (Aldabaa et al., 2015; Corwin and Scudiero, 2019).
Soil salinity is generally measured in laboratory through the analysis of aqueous extracts from disturbed soil samples (ECe), or of saturated soil paste (ECs) (Soil Survey Staff, 2014, Method 4F). Temperature has an effect on EC, because EC increases at approximately 1.9% per centigrade over the range of 15–35°C (Rhoades et al., 1989). Consequently, EC is expressed at a reference temperature of 25°C for purposes of comparison (Corwin and Lesch, 2003). An empiric equation to calculate the amount of soluble salts from electrical conductivity was developed by Marion and Babcock, 1976: (Eq. 3):
where TSS is the total soluble salt concentration (mmol·l−1), and EC is electrical conductivity (mS·cm−1).
Several rapid and inexpensive methods for mapping and monitoring soil salinity have been developed during recent decades. The methods include both proximal and remote sensing technologies.
Proximal Soil Sensing
Proximal soil sensing (PSS) refers to field-based techniques that directly or indirectly measure soil properties, employing one or more sensors close to, or in a direct contact with the soil surface (Viscarra Rossel et al., 2010). The sensors may be hand-held, or pulled by vehicles. PSS can efficiently acquire high frequency soil information, allowing the assessment of soil spatial variability by geostatistical analysis. PSS technologies include contact electrodes designed for electrical resistivity (ER) methods, electromagnetic induction (EMI), mechanical sensors, gamma-ray spectroscopy, vis-NIR diffuse reflectance spectroscopy (VNIR-DRS), and laser induced breakdown spectroscopy (LIBS) (Viscarra Rossel et al., 2011). Among these technologies, the sensors based on soil electrical resistivity (or conductivity), namely ER and EMI sensors, are the most common for mapping soil salinity (Scudiero et al., 2013), texture (Doolittle and Brevik, 2014), moisture (Martini et al., 2017), and depth (Priori et al., 2013). ER techniques involve contact electrodes that directly inject electrical current into the soil, and measure the electrical potential drop due to the electrical resistivity of a determined volume of soil. ER measurements require at least four electrodes, two for injecting the current into the soil (current electrodes), and two for measuring the resulting potential difference (potential electrodes). Available ER apparatus usually have other electrodes that measure the potential differences at different depths. Both the penetration depth of the electrical current and the volume of the measured soil grow as the spacing between inter-electrodes increases (Samouelian et al., 2005).
Electrical conductivity of the bulk soil, also called apparent electrical conductivity (ECa), is the inverse of ER and can be measured by EMI without direct contact with the soil (Sudduth et al., 2003; Doolittle and Brevik, 2014). A transmitter coil located at one end of the EMI sensor produces an electromagnetic field, which induces circular eddy current loops in the soil; the magnitude of the loops is directly proportional to ECa. Such current loops produce a secondary electromagnetic field that is intercepted by the receiver coils of the instrument (Corwin and Yemoto, 2020). The differences in amplitude and phase between the secondary and the primary electromagnetic fields are related to the soil’s properties, as well as to the coils’ spacing and orientation, EM field frequency, and distance of the sensor from the ground surface (Doolittle and Brevik, 2014). Simultaneous assessments at multiple depths by commercial EMI and ER sensors make multi-layer inversion modeling possible (Mester et al., 2011). Mapping both the lateral and vertical variations of soil properties produce highly detailed 3D models (Huang et al., 2017b; Jiang et al., 2019). Another sensor generally used for monitoring soil ECa is the time domain reflectometer (TDR). The TDR, a stationary sensor generally installed within the soil at determined depths, monitors temporal variations of moisture content and ECa. The TDR measures the propagation time of an electromagnetic wave to travel down a soil probe and back, which is a function of the dielectric constant of the porous media (Noborio, 2001). Several TDR technologies for soil monitoring (including moisture, ECa, and temperature) are available.
ECa, or its inverse ER is a function of several soil properties, including soil texture, amount and pattern of voids, soil-water content, fluid electrical conductivity (solute concentration), and temperature (Corwin and Yemoto, 2020). In particular, the amount and type of clay minerals strongly affects ECa, because of their high ionic conductivity and high surface area. In general, 1:1 clay minerals (kaolinite, halloysite) have a relatively low surface area and lower ion exchange capacity than 2:1 interlayer clay minerals (illite, vermiculite, montmorillonite), which provide more spaces into which cations can be adsorbed (Kriaa et al., 2014). At the same time, the electrical current in soils is mainly based on the displacement of ions in pore-water, and is therefore greater with the presence of dissolved salts. Thus, the electrical current in soils strongly depends on the amount of water in the pores and on the concentration of soluble salts. Several papers reported that although direct causal relationships do not exist between exchangeable sodium and ECa, indirect and site-specific relationships are commonly found, and can be used to map SAR spatial variability (Amezketa, 2007; Heilig et al., 2011; Ganjegunte et al., 2014). High content of exchangeable sodium decreases soil aggregation and increases bulk density. Soil bulk density is another important feature influencing ECa measurements, because high soil porosity and aggregation increase the soil-air content, and thus lower the ECa (Corwin and Lesch, 2003; Heilig et al., 2011). Further studies are needed to assess the usefulness of mapping the ECa for identifying spatial variability of soil sodicity in a wider range of saline and non-saline soils (Amezketa, 2007; Ganjegunte et al., 2014).
VNIR-DRS is another promising proximal sensing technique for estimating EC and soil salinity. Aldabaa et al. (2015) compared several models for predicting EC of saline soils in Texas, United States, using VNIR-DRS spectra, and found good accuracy for all models, as shown by high residual prediction deviation (RPD; from 2.49 to 3.1). Similar performance indices of EC prediction in saline soils from VNIR-DRS spectra were found by Farifteh et al. (2007) and Peng et al. (2016). At the same time, other experiments studying the relationships between VNIR-DRS and ECe in different soil types, from very low to medium EC and of different textures, reported much lower prediction performance, and site-specific relationships (Zornoza et al., 2008; Zovko et al., 2018). The spectral response of saline soils can be explained by vibrational absorption due to water molecules chemically bound as part of the crystal structure of the evaporate minerals (Howari et al., 2002). Sensitive bands for soils affected by Na2SO4 type salts were identified from 1,920 to 2,230 nm, for NaCl type salts between 1,970 and 2,450 nm, and for Na2CO3 type salts from 350 to 400 nm (Wang et al., 2012). The results reported from these studies demonstrated that VNIR-DRS can determine not only EC, but also the type of salt and mineralogy in saline soils and crusts (Howari et al., 2002). Regarding the use of VNIR-DRS for ESP estimation, a recent paper by Zhao et al. (2021) on cotton plantations in Australia, reported poor accuracy of predictive models (RPD <1.4), and recommend not using this technique for ESP prediction. The same results of low accuracy of VNIR-DRS prediction for ESP estimation were reported by Chang et al. (2001), as well as by Zornoza et al. (2008).
Remote Sensing Methods
Various remote sensing data acquisition techniques have been applied for identifying and monitoring soil salinity in salt-affected soils. Indeed, remotely sensed data can potentially be used for low-cost mapping and temporal monitoring of topsoil salinity across extensive areas. Therefore, the topic of remote sensing applications for soil salinity assessment has received tremendous attention over the last decade. The availability of new free multispectral images, such as Landsat 8, Sentinel 2 and Hyperion, promote this trend.
The earliest review of remote sensing of salt-affected soils, by Mougenot et al. (1993), included the detection of calcite and various salts on the ground surface. Successive thematic reviews on remote sensing methods for monitoring salt-affected soils were published by Metternicht and Zinck (2003), Farifteh et al. (2006), Ben-Dor et al. (2008), Corwin and Scudiero (2019), and Mahajan et al. (2020).
In salt-affected areas, soil moisture is very low during the dry season, and salts may precipitate around the soil surface, creating efflorescence, crusts, puffy structures, and cracks. These surface features in bare soils can be easily detected by aerial and satellite images, because they accentuate the light color and affect the roughness of topsoil (Metternicht and Zinck, 2003). Several authors have determined key spectral absorption bands of salt-affected soils in the range of visible (Vis: 450–740 nm), near infrared (NIR: 780–1,000 nm), and short-wave infrared (1,550–1,650 nm and 2,100–2,300 nm) wavelengths (Khan et al., 2005; Douaoui et al., 2006; Nield et al., 2007; El Harti et al., 2016; Wang et al., 2019). These VNIR-SWIR absorption peaks are mainly associated with the internal vibration status of anions or water molecules, which are trapped in the crystal structure of the salts (Farifteh et al., 2008). The major spectral indices commonly used to monitor salt-affected soils are summarized in Table 4. The use of remote sensing for the assessment of soil sodicity is less common than for assessing soil salinity. A relatively rare example for such an assessment is a study by Bai et al. (2016), who reported good correlation between Landsat 8-OLI bands 1, 2, 3, and 4 and soil pH in alkali soils of northeastern China.
Often, topsoil figures of salinity or sodicity can be blurred by vegetation, which creates spectral confusions with the salt reflectance properties. Moreover, salt that does not form clear surface features, or salinity/sodicity in deeper soil layers, cannot be directly differentiated by remote sensing. In these cases, indirect drivers, based on the response of vegetation to salinity stress, are often used (Metternicht and Zinck, 2003). The negative impact of soil salinity/sodicity on plant growth was monitored by several vegetation indices, such as the normalized difference vegetation index (NDVI) (Scudiero et al., 2013; Gorji et al., 2017), the enhanced vegetation index (EVI) (Lobell et al., 2010), and the soil-adjusted vegetation index (SAVI) (Allbed et al., 2014). Scudiero et al. (2014) proposed a specific empirical index for plant salinity stress, named canopy response salinity index (CRSI), which includes the RGB (red-green-blue, the Vis band) and the NIR bands of Landsat 7. Vegetation indices show better performance when corrected using soil indices, a finding that demonstrates both the effect of soil on the spectral response of the surface and the presence of a discontinuous or weakly-developed cover (Metternicht and Zinck, 2003). For this reason, multivariate (PCA, PLS) and computational methods (Support Vector Machine, Artificial Neural Networks) for predicting soil salinity from several remote sensing indices, both pedological and vegetal, are currently considered to be the best approach for salinity mapping (Scudiero et al., 2014; Fan et al., 2015; Taghadosi et al., 2019; Wang et al., 2019; Mahajan et al., 2020).
Thermal infrared images, either obtained from satellites or airborne sensors, are also used for estimating both moisture and salt content of soil, although moderate results were obtained when using the thermal range spectrum alone for soil salinity prediction (Metternicht and Zinck, 2003). At the same time, it was demonstrated that the inclusion of thermal infrared bands into Vis-NIR bands can strongly improve the prediction of soil salinity (Verma et al., 1994; Aldabaa et al., 2015). Particularly, it was shown that thermal bands help to distinguish Na+ from other salts in alkaline soils (Farifteh et al., 2006).
Satellite microwaves, both passive and active, are also used for mapping and monitoring salt-affected lands (Bell et al., 2001; Wu et al., 2018). The high penetration of microwaves through clouds and its sensitivity to dielectric properties make this possible (Gong et al., 2013). In general, microwave bands at lower frequencies, especially the lowest L-bands (1.25 GHz), are considered suitable for detecting salinity under different settings, although soil moisture strongly affects the dielectric properties and the microwave images (Metternicht and Zinck, 2003). Details regarding microwave technology for monitoring soil salinity are available in Gong et al. (2013) and in Wu (2019).
Prevention, Mitigation, and Restoration of Saline and Sodic Soils
Site Selection
Judicious site selection of new agricultural lands minimizes the risk of water logging and soil salinization. First, coastal sites that are highly prone to seawater intrusion, as well as inland sites prone to saltwater intrusion (see: Duan, 2016), should be avoided. Second, sites with a shallow aquifer, with a risk of secondary salinization by capillary force of groundwater (see: Devkota et al., 2015), should also be avoided. Third, challenging physical settings including certain climatic, lithologic, topographic, and pedologic conditions that increase the risk of water logging and prevent effective drainage of excess water from the target land unit (see: Gebrehiwot, 2018) should be excluded.
However, challenging terrains are often the only available option, necessitating their use for agriculture. In these events, special attention should be paid for establishing artificial drainage systems, which must effectively control the groundwater table, lowering the salt-affected soil layer far below the crops’ root zone. According to the physical settings, agro-technical level of development, and available funds, this could be implemented using open canal or tunnel systems around the fields’ circumference, or by installing a subsurface drainage system across the field (Cuevas et al., 2019). Both circumferential and subsurface drainage systems can be effective in minimizing the fields’ risk of soil salinization. However, in sodic lands, drainage systems may not effectively remove the Na+ from the soil (NDSU Extension Service–Subsurface tile drainage). Similarly, in saline-sodic lands, drainage will cause the preferential removal of Ca2+ and Mg2+, making Na+ dominant in the soil (NDSU Extension Service, 2014—Saline and sodic soils). Regardless, to prevent potential damage further downstream, effluent water flow should be constrained in retention ponds, where it can be monitored and treated before being released to the environment or reused for agriculture.
Means for prevention, mitigation, and restoration of saline and sodic soils are discussed in the following sub-sections, with an emphasis on aspects related to both recharge (where net movement of water is downward) and discharge (where net movement of water is upward) processes, and their consequences to the upper soil layers and underground aquifer.
Salt Flushing and Leaching
Apparently, the uppermost salt-affected soil layer can be mechanically removed using heavy tractor-scraper machineries. However, this practice is rather expensive, and its efficacy for reducing soil salinity is usually short-term (Cuevas et al., 2019). At the same time, flushing with sufficient volume of high-quality water is suitable for heavy textured soils with salt crusts, where the flushed salts from the soil surface enter the drainage system and are thus removed from the target land (Shahid et al., 2018b).
Regardless, saline soils can be restored by leaching the salts out of the soil profile through excess irrigation, coupled with a drainage system deep enough to prevent capillary rise of the water back into the root zone or soil surface (Cuevas et al., 2019). For example, in west-central Kazakhstan, pre-season technical inundation lasting several days is common in furrow irrigation agricultural systems. Also, throughout the cropping season, irrigation water is applied beyond the crops’ requirements, leaching the salts from the rooting zone (Devkota et al., 2015). This excess water, referred to as the leaching fraction, must increase along the growing season, accordingly with the growth of the crops’ root system (Cuevas et al., 2019). Often, the economic and environmental costs of intentional flushing and leaching of salts are rather high, questioning their viability and sustainability over the long-run. Yet, the partial or full subsidy of agricultural water in many countries, alongside ignorance regarding the environmental footprint of agriculture at all levels (i.e., from the farm level to the national and international levels), allow these practices to persist in extensive parts of the world. Regardless, as shown by Driessen et al. (2001), frequent leaching may remove Ca2+ and Mg2+ from the soil profile, increasing SAR if Na+ is present, and consequently increasing sodicity and forming of solonetz.
Chemical Remediation
Saline soils cannot be restored by chemical methods (Shahid et al., 2018b). At the same time, sodic soils can be restored by applying Ca2+ to displace the excess Na+ from the exchange complex. Soluble sources of Ca2+ are released to the soil solution, replacing the excess Na+ in the soil’s exchange complex, thus soluble Na+ becomes available for leaching. Because lime is only slightly soluble in water, it cannot provide enough Ca2+ to displace Na+. Therefore, the most widely used Ca2+ sources are highly soluble minerals such as gypsum (CaSO4·2H2O) (Qadir et al., 2007) and gypsum-like byproducts–e.g., phosphogypsum (a byproduct of phosphoric acid manufacturing), coalgypsum (a byproduct of coal power plants), and lactogypsum (a byproduct of lactic acid and lactate manufacturing) (Amezketa et al., 2005). Following the application of such amendments, and after the Na+ is solubilised, the soluble salts must be leached (Paz et al., 2020) by excess irrigation. The rate of gypsum application varies greatly, and depends on the magnitude of sodification, and the prevailing biophysical conditions, of which most important are the prevailing climate, parent material, soil type, quality of irrigation and underground water, and the coming crop variety (Amezketa et al., 2005; Qadir et al., 2007; Paz et al., 2020). Chemical remediation of saline-sodic soils is similar to that of sodic soils, followed by salinity remediation practices through improving soil drainage, lowering the water-table level, and leaching of salts (other than Na+) (NDSU Extension Service, 2014—Saline and sodic soils).
Organic and Microbial Remediation
Application of organic amendments, such as livestock manures and composts, increases the soil organic carbon concentration and improves the soil’s macro-aggregation processes and structure formation. These processes improve soil permeability, increasing the leaching capacity of salts from the rhizosphere, and simultaneously decrease evaporation rates, reducing salt accumulation in the surface soil layer (Lakhdar et al., 2009). In addition to livestock-derived organic amendments, this practice can also utilize waste materials, such as municipal solid wastes, transforming these environmental burdens into highly valorized resources (Lakhdar et al., 2009). Yet, special attention should be paid when using highly-saline solid wastes (or manures), which their application may aggravate soil salinity. In sodic lands, the application of organic amendments combined with gypsum was reported to better alleviate soil sodicity than the application of gypsum alone (Gonçalo et al., 2020). The low cost of organic amendments makes them widely utilized for alleviation of soil salinity and sodicity around the world. In addition to these “regular” organic additives, specific, designated organic soil conditioners may also be applied, despite being comparatively expensive. Similar to regular organic amendments, these conditioners are more effective in sodic soils when combined with gypsum (Cuevas et al., 2019).
Tillage Schemes
Soil tillage is a regular practice in croplands, aimed at seedbed preparation. Yet, the intensity of tillage depends not only on the prevailing physical conditions and cropping history, but also on traditions, viewpoints, and beliefs. Alongside the benefits of conventional tillage, such as soil loosening and aeration, several unintentional disadvantages are known, such as the damage to soil structure, increased oxidation of soil organic carbon, breakdown of macro-aggregates, decreased hydraulic conductivity, and increased soil erodibility (Blanco-Canqui and Ruis, 2018). The degraded permeability of the soil lowers its salt leaching efficacy, leading to salt accumulation in the rhizosphere. In recent decades, tremendous advancements in the conservation agricultural sector have led to the development of reduced tillage practices. It was reported that minimum tillage, and particularly no-till systems, improve leaching and negate soil salinity (Botha, 2013). Alongside with the tillage system, the management of crop residue–i.e., the removal vs retention of crop residue–has a tremendous impact on the susceptibility of soil to salinization and sodification (Bezborodov et al., 2010). It was demonstrated that surface mulching (e.g., by the crop residue) decreases evaporation rates, consequently lowering soil salinity (Stavi, 2020) and sodicity (Verhulst et al., 2009). Ground surface mulching is especially relevant in drylands, and where saline water is regularly used for irrigation (Stavi, 2020).
Irrigation Schemes
To minimize aquifer recharge with excess salts and thus limit soil salinization (Finlayson et al., 2010), flood and furrow irrigation should be negated wherever possible (Cuevas et al., 2019). Yet, under certain conditions, technical inundation is required to reduce soil salinity before or during the cropping season (Devkota et al., 2015). Similarly, in non-flooding irrigation methods that use routine, frequent high dose irrigation to leach salts from the rhizosphere increase the risk of groundwater salinization (Devkota et al., 2015). This risk is particularly relevant in drylands, where the risk of soil salinization is high even when high-quality water is used for irrigation (Cuevas et al., 2019).
Conversely, water-saving irrigation methods, such as micro-irrigation systems, and specifically drip irrigation systems, can substantially decrease the risk of groundwater recharge, while effectively leaching salts from the dripper-formed “wet bulbs”, from which the plant roots extract water (Stavi, 2020). Yet, depending on the quality of irrigation-water, as well as on associated agronomic practices, soil salinization and sodification can still occur even under drip irrigation systems (Liu et al., 2020; Stavi, 2020). Recent developments in precision irrigation technologies significantly increase the crop’s water-use efficiency, and may decrease agriculture’s environmental footprint. For example, the irrigation-on-demand (IOD) technology is based on drippers with built-in tensiometers that are directly activated by the crop’s roots. It provides the plant with precise water requirements, specified to its variety and phenological stage (Tal, 2016).
Fertilizing and Manuring Schemes
Use of chemical fertilizer may exacerbate rhizosphere salinization. Moreover, excess fertilizing can increase nutrient recharge into the groundwater (Finlayson et al., 2010). This is not only wasteful in terms of fertilizing efficiency, it also increases the salinity of aquifers (Finlayson et al., 2010), and contaminates them with hazardous materials (Cuevas et al., 2019). Specifically, drinking water from aquifers contaminated with residual nitrate (NO3−) may cause infant methemoglobinemia, certain cancers, and neural tube defects (Ward et al., 2018). Similar effects were reported for organic nutrient management systems, where the use of livestock manure salinizes the soil’s upper layer and increases the leaching of salts and contaminants to the aquifers (Manitoba, 2013). Specifically, manuring may enrich the underground water with pathogenic microorganisms and viruses, imposing a high risk to human health (Ward et al., 2018).
In addition to harming aquifers, runoff processes taking place on either chemically or organically fertilized agricultural lands, may remove excess fertilizers and displace them into surface waterbodies, increasing their salinity and enriching them with nutrients. This may cause eutrophication by algae and degradation of waterbodies (Withers et al., 2014). Recent advances in precision fertilizer systems may increase fertilizer use-efficiency, while reducing the risk of salinization and contamination of both underground and surface waterbodies. Specifically, fertigation technologies, in which dissolved fertilizers are distributed through drip irrigation systems, were shown to optimize fertilizer use-efficiency and reduce agriculture’s environmental footprint (Azad et al., 2020).
Phytoremediation
In sodic lands, crops can contribute to soil restoration through two mechanisms. Plant roots release CO2 and protons into the soil, which both increase acidity in the ambient soil. The CO2 is released by root respiration and bacterial decomposition of root exudates, while protons are released during cation uptake by plant roots. The increased acidity partially dissolves Ca-bearing soil minerals, such as lime, and therefore provides Ca2+, which displaces Na+ from the exchange complex.
Overall, crops with a high tolerance to both drought and salinity should be selected. Specifically, deep-root crop varieties that extract water from depths of several meters (Cuevas et al., 2019) reduce the risk of groundwater discharge and the upward migration of salts to the ground surface (Finlayson et al., 2010). Among grain crops, barley (Hordeum vulgare L.) is highly tolerant to dry conditions and soil salinity. Further, some relatively new barley cultivars proved to be exceptionally salt tolerant (Katerji et al., 2006). Among vegetable crops, potato (Solanum tuberosum L.), carrot (Daucus carota subsp. Sativus (Hoffm.) Schubl. & G. Martens), onion (Allium cepa L.), lettuce (Lactuca sativa L.), and cabbage (Brassica oleracea L.) were reported to have a comparatively high tolerance to moderately saline conditions (de Vos et al., 2016). Among forage crops, alfalfa (Medicago sativa L.) is known to tolerate soil salinity well, with some specific cultivars having exceptionally high tolerance (Scasta et al., 2012). Kallar grass (Leptochloa fusca (L.) Kunth.), a fodder crop, has been widely used in salt-affected and water-logged lands in Pakistan, and has proved to effectively ameliorate saline, sodic, and saline-sodic soils (Nadeem et al., 2017).
Regardless, crop rotation has been reported to effectively prevent soil salinization, where crops with high tolerance to salinity are planted during the dry period, while more sensitive crops are planted in the wet season, when rains wash the salts from the upper soil layers to the subsoil layers. Other agronomic practices, such as cover cropping and green manuring–where certain herbaceous crops are grown in the off-season or in rotation with main crops, and can either be left on the ground (cover crops) or inverted into the soil (through inversion tillage, for green manures)—were reported to decrease soil salinity while improving the bio-physio-chemical quality of soil (Cuevas et al., 2019). Among these herbaceous crops, salt-tolerant legumes, such as alfalfa and riverhemp (Sesbania spp.) are rather common for increasing the system’s nutrient availability and decreasing the soil’s salinity and solidity levels. Additionally, such legumes can be used as feed for livestock, either through on-site grazing or as harvested forage (Qadir et al., 2007). Overall, phytoremediation is a comparatively cheap method of land restoration. It is also environmentally friendly, as it avoids the use of synthetic products and improves carbon sequestration (Paz et al., 2020). Major practices of phytoremediation are summarized in Table 5A.
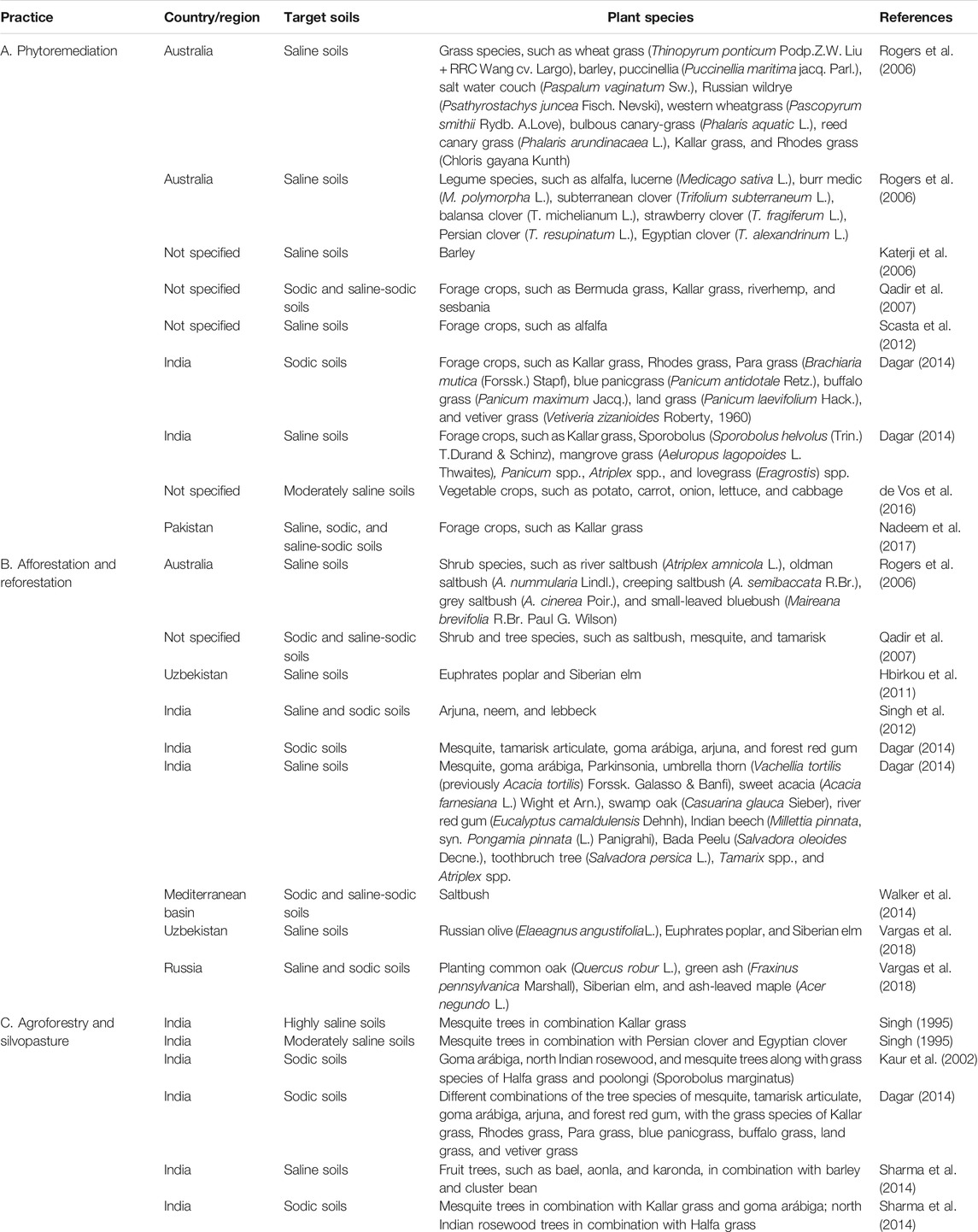
TABLE 5. Major practices of phytoremediation (A), afforestation and reforestation (B), and agroforestry and silvopasture (C), for soil salinity and sodicity prevention and restoration.
Afforestation and Reforestation
In addition to the “regular” agronomic means and practices, planting tree and shrub species with deep root systems and high water demand proved to be effective in controlling the discharge of underground water, limiting salinization or sodification of the upper soil layers, and subsequently restoring saline, sodic, and saline-sodic soils (Qadir et al., 2007; Finlayson et al., 2010). These trees and shrubs should be planted following certain procedures that facilitate them to overcome the upper and most saline or sodic layers. For example, furrow system, where the trees and shrubs are planted in the furrows’ salt-washed micro-habitats, may be suitable. Alternatively, sub-surface planting, where the trees and shrubs are planted in deep holes or trenches whose depth exceeds the most severely salt-affected surface layer, may be necessary (Dagar et al., 2001; Banyal et al., 2017).
In west-central Uzbekistan, afforestation of saline croplands with Euphrates poplar (Populus euphratica Oliv.) and Siberian elm (Ulmus pumila L.) proved to be highly effective in reducing soil salinity, while improving the soil’s aggregate stability and organic carbon pools (Hbirkou et al., 2011). In northern India, mesquite (Prosopis juliflora (Sw.) DC.), tamarisk articulata (Tamarix articulate Vahl.), and goma arábiga (Acacia nilotica (L.) Delile) were reported to be highly effective in restoring sodic soils. At the same time, forest red gum (Eucalyptus tereticornis Sm.), Indian rosewood (Dalbergia sissoo Roxb.), Manila tamarind (Pithecellobium dulce (Roxb.) Benth.), arjuna (Terminalia arjuna (Roxb.) Wight & Arn.), sausage tree (Kigelia pinnata (Jacq.) DC.), Parkinsonia (Parkinsonia aculeate L.), and grey-leaved saucer berry (Cordia rothii Roem. & Schult.) showed good survival but relatively low productivity (Dagar et al., 2001). In another northern Indian study, mixed reforested lands–comprising arjuna, neem (Azadirachta indica A. Juss. 1830), and lebbeck (Albizia lebbeck (L.) Benth.), among other tree species, were reported to restore sodic croplands, with specific improvement of the physio-chemical, biological, and bio-chemical properties of soil (Singh et al., 2012). Halophyte shrub species that are prevalent across the Mediterranean basin, such as saltbush (Atriplex halimus L.), are able to remove salts through epidermal glands on both surfaces of their leaves. This shrub species is widely utilized as feed for livestock animals (Walker et al., 2014). Major practices of afforestation and reforestation are summarized in Table 5B.
Further, in addition to regular afforestation and reforestation practices, agroforestry and silvopasture systems–in which trees or shrubs are planted at certain spatial patterns, and combined with grain, vegetable, or forage crops–are known to restore saline, sodic, and saline-sodic soils, while providing food, feed, and fiber. These systems provide food, feed, construction materials, fuelwood, and many other products, which can be utilized either for domestic consumption or for selling as a source of income (Dagar, 2014). In saline soils of northern India, fruit trees such as bael (Aegle marmelos (L.) Corrêa), aonla (Phyllanthus emblica L.), and karonda (Carissa carandas L.) are planted in combination with barley and cluster bean (Cyamopsis tetragonoloba (L.) Taub.). In sodic soils of the same region, mesquite trees are planted in combination with Kallar grass and goma arábiga, and north Indian rosewood trees are planted in combination with Halfa grass (Desmostachya bipinnata (L.) Stapf) (Sharma et al., 2014). Major practices of agroforestry and silvopasture systems are summarized in Table 5C.
Conclusion
Soil salinization and sodification affect extensive areas worldwide, but mostly characterize drylands. While primary salinity or sodicity occurs in a combination of certain physical settings, secondary salinity or sodicity is predominantly determined by agricultural activities, specifically irrigation. Climatic change exacerbates this hazard, and active interventions are required to safeguard dryland soils. Judicious selection of sites for the establishment of croplands, alongside with the use of water-saving irrigation technologies, can reduce the risk of waterlogging and soil salinization and sodification processes. In saline soils, routine measures should be taken to allow effective drainage of excess water, alongside with flushing and leaching of salts. In sodic and saline-sodic soils, chemical remediation measures, and specifically the use of gypsum-based soil amendments, could be effective in restoring affected lands. Further, the use of conservation agricultural practices, such as reduced tillage systems, crop residue management, manuring/composting, precision fertilizer schemes, and proper crop selection can considerably reduce the risk of soil salinity and sodicity. Specifically, the use of advanced forestry and agroforestry systems has a tremendous potential in restoring saline and sodic soils.
Author Contributions
All authors listed have made a substantial, direct, and intellectual contribution to the work and approved it for publication.
Conflict of Interest
The authors declare that the research was conducted in the absence of any commercial or financial relationships that could be construed as a potential conflict of interest.
Publisher’s Note
All claims expressed in this article are solely those of the authors and do not necessarily represent those of their affiliated organizations, or those of the publisher, the editors and the reviewers. Any product that may be evaluated in this article, or claim that may be made by its manufacturer, is not guaranteed or endorsed by the publisher.
Acknowledgments
This review paper was made possible due to the framework provided by the EU COST Action 19128 on ‘Pan-European Network for Climate Adaptive Forest Restoration and Reforestation’. The Dead Sea and Arava Science Center is supported by the Israel Ministry of Science and Technology. The authors gratefully acknowledge Michelle Finzi for proofreading of the manuscript. Also, the authors are grateful to three reviewers, whose comments considerably improved the manuscript.
References
Abbas, A., Khan, S., Hussain, N., Hanjra, M. A., and Akbar, S. (2013). Characterizing Soil Salinity in Irrigated Agriculture Using a Remote Sensing Approach. Phys. Chem. Earth, Parts A/B/C 55-57, 43–52. doi:10.1016/j.pce.2010.12.004
Aldabaa, A. A. A., Weindorf, D. C., Chakraborty, S., Sharma, A., and Li, B. (2015). Combination of Proximal and Remote Sensing Methods for Rapid Soil Salinity Quantification. Geoderma 239-240, 34–46. doi:10.1016/j.geoderma.2014.09.011
Alexandre, C., Borralho, T., and Durao, A. (2018). Evaluation of Salinization and Sodification in Irrigated Areas with Limited Soil Data: Case Study in Southern Portugal. Spanish J. Soil Sci. 8, 102–120. doi:10.3232/SJSS.2018.V8.N1.07
Allbed, A., Kumar, L., and Aldakheel, Y. Y. (2014). Assessing Soil Salinity Using Soil Salinity and Vegetation Indices Derived from IKONOS High-Spatial Resolution Imageries: Applications in a Date palm Dominated Region. Geoderma 230-231, 1–8. doi:10.1016/j.geoderma.2014.03.025
Amezketa, E., Aragüés, R., and Gazol, R. (2005). Efficiency of Sulfuric Acid, Mined Gypsum, and Two Gypsum By-Products in Soil Crusting Prevention and Sodic Soil Reclamation. Agron. J. 97, 983–989. doi:10.2134/agronj2004.0236
Amezketa, E. (2007). Use of an Electromagnetic Technique to Determine Sodicity in saline-sodic Soils. Soil Use Manage. 23, 278–285. doi:10.1111/j.1475-2743.2007.00094.x
Artzy, M., and Hillel, D. (1988). A Defense of the Theory of Progressive Soil Salinization in Ancient Southern Mesopotamia. Geoarchaeology 3, 235–238. doi:10.1002/gea.3340030306
Ayers, R. S., and Westcot, D. W. (1985). Water Quality for Agriculture. Rome: FAO. FAO Irrigation and Drainage Paper no. 29.
Azad, N., Behmanesh, J., Rezaverdinejad, V., Abbasi, F., and Navabian, M. (2020). An Analysis of Optimal Fertigation Implications in Different Soils on Reducing Environmental Impacts of Agricultural Nitrate Leaching. Sci. Rep. 10, 7797. doi:10.1038/s41598-020-64856-x
Bai, L., Wang, C., Zang, S., Zhang, Y., Hao, Q., and Wu, Y. (2016). Remote Sensing of Soil Alkalinity and Salinity in the Wuyu'er-Shuangyang River Basin, Northeast China. Remote Sensing 8, 163. doi:10.3390/rs8020163
Banyal, R., Rajkumar, M., Kumar, M., Yadav, R. K., and Dagar, J. C. (2017). “Agroforestry for Rehabilitation and Sustenance of Saline Ecologies,” in Agroforestry – Anecdotal to Modern Science. Editors C. J. Dagar, and V. P. Tewari (Berlin: Springer), 413–454. doi:10.1007/978-981-10-7650-3_16
Bauder, T. A., Waskom, R. M., Sutherland, P. L., and Davis, J. G. (2011). Irrigation Water Quality Criteria, Crop Series/irrigation. Fact Sheet No. 0.506. Fort Collins: Colorado State University Extension Publication.
Bell, D., Menges, C., Ahmad, W., and Van Zyl, J. J. (2001). The Application of Dielectric Retrieval Algorithms for Mapping Soil Salinity in a Tropical Coastal Environment Using Airborne Polarimetric SAR. Remote Sensing Environ. 75, 375–384. doi:10.1016/s0034-4257(00)00180-2
Ben-Dor, E., Metternicht, G., Goldshleger, N., Mor, E., Mirlas, V., and Basson, U. (2008). Review of Remote Sensing-Based Methods to Assess Soil Salinity. Boca Raton, FL, USA: CRC Press, 39–60.
Bezborodov, G. A., Shadmanov, D. K., Mirhashimov, R. T., Yuldashev, T., Qureshi, A. S., Noble, A. D., et al. (2010). Mulching and Water Quality Effects on Soil Salinity and Sodicity Dynamics and Cotton Productivity in Central Asia. Agric. Ecosyst. Environ. 138, 95–102. doi:10.1016/j.agee.2010.04.005
Blanco-Canqui, H., and Ruis, S. J. (2018). No-tillage and Soil Physical Environment. Geoderma 326, 164–200. doi:10.1016/j.geoderma.2018.03.011
Botha, P. B. (2013). “The Effect of Long-Term Tillage Practices on Selected Soil Properties in the Swartland Wheat Production Area of the Western Cape,” in Dissertation Presented for the Degree of Master of Soil Science in the Faculty of AgriSciences at Stellenbosch University. Available at: https://core.ac.uk/download/pdf/37410625.pdf.
Brown, V. A., Brown, K. C., Siddoway, F. H., Mayland, H. F., and Miller, M. R. (1982). Continuing Education Needs of Occupational Health Nurses. Occup. Health Nurs. 30, 22–26. U.S. Department of Agriculture Conservation Research Report No. 30. doi:10.1177/216507998203000404
Bui, E. N. (2017). “Causes of Soil Salinization, Sodification, and Alkalinization,” in Oxford Research Encyclopedia of Environmental Science (Oxford: Oxford University Press). doi:10.1093/acrefore/9780199389414.013.264
Burt, C. M., and Isbell, B. (2005). Leaching of Accumulated Soil Salinity under Drip Irrigation. Trans. ASAE 48, 2115–2121. doi:10.13031/2013.20097
Chang, C.-W., Laird, D. A., Mausbach, M. J., and Hurburgh, C. R. (2001). Near-Infrared Reflectance Spectroscopy-Principal Components Regression Analyses of Soil Properties. Soil Sci. Soc. Am. J. 65, 480–490. doi:10.2136/sssaj2001.652480x
Cook, B. I., Smerdon, J. E., Seager, R., and Coats, S. (2014). Global Warming and 21st century Drying. Clim. Dyn. 43, 2607–2627. doi:10.1007/s00382-014-2075-y
Corwin, D. L., and Lesch, S. M. (2003). Application of Soil Electrical Conductivity to Precision Agriculture. Agron.j. 95, 455–471. doi:10.2134/agronj2003.4550
Corwin, D. L., and Scudiero, E. (2019). Review of Soil Salinity Assessment for Agriculture across Multiple Scales Using Proximal And/or Remote Sensors. Adv. Agron. 158, 1–130. doi:10.1016/bs.agron.2019.07.001
Corwin, D. L., and Yemoto, K. (2020). Salinity: Electrical Conductivity and Total Dissolved Solids. Soil Sci. Soc. Am. J. 84, 1442–1461. doi:10.1002/saj2.20154
Cuevas, J., Daliakopoulos, I. N., del Moral, F., Hueso, J. J., and Tsanis, I. K. (2019). A Review of Soil-Improving Cropping Systems for Soil Salinization. Agronomy 9, 295. doi:10.3390/agronomy9060295
Dagar, J. C. (2014). “Greening Salty and Waterlogged Lands through Agroforestry Systems for Livelihood Security and Better Environment,” in Agroforestry Systems in India: Livelihood Security & Ecosystem Services, Advances in Agroforestry 10. Editors J.C. Dagar, A.K. Singh, and A. Arunachalam (Berlin: Springer), 273–332. doi:10.1007/978-81-322-1662-9_9
Dagar, J. C., Singh, G., and Singh, N. T. (2001). Evaluation of forest and Fruit Trees Used for Rehabilitation of Semiarid Alkali-Sodic Soils in India. Arid Land Res. Manage. 15, 115–133. doi:10.1080/15324980151062742
de Vos, A., Bruning, B., van Straten, G., Oosterbaan, R., Rozema, J., and van Bodegom, P., (2016). Crop Salt Tolerance under Controlled Field Conditions in The Netherlands, Based on Trials Conducted at Salt Farm Texel. Available at: https://www.salineagricultureworldwide.com/uploads/file_uploads/files/Report_Crop_Salt_Tolerance-Salt_Farm_Texel.pdf.
Devkota, M., Martius, C., Gupta, R. K., Devkota, K. P., McDonald, A. J., and Lamers, J. P. A. (2015). Managing Soil Salinity with Permanent Bed Planting in Irrigated Production Systems in Central Asia. Agric. Ecosyst. Environ. 202, 90–97. doi:10.1016/j.agee.2014.12.006
Di Liberto, V., Nuccio, P. M., and Paonita, A. (2002). Genesis of Chlorine and sulphur in Fumarolic Emissions at Vulcano Island (Italy): Assessment of pH and Redox Conditions in the Hydrothermal System. J. Volcanology Geothermal Res. 116, 137–150. doi:10.1016/s0377-0273(02)00215-9
Doolittle, J. A., and Brevik, E. C. (2014). The Use of Electromagnetic Induction Techniques in Soils Studies. Geoderma 223-225, 33–45. doi:10.1016/j.geoderma.2014.01.027
Douaoui, A. E. K., Nicolas, H., and Walter, C. (2006). Detecting Salinity Hazards within a Semiarid Context by Means of Combining Soil and Remote-Sensing Data. Geoderma 134, 217–230. doi:10.1016/j.geoderma.2005.10.009
Driessen, P., Deckers, J., Spaargaren, O., and Nachtergaele, F. (2001). Lecture Notes on the Major Soils of the World. Rome: Food and Agriculture Organization. Available at: http://www.fao.org/3/y1899e/y1899e.pdf.
Duan, Y. (2016). Saltwater Intrusion and Agriculture: a Comparative Study between the Netherlands and China. Stockholm: TRITALWR Degree Project. Available at: http://www.diva-portal.org/smash/get/diva2:1060822/FULLTEXT01.pdf.
El Harti, A., Lhissou, R., Chokmani, K., Ouzemou, J.-e., Hassouna, M., Bachaoui, E. M., et al. (2016). Spatiotemporal Monitoring of Soil Salinization in Irrigated Tadla Plain (Morocco) Using Satellite Spectral Indices. Int. J. Appl. Earth Observation Geoinformation 50, 64–73. doi:10.1016/j.jag.2016.03.008
European Communities (2009). Salinization and Sodification, Fact Sheet No. 4. Brussels: European Commission. Available at: https://esdac.jrc.ec.europa.eu/projects/SOCO/FactSheets/ENFactSheet-04.pdf.
Fan, X., Liu, Y., Tao, J., and Weng, Y. (2015). Soil Salinity Retrieval from Advanced Multi-Spectral Sensor with Partial Least Square Regression. Remote Sensing 7, 488–511. doi:10.3390/rs70100488
FAO/UNESCO (1974). Soil Map of the World. Rome: Food and Agriculture Organization of the United Nations.
Farifteh, J., Farshad, A., and George, R. J. (2006). Assessing Salt-Affected Soils Using Remote Sensing, Solute Modelling, and Geophysics. Geoderma 130, 191–206. doi:10.1016/j.geoderma.2005.02.003
Farifteh, J., Van der Meer, F., Atzberger, C., and Carranza, E. J. M. (2007). Quantitative Analysis of Salt-Affected Soil Reflectance Spectra: A Comparison of Two Adaptive Methods (PLSR and ANN). Remote Sensing Environ. 110, 59–78. doi:10.1016/j.rse.2007.02.005
Farifteh, J., Van der Meer, F., Van der Meijde, M., and Atzberger, C. (2008). Spectral Characteristics of Salt-Affected Soils: A Laboratory experiment. Geoderma 145, 196–206. doi:10.1016/j.geoderma.2008.03.011
Finlayson, J., Bathgate, A., Nordblom, T., Theiveyanathan, T., Farquharson, B., Crosbie, R., et al. (2010). Balancing Land Use to Manage River Volume and Salinity: Economic and Hydrological Consequences for the Little River Catchment in Central West, New South Wales, Australia. Agric. Syst. 103, 161–170. doi:10.1016/j.agsy.2009.12.003
Fitzpatrick, R., Boucher, S., Naidu, R., and Fritsch, E. (1994). Environmental Consequences of Soil Sodicity. Soil Res. 32, 1069–1093. doi:10.1071/sr9941069
Follett, R. H., and Soltanpour, P. N. (2002). Irrigation Water Quality Criteria. Fort Collins: Colorado State University Publication No. 0, 506.
Forkutsa, I., Sommer, R., Shirokova, Y. I., Lamers, J. P. A., Kienzler, K., Tischbein, B., et al. (2009). Modeling Irrigated Cotton with Shallow Groundwater in the Aral Sea Basin of Uzbekistan: II. Soil Salinity Dynamics. Irrig. Sci. 27, 319–330. doi:10.1007/s00271-009-0149-0
Ganjegunte, G. K., Sheng, Z., and Clark, J. A. (2014). Soil Salinity and Sodicity Appraisal by Electromagnetic Induction in Soils Irrigated to Grow Cotton. Land Degrad. Develop. 25, 228–235. doi:10.1002/ldr.1162
Gebrehiwot, K. A. (2018). A Review on Waterlogging, Salinization and Drainage in Ethiopian Irrigated Agriculture. Sustain. Water Resour. Manag. 4, 55–62. doi:10.1007/s40899-017-0121-8
Ghassemi, F., Jakeman, A. J., and Nix, H. A. (1995). Salinisation of Land and Water Resources: Human Causes, Extent, Management and Case Studies. Wallingford: CABI Publishing, 526.
Gong, H., Shao, Y., Brisco, B., Hu, Q., and Tian, W. (2013). Modeling the Dielectric Behavior of saline Soil at Microwave Frequencies. Can. J. Remote Sensing 39, 17–26. doi:10.5589/m13-004
Gonçalo, F. F., Dias, N. D., Suddarth, S. R. P., Ferreira, J. F. S., Ray, G., Anderson, R. G., et al. (2020). Reclaiming Tropical saline-sodic Soils with gypsum and Cow Manure. Water 12, 57. doi:10.3390/w12010057
Gorji, T., Sertel, E., and Tanik, A. (2017). Monitoring Soil Salinity via Remote Sensing Technology under Data Scarce Conditions: A Case Study from Turkey. Ecol. Indicators 74, 384–391. doi:10.1016/j.ecolind.2016.11.043
Hassani, A., Azapagic, A., and Shokri, N. (2020). Predicting Long-Term Dynamics of Soil Salinity and Sodicity on a Global Scale. Proc. Natl. Acad. Sci. USA 117, 33017–33027. doi:10.1073/pnas.2013771117
Hbirkou, C., Martius, C., Khamzina, A., Lamers, J. P. A., Welp, G., and Amelung, W. (2011). Reducing Topsoil Salinity and Raising Carbon Stocks through Afforestation in Khorezm, Uzbekistan. J. Arid Environments 75, 146–155. doi:10.1016/j.jaridenv.2010.09.018
Heilig, J., Kempenich, J., Doolittle, J., Brevik, E. C., and Ulmer, M. (2011). Evaluation of Electromagnetic Induction to Characterize and Map Sodium-Affected Soils in the Northern Great Plains. Soil Surv. Horizons 52, 77–88. doi:10.2136/sh2011.3.0077
Howari, F. M., Goodell, P. C., and Miyamoto, S. (2002). Spectral Properties of Salt Crusts Formed on saline Soils. J. Environ. Qual. 31, 1453–1461. doi:10.2134/jeq2002.1453
Hu, Y., and Schmidhalter, U. (2005). Drought and Salinity: A Comparison of Their Effects on mineral Nutrition of Plants. Z. Pflanzenernähr. Bodenk. 168, 541–549. doi:10.1002/jpln.200420516
Huang, J., Koganti, T., Santos, F. A. M., and Triantafilis, J. (2017b). Mapping Soil Salinity and a Fresh-Water Intrusion in Three-Dimensions Using a Quasi-3d Joint-Inversion of DUALEM-421S and EM34 Data. Sci. Total Environ. 577, 395–404. doi:10.1016/j.scitotenv.2016.10.224
Huang, J., Li, Y., Fu, C., Chen, F., Fu, Q., Dai, A., et al. (2017a). Dryland Climate Change: Recent Progress and Challenges. Rev. Geophys. 55, 719–778. doi:10.1002/2016rg000550
IUSS Working Group WRB (2015). World Reference Base for Soil Resources 2014, Update 2015: International Soil Classification System for Naming Soils and Creating Legends for Soil Maps, 106. Vienna: World Soil Resources Reports No.
Ivushkin, K., Bartholomeus, H., Bregt, A. K., Pulatov, A., Kempen, B., and de Sousa, L. (2019). Global Mapping of Soil Salinity Change. Remote Sensing Environ. 231, 111260. doi:10.1016/j.rse.2019.111260
Jamil, A., Riaz, S., Ashraf, M., and Foolad, M. R. (2011). Gene Expression Profiling of Plants under Salt Stress. Crit. Rev. Plant Sci. 30, 435–458. doi:10.1080/07352689.2011.605739
Jiang, Q., Peng, J., Biswas, A., Hu, J., Zhao, R., He, K., et al. (2019). Characterising Dryland Salinity in Three Dimensions. Sci. Total Environ. 682, 190–199. doi:10.1016/j.scitotenv.2019.05.037
Jolly, I. D., McEwan, K. L., and Holland, K. L. (2008). A Review of Groundwater-Surface Water Interactions in Arid/semi-Arid Wetlands and the Consequences of Salinity for Wetland Ecology. Ecohydrol. 1, 43–58. doi:10.1002/eco.61
Katerji, N., van Hoorn, J. W., Hamdy, A., Mastrorilli, M., Fares, C., Ceccarelli, S., et al. (2006). Classification and Salt Tolerance Analysis of Barley Varieties. Agric. Water Manage. 85, 184–192. doi:10.1016/j.agwat.2006.04.006
Kaur, B., Gupta, S. R., and Singh, G. (2002). Bioamelioration of a Sodic Soil by Silvopastoral Systems in Northwestern India. Agrofor. Syst. 54, 13–20. doi:10.1023/a:1014221306004
Khan, N. M., Rastoskuev, V. V., Sato, Y., and Shiozawa, S. (2005). Assessment of Hydrosaline Land Degradation by Using a Simple Approach of Remote Sensing Indicators. Agric. Water Manage. 77, 96–109. doi:10.1016/j.agwat.2004.09.038
Kriaa, A., Hajji, M., Jamoussi, F., and Hamzaoui, A. H. (2014). Electrical Conductivity of 1 : 1 and 2 : 1 clay Minerals. Surf. Engin. Appl. Electrochem. 50, 84–94. doi:10.3103/s1068375514010104
Lakhdar, A., Rabhi, M., Ghnaya, T., Montemurro, F., Jedidi, N., and Abdelly, C. (2009). Effectiveness of Compost Use in Salt-Affected Soil. J. Hazard. Mater. 171, 29–37. doi:10.1016/j.jhazmat.2009.05.132
Litalien, A., and Zeeb, B. (2020). Curing the Earth: A Review of Anthropogenic Soil Salinization and Plant-Based Strategies for Sustainable Mitigation. Sci. Total Environ. 698, 134235. doi:10.1016/j.scitotenv.2019.134235
Liu, H., Li, M., Zheng, X., Wang, Y., and Anwar, S. (2020). Surface Salinization of Soil under Mulched Drip Irrigation. Water 12, 3031. doi:10.3390/w12113031
Lobell, D. B., Lesch, S. M., Corwin, D. L., Ulmer, M. G., Anderson, K. A., Potts, D. J., et al. (2010). Regional-scale Assessment of Soil Salinity in the Red River Valley Using Multi-Year MODIS EVI and NDVI. J. Environ. Qual. 39, 35–41. doi:10.2134/jeq2009.0140
Mahajan, G. R., Das, B., Gaikwad, B., Murgaonkar, D., Desai, A., Morajkar, S., et al. (2020). Monitoring Properties of the Salt-Affected Soils by Multivariate Analysis of the Visible and Near-Infrared Hyperspectral Data. Catena 198, 105041. doi:10.1016/j.catena.2020.105041
Mahmuduzzaman, M., Ahmed, Z. U., Nuruzzaman, A. K. M., and Ahmed, F. R. S. (2014). Causes of Salinity Intrusion in Coastal belt of Bangladesh. Int. J. Plant Res. 4 (4A), 8–13. doi:10.5923/s.plant.201401.02
Manitoba (2013). Effects of Manure and Fertilizer on Soil Fertility and Soil Quality. Available at: https://www.gov.mb.ca/agriculture/environment/nutrient-management/pubs/effects-of-manure%20-fertilizer-on%20soil%20fertility-quality.pdf.
Marion, G. M., and Babcock, K. L. (1976). Predicting Specific Conductance and Salt Concentration in Dilute Aqueous Solutions. Soil Sci. 122, 181–187. doi:10.1097/00010694-197610000-00001
Martini, E., Wollschläger, U., Musolff, A., Werban, U., and Zacharias, S. (2017). Principal Component Analysis of the Spatiotemporal Pattern of Soil Moisture and Apparent Electrical Conductivity. Vadose Zone J. 16, 1–12. doi:10.2136/vzj2016.12.0129
Mester, A., van der Kruk, J., Zimmermann, E., and Vereecken, H. (2011). Quantitative Two-Layer Conductivity Inversion of Multi-Configuration Electromagnetic Induction Measurements. Vadose Zone J. 10, 1319–1330. doi:10.2136/vzj2011.0035
Metternicht, G. I., and Zinck, J. A. (2003). Remote Sensing of Soil Salinity: Potentials and Constraints. Remote Sensing Environ. 85, 1–20. doi:10.1016/s0034-4257(02)00188-8
Monteiro, F. M., Fonseca, M. M., Madeira, M. A., and Herbillon, A. J. (2012). Driving Factors Determining the Occurrence of Sodic Soils in Dry Subhumid Mediterranean Areas. Z. Pflanzenernähr. Bodenk. 175, 94–100. doi:10.1002/jpln.201000056
Mougenot, B., Pouget, M., and Epema, G. F. (1993). Remote Sensing of Salt Affected Soils. Remote Sensing Rev. 7, 241–259. doi:10.1080/02757259309532180
Munns, R., Passioura, J. B., Colmer, T. D., and Byrt, C. S. (2019). Osmotic Adjustment and Energy Limitations to Plant Growth in saline Soil. New Phytol. 225, 1091–1096. doi:10.1111/nph.15862
Nadeem, M. Y., Umar, W., Chattha, M. U., Khan, I., Safeer, M., and Saif, M. A. (2017). Kallar Grass “The Salt Grass” an Important Fodder Crop to Ameliorate Salt-Effected Soils. 05 – TechnologyTimes.Pk. Available at: https://www.adssc/Downloads/KallarGrass.pdf.
NDSU Extension Service (2014). Saline and Sodic Soils. Fargo: North Dakota State University. Available at: https://www.ndsu.edu/soilhealth/wp-content/uploads/2014/07/Saline-and-Sodic-Soils-2-2.pdf.
NDSU Extension Service (). Subsurface Tile Drainage. Fargo: North Dakota State University. Available at: https://www.ag.ndsu.edu/waterquality/documents/Tile%20drainage%20brochure%20-14.pdf.
Nield, S. J., Boettinger, J. L., and Ramsey, R. D. (2007). Digitally Mapping Gypsic and Natric Soil Areas Using Landsat ETM Data. Soil Sci. Soc. Am. J. 71, 245–252. doi:10.2136/sssaj2006-0049
Noborio, K. (2001). Measurement of Soil Water Content and Electrical Conductivity by Time Domain Reflectometry: a Review. Comput. Elect. Agric. 31, 213–237. doi:10.1016/s0168-1699(00)00184-8
Pannell, D. J. (2001). Dryland Salinity: Economic, Scientific, Social and Policy Dimensions. Aust. J. Agric. Resource Econ. 45, 517–546. doi:10.1111/1467-8489.00156
Paz, A., Hristov, B., Amezketa, E., Falsone, G., Zambujo, J., Canfora, L., et al. (2020). Minipaper: Prevention, Mitigation and Adaptation Strategies for Soil Salinisation at Farm Level. Brussels: EIP-AGRI Focus Group Soil salinization. Available at: https://ec.europa.eu/eip/agriculture/sites/agri-eip/files/fg36_minipaper_preventinomitigationadaptation_2020_en.pdf.
Peng, J., Ji, W., Ma, Z., Li, S., Chen, S., Zhou, L., et al. (2016). Predicting Total Dissolved Salts and Soluble Ion Concentrations in Agricultural Soils Using Portable Visible Near-Infrared and Mid-infrared Spectrometers. Biosyst. Eng. 152, 94–103. doi:10.1016/j.biosystemseng.2016.04.015
Priori, S., Fantappič, M., Magini, S., and Costantini, E. A. C. (2013). Using the ARP-03 for High-Resolution Mapping of Calcic Horizons. Int. Agrophys. 27, 313–321. doi:10.2478/v10247-012-0100-0
Qadir, M., Oster, J. D., Schubert, S., Noble, A. D., and Sahrawat, K. L. (2007). Phytoremediation of Sodic and Saline‐Sodic Soils. Adv. Agron. 96, 197–247. doi:10.1016/s0065-2113(07)96006-x
Rahman, M. M., Penny, G., Mondal, M. S., Zaman, M. H., Kryston, A., Salehin, M., et al. (2019). Salinization in Large River Deltas: Drivers, Impacts and Socio-Hydrological Feedbacks. Water Security 6, 100024. doi:10.1016/j.wasec.2019.100024
Reid, R., and Hayes, J. (2003). Mechanisms and Control of Nutrient Uptake in Plants. Int. Rev. Cytol. 229, 73–114. doi:10.1016/s0074-7696(03)29003-3
Rhoades, J. D., Manteghi, N. A., Shouse, P. J., and Alves, W. J. (1989). Estimating Soil Salinity from Saturated Soil-Paste Electrical Conductivity. Soil Sci. Soc. America J. 53, 428–433. doi:10.2136/sssaj1989.03615995005300020019x
Rodríguez Rodríguez, A. Í., Gonz Soto, M. Á., Hern Hernndez, L. Á., Jim Mendoza, C. É., Ortega González, M. J., Padr Padrón, P. Ó., et al. (1993). Assessment of Soil Degradation in the Canary Islands (Spain). Land Degrad. Dev. 4, 11–20.
Rogers, M. E., Craig, A. D., Munns, R. E., Colmer, T. D., Nichols, P. G. H., Malcolm, C. V., et al. (2006). Corrigendum to: The Potential for Developing Fodder Plants for the Salt-Affected Areas of Southern and Eastern Australia: an Overview. Aust. J. Exp. Agric. 46, 1665. doi:10.1071/ea04020_co
Samouëlian, A., Cousin, I., Tabbagh, A., Bruand, A., and Richard, G. (2005). Electrical Resistivity Survey in Soil Science: A Review. Soil Tillage Res. 83, 173–193. doi:10.1016/j.still.2004.10.004
Scasta, J. D., Trostle, C. L., and Foster, M. A. (2012). Evaluating Alfalfa (Medicago Sativa L.) Cultivars for Salt Tolerance Using Laboratory, Greenhouse and Field Methods. J. Agric. Sci. 4, 90–103. doi:10.5539/jas.v4n6p90
Scudiero, E., Skaggs, T. H., and Corwin, D. L. (2014). Regional Scale Soil Salinity Evaluation Using Landsat 7, Western San Joaquin Valley, California, USA. Geoderma Reg. 2-3, 82–90. doi:10.1016/j.geodrs.2014.10.004
Scudiero, E., Teatini, P., Corwin, D. L., Deiana, R., Berti, A., and Morari, F. (2013). Delineation of Site-specific Management Units in a saline Region at the Venice Lagoon Margin, Italy, Using Soil Reflectance and Apparent Electrical Conductivity. Comput. Elect. Agric. 99, 54–64. doi:10.1016/j.compag.2013.08.023
Shahid, S. A., Zaman, M., and Heng, L. (2018a). “Introduction to Soil Salinity, Sodicity and Diagnostics Techniques,” in Guideline for Salinity Assessment, Mitigation and Adaptation Using Nuclear and Related Techniques. Editors M. Zaman, S.A. Shahid, and L. Heng (Vienna: International Atomic Energy Agency), 1–42. doi:10.1007/978-3-319-96190-3_1
Shahid, S. A., Zaman, M., and Heng, L. (2018b). “Salinity and Sodicity Adaptation and Mitigation Options,” in Guideline for Salinity Assessment, Mitigation and Adaptation Using Nuclear and Related Techniques. Editors M. Zaman, S. A. Shahid, and L. Heng (Vienna: International Atomic Energy Agency), 55–89. doi:10.1007/978-3-319-96190-3_3
Sharma, D. K., Chaudhari, S. K., and Singh, A. (2014). In Salt Affected Soils Agroforestry Is a Promising Option. Indian Farming 63, 19–22.
Shrivastava, P., and Kumar, R. (2015). Soil Salinity: A Serious Environmental Issue and Plant Growth Promoting Bacteria as One of the Tools for its Alleviation. Saudi J. Biol. Sci. 22, 123–131. doi:10.1016/j.sjbs.2014.12.001
Singh, A. (2015). Soil Salinization and Waterlogging: A Threat to Environment and Agricultural Sustainability. Ecol. Indicators 57, 128–130. doi:10.1016/j.ecolind.2015.04.027
Singh, G. (1995). An Agroforestry Practice for the Development of Salt Lands usingProsopis Juliflora andLeptochloa Fusca. Agroforest Syst. 29, 61–75. doi:10.1007/bf00711282
Singh, K., Pandey, V. C., Singh, B., and Singh, R. R. (2012). Ecological Restoration of Degraded Sodic Lands through Afforestation and Cropping. Ecol. Eng. 43, 70–80. doi:10.1016/j.ecoleng.2012.02.029
Soil Survey Staff (1954). Diagnosis and Improvement of saline and Alkali Soils. Agriculture Handbook, U.S. Department of Agriculture. Nat. Resour. Conservation Serv. 60, 83–100. doi:10.1093/aibsbulletin/4.3.14-a
Soil Survey Staff (2014). “Kellogg Soil Survey Laboratory Methods Manual,” in Soil Survey Investigations Report No. 42, Version 5.0. R. Soil Survey Staff (Washington, DC: U.S. Department of Agriculture, Natural Resources Conservation Service).
Stavi, I. (2020). On-site Use of Plant Litter and Yard Waste as Mulch in Gardening and Landscaping Systems. Sustainability 12, 7521. doi:10.3390/su12187521
Stavi, I., Shem-Tov, R., Ragolsky, G., and Lekach, J. (2017). Ancient to Recent-Past Runoff Harvesting Agriculture in Recharge Playas of the Hyper-Arid Southern Israel. Water 9, 991. doi:10.3390/w9120991
Sudduth, K. A., Kitchen, N. R., Bollero, G. A., Bullock, D. G., and Wiebold, W. J. (2003). Comparison of Electromagnetic Induction and Direct Sensing of Soil Electrical Conductivity. Agron.j. 95, 472–482. doi:10.2134/agronj2003.4720
Sverdrup, H., and Warfvinge, P. (1988). Weathering of Primary Silicate Minerals in the Natural Soil Environment in Relation to a Chemical Weathering Model. Water Air Soil Pollut. 38, 387–408.
Taghadosi, M. M., Hasanlou, M., and Eftekhari, K. (2019). Retrieval of Soil Salinity from Sentinel-2 Multispectral Imagery. Eur. J. Remote Sensing 52, 138–154. doi:10.1080/22797254.2019.1571870
Tal, A. (2016). Rethinking the Sustainability of Israel's Irrigation Practices in the Drylands. Water Res. 90, 387–394. doi:10.1016/j.watres.2015.12.016
Thenkabail, P. S. (2010). Global Croplands and Their Importance for Water and Food Security in the Twenty-First century: Towards an Ever green Revolution that Combines a Second green Revolution with a Blue Revolution. Remote Sensing 2, 2305–2312. doi:10.3390/rs2092305
Vargas, R., Pankova, E. I., Balyuk, S. A., Krasilnikov, P. V., and Khasankhanova, G. M. (2018). Handbook for saline Soil Management. Rome: Food and Agriculture organization of the United Nations. Available at: http://www.fao.org/3/i7318en/I7318EN.pdf.
Verhulst, N., Govaerts, B., Verachtert, E., Kienle, F., Limon-Ortega, A., Deckers, J., et al. (2009). The Importance of Crop Residue Management in Maintaining Soil Quality in Zero Tillage Systems; A Comparison between Long-Term Trials in Rainfed and Irrigated Wheat Systems. New Delhi: 4th World Congress on Conservation Agriculture. Available at: https://core.ac.uk/download/pdf/34474248.pdf.
Verma, K. S., Saxena, R. K., Barthwal, A. K., and Deshmukh, S. N. (1994). Remote Sensing Technique for Mapping Salt Affected Soils. Int. J. Remote Sensing 15, 1901–1914. doi:10.1080/01431169408954215
Viscarra Rossel, R. A., Adamchuk, V. I., Sudduth, K. A., McKenzie, N. J., and Lobsey, C. (2011). Proximal Soil Sensing: an Effective Approach for Soil Measurements in Space and Time. Adv. Agron. 113, 243–291. doi:10.1016/b978-0-12-386473-4.00005-1
Viscarra Rossel, R., McBratney, A. B., and Minasny, B. (2010). Proximal Soil Sensing. Springer Science & Business Media.
Walker, D. J., Lutts, S., Sánchez-García, M., and Correal, E. (2014). Atriplex Halimus L.: Its Biology and Uses. J. Arid Environments 100-101, 111–121. doi:10.1016/j.jaridenv.2013.09.004
Wang, J., Ding, J., Yu, D., Ma, X., Zhang, Z., Ge, X., et al. (2019). Capability of Sentinel-2 MSI Data for Monitoring and Mapping of Soil Salinity in Dry and Wet Seasons in the Ebinur Lake Region, Xinjiang, China. Geoderma 353, 172–187. doi:10.1016/j.geoderma.2019.06.040
Wang, Q., Li, P., and Chen, X. (2012). Modeling Salinity Effects on Soil Reflectance under Various Moisture Conditions and its Inverse Application: A Laboratory experiment. Geoderma 170, 103–111. doi:10.1016/j.geoderma.2011.10.015
Ward, M., Jones, R., Brender, J., de Kok, T., Weyer, P., Nolan, B., et al. (2018). Drinking Water Nitrate and Human Health: An Updated Review. Ijerph 15, 1557. doi:10.3390/ijerph15071557
Welle, P. D., and Mauter, M. S. (2017). High-resolution Model for Estimating the Economic and Policy Implications of Agricultural Soil Salinization in California. Environ. Res. Lett. 12, 094010. doi:10.1088/1748-9326/aa848e
Whipkey, C. E., Capo, R. C., Chadwick, O. A., and Stewart, B. W. (2000). The Importance of Sea spray to the Cation Budget of a Coastal Hawaiian Soil: a Strontium Isotope Approach. Chem. Geology. 168, 37–48. doi:10.1016/s0009-2541(00)00187-x
Wichelns, D., and Qadir, M. (2014). Achieving Sustainable Irrigation Requires Executive Management of Salts, Soil Salinity, and Shallow Groundwater. Agric. Water Manag. 157, 31–38. doi:10.1016/j.agwat.2014.08.016
Withers, P., Neal, C., Jarvie, H., and Doody, D. (2014). Agriculture and Eutrophication: Where Do We Go from Here?. Sustainability 6, 5853–5875. doi:10.3390/su6095853
Wu, W. (2019). “A Brief Review on Soil Salinity Mapping by Optical and Radar Remote Sensing,” in Research Developments In Saline Agriculture (Singapore: Springer), 53–65. doi:10.1007/978-981-13-5832-6_2
Wu, W., Zucca, C., Muhaimeed, A. S., Al-Shafie, W. M., Fadhil Al-Quraishi, A. M., Nangia, V., et al. (2018). Soil Salinity Prediction and Mapping by Machine Learning Regression in Central Mesopotamia, Iraq. Land Degrad. Dev. 29, 4005–4014. doi:10.1002/ldr.3148
Zhao, D., Arshad, M., Li, N., and Triantafilis, J. (2021). Predicting Soil Physical and Chemical Properties Using vis-NIR in Australian Cotton Areas. Catena 196, 104938. doi:10.1016/j.catena.2020.104938
Zinck, J. A., and Metternicht, G. (2009). “Soil Salinity and Salinization hazard. Remote Sensing of Soil Salinization,” in Remote Sensing of Soil Salinization: Impact on Land Management. Editors J.A. Zinck, and G. Metternicht. 1st Ed. (Boca Raton: CRC Press), 3–18.
Zornoza, R., Guerrero, C., Mataix-Solera, J., Scow, K. M., Arcenegui, V., and Mataix-Beneyto, J. (2008). Near Infrared Spectroscopy for Determination of Various Physical, Chemical and Biochemical Properties in Mediterranean Soils. Soil Biol. Biochem. 40, 1923–1930. doi:10.1016/j.soilbio.2008.04.003
Keywords: climate change, crop selection, drought stress, environmental sustainability, precision agriculture, primary salinity and sodicity, secondary salinity and sodicity, water logging
Citation: Stavi I, Thevs N and Priori S (2021) Soil Salinity and Sodicity in Drylands: A Review of Causes, Effects, Monitoring, and Restoration Measures. Front. Environ. Sci. 9:712831. doi: 10.3389/fenvs.2021.712831
Received: 21 May 2021; Accepted: 26 July 2021;
Published: 11 August 2021.
Edited by:
Lu-Jun Li, Northeast Institute of Geography and Agroecology (CAS), ChinaReviewed by:
Manoj Kumar Jhariya, Sant Gahira Guru Vishwavidyalaya, IndiaZhichun Wang, Northeast Institute of Geography and Agroecology (CAS), China
Jagdish Chander Dagar, Indian Council of Agricultural Research (ICAR), India
Copyright © 2021 Stavi, Thevs and Priori. This is an open-access article distributed under the terms of the Creative Commons Attribution License (CC BY). The use, distribution or reproduction in other forums is permitted, provided the original author(s) and the copyright owner(s) are credited and that the original publication in this journal is cited, in accordance with accepted academic practice. No use, distribution or reproduction is permitted which does not comply with these terms.
*Correspondence: Ilan Stavi, aXN0YXZpQGFkc3NjLm9yZw==