- 1Department of Biological Sciences, Faculty of Science, King Abdulaziz University (KAU), Jeddah, Saudi Arabia
- 2Department of Biology, Faculty of Life Sciences, Ahmadu Bello University, Zaria, Nigeria
Anthropogenic activities have been on the increase in the urban environment and have led to contamination of the environment with toxic metals. However, mangrove plants’ response to heavy metal stress due to anthropogenic activities explains the metal bioavailability and its potential ecotoxicological effect. We carried out a multi-approach study to investigate i) if the concentrations of metals (Cr, Mn, Fe, Co, Ni, Cu, Zn, As, Mo, Cd, Pb, Sr, and V) in mangroves at the central Red Sea are due to the anthropogenic influence and are above the sediment quality guidelines and ii) if an increase in metal concentration influences height and antioxidant enzyme (catalase, CAT; glutathione S-transferase, GST; and superoxide dismutase, SOD) activities in Avicennia marina. There were significant variations (p < 0.05) in metal concentrations and antioxidants across the six mangrove ecosystems with higher concentrations at Al Lith (LT) and south Jeddah (SJ). Even though the concentrations of metals in mangrove leaves are slightly higher in LT than SJ, principal component analysis showed that higher concentrations of Cr, Co, Zn, Cd, and Pb in mangrove leaves from SJ influence higher antioxidant enzyme activities and the lowest average mangrove height (2.01 m). This suggests that higher metal concentrations be factors for the biggest stress in these mangrove ecosystems. However, among the 13 metals, Cr (82.07 mg/kg), Cu (41.29 mg/kg), and As (10.30 mg/kg) in sediments have values within the ERL range of probable effect, while Ni (53.09 mg/kg) was above the ERM threshold. Hence, there is need to focus on monitoring these metals in mangrove sediments and their anthropogenic sources.
Introduction
Anthropogenic activities have been on the increase in the urban environment and have led to contamination of the environment with pollutants such as toxic metals (Song et al., 2014; Bakshi et al., 2018; Aljahdali and Alhassan, 2020a; Aljahdali and Alhassan, 2020b). Heavy metals get into the coastal environments with ease, settling in benthic sediment with the tendency to become persistent and accumulate in bio-systems, leading to physiological changes (Zhou et al., 2008; Aljahdali and Alhassan, 2020c). Pollution, habitat degradation, and overexploitation of natural resources are intensive anthropogenic activities that negatively affect biodiversity and ecosystem services (Ruiz-Compean et al., 2017). Stressors of anthropogenic origin in coastal environmental degradation, such as high concentrations of toxic metals from the urban environment, can accumulate in benthic sediment (Van der Oost et al., 2003). However, identification of pollutant sources is achieved using sediments to determine pathways of distribution and locate sinks of pollutants in the aquatic environment (Ruiz-Compean et al., 2017; Aljahdali and Alhassan, 2020a; Aljahdali and Alhassan, 2020d). This is achieved due to the vital role of benthic sediment in pollutants’ dynamics (Aljahdali et al., 2021b).
Mangroves are unique intertidal ecosystems distributed on a large area of the marine environment in tropical and subtropical regions of the world (Wang et al., 2013a; Chaudhuri et al., 2014). They have the function of protecting the coastlines against harmful effects of natural disasters such as erosion, flood, and storm surges (Zhang et al., 2012). However, the threat to mangrove ecosystems has been on the increase due to anthropogenic chemicals from the ever-increasing industrialization and urbanization centered on population growth (Polidoro et al., 2010; Chaudhuri et al., 2014). Mangrove plants such as Avicennia marina can be used to remove contaminants from the sediment; this is known as green remediation used widely in most developing parts of the world (Nouri et al., 2017; Almahasheer et al., 2018). Mangroves play a vital role in removing contaminants from sediments, leading to improved sediment quality in coastal ecosystems due to their ability to grow in chemical-polluted coastal environments (Chai et al., 2019). The efficiency of mangrove ecosystems in metal bioaccumulation and the ability to trap sediments leading to increased sediment accretion have an influence on metal filters and sinks in mangroves in coastal environments (Wang et al., 2013b; Marchand et al., 2016). However, the justification of mangroves as a bio-indicator of metal pollution points to their ability to accumulate various metal concentrations in leaves which correlates with that of sediments and other components of the environment (Pinheiro et al., 2012; Aljahdali and Alhassan, 2020a).
Efficient bio-monitoring of metal stress has been reported in mangroves due to their robust potential of antioxidant enzyme activity during utilization of defense systems, either enzymatic or non-enzymatic, for survival in environments polluted with metals (Bakshi et al., 2018; Aljahdali and Alhassan, 2020d). The specific physiological response in mangroves involves the production of reactive oxygen species (ROS), malondialdehyde (MDA), and antioxidants such as catalase (CAT), superoxide dismutase (SOD), and glutathione S-transferase (GST), among others. They are used as biomarkers of changes in the pristine nature of the environment, plant physiology, and oxidative stress due to metal pollution (Duarte et al., 2013; Aljahdali and Alhassan, 2020b; Aljahdali and Alhassan, 2020c).
Studies in the central Red Sea mangroves on contaminant levels using sediment quality guidelines and antioxidant enzyme activities as biomarkers of stress are few. In addition, the nonexistence of a monitoring framework presently for contaminants in the central Red Sea due to anthropogenic activities is of major concern. Here, we test the hypotheses that i) metal concentrations in mangroves at the central Red Sea are due to the anthropogenic influence and are above the sediment quality guidelines and ii) the increase in metal concentration influences mangrove height and antioxidant enzyme activities in A. marina. An essential function of antioxidants is to prevent oxidative damage of cells in bio-systems by scavenging ROS produced during stress conditions (Luo and Gu, 2018; Farzana et al., 2019; Aljahdali and Alhassan, 2020a; Aljahdali et al., 2021b). This research aims to establish how anthropogenic activities and metal concentrations interact to cause stress in mangroves. Our research would help design the appropriate management and monitoring policies to conserve this unique and vital ecosystem.
Materials and Methods
Study Area
The Red Sea comprises about 135 km2 area of mangroves distributed up to the northern boundary of mangroves at 28.207302oN. The central Red Sea is in an arid environment possessing high temperature and sparse rainfall; the mean annual (sporadic) rainfall in the Jeddah region is 55 mm (Aljahdali et al., 2021a). Some mangrove habitats of the Saudi Arabian Red Sea develop as a narrow fringe that could support halophytes located along the shore and adjacent to sand flats that may sometimes be flooded (Almahasheer et al., 2016; Rasul and Stewart, 2018).
The sampling locations (Figure 1) in this study were chosen based on the spatial distribution of mangroves and anthropogenic activities. Precisely, six mangrove stands were chosen, which include the following: Al Lith (LT) (20°08ʹ∼18.7''ʺN, 40°16ʹ∼41.74ʺE): production and extraction of living resources (huge aquaculture industry and fishing) and extraction of non-living resources (capital dredging); south Jeddah (SJ) (20°15ʹ∼43.92ʺN, 40°25ʹ∼11.37ʺE): high population with over 3 million inhabitants, extraction of natural resources (fishing) and main seaport, power plant, recreation, and tourism activities, and dredging for maintenance; Dahban (DB) (21°59ʹ∼05.1ʺN, 38°58ʹ∼42.9ʺE): recreation-like activities in the catchment and fishing in the mangroves; Thuwal Island (TH) (22°16ʹ∼36.99ʺN, 39°05ʹ∼00.34ʺE): tourism or recreation, fishing, and desalination plant; Rabigh Lagoon (RB) (22°53ʹ∼51.86ʺN, 38°55ʹ∼13.25ʺE): large petrochemical complex at the catchment (refinery), receives land runoff passing through agricultural fields, stagnant water due to decreased circulation, and livestock activities such as camel grazing; and Mastorah (MA) (23°07ʹ∼46.49ʺN, 38°47ʹ∼56.99ʺE): absence of anthropogenic source of chemical pollution but ∼50 km to the north is an industrial activity.
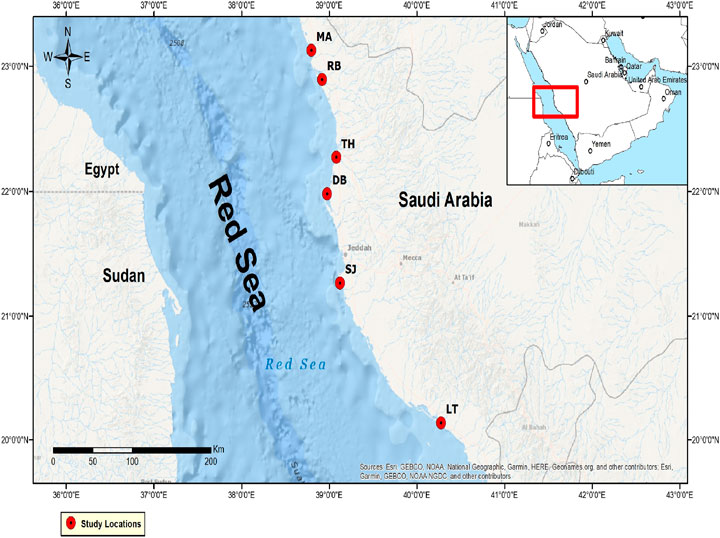
FIGURE 1. Map showing six mangrove ecosystems in central Red Sea, Saudi Arabia. LT: Al Lith; SJ: south Jeddah; DB: Dahban; TH: Thuwal; RB: Rabigh; MA: Mastorah.
Sample Collection and Chemical Analysis
Samples of matured leaves of A. marina mangroves and surface sediments (0–20 cm) were collected from six mangrove stands at LT, SJ, DB, TH, RB, and MA. Leaves and sediments from the mangrove ecosystems were sampled twice monthly, from May 2019 to April 2020. Sediments were sampled using a Van Veen grab of 250 cm2. Leaves were collected from 15 mangrove trees in each of the mangrove stands together with 15 surface sediment samples, placed in clean zip-lock bags inside an ice cooler box, and were conveyed to the laboratory.
All leaf samples were washed with deionized water and oven-dried at 40°C for 48 h, ground into powder using an agate mortar and pestle, and passed through a 53 μm sieve. 0.2 g of the ground and sieved leaf sample was digested in HNO3 and H2O2 (3:1) at 180°C for 45 min.
For sediment samples, 0.4 g of dried sediments was weighed and placed into a 50 ml digestion vessel, and 8 ml of HNO3: HCl (1:1) was added. The vessel was kept inside an Anton-Paar PE Multiwave 3000 microwave oven and digested at 200°C for about 1 h (USEPA, 1994). The vessel was filled by adding ultrapure Millipore Q water and placed on a shaker for 24 h.
The GF/F filter (Whatman) was used to filter the solution obtained from digested leaf and sediment samples, and later, metal concentrations were analyzed in the filtrates using a Varian 720-ES inductively coupled plasma-optical emission spectrometer (ICP-OES). The quality of the analytical method was verified using the standard reference material GSS-1 for sediments and GSV-2 for leaves. The accuracy of the analytical method was stated as recovery in percentage (93.19–119.45%) (Supplementary Table S1). For precision and accuracy of analysis, 10% of samples were repeatedly analyzed for every analytical batch. The preparation of calibration standard solutions was achieved by diluting ICP multi-element standard solutions IV and IX (Merck, Germany). In addition, 2% HNO3 solution was used as blank.
Grain Size Analysis
Oven-dried (40°C) sediment samples were weighed to determine the total dry weight. To facilitate the disintegration of solidified aggregates, distilled water was used to soak the dried sediments overnight. The sediments were then washed and made to pass through 0.063 and 2 mm sieves to separate fractions of gravel (>2 mm), coarse grain (0.063∼2 mm), and mud (clay and silt, <0.063 mm). The different sediment grain size percentages were then determined after the residue fractions were dried at 40°C and weighed (Prasad and Ramanathan, 2008).
Sediment Quality Index and Guidelines
The sediment quality index used was the enrichment factor (EF) as described by Abrahim and Parker (2008) using the following formula:
Here, Fe (iron) is used as the sample reference metal, Mx and Fex are the concentrations of metals and Fe (iron) in sediments, and Mb and Feb are suitable background concentrations of metals and Fe (iron). The classification of the metal contamination level is presented in Supplementary Table S2.
The sediment quality guidelines (Long et al., 1995) were used for comparison with our results. They comprise two types of guidelines, namely, effects range low (ERL) and effects range median (ERM), and describe three classes of concentration ranges for a specific pollutant. The values of pollutants/metals less than ERL imply a minimal-effects range. Values greater than or equal to ERL but less than ERM imply a possible-effects range in which effects will sometimes occur, while the values of pollutants greater than or equal to ERM imply a probable-effects range in which the effects of pollutants would always occur.
Bioaccumulation of Metals in Mangrove A. marina
To determine metal bioaccumulation in A. marina leaves, the bio-concentration factor (BCF) was computed to reveal the capacity of the plant to accumulate pollutants/metals using the following formula:
where Cleaf and Csediment are concentrations of a given element in leaves and sediments.
Assay of Catalase, Glutathione S-Transferase, and Superoxide Dismutase in A. marina
The leaf samples were washed with distilled water to remove debris and then pulverized using an ice-cold mortar and pestle with 0.01 M phosphate buffer (pH 7), and then centrifugation was performed at 14,000 rpm at 4°C for 24 min (Bakshi et al., 2018; Aljahdali and Alhassan, 2020a). After centrifuging, the supernatant was used to measure antioxidants on a Labtronics spectrophotometer (Model: LT-291 Single Beam UV-VIS).
The assay of CAT was carried out by using the reaction mixtures containing 0.01 M phosphate buffer, 30 mM hydrogen peroxide, and the enzyme extract. Activities were measured at an absorbance of 230 nm for 2 min in μmol/min/mg protein (Chandlee and Scandalios, 1984).
The reaction mixture containing 1 mM 1-chloro-2,4-dinitrobenzene (CDNB), 0.1 M potassium phosphate buffer of pH 6.5, 1–40 mM GSH, and 100 μl of leaf extracts in a total volume of 1 ml was used for the assay of GST. GST was measured at 340 nm per min after GS-DNB production and recorded with a spectrophotometer at 25°C (Habig et al., 1974).
The protocol proposed by Keyster et al. (2012) was used for the assay of SOD. 10 μl of the enzyme extract was added to a reaction mixture containing 0.1 mM xanthine, 6.25 nM xanthine oxidase, 50 mM K2HPO4 of pH 7.8, 0.1 mM EDTA, 0.025% (w/v) Triton X-100, and 0.1 mM 2-(4-iodophenyl)-3-(4-nitrophenyl)-5-(2,4-disulfophenyl)-2H-tetrazolium (WST-1). The SOD activity was measured on a spectrophotometer at an absorbance of 450 nm at 37°C for 20 min. The specific activity was recorded in units/mg protein, where 1U of enzyme activity = enzyme concentration required to avoid 50% production of chromogen under conditions of the assay for 1 min.
Data Analysis
Homogeneity of variance and a test of normality were carried out using Levene’s homogeneity of variance and Shapiro–Wilk tests. One-way analysis of variance (ANOVA) was used to determine significant variations in metal concentrations in sediments and leaves of A. marina, sediment grain sizes, and antioxidants across the six mangrove stands. Tukey’s post hoc test was used for mean separation at a 5% significant level where statistical significance was recorded. Dimensional principal component analysis (PCA) was used to determine the influence of sediment grain sizes on metal concentrations in sediments and the influence of metals on A. marina height and antioxidants. The correlation heat map/matrix was used to test the relationship between metals in sediments and leaves of A. marina. Data analysis was achieved using R for Windows (v. 4.0.3).
Results
Variability of Particle Size and Metal Concentration in Sediments
The grain size of sediments varies significantly (ANOVA, p < 0.05) among the six mangrove stands. The coarse grain (0.063–2 mm) type ranged from 45.50% at Rabigh (RB) to 88.83% at south Jeddah (SJ), the lowest percentage of clay and silt particles was recorded at SJ (10.53%), while gravels ranged from 0.20% at RB and Dahban (DB) to 0.63% at SJ (Table 1).
A significant difference in sediment grain size types was observed among the study sites (Table 1). The highest clay and silt grain size type was recorded at RB (54.29%), about fivefold than SJ with the lowest clay and silt grain size type. However, coarse (88.83%) and gravel (0.63%) sediment grain sizes at SJ were the highest among the study sites but not substantially different from the percentage coarse sediment type at LT. In addition, the lowest percentage of coarse (45.50%) sediment and gravel (0.20%) was revealed in RB mangrove ecosystems, while RB clay and silt particles’ percentage was only about 1.19 times its coarse sediment particles (Table 1).
The mean concentrations of metals in sediments in this study showed a substantial wide range of variations among the six mangrove stands under study (Table 1). In total, the average of mangrove stands’ metal concentrations was low compared to international thresholds. However, the concentrations were higher than those reported by the Environmental Protection and Control Department of Saudi Arabia (EPCDSA, 2010) except for Cd and Pb (Table 2). The average concentrations of 13 metals (Cr, Mn, Fe, Co, Ni, Cu, Zn, As, Mo, Cd, Pb, Sr, and V) (mg/kg) in sediments analyzed in this study significantly (ANOVA, p < 0.05) differed among mangrove stands. In addition, LT and SJ mangrove stands recorded higher concentrations of metals than DB, Thuwal (TH), RB, and Mastorah (MA), except for concentrations of Fe (2,583.47 mg/kg) and As (13.81 mg/kg), Mo (1.42 mg/kg), and Sr (7,715.28 mg/kg) recorded at MA, DB, and TH, respectively (ANOVA, p < 0.05) (Table 1). Specifically, concentrations of Cr, Mn, Co, Ni, Cd, Pb, and V were significantly higher in LT sediments than the other mangrove sediments but not far from concentrations in SJ. A strong positive correlation existed between eight metals (Cd, Cu, Pb, Cr, V, Mn, Co, and Ni) in mangrove sediments (Figure 2A). The dimensional principal component analysis at 95% confidence interval revealed the influence of sediment grain size on metal concentrations (Figures 3A,B). Dimensions 1 and 2 with the highest variation accounted for 47.3 and 22.3% of the total variation, with clay and silt sediment grain size influencing all the metals except Mo and Sr, which were influenced by coarse sediments and gravels. All the sediment grain size types and metals had high contribution to the total variation except Fe, Zn, Mo, and As (Figure 3A). However, LT and RB form no cluster with other study areas based on grain size types and sediment metal concentrations (Figure 3B).
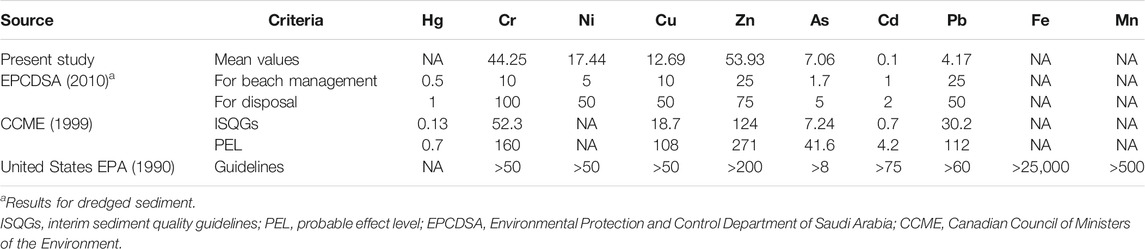
TABLE 2. Maximum levels of metals (mg/kg) allowed by environmental departments in several countries.
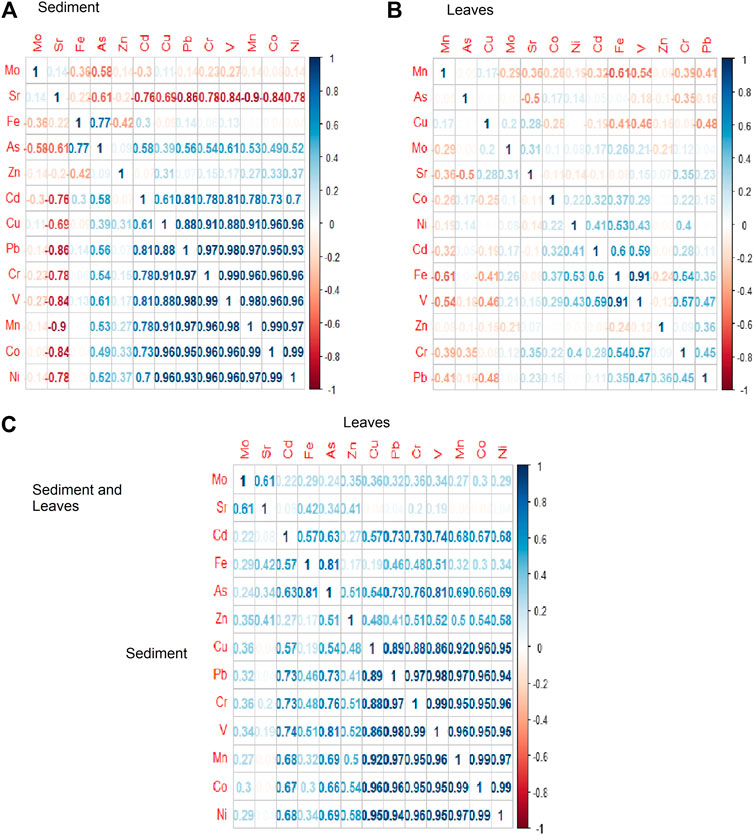
FIGURE 2. Correlation (r) matrix for metal concentrations in the sediment (A), leaves (B), and between metals in sediment and leaves (C).
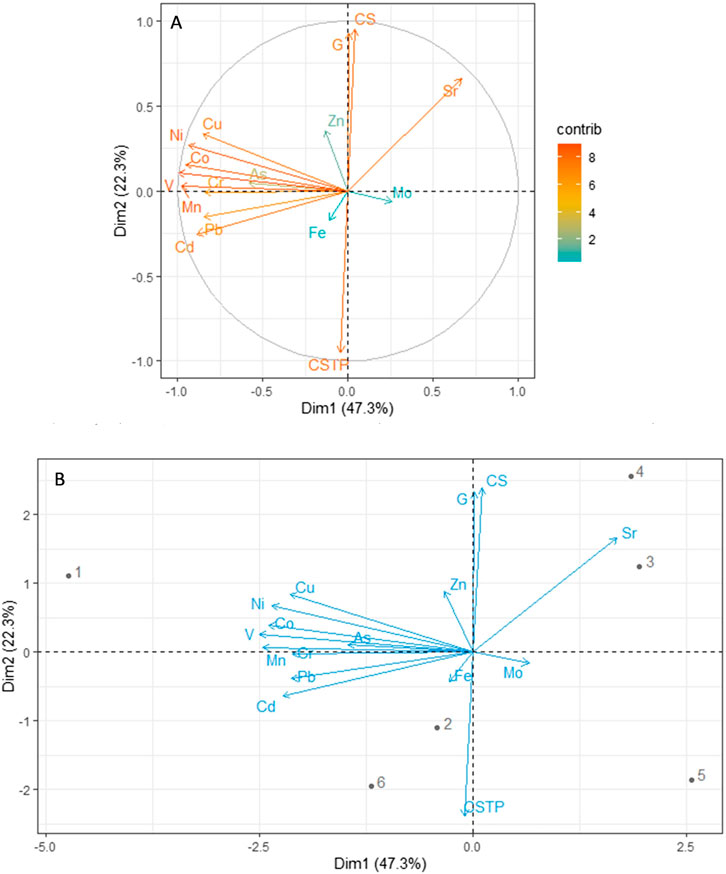
FIGURE 3. Principal component analysis for the relationship between metal concentrations in sediments and grain sizes. (A) Contribution to dimensions and total variation and (B) biplot. Key: CS, coarse sand; CSTP, clay and silt particles; G, gravels; 1, Al Lith; 2, south Jeddah; 3, Dahban; 4, Thuwal; 5, Rabigh; 6, Mastorah.
Anthropogenic activities and factors influencing them such as dredging, extraction of living resources (fishing), tourism and recreational activities, grazing by camels, agricultural activities, human population, and industrialization were recorded and vary from one mangrove ecosystem to another. However, PCA at 95% confidence interval with components 1 and 2 accounting for 66.5% of the total variation revealed human population, industrialization, extraction of living resources, dredging, and agricultural activities to influence Cr, Mn, Co, Ni, Cu, Zn, As, Cd, Pb, and V concentrations (Figures 4A,B). Factors such as human population, industrialization, agricultural activities, and extraction of living resources had a higher contribution to the total variation (Figure 4A).
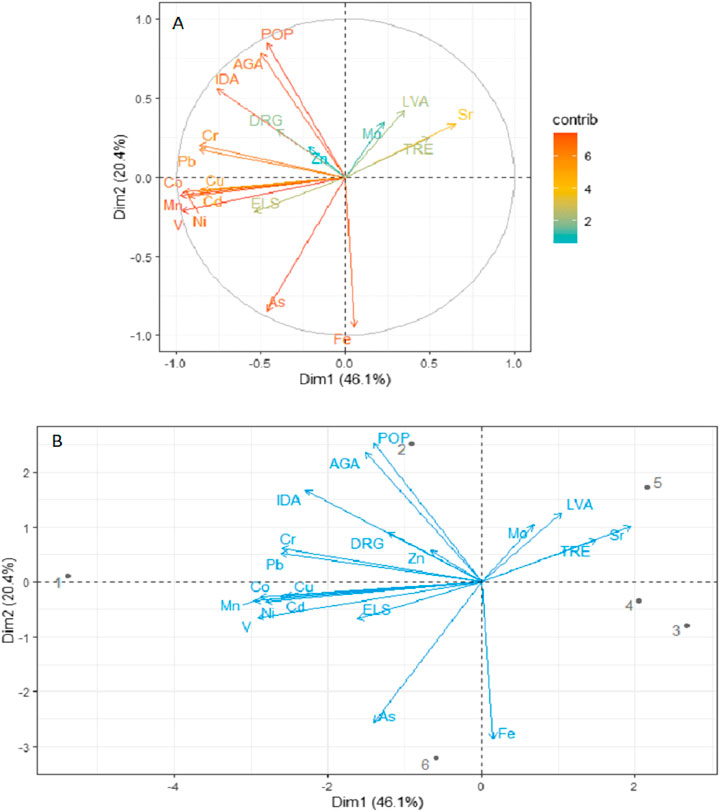
FIGURE 4. Principal component analysis for the relationship between metal concentrations and anthropogenic activities. (A) Contribution to dimensions and total variation and (B) biplot. Key: POP, human population; DRG, dredging; ELS, extraction of living resources (fishing); TRE, tourism and recreation; LVA, livestock activities; AGA, agricultural activities; IDA, industrialization; 1, Al Lith; 2, south Jeddah; 3, Dahban; 4, Thuwal; 5, Rabigh; 6, Mastorah.
Sediment Enrichment Factor and Quality Guidelines
In total, the six mangrove ecosystems are extremely enriched with Sr (≥40), and significant enrichment (5–19.99) of sediments with Mn, Cu, Zn, Cd, Cr, Ni, As, Mo, and V was also established. In contrast, the mangrove stands were moderately enriched (2–4.99) with Pb (Figure 5A). The concentrations of Cr in LT and SJ were above the ERL threshold but lower than ERM. Similarly, the concentrations of Cu and As at LT were above the ERL threshold but fell between ERL and ERM. In addition, Ni concentration at LT was above the ERM threshold (Table 1).
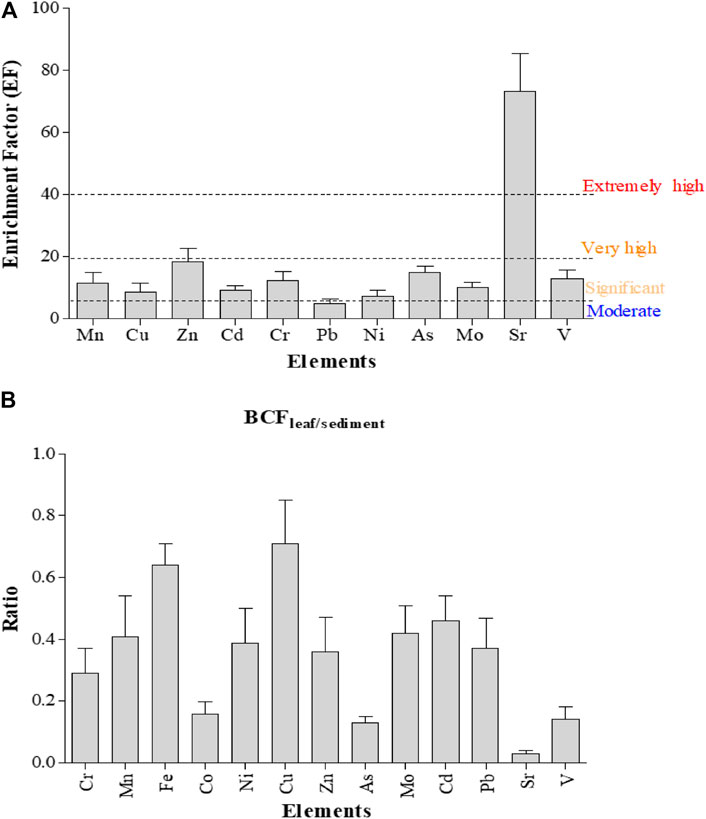
FIGURE 5. Metal enrichment factor (A) and bioconcentration factor (BCF) for metal concentrations in six mangrove ecosystems (B).
Metals in A. marina Leaves
The average concentrations of heavy metals determined in A. marina leaves were significantly (ANOVA, p < 0.05) different across the six mangrove stands (Table 3). Similar to concentrations in sediments, metal concentrations were higher in LT and SJ except for Cu (5.28 mg/kg) and Mo (0.39 mg/kg), Sr (95.37 mg/kg), and Fe (1,595.18 mg/kg) and V (7.60 mg/kg) at DB, TH, and MA, respectively (Table 3). A strong correlation was formed between only Fe and V in leaves (Figure 2B). However, for correlation between metals in leaves and sediments combined, Cu, Pb, Cr, V, Mn, Co, and Ni formed a strong positive correlation (Figure 2C). In addition, the BCF of metal concentrations was low and 1/2 the concentrations in sediment, except for Cr, Co, As, and V where concentrations were almost 1/4 of the sediment concentrations, while Sr concentration in leaves was even 1/30 of the concentration in sediments. This suggests that Sr concentration in sediments was about 30 times the concentration in mangrove leaves (Figure 5B).
Antioxidants and Mangrove Height
The activities of three antioxidant enzymes (CAT, GST, and SOD) used as biomarkers of oxidative stress in A. marina vary significantly (ANOVA, p < 0.05) across the six mangrove stands in the central Red Sea (Table 3). The highest concentration of CAT (9.20 μmol/mg protein), GST (60.27 μmol/mg protein), and SOD (7.40 μmol/mg protein) was recorded at SJ and corresponded to the lowest average mangrove height (2.01 m), which was not much different from the average mangrove height (2.05 m) at LT. In contrast, the lowest activities of CAT (5.35 μmol/mg protein) and GST (9.80 μmol/mg protein) were at TH, and lowest activities of SOD (3.56 μmol/mg protein) were recorded at DB. At 95% confidence interval, dimension-based PCA for antioxidants, mangrove heights, and metals revealed dimensions 1 and 2, accounting for 39.4 and 26.7% of the total variation (Figures 6A,B). It also revealed a strong negative relationship between mangrove height (r = −0.7589) and the antioxidant enzymes (CAT, GST, and SOD) (Figure 6B). In addition, Pb (r = 0.8601), Co (0.8206), and Zn (0.8126) concentrations in leaves appear to influence antioxidant enzymes than any other metals in A. marina. However, Fe, Cd, V, Co, Zn, Pb, Cr, and Ni, together with antioxidants and mangrove height, have a higher contribution to the dimensional variation (Figure 6A). In terms of antioxidants and metals in leaves, clusters were formed between SJ and LT, and DB and TH, while RB and MA stand separately, forming no cluster with any study area (Figure 6B). However, Pb appears to be the biggest cause of antioxidant activities at SJ and LT and influencing their mangrove height.
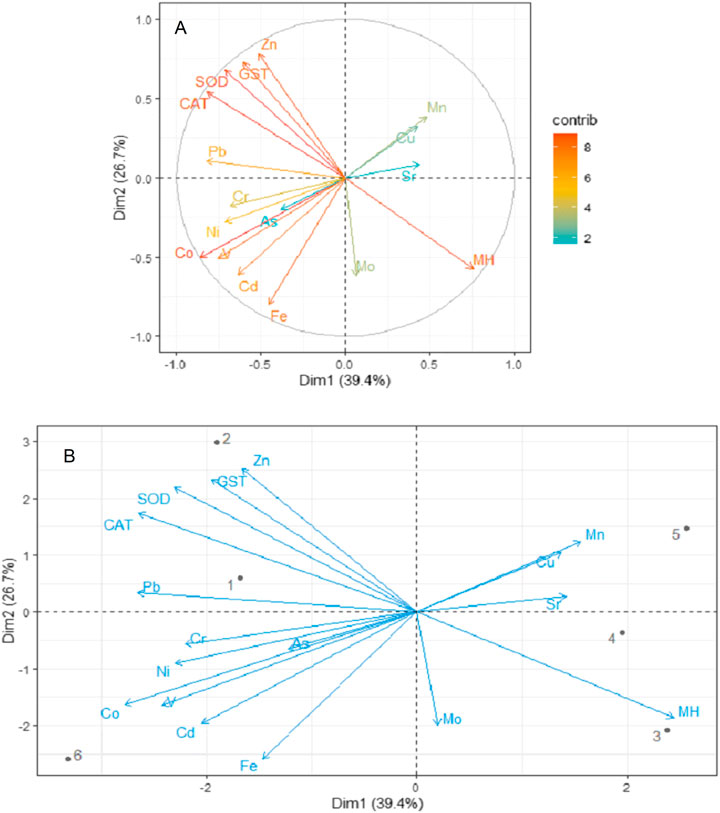
FIGURE 6. Principal component analysis for the relationship between metal concentrations and antioxidants in the mangroves. (A) Contribution to dimensions and total variation and (B) biplot. Key: CAT, catalase; GST, glutathione S-transferase; SOD, superoxide dismutase; MH, mangrove height; 1, Al Lith; 2, south Jeddah; 3, Dahban; 4, Thuwal; 5, Rabigh; 6, Mastorah.
Discussion
The concentrations of heavy metals determined in this study for A. marina leaves and sediments in six mangrove stands located in the central Red Sea revealed low to moderate concentrations when compared to earth crust average values (Taylor and McLennan, 2001), enrichment factor (Abrahim and Parker, 2008), sediment quality guidelines (Long et al., 1995), and reported concentrations in other studies from mangrove ecosystems (Abu-Hilal and Badran, 1990; Carral et al., 1996; Buccolieri et al., 2006; Chaudhuri et al., 2014; Song et al., 2014; Almahasheer, 2019) (Table 4). However, sediments were extremely enriched with Sr (Abrahim and Parker, 2008). Similar concentrations of heavy metals to the concentrations in this present study except V were also reported from other locations in central Red Sea and northern and southern parts of the Red Sea (Hanna, 1992; Fowler et al., 1993; Badr et al., 2009; Soliman et al., 2015; Abu-Zied and Hariri, 2016; Youssef and El-Sorogy, 2016; Ruiz-Compean et al., 2017; Almahasheer et al., 2018). This suggests that the six mangrove ecosystems investigated in this study might not be under severe heavy metal pollution despite the different anthropogenic activities going on in these ecosystems and their catchment.
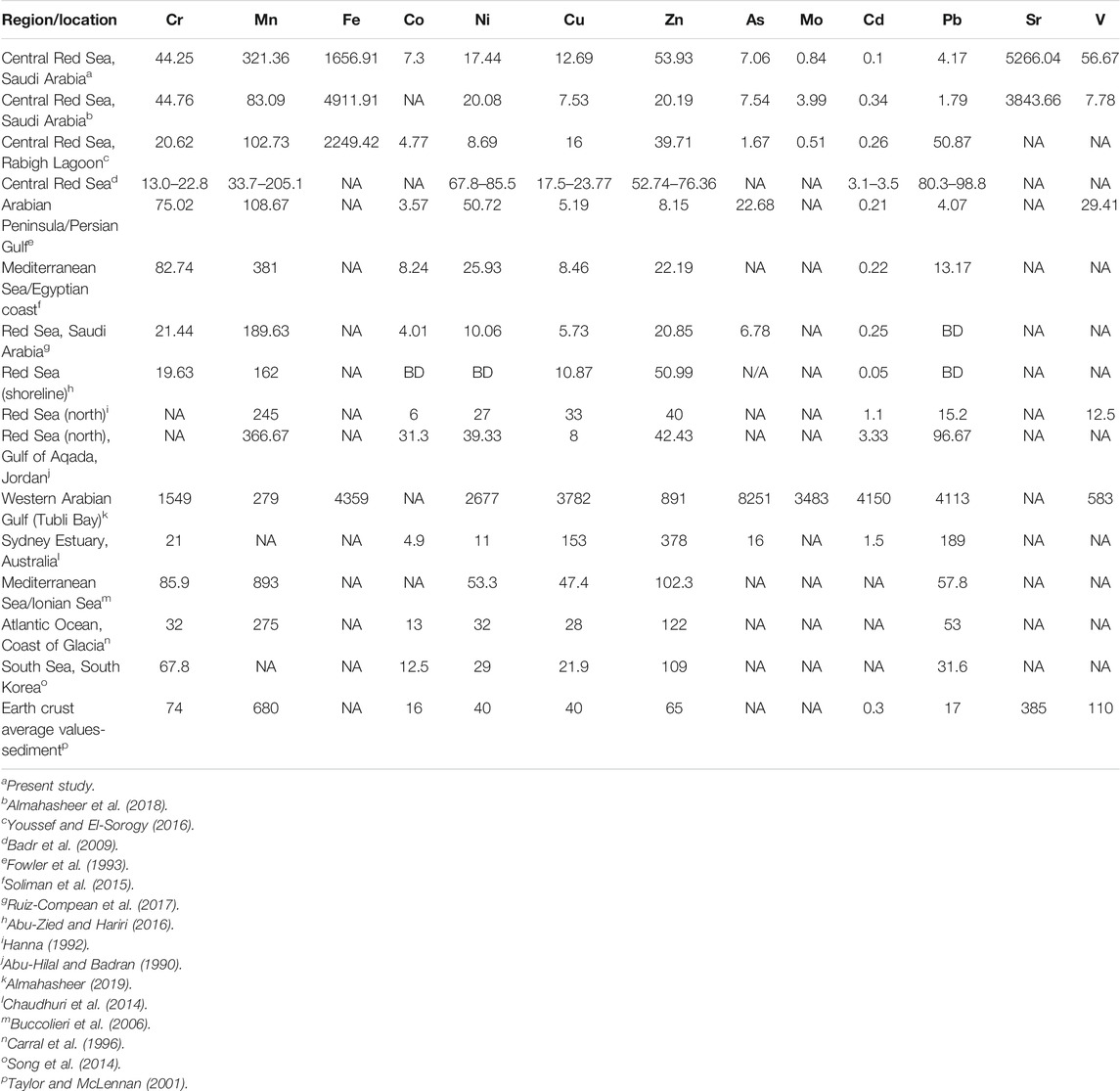
TABLE 4. Comparison of metal concentrations to those in other studies in the Red Sea and some reference studies from other parts of the world.
Impacts of Anthropogenic Activities on Metal Concentrations in Sediments
The connection between heavy metal contamination in the mangrove stands investigated in this study and anthropogenic sources was not too apparent in some sites sampled. However, metal contamination in south Jeddah (SJ) mangrove stands to some extent showed a relationship with anthropogenic activities (Figure 4B). Together with Al Lith (LT) mangrove stands, high concentrations of Pb, Cd, and Cr could be linked to industrial activities located in this region of the kingdom. This could give an insight into a strong positive correlation (r = 0.7542) between industrialization as an anthropogenic activity and metals such as Cr, Mn, Co, Ni, Cu, Zn, As, Cd, Pb, and V (Figure 4A). Elsewhere, in the shoreline of Red Sea, higher concentrations of Cr and Pb were recorded in Jeddah, Cr concentration in our study is about 1.6 times the Cr concentration (50 mg/kg) reported, while Pb concentration in our study is about 1/7 of the concentration (64 mg/kg) reported (Abu-Zied and Hariri, 2016). This suggests more concentration of Pb in Jeddah than only south Jeddah but higher concentrations of Cr in south Jeddah. In contrast to our findings at LT, lower contamination of metals was reported (Abu-Zied and Hariri, 2016). Contamination of the Red Sea by metals has been reported to be influenced by increased population and discharge of industrial effluents (Ruiz-Compean et al., 2017). Precisely, metals such as Pb, Cd, Ni, and Cr originate from different industrial waste (Häder et al., 2020). Though, factors such as runoff passing through agricultural fields and other contaminated urban environments into the sea and metals from transportation emission from ships are serious sources of metal contamination in the marine environment (Christophoridis et al., 2019). Undeniably, various studies on mangrove metal contamination were carried out in environments close to anthropogenic sources and in shoreline areas where there is a probability of high metal contamination (Badr et al., 2009; Ruiz-Compean et al., 2017). The sampling sites, different locations of sampling, and the wider area of sampling in our study could form a key reason for the differences between our results and other previous studies (Badr et al., 2009; Almahasheer, 2019). For instance, Badr et al. (2009) carried out their study on metal contamination in three coastal areas of the Red Sea. They sampled near commercial and industrial facilities, while Youssef and El-Sorogy (2016) investigated a single lagoon in the central Red Sea. In contrast, we covered a broader area, considering six major mangrove ecosystems in the central Red Sea because of the objectives of our study. Hence, there is a possibility to observe variations between our results and those of the authors mentioned earlier.
Relatively high concentrations of metals such as As, Ni, Cr, Cd, Fe, and Pb at Dahban (DB), Thuwal (TH), Rabigh (RB), and especially at Mastorah (MA) without clear cut sources of pollution, industries, and low human population, can be associated with the role of dust and vegetated habitats in settling and accumulation of contaminants (Guo et al., 2014; Al-Taani et al., 2015; Richir et al., 2015; Bonanno and Di Martino, 2016). Concentrations of some nutrients and metals such as iron in the ocean have been linked to contributions by dust (Goudie, 2009). In addition, sandstorms are common in Saudi Arabia, influence the weather of the kingdom, and favor transportation of metals (Maghrabi et al., 2011). The geomorphological factors that may include regional siltation, sedimentation, tidal hydrodynamics, and sediment grain size have also been known to play a crucial role in the distribution of metal concentration (Agah et al., 2016; Bakshi et al., 2018). This explains the influence of grain size types, especially clay and silt grain size, on the distribution and concentrations of metals (Chaudhuri et al., 2014; Bakshi et al., 2018).
Sediment Quality Guidelines and Saudi Arabian Regulations
The enrichment factor (EF) gives information on the level of metal contamination and their sources, i.e., if natural or anthropogenic (Bakshi et al., 2018; Aljahdali and Alhassan, 2020a; Aljahdali and Alhassan, 2020c). High EF (> 10) of Mn, Zn, Cr, As, Mo, Sr, and V falling in the scale of significant, very high, and extremely high enrichment (Abrahim and Parker, 2008) suggests this metal enrichment may be due to anthropogenic sources (Zhou et al., 2020). However, it was established in our results based on comparison with international sediment quality guidelines that metal contamination is low to moderate in mangrove stands in the central Red Sea under study. The need to focus and/or monitor metals such as Cr, Cu, As, and Ni is the sine qua non because they are within the ERL range for the probable biological effect. Ni is even more important to be considered as it is the only metal with a concentration above the ERM threshold, while a low positive correlation between As (r = 0.31161) and clay and silt sediment grain size could be a result of contamination presumably due to an anthropogenic source (Caredda et al., 1999; Chaudhuri et al., 2014). Effluents from industries in the seasonal land runoff, agricultural waste, and household waste could form a key reason for the increased concentration of Ni above ERM (Cempel and Nikel, 2006; Aljahdali and Alhassan, 2020c). In another study in the Red Sea, Ni concentration was above ERM (Ruiz-Compean et al., 2017). However, the maximum concentration (65.62 mg/kg) was only 1.23 times the maximum concentration of Ni in our study.
Comparisons between our results and regulations for dredged sediments and beach management in Saudi Arabia set by the EPCDSA revealed Cr, Cu, As, and Ni average concentrations above established limits (EPCDSA, 2010) (Table 2). This further suggests the need to prioritize monitoring these metals in Saudi Arabia environs, even though the EPCDSA standard limit is set below international limits for low probable ecological effects such as those of the Canadian (1999) and USEPA (1994).
Metals in Mangrove A. marina and BCF
The mangrove A. marina leaves in this study are considered non-hyperaccumulators because the concentrations of metals in leaves are below 1% of those in mangrove sediments. The plant–sediment interaction based on metal accumulation is better estimated using the BCF. However, the BCFs for all the 13 metals were <1, suggesting an effective mechanism of detoxification or exclusion by A. marina (Almahasheer et al., 2018; Aljahdali and Alhassan, 2020a). In addition, it should be noted that some heavy metals determined in this study such as Fe, Cu, Mn, and Mo are essential for plant growth, if not in excess or above a limit where they become toxic (Appenroth, 2010). In contrast, Cr, Pb, As, and Cd are very toxic to bio-systems such as plants (Shahid et al., 2013; Almahasheer, 2019). The highest BCF in our study (Cu: 0.71) was the same as that in another study in the mangrove Avicennia species stand at Hooghly Matla estuary, India (Bakshi et al., 2018). However, our value for the highest BCF was about 1/2 the value (1.38) reported for Avicennia species in Sundarbans mangrove stands (Chowdhury et al., 2015). This suggests a more efficient accumulation of Cu by A. marina than other metals in the six mangrove stands in the central Red Sea studied.
A strong positive correlation between Fe and V in leaves and between Cu, Pb, Cr, V, Mn, Co, and Ni in leaves and sediments suggests possible same sources, either natural or anthropogenic, of these metals and the same mechanism of transportation and pattern of accumulation (Bastami et al., 2014). Besides the influence of metal accumulation and concentration in sediments on the corresponding concentration in leaves, efficient uptake of Cu, Pb, Cr, V, Mn, Co, and Ni gives an insight into the strong positive correlation between these metals in sediments and leaves (Bi et al., 2013; Aljahdali and Alhassan, 2020d). It is of importance to note that, for this reason, the scope of utilization of A. marina mangroves to bio-monitor metals such as Cu, Pb, Cr, V, Mn, Co, and Ni in marine pollution is possible.
Influence of Metals on Stress in Mangroves Across Central Red Sea
Stress enzymes, otherwise called antioxidant enzymes, such as CAT, GST, and SOD are some of the basic types and play an important role in scavenging reactive oxygen species (H2O2, OH, O2, etc.) generated by bio-systems in stress conditions (Bakshi et al., 2018; Asaeda and Barnuevo, 2019; Aljahdali and Alhassan, 2020a). This has led to the use of these enzymes as early signals in the form of biomarkers of physiological changes in A. marina due to exposure to drivers of oxidative stress (Caregnato et al., 2008; Aljahdali and Alhassan, 2020d). The negative correlation between the three antioxidants and mangrove height (r = −0.7589) for the six mangrove stands suggests response of antioxidants to oxidative stress (Farzana et al., 2019).
The relationship in terms of increased antioxidant activities with high metal concentration has been reported by previous studies (Nadgórska-Socha et al., 2008; Doğanlar and Atmaca, 2011). This could be a key reason for significant variation in antioxidants across the six mangroves, with high enzyme activities corresponding to high concentrations of heavy metals except for Al Lith (LT) and south Jeddah where antioxidant activities were higher at south Jeddah (SJ) with metal concentrations slightly lower than those at LT. However, concentrations of metals such as Cr, Co, Zn, Cd, and Pb were higher in mangrove leaves from SJ, and this might be the primary reason for greater antioxidant activities in SJ than LT, suggesting a higher contribution to stress by these metals. Cr, Cd, and Pb concentrations and excess concentrations of Zn and Co may induce oxidative stress and increase membrane lipid peroxidation in plants (Han et al., 2016); in response to physiological changes, antioxidant enzyme activities will increase (Aljahdali and Alhassan, 2020a; Aljahdali and Alhassan, 2020d). In addition, excessive concentrations of trace elements in an ecosystem have been associated with their large uptake by plants resulting in increased stress and decreased growth and development (Kosiorek and Wyszkowski, 2020). In another study at Matang Mangrove Forest Reserve, Malaysia, mangrove A. marina with the highest biomass, density, and height (12.9 m) had very low Zn (0.428 mg/kg), Cd (0.0046 mg/kg), and Pb (0.046 mg/kg) concentrations (Khan et al., 2019). It is worth pointing out that the values of Zn, Cd, and Pb in that study were 1/41, 1/17, and 1/9 of the concentrations in SJ mangroves with higher antioxidant enzyme activities and the lowest average mangrove height (2.01 m). Elsewhere, tissue- and site-specific antioxidant enzyme activities in Avicennia species due to metal pollution were reported (Harish and Murugan, 2011). In addition, an ex situ and in situ experiment in a mangrove ecosystem composed of A. marina in Australia revealed a correlation between increased antioxidant activities and increased metal concentration in mangrove leaves (Caregnato et al., 2008).
Conclusion
The mean concentrations of 13 heavy metals (Cr, Mn, Fe, Co, Ni, Cu, Zn, As, Mo, Cd, Pb, Sr, and V) in six mangrove ecosystems revealed a substantial wide range of variations. Higher concentrations of metals were recorded in Al Lith (LT) and south Jeddah (SJ) mangrove ecosystems. When sediment quality guidelines are considered, Cr, Cu, As, and Ni need to be given special attention and monitored because they fall within the ERL range for the probable biological effect. Ni is even more important to be considered as it is the only metal with a concentration above the ERM threshold. However, averages of the six mangrove ecosystems’ metal concentrations were low compared to international thresholds but higher than Environmental Protection and Control Department of Saudi Arabia values except for Cd and Pb.
The connection between heavy metal contamination in the mangrove ecosystems and anthropogenic sources was not too apparent in some sampling sites. However, metal contamination in south Jeddah (SJ) and Al Lith (LT) mangrove ecosystems showed a strong relationship with anthropogenic activities and factors such as industrialization, extraction of living resources, dredging, and agricultural activities. High concentrations of Pb, Cd, and Cr in SJ and LT could be linked to industrial activities in these regions of the kingdom. Even though the concentrations of metals in mangrove leaves are slightly higher in LT than SJ, metals such as Cr, Co, Zn, Cd, and Pb were higher in mangrove leaves from SJ with higher antioxidant enzyme activities and the lowest average mangrove height. This suggests that Cr, Co, Zn, Cd, and Pb concentrations are factors for the biggest stress in these mangrove ecosystems investigated. Our research would help design the appropriate management and monitoring policies by stakeholders of these unique and important ecosystems and bridge the gap of knowledge on anthropogenic activities and their influence on heavy metal contamination and stress in mangroves.
Data Availability Statement
The raw data supporting the conclusions of this article will be made available by the authors, without undue reservation.
Author Contributions
MA and AA designed the study and carried out the field surveys and laboratory analysis. AA performed statistical analyses. MA and AA contributed to the initial and final drafting of the manuscript, improvement, and approval for submission.
Funding
This project was funded by the National Plan for Science, Technology, and Innovation (MAARIFAH), King Abdulaziz City for Science and Technology, the Kingdom of Saudi Arabia, award number (14-ENV263-03).
Conflict of Interest
The authors declare that the research was conducted in the absence of any commercial or financial relationships that could be construed as a potential conflict of interest.
Acknowledgments
The authors acknowledge the Science and Technology Unit, King Abdulaziz University, for technical support.
Supplementary Material
The Supplementary Material for this article can be found online at: https://www.frontiersin.org/articles/10.3389/fenvs.2021.691257/full#supplementary-material
References
Abrahim, G. M. S., and Parker, R. J. (2007). Assessment of Heavy Metal Enrichment Factors and the Degree of Contamination in marine Sediments from Tamaki Estuary, Auckland, New Zealand. Environ. Monit. Assess. 136, 227–238. doi:10.1007/s10661-007-9678-2
Abu-Hilal, A. H., and Badran, M. M. (1990). Effect of Pollution Sources on Metal Concentration in Sediment Cores from the Gulf of Aqaba (Red Sea). Mar. Pollut. Bull. 21, 190–197. doi:10.1016/0025-326x(90)90501-x
Abu-Zied, R. H., and Hariri, M. S. B. (2016). Geochemistry and Benthic Foraminifera of the Nearshore Sediments from Yanbu to Al-Lith, Eastern Red Sea Coast, Saudi Arabia. Arab. J. Geosci. 9, 245. doi:10.1007/s12517-015-2274-9.pdf
Agah, H., saleh, A., Bastami, K. D., and Fumani, N. S. (2016). Ecological Risk, Source and Preliminary Assessment of Metals in the Surface Sediments of Chabahar Bay, Oman Sea. Mar. Pollut. Bull. 107, 383–388. doi:10.1016/j.marpolbul.2016.03.042
Al-Taani, A. A., Rashdan, M., and Khashashneh, S. (2015). Atmospheric Dry Deposition of mineral Dust to the Gulf of Aqaba, Red Sea: Rate and Trace Elements. Mar. Pollut. Bull. 92, 252–258. doi:10.1016/j.marpolbul.2014.11.047
Aljahdali, M. O., and Alhassan, A. B. (2020a). Ecological Risk Assessment of Heavy Metal Contamination in Mangrove Habitats, Using Biochemical Markers and Pollution Indices: A Case Study of Avicennia marina L. In the Rabigh Lagoon, Red Sea. Saudi J. Biol. Sci. 27, 1174–1184. doi:10.1016/j10.1016/j.sjbs.2020.02.004
Aljahdali, M. O., and Alhassan, A. B. (2020d). Heavy Metal Accumulation and Anti-oxidative Feedback as a Biomarker in Seagrass Cymodocea Serrulata. Sustainability 12, 2841. doi:10.3390/su12072841
Aljahdali, M. O., and Alhassan, A. B. (2020b). Metallic Pollution and the Use of Antioxidant Enzymes as Biomarkers in Bellamya Unicolor (Olivier, 1804) (Gastropoda: Bellamyinae), Water 12, 202. doi:10.3390/w12010202
Aljahdali, M. O., and Alhassan, A. B. (2020c). Spatial Variation of Metallic Contamination and its Ecological Risk in Sediment and Freshwater Mollusk: Melanoides Tuberculata (Müller, 1774) (Gastropoda: Thiaridae). Water 12, 206. doi:10.3390/w12010206
Aljahdali, M. O., Alhassan, A. B., and Zhang, Z. (2021b). Environmental Factors Causing Stress in Avicennia marina Mangrove in Rabigh Lagoon along the Red Sea: Based on a Multi-Approach Study. Front. Mar. Sci. 8, 328. doi:10.3389/fmars.2021.646993
Aljahdali, M. O., Munawar, S., and Khan, W. R. (2021a). Monitoring Mangrove Forest Degradation and Regeneration: Landsat Time Series Analysis of Moisture and Vegetation Indices at Rabigh Lagoon, Red Sea. Forests 12, 52. doi:10.3390/f12010052
Almahasheer, H., Duarte, C. M., and Irigoien, X. (2016). Nutrient Limitation in central Red Sea Mangroves. Front. Mar. Sci. 3, 271. doi:10.3389/fmars.2016.00271
Almahasheer, H. (2019). High Levels of Heavy Metals in Western Arabian Gulf Mangrove Soils. Mol. Biol. Rep. 46, 1585–1592. doi:10.1007/s11033-019-04603-2
Almahasheer, H., Serrano, O., Duarte, C. M., and Irigoien, X. (2018). Remobilization of Heavy Metals by Mangrove Leaves. Front. Mar. Sci. 5, 484. doi:10.3389/fmars.2018.00484
Appenroth, K.-J. (2010). What Are "heavy Metals" in Plant Sciences? Acta Physiol. Plant 32, 615–619. doi:10.1007/s11738-009-0455-4.pdf
Asaeda, T., and Barnuevo, A. (2019). Oxidative Stress as an Indicator of Niche-Width Preference of Mangrove Rhizophora Stylosa. For. Ecol. Management 432, 73–82. doi:10.1016/j10.1016/j.foreco.2018.09.015
Badr, N. B. E., El-Fiky, A. A., Mostafa, A. R., and Al-Mur, B. A. (2009). Metal Pollution Records in Core Sediments of Some Red Sea Coastal Areas, Kingdom of Saudi Arabia. Environ. Monit. Assess. 155, 509–526. doi:10.1007/s10661-008-0452-x
Bakshi, M., Ghosh, S., Chakraborty, D., Hazra, S., and Chaudhuri, P. (2018). Assessment of Potentially Toxic Metal (PTM) Pollution in Mangrove Habitats Using Biochemical Markers: A Case Study on Avicennia officinalis L. In and Around Sundarban, India. Mar. Pollut. Bull. 133, 157–172. doi:10.1016/10.1016/j.marpolbul.2018.05.030
Bastami, K. D., Bagheri, H., Kheirabadi, V., Zaferani, G. G., Teymori, M. B., Hamzehpoor, A., et al. (2014). Distribution and Ecological Risk Assessment of Heavy Metals in Surface Sediments along Southeast Coast of the Caspian Sea. Mar. Pollut. Bull. 81, 262–267. doi:10.1016/j.marpolbul.2014.01.029
Bi, X., Liang, S., and Li, X. (2013). A Novel In Situ Method for Sampling Urban Soil Dust: Particle Size Distribution, Trace Metal Concentrations, and Stable lead Isotopes. Environ. Pollut. 177, 48–57. doi:10.1016/j10.1016/j.envpol.2013.01.045
Bonanno, G., and Di Martino, V. (2016). Seagrass Cymodocea Nodosa as a Trace Element Biomonitor: Bioaccumulation Patterns and Biomonitoring Uses. J. Geochemical Exploration 169, 43–49. doi:10.1016/j10.1016/j.gexplo.2016.07.010
Buccolieri, A., Buccolieri, G., Cardellicchio, N., Dell'Atti, A., Di Leo, A., and Maci, A. (2006). Heavy Metals in marine Sediments of Taranto Gulf (Ionian Sea, Southern Italy). Mar. Chem. 99, 227–235. doi:10.1016/j.marchem.2005.09.009
Canadian, M. O. (1999). E (2002) Canadian Sediment Quality Guidelines for the protection of Aquatic Life: Summary Tables. Can. Environ. Qual. Guidel.
Caredda, A. M., Cristini, A., Ferrara, C., Lobina, M. F., and Baroli, M. (1999). Distribution of Heavy Metals in the Piscinas beach Sediments (SW Sardinia, Italy). Environ. Geology. 38, 91–100. doi:10.1007/s002540050405.pdf
Caregnato, F. F., Koller, C. E., MacFarlane, G. R., and Moreira, J. C. F. (2008). The Glutathione Antioxidant System as a Biomarker Suite for the Assessment of Heavy Metal Exposure and Effect in the Grey Mangrove, Avicennia marina (Forsk.) Vierh. Mar. Pollut. Bull. 56, 1119–1127. doi:10.1016/j10.1016/j.marpolbul.2008.03.019
Carral, E., Puente, X., Villares, R., and Carballeira, A. (1996). Background Heavy Metal Levels in Estuarine Sediments and Organisms in Galicia (Northwest Spain) as Determined by Modal Analysis. Oceanogr. Lit. Rev. 7, 728. doi:10.1016/0048-9697(95)04788-3
Cempel, M., and Nikel, G. (2006). Nickel: a Review of its Sources and Environmental Toxicology. Polish J. Environ. Stud. 15, 8349. doi:10.15244/pjoes/87881
Chai, M., Li, R., Tam, N. F. Y., and Zan, Q. (2019). Effects of Mangrove Plant Species on Accumulation of Heavy Metals in Sediment in a Heavily Polluted Mangrove Swamp in Pearl River Estuary, China. Environ. Geochem. Health 41, 175–189. doi:10.1007/s10653-018-0107-y
Chandlee, J. M., and Scandalios, J. G. (1984). Analysis of Variants Affecting the Catalase Developmental Program in maize Scutellum. Theoret. Appl. Genet. 69, 71–77. doi:10.1007/BF00262543
Chaudhuri, P., Nath, B., and Birch, G. (2014). Accumulation of Trace Metals in Grey Mangrove Avicennia marina fine Nutritive Roots: the Role of Rhizosphere Processes. Mar. Pollut. Bull. 79, 284–292. doi:10.1016/j.marpolbul.2013.11.024
Chowdhury, R., Favas, P. J. C., Pratas, J., Jonathan, M. P., Ganesh, P. S., and Sarkar, S. K. (2015). Accumulation of Trace Metals by Mangrove Plants in Indian Sundarban Wetland: Prospects for Phytoremediation. Int. J. Phytoremediation 17, 885–894. doi:10.1080/15226514.2014.981244
Christophoridis, C., Bourliva, A., Evgenakis, E., Papadopoulou, L., and Fytianos, K. (2019). Effects of Anthropogenic Activities on the Levels of Heavy Metals in marine Surface Sediments of the Thessaloniki Bay, Northern Greece: Spatial Distribution, Sources and Contamination Assessment. Microchemical J. 149, 104001. doi:10.1016/10.1016/j.microc.2019.104001
Doğanlar, Z. B., and Atmaca, M. (2011). Influence of Airborne Pollution on Cd, Zn, Pb, Cu, and Al Accumulation and Physiological Parameters of Plant Leaves in Antakya (Turkey). Water, Air. Soil Pollut. 214, 509–523. doi:10.1007/s11270-010-0442-9
Duarte, B., Santos, D., and Caçador, I. (2013). Halophyte Anti-oxidant Feedback Seasonality in Two Salt Marshes with Different Degrees of Metal Contamination: Search for an Efficient Biomarker. Funct. Plant Biol. 40, 922–930. doi:10.1071/fp12315
Farzana, S., Cheung, S. G., Zhou, H. C., and Tam, N. F. Y. (2019). Growth and Antioxidative Response of Two Mangrove Plants to Interaction between Aquaculture Effluent and BDE-99. Sci. Total Environ. 662, 796–804. doi:10.1016/j.scitotenv.2019.01.263
Fowler, S. W., Readman, J. W., Oregioni, B., Villeneuve, J.-P., and McKay, K. (1993). Petroleum Hydrocarbons and Trace Metals in Nearshore Gulf Sediments and Biota before and after the 1991 War: an Assessment of Temporal and Spatial Trends. Mar. Pollut. Bull. 27, 171–182. doi:10.1016/0025-326X(93)90022-C
Goudie, A. S. (2009). Dust Storms: Recent Developments. J. Environ. Manage. 90, 89–94. doi:10.1016/j.jenvman.2008.07.007
Guo, L., Chen, Y., Wang, F., Meng, X., Xu, Z., and Zhuang, G. (2014). Effects of Asian Dust on the Atmospheric Input of Trace Elements to the East China Sea. Mar. Chem. 163, 19–27. doi:10.1016/10.1016/j.marchem.2014.04.003
Habig, W. H., Pabst, M. J., and Jakoby, W. B. (1974). Glutathione S-Transferases. J. Biol. Chem. 249, 7130–7139. doi:10.1016/s0021-9258(19)42083-8
Häder, D.-P., Banaszak, A. T., Villafañe, V. E., Narvarte, M. A., González, R. A., and Helbling, E. W. (2020). Anthropogenic Pollution of Aquatic Ecosystems: Emerging Problems with Global Implications. Sci. Total Environ. 713, 136586. doi:10.1016/j.scitotenv.2020.136586
Han, Y., Zhang, L., Yang, Y., Yuan, H., Zhao, J., Gu, J., et al. (2016). Pb Uptake and Toxicity to Iris Halophila Tested on Pb Mine Tailing Materials. Environ. Pollut. 214, 510–516. doi:10.1016/j10.1016/j.envpol.2016.04.048
Hanna, R. G. M. (1992). The Level of Heavy Metals in the the Red Sea after 50 Years. Sci. Total Environ. 125, 417–448. doi:10.1016/0048-9697(92)90405-h
Harish, S. R., and Murugan, K. (2011). Oxidative Stress Indices in Natural Populations of Avicennia alba Blume. As Biomarker of Environmental Pollution. Environ. Res. 111, 1070–1073. doi:10.1016/j.envres.2011.07.002
Keyster, M., Klein, A., and Ludidi, N. (2012). Caspase-like Enzymatic Activity and the Ascorbate-Glutathione Cycle Participate in Salt Stress Tolerance of maize Conferred by Exogenously Applied Nitric Oxide. Plant Signaling Behav. 7, 349–360. doi:10.4161/psb.18967
Khan, W. R., Zulkifli, S. Z., Kasim, M. R. B. M., Pazi, A. M., Mostapa, R., and Nazre, M. (2019). Mangrove Productivity Estimation Using Modelling Approach and Tree Parameters Assessment. Trop. Conserv. Sci. 12. doi:10.1177/1940082919872137
Kosiorek, M., and Wyszkowski, M. (2020). Remediation of Cobalt-Contaminated Soil Using Manure, clay, Charcoal, Zeolite, Calcium Oxide, Main Crop (Hordeum Vulgare L.), and After-Crop (Synapis alba L.). Minerals 10, 429. doi:10.3390/min10050429
Long, E. R., MacDonald, D. D., Smith, S. L., and Calder, F. D. (1995). Incidence of Adverse Biological Effects within Ranges of Chemical Concentrations in marine and Estuarine Sediments. Environ. Manage. 19, 81–97. doi:10.1007/BF02472006.pdf
Luo, L., and Gu, J.-D. (2018). Nutrient Limitation Status in a Subtropical Mangrove Ecosystem Revealed by Analysis of Enzymatic Stoichiometry and Microbial Abundance for Sediment Carbon Cycling. Int. Biodeterioration Biodegradation 128, 3–10. doi:10.1016/j.10.1016/j.ibiod.2016.04.023
Maghrabi, A., Alharbi, B., and Tapper, N. (2011). Impact of the March 2009 Dust Event in Saudi Arabia on Aerosol Optical Properties, Meteorological Parameters, Sky Temperature and Emissivity. Atmos. Environ. 45, 2164–2173. doi:10.1016/j.atmosenv.2011.01.071
Marchand, C., Fernandez, J.-M., and Moreton, B. (2016). Trace Metal Geochemistry in Mangrove Sediments and Their Transfer to Mangrove Plants (New Caledonia). Sci. Total Environ. 562, 216–227. doi:10.1016/j.scitotenv.2016.03.206
Nadgórska-Socha, A., Kafel, A., and Gospodarek, J. (2008). Heavy Metals in Leaves and Physiological of Philadelphus Coronarus L. In Urban and Unpolluted Areas. Scripra Fac. Nat. Univ. Ostrav. 186, 278–284. doi:10.1007/s10646-016-1654-6
Nouri, H., Chavoshi Borujeni, S., Nirola, R., Hassanli, A., Beecham, S., Alaghmand, S., et al. (2017). Application of green Remediation on Soil Salinity Treatment: a Review on Halophytoremediation. Process Saf. Environ. Prot. 107, 94–107. doi:10.1016/j.psep.2017.01.021
Pinheiro, M. A. A., Silva, P. P. G. e., Duarte, L. F. d. A., Almeida, A. A., and Zanotto, F. P. (2012). Accumulation of Six Metals in the Mangrove Crab Ucides Cordatus (Crustacea: Ucididae) and its Food Source, the Red Mangrove Rhizophora Mangle (Angiosperma: Rhizophoraceae). Ecotoxicology Environ. Saf. 81, 114–121. doi:10.1016/10.1016/j.ecoenv.2012.05.004
Polidoro, B. A., Carpenter, K. E., Collins, L., Duke, N. C., Ellison, A. M., Ellison, J. C., et al. (2010). The Loss of Species: Mangrove Extinction Risk and Geographic Areas of Global Concern. PLoS One 5, e10095. doi:10.1371/journal.pone.0010095
Prasad, M. B. K., and Ramanathan, A. L. (2008). Sedimentary Nutrient Dynamics in a Tropical Estuarine Mangrove Ecosystem. Estuar. Coast. Shelf Sci. 80, 60–66. doi:10.1016/j.ecss.2008.07.004
Rasul, N. M. A., and Stewart, I. C. F. (2018). Oceanographic and Biological Aspects of the Red Sea. Nature Switzerland: Springer. doi:10.1007/978-3-319-99417-8
Richir, J., Salivas-Decaux, M., Lafabrie, C., Lopez y Royo, C., Gobert, S., Pergent, G., et al. (2015). Bioassessment of Trace Element Contamination of Mediterranean Coastal Waters Using the Seagrass Posidonia Oceanica. J. Environ. Manage. 151, 486–499. doi:10.1016/j.jenvman.2014.11.015
Ruiz-Compean, P., Ellis, J., Cúrdia, J., Payumo, R., Langner, U., Jones, B., et al. (2017). Baseline Evaluation of Sediment Contamination in the Shallow Coastal Areas of Saudi Arabian Red Sea. Mar. Pollut. Bull. 123, 205–218. doi:10.1016/j.marpolbul.2017.08.059
Shahid, M., Ferrand, E., Schreck, E., and Dumat, C. (2013). Behavior and Impact of Zirconium in the Soil-Plant System: Plant Uptake and Phytotoxicity. Rev. Environ. Contam. Toxicol. 221, 107–127. doi:10.1007/978-1-4614-4448-0_2
Soliman, N. F., Nasr, S. M., and Okbah, M. A. (2015). Potential Ecological Risk of Heavy Metals in Sediments from the Mediterranean Coast, Egypt. J. Environ. Health Sci. Engineer 13, 1–12. doi:10.1186/s40201-015-0223-x
Song, Y., Choi, M. S., Lee, J. Y., and Jang, D. J. (2014). Regional Background Concentrations of Heavy Metals (Cr, Co, Ni, Cu, Zn, Pb) in Coastal Sediments of the South Sea of Korea. Sci. Total Environ. 482–483, 80–91. doi:10.1016/j.scitotenv.2014.02.068
Taylor, S. R., and McLennan, S. M. (2001). Chemical Composition and Element Distribution in the Earth's Crust. Encycl. Phys. Sci. Technol. 312. 10.1016/B0-12-227410-5/00097-1
United States Environmental Protection Agency (1994). Method 3051 A. Microwave Assisted Acid Digestion of Sediments, Sludge’s, Soils and Oils. Washington, DC:USEPA, U.S. Government Printing Office. Available at: http://www.epa.∼gov/SW-846/pdfs/3051a.pdf.
Van der Oost, R., Beyer, J., and Vermeulen, N. P. E. (2003). Fish Bioaccumulation and Biomarkers in Environmental Risk Assessment: a Review. Environ. Toxicol. Pharmacol. 13, 57–149. doi:10.1016/S1382-6689(02)00126-6
Wang, S.-L., Xu, X.-R., Sun, Y.-X., Liu, J.-L., and Li, H.-B. (2013a). Heavy Metal Pollution in Coastal Areas of South China: a Review. Mar. Pollut. Bull. 76, 7–15. doi:10.1016/j.marpolbul.2013.08.025
Wang, Y., Qiu, Q., Xin, G., Yang, Z., Zheng, J., Ye, Z., et al. (2013b). Heavy Metal Contamination in a Vulnerable Mangrove Swamp in South China. Environ. Monit. Assess. 185, 5775–5787. doi:10.1007/s10661-012-2983-4
Youssef, M., and El-Sorogy, A. (2016). Environmental Assessment of Heavy Metal Contamination in Bottom Sediments of Al-Kharrar Lagoon, Rabigh, Red Sea, Saudi Arabia. Arab. J. Geosci. 9, 474. doi:10.1007/s12517-016-2498-3.pdf
Zhang, K., Liu, H., Li, Y., Xu, H., Shen, J., Rhome, J., et al. (2012). The Role of Mangroves in Attenuating Storm Surges. Estuarine, Coastal Shelf Sci. 102–103, 11–23. doi:10.1016/j10.1016/j.ecss.2012.02.021
Zhou, H., Chun, X., Lü, C., He, J., and Du, D. (2020). Geochemical Characteristics of Rare Earth Elements in Windowsill Dust in Baotou, China: Influence of the Smelting Industry on Levels and Composition. Environ. Sci. Process. Impacts 22, 2398–2405. doi:10.1039/D0EM00273A
Keywords: heavy metals, anthropogenic activities, mangrove, stress, antioxidants, Rabigh
Citation: Alhassan AB and Aljahdali MO (2021) Sediment Metal Contamination, Bioavailability, and Oxidative Stress Response in Mangrove Avicennia marina in Central Red Sea. Front. Environ. Sci. 9:691257. doi: 10.3389/fenvs.2021.691257
Received: 05 April 2021; Accepted: 17 May 2021;
Published: 14 June 2021.
Edited by:
Andrew Hursthouse, University of the West of Scotland, United KingdomReviewed by:
Naga Raju Maddela, Technical University of Manabi, EcuadorAn Liu, Shenzhen University, China
Copyright © 2021 Alhassan and Aljahdali. This is an open-access article distributed under the terms of the Creative Commons Attribution License (CC BY). The use, distribution or reproduction in other forums is permitted, provided the original author(s) and the copyright owner(s) are credited and that the original publication in this journal is cited, in accordance with accepted academic practice. No use, distribution or reproduction is permitted which does not comply with these terms.
*Correspondence: Abdullahi Bala Alhassan, YWFsaGFzc2FuMDAyMUBzdHUua2F1LmVkdS5zYQ==; Mohammed Othman Aljahdali, bW9hbGphaGRhbGlAa2F1LmVkdS5zYQ==