- 1School of Environment, Harbin Institute of Technology, Harbin, China
- 2Guangdong Provincial Key Laboratory of Soil and Groundwater Pollution Control, School of Environmental Science and Engineering, Southern University of Science and Technology, Shenzhen, China
- 3State Environmental Protection Key Laboratory of Integrated Surface Water-Groundwater Pollution Control, School of Environmental Science and Engineering, Southern University of Science and Technology, Shenzhen, China
- 4Department of Sciences, University of Roma Tre, Rome, Italy
- 5S.S. Papadopulos and Associates, Inc., Rockville, MD, United States
China is facing frequent waterlogging and an increasing water scarcity that mirrors the fast urban and economic expansion of the last 4 decades. To mitigate these issues, the government promulgated the “Sponge City” strategy; a concept rooted in practices in western countries aimed at collecting and reusing 65–90% of urban rainfall. The application consists of absorbent infrastructures such as green roofs and rain gardens combined with the pre-existing urban environment. However, due to climate heterogeneities and the different urbanization contexts in China, these goals may seem overly ambitious in many areas of the country. Compact urbanization, together with heavy rainfall concentrated in short events, puts dramatic stresses on these infrastructures. At the same time, overdesigned infrastructures are expensive and may not be practical to retrofit in existing urban areas. In this paper, the role of urban aquifers as natural Sponge City elements are investigated throughout China. The method of implementation is inexpensive and easy to apply, favoring the direct infiltration to the subsoil after the conversion of the urban surfaces from impervious to permeable. Infiltration to urban aquifers alleviates the pressure on sewers, urban streams, as well as waste-water treatment plants. Considering urban aquifers with different hydraulic characteristics, water table dynamics after large infiltration events from rainfall are simulated via numerical analysis. Hydrogeological and geomorphological analyses are carried out to individuate criteria for the mapping of high absorbance areas at the regional and local scales. A Sponge City approach involving the urban aquifers can represent a winning formula for the success of this ambitious but compelling plan.
Introduction
Waterlogging and water scarcity are severe issues as highlighted in the 2020 World Water Development Report (WWDR, 2020). Climate change projections indicate with high confidence that extreme precipitation events and heatwaves will become globally more intense and frequent, increasing flood and drought risks (Polade et al., 2014). Adaptation and mitigation are two complementary strategies for managing and reducing the risks of climate change on water resources. Engineering and technological options, as well as social and institutional measures, can transform the climate change from risk to opportunity (WWDR, 2020).
In China, the fast urbanization of the last decades is a concurrent cause to the serious water problems the country is facing (Zheng et al., 2010; Liu et al., 2013; Zheng and Gorelick, 2015, Hua et al., 2020). Megacities are distributed along the eastern coastline and the main river valleys (Figure 1A); due to the dry climate and related water scarcity, internal sectors are less populated. Climate is also responsible for the different water management practices between the northern and the southern parts of China. Temperate to tropical areas in the south mitigate the dry periods by collecting the copious summer rainfall via an extended system of dams and artificial basins (Chen et al., 2016); the drier plains in the north rely on the exploitation of the decreasing groundwater resources (Liu et al., 2008; Wang et al., 2018). Differences in water management strategies also reflect the distribution of the Gross Domestic Product (GDP) that varies considerably among the provinces (Figure 1B). Despite the climate and economic diversities, a significant amount of water is concentrated in Chinese cities and megacities as a result of impervious areas and external sources of water (Liu et al., 2013; Zhao et al., 2015). Pristine resources from outside satisfy the water demand and later flow to sewers, WWTPs (Waste-Water Treatment Plants), and urban channels. Flow from rainfalls and storms represent a transient but significant increment of the urban runoff as buildings, roads and other urban surfaces are impervious. In fact, more than climate, the waterlogging risk (Figure 1C) reflects the concentration of urban centers.
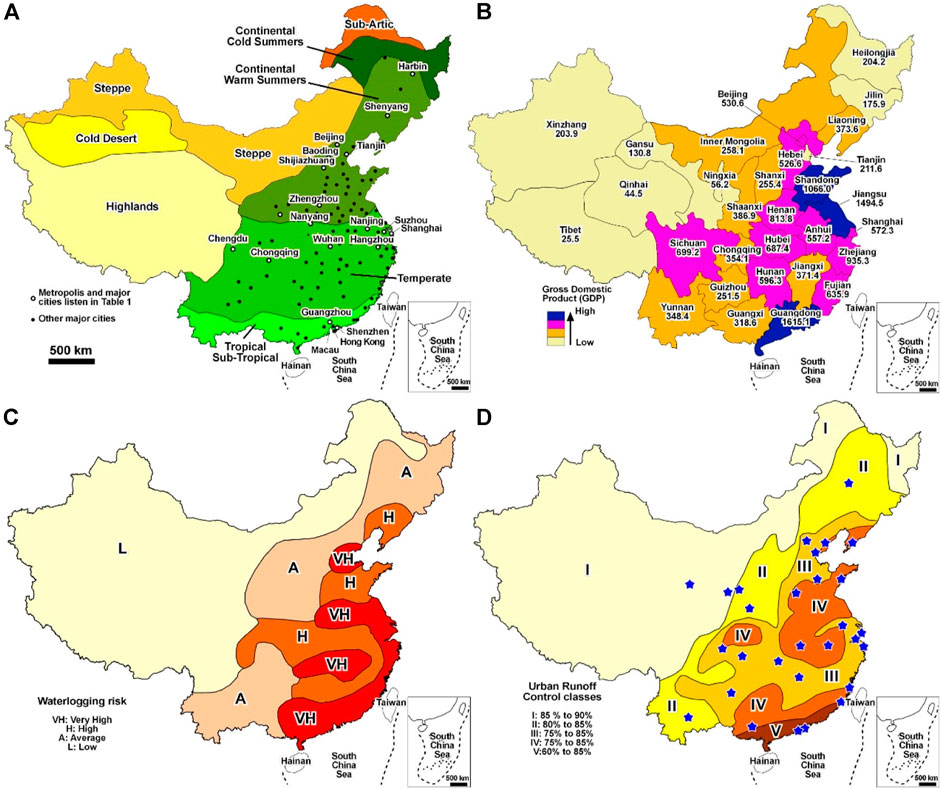
FIGURE 1. Climate distribution of China and major cities (A); province Gross Domestic Product [$] (B); waterlogging risk (C); urban runoff control classification according to MOHUD (2014) with mandated percentage goals for collection and reuse, blue stars indicate the Sponge City pilot areas (D).
To mitigate the waterlogging risk and simultaneously tackle the water scarcity, the Chinese government promulged the Sponge City concept. Sponge City is based on the western strategies and practice known as Low Impact Development (in United.States and Canada), Best Management Practices, Sustainable Drainage System (United Kingdom), or Water Sensitive City (Australia) (Fletcher et al., 2015; Huang et al., 2020). All these practices slow and attenuate the flow of storm water from buildings and infrastructures. Sponge City is ambitious (Randall et al., 2019); current guidelines (MOHURD-Ministry of Housing and Urban Rural Development, 2014) mandate the collection and reuse of a significant amount of the urban rainfall according to the climate characteristics (Figure 1D). Sponge City projects have already been started in 30 cities and megacities with several pilot sites and an enlargement to other cities from 2020 to 2030 (SC-State Council, 2015; Xia et al., 2017). The concept consists of a combination of facilities collecting stormwater, connected to pipes and tanks (Figure 2A), that is later reused after treatment at theWWTPs. Facilities also aim to restore the ecological functions of urban rivers and streams, modernize the urban architecture, and provide economic advantages (Nguyen et al., 2019). Sponge City can represent a cure-all strategy but requires huge investments, especially at the long-term scale. Beside the installation costs, the treatment of collected water, gardening and pipe maintenance is expensive and Sponge City retrofitting with pipes and tanks is challenging in urban areas.
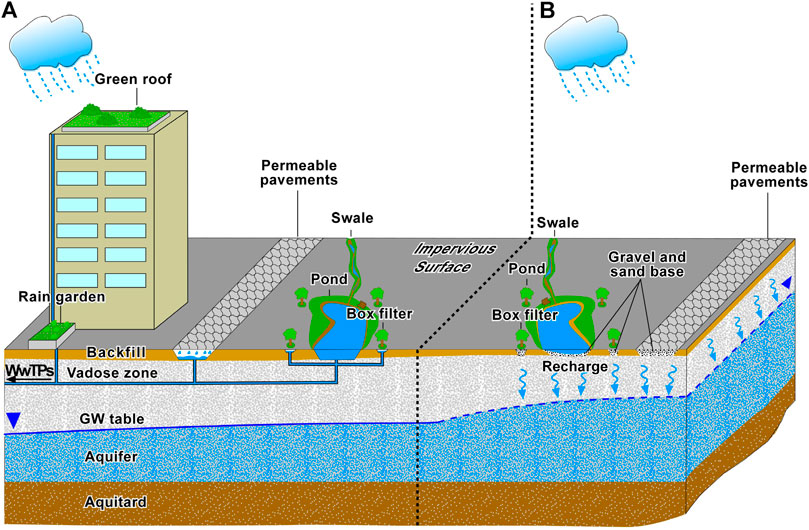
FIGURE 2. Classic Sponge City design with water collected via pipes and tanks (A); infiltration-based installations, backfill continuity is interrupted by a base of gravel and sand favoring infiltration (B).
For an improvement of the Sponge City concept, it is proposed that urban aquifers be used as natural Sponge City tanks where there is sufficient infiltration potential (Figure 2B). In fact, the impervious coverage from pavements and infrastructures blocks the natural infiltration and recharge to urban aquifers, which are typically only recharged via leakage of pipes and tanks, irrigation excess or rainfall infiltration from gardens and flowerbeds (Lerner, 2002). Infiltration-based installations are cheaper as the installation and setup of subterranean pipes and tanks, infrastructures monitoring, and the treatment of collected water can be avoided. Only a layer of sand and gravel in contact with the vadose zone is required to by-pass the less permeable backfill (Figure 2B).
The absorbance capacity of aquifers is heterogeneous and depends on the local hydrogeological features that are influenced by geology and geomorphology. However, the impacts of the spatial heterogeneity on the development of Sponge City are poorly understood.
The objectives of this paper are 1) investigate in the urban context the water table dynamics via numerical methods (Niswonger et al., 2011) after a copious rainfall; 2) perform a regional scale analyses to classify the urban aquifers of China; 3) point out the most profitable infiltration-based approach that can be used to improve the cost-benefit ratio of Sponge City, with examples from the most populous cities of China.
Materials and Methods
Infiltration Criteria and Investigations
Infiltration-based installations are efficacious in areas with great absorbance capacity. Silty to clayey soils can absorb a limited quantity of water while greater amounts run off to streams and channels. On the contrary, average to high permeability soils, such as silty sands and gravels, have the capacity to infiltrate a large amount of rain, transferring the water to the aquifers, via the vadose zone (Figure 2B). Hydraulic conductivity of shallow soils is a parameter easy to characterize, even in the urban areas where local heterogeneities can locally block the infiltration process. The storage capacity and groundwater dynamics of urban aquifers are more complicated. The location of the groundwater table is the second fundamental parameter for assessing the storage capacity of an urban aquifer. Infiltration triggers a rise of the groundwater table, inversely proportional to the specific yield (Figure 2B).
High permeability soils with a deep groundwater table are not ubiquitous. The bedrock in the mountainous and hilly terrains is generally impervious, favoring runoff and soil erosion due to low rainfall infiltration on the bedrock outcrops. At the regional scale, alluvial sequences have a fining sequence of sediments and hydraulic conductivity decreases moving downstream, as rivers and streams progressively lose their capacity to transport the eroded material (Freeze and Cherry, 1979). However, alluvial plains and coastal areas generally have a shallow groundwater table near the ground surface. Mapping suitable areas at regional scale is not trivial. The classical approach consists of field investigations and modelling (Brassington, 2007). Hydrogeological maps and three-dimensional models characterize the volume and hydraulic conductivity of soils; water wells and piezometers monitor the groundwater table; and numerical modelling can simulate the groundwater table under different conditions (Anderson et al., 2015). Analysis of satellite images record the urban expansion providing an overview of the hydrogeological zones converted from rural to urban. A great amount of data and long-term observations are required.
At regional scale, the classical investigation approach is not practical, because of the huge amount of data required A numerical analysis of an urban aquifer connected to a drain is used to understand water-table dynamics as a function of specific yield and the hydraulic conductivity following a large infiltration event. Considering the numerical results, the hydrogeological complexes of China are reinterpreted as a function of their infiltration and storage capacity. The resulting conceptual model is applied and discussed for the seventeen most populous megacities of China, highlighting their absorbance capacity at the regional scale. Major cities suitable for infiltration-based installations (Figure 2B) are identified.
Flow Model Construction
A two-dimensional numerical model was constructed using MODFLOW-NWT code (Niswonger et al., 2011) with the GMS® graphical interface to investigate water-table dynamics following an infiltration event. The model domain is discretized with square cells, with a size of 2 m. The model represents a homogeneous aquifer with a base of 200 m, discharging the groundwater flow in a stream or drain located at 10 m a.s.l. Drain is introduced via the Drain Package (DRN), with a hydraulic conductance of 0.001 m2/s. First stress period, in steady-state conditions, has a null infiltration and simulates dry periods. Second stress period simulates a huge rainstorm with a length of 1 h and an infiltration from the top layer of 200 mm (CMA, China Metereological Administration, 2013), via Recharge Package (RCH). Remaining 14 stress periods have a length of 1 day and null infiltration. Unsaturated Zone Flow Package (UZF) is used to simulate the water table rise through the dry cells located above the water table. Three soils with decreasing horizontal hydraulic conductivity and specific yield are considered: soil 1 (Kh = 1•10−4 m/s and Sy = 0.3); soil 2 (Kh = 1•10−5 m/s and Sy = 0.25); soil 3, (Kh = 1•10−6 m/s and Sy = 0.2). Soil range represents aquifers from coarse sand to a silty sand. Figure 3 schematizes the numerical model. Vertical hydraulic conductivity is set equal to 1•10–4 m/s to simulate the rise of the water table in undrained conditions. This condition is precautionary as is assumed that all the water is absorbed by the soil before the seepage processes start.
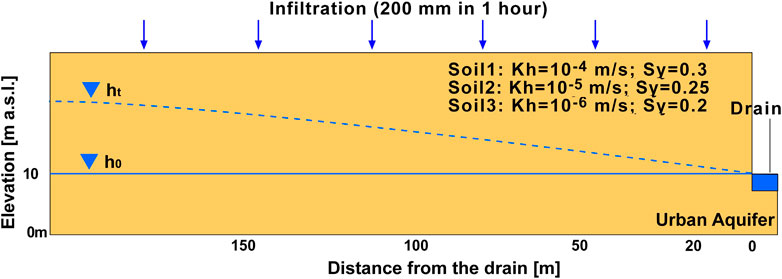
FIGURE 3. Schematic of the numerical model; h0 indicates the water table in steady-state conditions, ht the water table during the seepage.
Hydrogeology of China
The hydrogeology of China reflects the complex geological evolution of the area. Pre-Cambrian to Mesozoic bedrock sequences, outcropping throughout the mountain chains, alternate with Tertiary to Quaternary basins filled with unconsolidated sediment (Yin, 2006; Li et al., 2014; Zhang et al., 2020). For the sake of simplicity, eight hydrogeological units are used to describe the hydrogeology of China as shown on Figure 4 and as described below:
-The alluvial sequence unit (1) fills the Neogene-Quaternary basins and consists in alternation of fine and coarse soils, deposited in a fluvial to lacustrine environment. From a hydrogeological point of view, the unit is a multi-layer aquifer with a high hydraulic conductivity and high specific yield.
-The piedmont unit (2) lies between the alluvial sequence and bedrock units and consists in a thick accumulation of coarse sediments, mainly from rivers. It is considered as a single aquifer, with a high hydraulic conductivity and high specific yield.
-The delta unit (3) consists of an accumulation of fine Quaternary sediments, mainly silt and clay, arranged in a multi-layer aquifer. Because of the high content of clay, the hydraulic conductivity and high specific yield is average to low.
-The loess unit (4) is a thick accumulation of Quaternary eolian deposits, forming an extended plateau in the central sector of the Yellow River. Sequence has a dominant silty and loamy texture that confers an average hydraulic conductivity and an average specific yield.
-The karst unit (5) consists in thick carbonate sequences from Pre-Cambrian to Mesozoic. Tropical to sub-tropical areas show mature karst landforms with large water-level responses to seasonal recharge. In cold and dry climates, karst processes are not well-developed because of the low precipitation and, secondarily, because carbonate sequences are generally overlain by less permeable Paleozoic shales or Quaternary sediments. Hydraulic conductivity is variable from average to very high.
-The fractured bedrock unit (6) and impervious bedrock unit (7) characterize the mountain sectors. Hydraulic conductivity depends on the fracture density that is related to the tectonic activity. Slopes are covered by weathering blankets representing a shallow aquifer. Thickness and hydraulic conductivity of these aquifers vary according to climate, lithology and morphology. Warm-humid tropical areas have weathered blankets tens of meter thick consisting of clayey sands. In cold and dry areas, the weathered blanket is typically only a few meters thick with sandy gravel, detritus and bedrock blocks.
-The permafrost unit (8) is constituted by bedrock with a very low to low hydraulic conductivity because of the frozen terrains. Unit extensively outcrops throughout the Qinghai-Tibet Plateau.
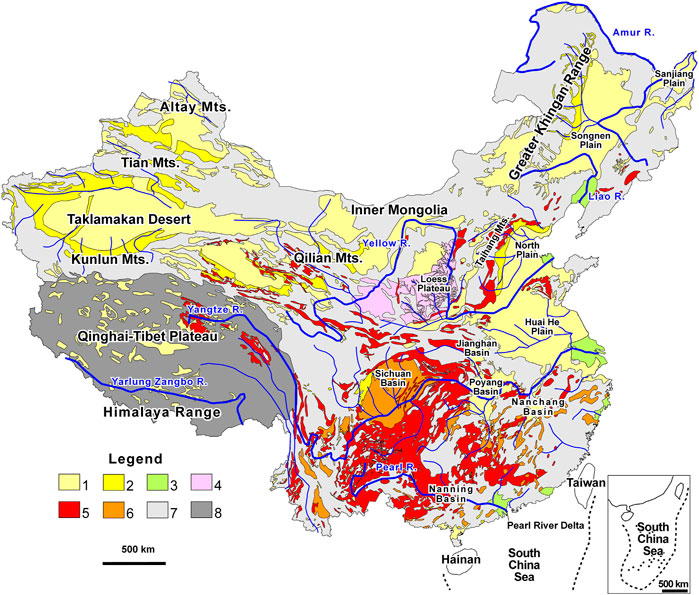
FIGURE 4. Hydrogeological map of China. Key to the Legend: 1) Alluvial sequence; 2) Piedmont bands; 3) Delta 4) Loess; 5) Fissured Bedrock; 6) Karstified carbonate sequence; 7) Impervious bedrock; 8) Permafrost. Redrawn and simplified from CGS (1979).
Results and Discussion
Numerical Results
The model simulates the water table dynamics of an urban aquifer connected to a drain. Parameter of the urban aquifer vary among the three soils. Due to the undrained conditions, the water table instantly rises in the first hour of the simulation (Figure 5). In soil 1 the simulated water-table rise is 0.67 m; in soil 2 is 0.79 m; in soil 3 is 1 m.
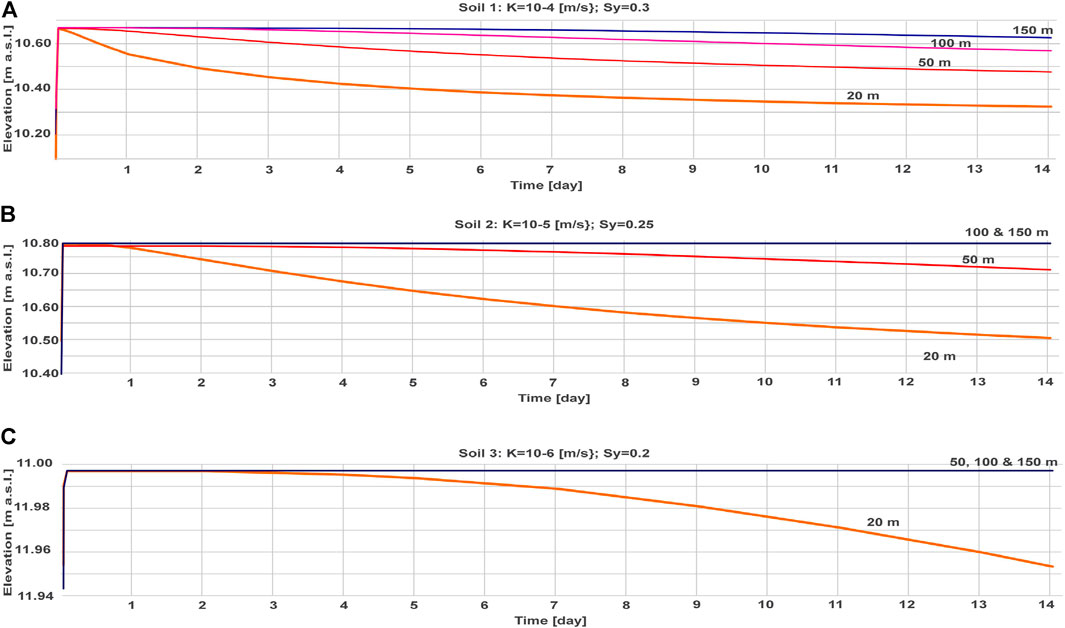
FIGURE 5. Water-level declines in Soil 1 (A) Soil 2 (B), and Soil 3 (C) in the two weeks after the recharge of 200 mm in 1 hour caused by a rainstorm. Water-level hydrographs are presented at 20, 50, 100, and 150 m from the drain.
Following the peak water-table rise, the water table slowly declines. The simulated water levels at 20, 50, 100, and 150 m from the drain are shown on Figure 5. After 14 days and at 20 m from the drain, soil 1 dissipates 46.3% of the initial increment; at 50 m from the dissipation is 30.2%, at 100 m is 12.3% and at 150 m is 3.5% (Figure 5A). Hydraulic characteristics of soil 1 correspond to a coarse medium sand. Soil 1 the piedmont unit (2) and the paleochannels of the alluvial sequence unit (1). If gravel is present, soil becomes more conductive and seepage processes are quicker. At 20 m from the drain, soil 2 dissipates 36.3 % of the initial increment and at 50 m 7.8 %. At 100 m and 150 m from the drain, dissipation is null at the end of the simulation (Figure 5B). Hydraulic characteristics of soil 2 correspond to fine sand to silty sand; investigated soil is representative of the alluvial sequence unit (1). Soil 3 dissipates 0.4 % of the initial increment at 20 m from the drain. At 50, 100 and 150 m from the drain, water table remains steady after 14 days (Figure 5C). Hydraulic characteristics of soil 3 correspond to sandy silt or a mixture of sand, silt and clay. It is representative of the lacustrine sequence of the delta unit (3) and the alluvial sequence unit (1). In case of a higher content of clay, these units present a lower conductivity and less capacity to dissipate the infiltrated water.
Proposed Conceptual Model
Urbanized aquifers are located throughout the Quaternary alluvial sequence. In these large systems tectonically triggered, a remarkable decrease in hydraulic conductivity and sediment size occurs moving downstream as transport capacity of the rivers decreases as the morphology flattens. In accordance with the geomorphological features, characteristics of urban aquifers are reinterpreted according to their absorbance capacity (Figure 6). Absorbance capacity of urbanized fractured and karst sequence is also evaluated.
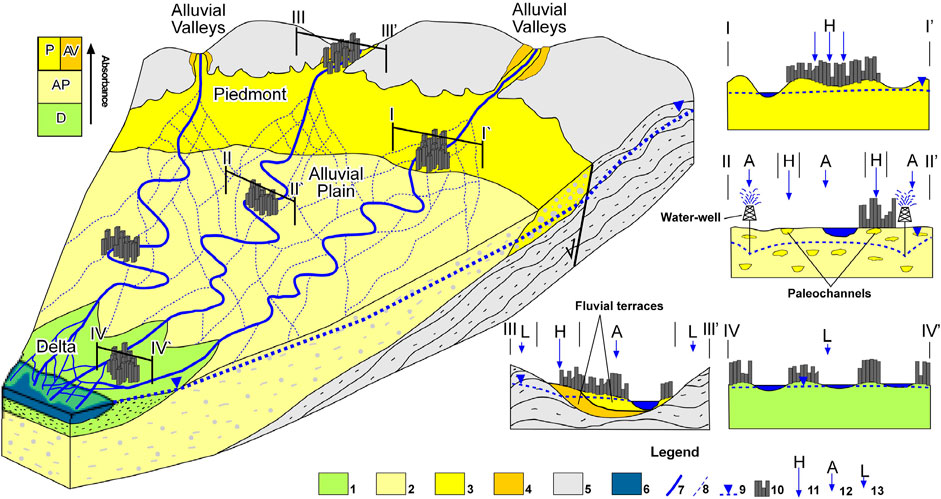
FIGURE 6. Conceptual zonation for rainfall potential at the catchment scale. Key to the legend: Deltaic sequence (1); Alluvial plain sequence (2); Piedmont and recent terrace sequences (3); older terrace sequence (4); bedrock (5); seawater (6); river (7); paleochannels (8); groundwater table (9); urban areas (10); High (11), Average (12) and Low (13) potential absorbance.
The Piedmont
The piedmont connects mountain terrains and plains (Figure 6). It consists of a thick sequence of highly porous and permeable sediments, mainly sand and gravel, accumulated by alluvial and gravitative processes (Bowman, 2017). The sediments on the Piedmont are the product of slope readjustment triggered by changes in the base level of rivers and the sea, induced by tectonic or climate factors at the geologic time scale. The width of the Piedmont can be large and interconnected with alluvial fans. Thickness and width of the piedmont depends on the accumulation space created by tectonics.
In the Songnen, the Huai He, the North Plains, the Sichuan Basin, and the endorheic basins in the northwest the piedmonts are several kilometers wide (Figure 4), hosting numerous cities and megacities (CGS, 1979). Rivers and streams are incised, and their base level is tens of meters lower than the ground level of the cities (Zhang and Fei, 2008). In combination with the highly permeable soils with a small hydraulic gradient throughout the aquifers, these areas are characterized by a high absorbance capacity. Basins and valleys of southern China are smaller because the reduced tectonic activity and sediments are less permeable due to the higher clay content, favored by the warmer weather. However, in tropical climates, flow landslides intersect the weathered blankets increasing the thickness of the piedmont bodies (Lancia et al., 2020b). Compared to the northern basins triggered by tectonics, piedmont bands are less thick, smaller and less permeable but able to absorb the copious runoff of the tropical areas. Lancia et al. (2020a) pointed out the great absorbance capacity of a thin piedmont located in Shenzhen in southern China, under sub-tropical conditions (Figure 1A); Water-level fluctuations on the piedmont indicate a water table that responds rapidly to precipitation, able to absorb the runoff during the storms and transmit water to downstream aquifers. Performed numerical analysis (Figure 5A) confirm the capacity of this unit to dissipate the overpressure after remarkable infiltration. This zone is strategic for managing storm water runoff. Because of the higher costs of construction and management, the piedmont is generally urbanized only at the end of the expansion process, when flat alluvial plains and valleys are completely developed.
Alluvial Plains and Valleys
The alluvial plains originated from sediments eroded throughout the mountain sectors, and subsequently transported and deposited by fluvial activity (Freeze and Cherry, 1979). Riverbeds and paleochannels consist of permeable deposits, coarse sand, and gravel, with variable thickness and width. A mixture of less permeable sediments, silty sands to clay and silt, was deposited during the flood events among the riverbeds. Despite the soil heterogeneity, the groundwater table is generally shallow and near ground surface, due to the flat morphology. As observed in the numerical analysis (Figure 5B), to dissipate infiltrated water a consistent number of drains are required, increasing the costs of the infiltration-based installations. As a result, these areas have a reduced absorbance potential. The main basins of southern China are traversed by major rivers that contribute to keep the groundwater levels steady.
Anthropogenic activities of the last decades depleted the local groundwater resources, depressing the groundwater table. In northern China, the groundwater table is located tens meter from the ground level (Zheng et al., 2010; Cao et al., 2013). Because of the depressed groundwater table, most of the rivers and streams are seasonally dry and act as natural Sponge City installations, with storm runoff rapidly infiltrating into the aquifer (Figure 6). During urbanization, rivers were deviated, and permeable riverbeds urbanized. The detection and reconversion of those areas via infiltration-based installations would provide substantial benefits. The drainage network naturally changes at the geological time scales, leaving numerous channels buried at different depth throughout the alluvial plain. Paleochannels represent the most important absorbance elements and are developed along old drainage directions. Analysis of satellite images and aerial photos can be used to detect and map the paleochannel, considering the tone, color, and pattern of the image. Paleochannels are subsequently verifiable via direct investigations. Despite their lower resolution, older image datasets are useful as areas are less urbanized. Wu et al. (1996) mapped five series of paleochannels in the North Plain, arranged in 20 paleochannels zones, several of them exceeding 100 km in length.
Delta Regions
Deltas are in proximity of river outlets, consisting of clayey to silty dominated sequences. In China, numerous metropolitan areas, including Shanghai and Guangzhou, are built on these sequences, thanks to the flat morphology and the proximity to the coastline. In wet climates, the water demand typically is satisfied by upstream reservoirs and basins; in dry climates deeper aquifers located several hundred meters below the ground surface are utilized for water supply. Because of the abundant impervious layers and lenses characterizing the sequence, the groundwater table is shallow and near the ground surface. Shallow surface waters and seawater intrusions offset the local groundwater table drawdown triggered by shallow wells. Therefore, absorbance capacity is low at the regional scale (Figure 6). Numerical analysis (Figure 5C) suggest that the dissipation of infiltrated water occurs only in proximity of the drains within two weeks of an infiltration event.
Fractured and Karstified Carbonate Bedrock
Karst and fractured bedrock aquifers are natural sponge city facilities. These systems are characterized by an optimal absorbance capacity. These aquifers occur throughout China (Figure 4) and villages and major cities rely on these for water supply (Daoxian, 1991). Fracturing ratio, mainly related to the tectonic stresses, influences the hydraulic conductivity. Fractured bands are distributed along the main tectonic stresses.
Karst processes have a regional impact on groundwater circulation in the urban environment. In northern China, karst processes coincide with rural mountain reliefs, often interbedded with less permeable soils and naturally preserved from pollution. On the contrary, extensive and continuous karst fields characterize wide portion of southern China, with villages and urban centers built on it. Because most of the rainfall is concentrated in the summer months, episodes of drought in the mountain villages alternate with river floods in the valleys. With a regional groundwater table located even hundreds of meters below the ground surface, cities and villages are built in correspondence of dolinen, polje or other karst features able to retain the storm water for a longer period like a Sponge City facility, thanks to impermeable deposits as terre rosse or bauxite levels (Daoxian, 1991). Thus, even if the absorbance rate and the regional water availability are high, smaller communities can suffer water scarcity and the introduction of above the ground Sponge City facilities is recommended to store a strategic amount of water for exceptional drought events.
Absorbance Capacity of Chinese Megacities
The hydrogeological setting and absorbance capacity of the 17 most populous megacities of China are summarized in Table 1. During the latest urban expansion, Chinese megacities expanded in areas with high hydraulic conductivity soils. In Beijing, the piedmont bands of Taihang Mts. were continuously urbanized and in a minor way in Shijiazhuang and Baoding; in Chonqing, Guangzhou and Shenzhen, the hilly and mountain sectors are the only areas preserved from urbanization. Bounded by morphological barriers, these cities expanded to the piedmonts and later urbanization intensified in the nearby valleys. The recent impermeabilization of high absorbance areas at the megacity scale is a triggering factor for both waterlogging as well as water scarcity. Infiltration-based installations applied at large scale can provide immediate benefits.
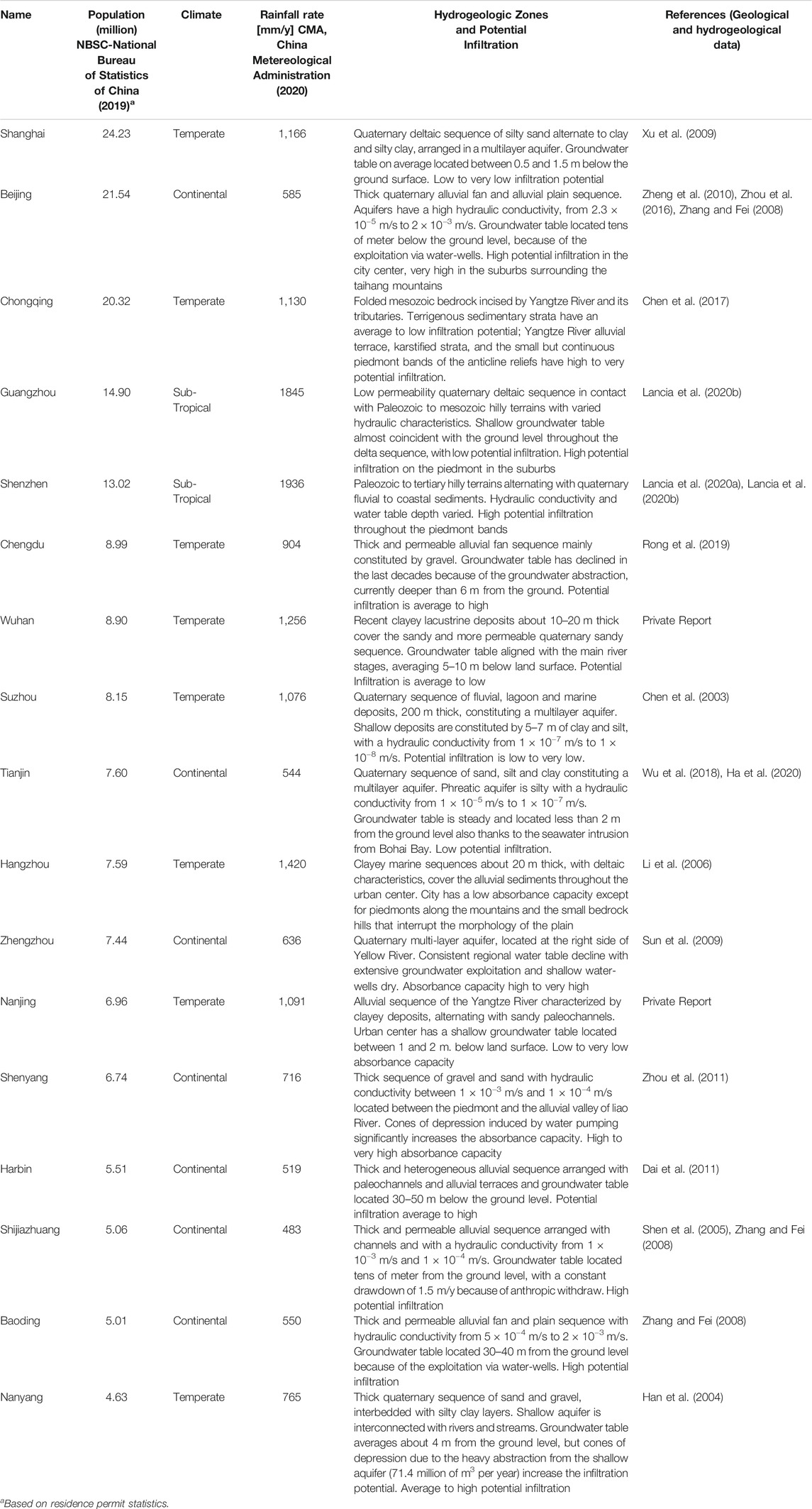
TABLE 1. Hydrogeological characteristics and absorbance capacity of the 17 most populous Chinese cities.
In the wide alluvial plains and valleys (Figure 6), exploitation of the urban aquifers for water supply to meet the insatiable water demand of the megacities amplifies the absorbance capacity. The regional groundwater table of alluvial plains in the megacities of Harbin, Beijing, and Baoding has been significantly depressed and absorbance is high in correspondence of the high permeability terrain. In the recent years, the groundwater table in the southern cities of Chengdu and Nanyang has declined to several meters (Han et al., 2004; Rong et al., 2019) below ground surface because of the increasing water demand; a worrying trend. According to our analysis, only the sub-tropical alluvial plains characterized by a good system of dams and reservoirs have a low absorbance potential.
Megacities built on deltaic deposits have a low absorbance potential because the groundwater table is at or near the ground surface (Figure 7). The absorbance capacity remains low regardless of rainfall rate. Rainfall varies significantly from north to south and is 1,500 mm/y greater at Shenzhen in the south than in Tianjin in the north. The low hydraulic conductivity of the shallower layers and the frequent saline intrusions result in limited exploitation of the urban aquifers. The exploitation of deeper aquifers, related to different paleo-environment, do not have any impact on the absorbance capacity on the shallow aquifer.
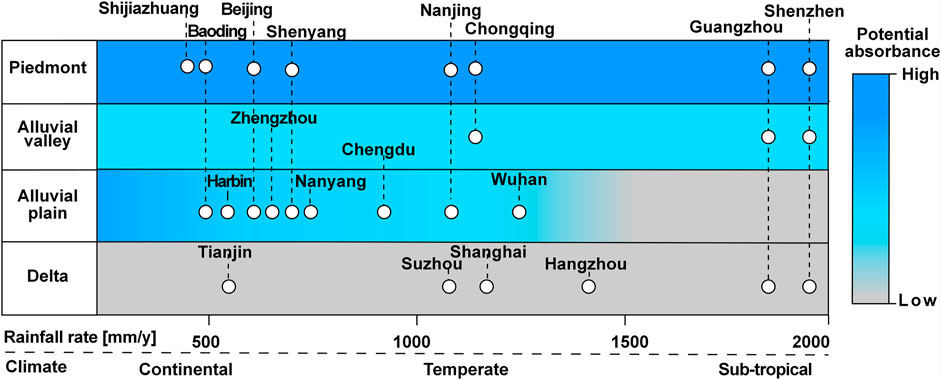
FIGURE 7. Subsoil characteristics and rainfall rate define the absorbance potential at the regional scale. Alluvial valleys fractured to karst bedrock and piedmont have a good potential absorbance at any rainfall rate. The recent exploitation of urban aquifers has increased absorbance potential on the alluvial plains and in the Sichuan Basin at Chengdu. A low potential absorbance characterizes the delta areas in all climatic regimes.
Aquifer Management Optimization
Conductive aquifers are able to dissipate infiltrated water in a reasonable amount of time and the application of infiltration-based installations are profitable (e.g., soil 1, Figure 5A). In less permeable deposits, a denser network of drains can be introduced to favor the dissipation of the infiltrated water, increasing the costs. Recharge with undrained conditions is conservative as it simulates the maximum water table increment associated with a single rainstorm. In fact, it is assumed that all the water infiltrates from the top level to the water table through the vadose zone during the rainstorm. In the practice, soil can absorb the stormwater in a slower amount of time, temporarily flooding the infiltration-based installations. Natural infiltration rates in gravelly to silty loam is estimated by Johnson (1963) between 126.0 and 12.7 cm per hour. If expected infiltration from storms are higher than soil infiltration rate and these installations cannot be flooded, a thicker base of gravel and sand can increase the collection of the water storm. A thicker base, though, would increases the installation and setup cost. Therefore, the thickness of the installation base must be designed according to the climate and the groundwater characteristics.
Hydrogeological analyses, supported by numerical evidence, have identified the infiltration potential in major urban areas in China, according to the hydrogeological and climate feature and the anthropogenic activities (Table 1). These analyses, following the procedures outlined in this study, could be easily expanded to cover other urban areas in China. As absorbance capacity sensibly varies along the catchment (Figure 6), Sponge City plans are optimized if realized at the regional scale. As the infiltration-based installations are inexpensive relative to other Sponge City components, urban areas characterized by a high infiltration capacity should be exploited to their fullest (Lancia et al., 2020a). In small catchments with high rate of urbanization, large use of infiltration-based features also can mitigate the flood risk.
Benefits include the larger available storage capacity that could be economically convenient as it increases the baseflow of streams and rivers in urban areas supported by groundwater. This will result in aesthetic and ecological improvements of the urban environment (Nguyen et al., 2019). The major risk associated with infiltration is that groundwater may become polluted if the storm water is polluted. Although subsoils have the capacity to attenuate pollutants, infiltration should be limited to clean water from roofs, parks, and residential streets. The risks of pollution and chemical spills in major roads and industrial areas is high and connection with aquifers should be avoided (Lancia et al., 2020a).
Increased aquifer recharge can also reduce the contaminant load from sewers. Sewer pipes, located above the groundwater table, tend to exfiltrate pollutants via cracks and fractures. If infiltration-based installations increase the groundwater table over the sewer pipes, flow is inverted and groundwater infiltrates into the sewer preventing the pollutant leakage (Chisala and Lerner, 2008; Su et al., 2020). This beneficial effect is transitory and efficacious just after the rain before the water table declines as groundwater flows to river and streams. However, in megacities with a tropical climate the effect can be long lasting. At the same time, a water table higher than the pressurized pipes also reduces the water loss from the water-supply system, mitigating to an extent water scarcity (Lerner, 2002). According to MOHURD-Ministry of Housing and Urban Rural Development (2014), the water supply systems of China have a water loss rate between 15 and 25%.
Conclusion
The application of the Sponge City concept is an excellent opportunity to address water scarcity and mitigate the waterlogging risk in the urban areas of China. Together with the classic Sponge City elements, numerical and hydrogeological analysis suggest that infiltration-based installations have great potential in many areas of the country. Infiltration-based installations can provide both considerable economic and environment benefits and should be the preferred Sponge City strategy in less developed areas of China, due to the minor costs. Because of the varied lithological, climatic and hydrogeological setting of Chinese urban areas, guidelines developed at the provincial scale, rather than the national scale, can be more efficacious. With the implementation of the suggested methods, based on both climate and infiltration capacity, a more precise definition of the runoff control classes is possible. Performed hydrogeological analysis assess the potential infiltration of the Chinese megacities. The potential infiltration is high along the piedmont or along the alluvial valleys characterized by permeable soils; megacities settled on less permeable delta sequence have a low potential infiltration. The drawdown of the water level, due to anthropic or climate factors, can increment the potential infiltration.
Infiltration-based installations require detailed characterization of the urban aquifers. Preliminary studies, including monitoring of the water table and incremented infiltration scenarios after retrofitting, are essential to optimize the Sponge City plan. According to the outlined criteria, it is possible that the capital and operating costs of the Sponge City facilities can be kept within a manageable range, while at the same time an additional source of water can be provided to reduce water scarcity. The proposed conceptual model also highlights new policies for urban expansions. New urban developments should be prioritized in the low infiltration areas. Urbanization on high infiltration zones should be limited or prohibited.
Data Availability Statement
The original contributions presented in the study are included in the article/Supplementary Material, further inquiries can be directed to the corresponding authors.
Author Contributions
All authors listed have made a substantial, direct, and intellectual contribution to the work and approved it for publication
Funding
The study was supported by the National Key R&D Program of China (project numbers 2016YFC0402806, and 2018YFE0206200), National Natural Science Foundation of China (Grant No. 41861124003); and the High-level Special Funding of the Southern University of Science and Technology (Grant Nos. G02296302, G02296402).
Conflict of Interest
Author CA was employed by the company S.S. Papadopulos and Associates, Inc.
The remaining authors declare that the research was conducted in the absence of any commercial or financial relationships that could be construed as a potential conflict of interest.
References
Anderson, M. P., Woessner, W. W., and Hunt, R. J. (2015). Applied Groundwater Modelling – Simulation of Flow and Advective Transport. 2nd Ed. Amsterdam: Academic Press, Inc. Elsevier, 535.
Cao, G., Zheng, C., Scanlon, B. R., Liu, J., and Li, W. (2013). Use of Flow Modeling to Assess Sustainability of Groundwater Resources in the North China Plain. Water Resour. Res. 49, 159–175. doi:10.1029/2012WR011899
CGS (1979). Chinese Geological Survey, 1. Beijing: Hydrogeological map of People`s Republic of China, scale.
Chen, C., Pei, S., and Jiao, J. (2003). Land Subsidence Caused by Groundwater Exploitation in Suzhou City, China. Hydrogeology J. 11, 275–287. doi:10.1007/s10040-002-0225-5
Chen, J., Shi, H., Sivakumar, B., and Peart, M. R. (2016). Population, Water, Food, Energy and Dams. Renew. Sustain. Energ. Rev. 56, 18–28. doi:10.1016/j.rser.2015.11.043
Chen, K.-L., Wu, H.-N., Cheng, W.-C., Zhang, Z., and Chen, J. (2017). Geological Characteristics of Strata in Chongqing, China, and Mitigation of the Environmental Impacts of Tunneling-Induced Geo-Hazards. Environ. Earth Sci. 76, 10. doi:10.1007/s12665-016-6325-7
Chisala, B. N., and Lerner, D. N. (2008). Distribution of Sewer Exfiltration to Urban Groundwater. Proc. Inst. Civil Eng. - Water Manage. 161 (6), 333–341. doi:10.1680/wama.2008.161.6.333
CMA, China Metereological Administration (2013). Classification Standard of Precipitation Intensity. [in Chinese] Retrieved from http://www.cma.gov.cn/2011xzt/2013zhuant/20130620_3/2013062002/201308/t20130816_223400.html (Accessed June 2020).
CMA, China Metereological Administration (2020). Climate Bulletins of Chinese Cities. [in Chinese] Retrieved from http://www.cma.gov.cn (Accessed June 2020).
Dai, C. L., Li, Z. J., Du, S. M., and Liu, C. H. (2011). Evaluation of Shallow Groundwater Quantity in Harbin by Numerical Modeling. Adv. Mater. Research. 255-260, 2745–2750. doi:10.4028/www.scientific.net/amr.255-260.2745
Fletcher, T. D., Shuster, W., Hunt, W. F., Ashley, R., Butler, D., Arthur, S., et al. (2015). SUDS, LID, BMPs, WSUD and More - the Evolution and Application of Terminology Surrounding Urban Drainage. Urban Water J. 12 (7), 525–542. doi:10.1007/s12665-016-6325-710.1080/1573062x.2014.916314
Ha, D., Zheng, G., Loáiciga, H. A., Guo, W., Zhou, H., and Chai, J. (2020). Long-term Groundwater Level Changes and Land Subsidence in Tianjin, China. Acta Geotech. 16, 1303–1314. doi:10.1007/s11440-020-01097-2
Han, J., Wang, B., and Cao, B. (2004). Division of Groundwater Systems and Significance of Water Supply in Nanyang City, 26. China: Ground Water, 14–16.1 [in Chinese.
Hua, S., Jing, H., Yao, Y., Guo, Z., Lerner, D. N., Andrews, C. B., et al. (2020). Can Groundwater Be Protected from the Pressure of china's Urban Growth? Environ. Int. 143, 105911. doi:10.1016/j.envint.2020.105911
Huang, Y., Tian, Z., Ke, Q., Liu, J., Irannezhad, M., Fan, D., et al. (2020). Nature‐based Solutions for Urban Pluvial Flood Risk Management. WIREs Water 7, e1421. doi:10.1002/wat2.1421
Johnson, A. I. (1963). A Field Method for Measurement of Infiltration. General Ground-Water Techniques. Denver: U.S. Geological Survey Water-Supply Paper.
Lancia, M., Su, H., Tian, Y., Xu, J., Andrews, C., Lerner, D. N., et al. (2020b). Hydrogeology of the Pearl River Delta, Southern China. J. Maps 16 (2), 388–395. doi:10.1080/17445647.2020.1761903
Lancia, M., Zheng, C., He, X., Lerner, D. N., Andrews, C., and Tian, Y. (2020a). Hydrogeological Constraints and Opportunities for “Sponge City” Development: Shenzhen, Southern China. J. Hydrol. Reg. Stud. 28, 100679. doi:10.1016/j.ejrh.2020.100679
Lerner, D. N. (2002). Identifying and Quantifying Urban Recharge: a Review. Hydrogeology J. 10, 143–152. doi:10.1007/s10040-001-0177-1
Li, C., Tang, X., and Ma, T. (2006). Land Subsidence Caused by Groundwater Exploitation in the Hangzhou-Jiaxing-Huzhou Plain, China. Hydrogeol J. 14, 1652–1665. doi:10.1007/s10040-006-0092-6
Li, J., Zhang, Y., Dong, S., and Johnston, S. T. (2014). Cretaceous Tectonic Evolution of South China: A Preliminary Synthesis. Earth-Science Rev. 134, 98–136. doi:10.1016/j.earscirev.2014.03.008
Liu, J., Zang, C., Tian, S., Liu, J., Yang, H., Jia, S., et al. (2013). Water Conservancy Projects in China: Achievements, Challenges and Way Forward. Glob. Environ. Change 23 (3), 633–643. doi:10.1016/j.gloenvcha.2013.02.002
Liu, J., Zheng, C., Zheng, L., and Lei, Y. (2008). Ground Water Sustainability: Methodology and Application to the North China Plain. Groundwater 46 (6), 897–909. doi:10.1111/j.1745-6584.2008.00486.x
MOHURD-Ministry of Housing and Urban Rural Development (2014). Technical Guidelines of the Sponge City Development—Low Impact Development Systems for Storm Water. Beijing: Beijing University of Civil Engineering and Architecture, 86. [in Chinese] Retrieved from http://www.mohurd.gov.cn/wjfb/201411/W020141102041225.pdf
NBSC-National Bureau of Statistics of China (2019). Statistical Bulletin on National Economic and Social Development. [in Chinese] Year 2018. Online report. Available at: http://www.stats.gov.cn/tjsj/ndsj/2019/indexch.htm (Accessed June 2020).
Nguyen, T. T., Ngo, H. H., Guo, W., Wang, X. C., Ren, N., Li, G., et al. (2019). Implementation of Specific Urban Water Management-Sponge City, 652. Science of the Total Environment, 147–162. doi:10.1016/j.scitotenv.2018.10.168
Niswonger, R. G., Panday, S., and Ibaraki, M. (2011). MODFLOW-NWT, A Newton Formulation for MODFLOW-2005. U.S Geological Survey Techniques and Methods, 6-A37.
Polade, S. D., Pierce, D. W., Cayan, D. R., Gershunov, A., and Dettinger, M. D. (2014). The Key Role of Dry Days in Changing Regional Climate and Precipitation Regimes. Scientific Rep. 4, 1–8. doi:10.1038/srep04364
Randall, M., Sun, F., Zhang, Y., and Jensen, M. B. (2019). Evaluating Sponge City Volume Capture Ratio at the Catchment Scale Using SWMM. J. Environ. Manage. 246, 745–757. doi:10.1016/j.jenvman.2019.05.134
Rong, X., Lu, H., Wang, M., Wen, Z., and Rong, X. (2019). Cutter Wear Evaluation from Operational Parameters in EPB Tunneling of Chengdu Metro. Tunnelling Underground Space Tech. 93, 103043. doi:10.1016/j.tust.2019.103043
SC-State Council (2015). The General Office of the State council Advice on Promoting Construction of Sponge Cities. Beijing: GOSCThe State Council, 75. Available at: http://www.gov.cn/zhengce/content/2015-10/16/content_10228.htm.
Shen, Y., Tang, C., Xiao, J., Oki, T., and Kanae, S. (2005). “Effects of Urbanization on Water Resource Development and its Problems in Shijiazhuang, China. Sustainable Water Management Solutions for Large Cities” in Proceedings of Symposium S2 Held during the Seventh IAHS Scientific Assembly at Foz do Iguaçu, Brazil, April 2005. Editors D. A. Savic, M. A. Marino, H. H. Savenije, and J. C. Bertoni (Brazil: IAHS Publ).
Su, X., Liu, T., Beheshti, M., and Prigiobbe, V. (2020). Relationship between Infiltration, Sewer Rehabilitation, and Groundwater Flooding in Coastal Urban Areas. Environ. Sci. Pollut. Res. 27, 14288–14298. doi:10.1007/s11356-019-06513-z
Sun, R., Jin, M., Giordano, M., and Villholth, K. G. (2009). Urban and Rural Groundwater Use in Zhengzhou, China: Challenges in Joint Management. Hydrogeol J. 17 (17), 1495–1506. doi:10.1007/s10040-009-0452-0
Wang, Y., Zheng, C., and Ma, R. (2018). Review: Safe and Sustainable Groundwater Supply in China. Hydrogeol J. 26, 1301–1324. doi:10.1007/s10040-018-1795-1
Wu, C., Xu, Q., Zhang, X., and Ma, Y. (1996). Paleochannels on the North China Plain: Types and Distributions. Geomorphology 18, 5–14.
Wu, Y.-X., Lyu, H.-M., Shen, J. S., and Arulrajah, A. (2018). Geological and Hydrogeological Environment in Tianjin with Potential Geohazards and Groundwater Control during Excavation. Environ. Earth Sci. 77, 1–17. doi:10.1007/s12665-018-7555-7
Xia, J., Zhang, Y., Xiong, L., He, S., Wang, L., and Yu, Z. (2017). Opportunities and Challenges of the Sponge City Construction Related to Urban Water Issues in China. Sci. China Earth Sci. 60, 652–658. doi:10.1007/s11430-016-0111-8
Xu, Y.-S., Shen, S.-L., and Du, Y.-J. (2009). Geological and Hydrogeological Environment in Shanghai with Geohazards to Construction and Maintenance of Infrastructures. Eng. Geology. 109, 241–254. doi:10.1016/j.enggeo.2009.08.009
Yin, A. (2006). Cenozoic Tectonic Evolution of the Himalayan Orogen as Constrained by Along-Strike Variation of Structural Geometry, Exhumation History, and Foreland Sedimentation. Earth-Science Rev. 76 (1-2), 1–131. doi:10.1016/j.earscirev.2005.05.004
Zhang, Z.-K., Ling, M.-X., Lin, W., Sun, M., and Sun, W. (2020). "Yanshanian Movement" Induced by the Westward Subduction of the Paleo-Pacific Plate. Solid Earth Sci. 5 (2), 103–114. doi:10.1016/j.sesci.2020.04.002
Zhang, Z., and Fei, Y. (2008). Atlas of Groundwater Sustainable Utilization in North China Plain. Beijing: China Map Publishing House, 185. [in Chinese].
Zhao, X., Liu, J., Liu, Q., Tillotson, M. R., Guan, D., and Hubacek, K. (2015). Physical and Virtual Water Transfers for Regional Water Stress Alleviation in China. Proc. Natl. Acad. Sci. USA 112 (4), 1031–1035. doi:10.1073/pnas.1404130112
Zheng, C., and Gorelick, S. (2015). Global Change and the Groundwater Management challenge. Water Resource Res. 51, 1–21. doi:10.1002/2014WR016825
Zheng, C., Liu, J., Cao, G., Kendy, E., Wang, H., and Jia, Y. (2010). Can China Cope with its Water Crisis?-Perspectives from the North China Plain. Groundwater 48 (3), 350–354. doi:10.1111/j.1745-6584.2010.00695_3.x
Zhou, H., Wang, D., Sun, C., Tian, W., Yang, P., and Teng, F. (2011). Simulation Study on the Recovery and Storage of the Funnel of the Water Source in the central City of Shenyang. J. China Hydrol. 31 (5), 47–51. [in Chinese].
Keywords: geomorphology, groundwater, hydrogeology, urban aquifers, waterlogging
Citation: Jin M, Lancia M, Tian Y, Viaroli S, Andrews C, Liu J and Zheng C (2021) Hydrogeological Criteria to Improve the Sponge City Strategy of China. Front. Environ. Sci. 9:700463. doi: 10.3389/fenvs.2021.700463
Received: 26 April 2021; Accepted: 14 June 2021;
Published: 02 July 2021.
Edited by:
Prosun Bhattacharya, Royal Institute of Technology, SwedenReviewed by:
Trista McKenzie, University of Hawaii at Manoa, United StatesKangning Xu, Beijing Forestry University, China
Copyright © 2021 Jin, Lancia, Tian, Viaroli, Andrews, Liu and Zheng. This is an open-access article distributed under the terms of the Creative Commons Attribution License (CC BY). The use, distribution or reproduction in other forums is permitted, provided the original author(s) and the copyright owner(s) are credited and that the original publication in this journal is cited, in accordance with accepted academic practice. No use, distribution or reproduction is permitted which does not comply with these terms.
*Correspondence: Michele Lancia, bGFuY2lhQHN1c3RlY2guZWR1LmNu; Chunmiao Zheng, emhlbmdjbUBzdXN0ZWNoLmVkdS5jbg==
†These authors have contributed equally to this work