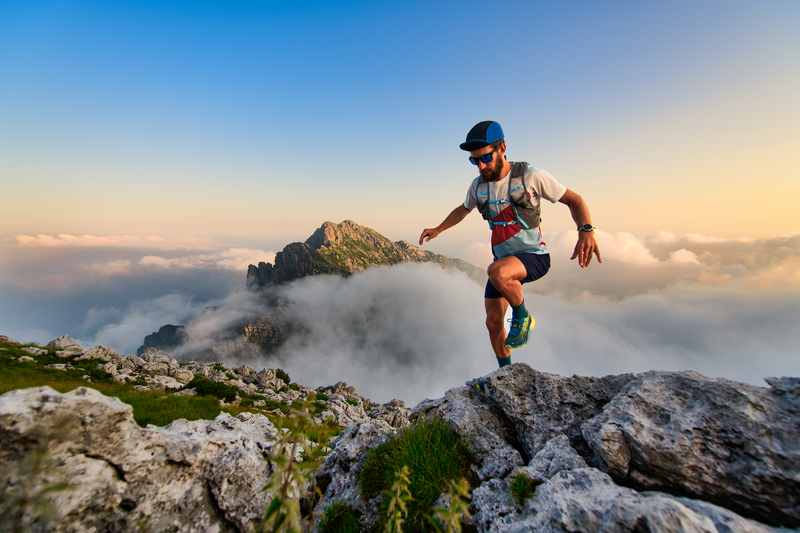
95% of researchers rate our articles as excellent or good
Learn more about the work of our research integrity team to safeguard the quality of each article we publish.
Find out more
REVIEW article
Front. Environ. Sci. , 15 June 2021
Sec. Toxicology, Pollution and the Environment
Volume 9 - 2021 | https://doi.org/10.3389/fenvs.2021.688430
This article is part of the Research Topic Exploring Plant Rhizosphere, Phyllosphere and Endosphere Microbial Communities to Improve the Management of Polluted Sites View all 28 articles
Rare earth (RE) elements are a group of 17 chemical elements including the 15 lanthanides plus Yttrium and Scandium. RE have been identified as critical elements due to their special properties (e.g., catalytic, metallurgical, nuclear, electrical, magnetic, and luminescent) and various applications in many modern technologies, environment and economic areas. Thus, the demand for RE has increased significantly during the last decades. This demand has induced an increase in mining activities and consequently a release of RE into the surrounding environment, causing a potential threat to human health and the environment. Therefore, investigations leading to new solutions for the RE recycling from alternate resources like electronic, mining and industrial wastes, has been rapidly growing. In spite of that, recycling stays extremely difficult, expensive and is currently not seen as a significant solution. The concept of phytomanagement is a promising solution when conventional mining methods are no longer cost-effective, not to mention all the ecosystem services provided by plants. The phytoextraction service allows the extraction and recovery of RE from soils or industrial wastes (e.g., phosphogypsum from phosphoric acid production) with the prospect of economic added value. To date, some twenty hyperaccumulator plant species (almost ferns such as Dicranopteris dicthotoma) accumulate high concentrations of RE especially in their erial parts. While the potential roles of native bacteria in mobilization of RE from ores remains slightly documented, those of Plant Growth Promoting Rhizobacteria (PGPR) is much less. PGPR are indeed able to mobilize metals and/or to stimulate plant development in the aim to increase the amount of RE extracted by the plant with then a higher phytoextraction efficiency. Yet to date, only a few studies have been devoted to RE using coupled bioaugmentation-phytoextraction. This review summarizes the data regarding 1) the source of RE (RE-accumulating sediments, soils naturally rich in RE, wastes) and their bioavailability in these matrices, 2) plants identified as RE hyperaccumulator and their potential for RE phytomining, 3) isolation and selection of indigenous bacteria stemming from RE contaminated matrices, such as soil, for their potential ability to increase phytoextraction performances and 4) bioaugmentation-assisted phytoextraction studies dealing with RE.
The rare earth elements (RE) are strategic metals because of their increasing importance in high technology applications and renewable energy. RE is relatively abundant in the earth’s crust and widely distributed around the world, but is rarely concentrated, as is the case with most metals, in mines, making it economically viable to exploit. Currently, RE are extracted mostly in China which supplies more than 85% of the World’s RE, to meet the growing global demand. The RE demand increased significantly during the last decades, which will put great pressure on the current RE supply chain. This continuously demand has induced an increase in mining activities and consequently a release of RE into the soil especially surrounding mining areas, causing a potential threat to human health and the environment. Currently, conventional hydrometallurgical extraction of RE is realized by using harsh acidic and basic conditions at high temperature (Zhang and Edwards, 2013), which release a large amounts of toxic and radioactive waste, Furthermore, the extraction efficiency is dependent on ores containing high concentrations of RE, which restricts the possibility of recovery. Therefore, investigations leading to new solutions for the RE recycling from alternate resources like electronic, mining and industrial wastes, has been rapidly growing. In spite of that, recycling stays extremely difficult, expensive and is still quite limited and was calculated in 2011 to be around one per cent of RE supply. This had led to the development of processes which are more economically feasible and environmentally friendly. On the one hand, phytoremediation (i.e., phytoextraction) appears as an innovative solution that may help to reduce the high levels of RE in soils through the use of RE hyperaccumulating plants. On the other hand, the use of bacteria (bioaugmentation) capable of mobilizing RE and/or stimulating plant growth (PGPR – Plant Growth Promoting Rhizobacteria—properties) could serve to increase the quantity of RE extracted by the plant and consequently, improve phytoextraction efficiency. The association of these two techniques (bioaugmentation and phytoextraction) may offer an attractive alternative to conventional processes in yielding a cost-effective and ecological solution. To the best of our knowledge, no research coupling bioaugmentation and phytoextraction has yet to be implemented to treat RE.
This study provides an overview of the current literature relating to various aspects of RE in the soil environment. RE properties, main applications, resources, recovery from waste and potential health and environmental risk are characterized. The main focus in this review is about the analysis of the RE phytoextraction process, the ability of plants to hyperaccumulate RE and the possibility of enhancing phytoextraction process with the use of microorganisms (bioaugmentation) to mobilize RE and/or to promote plant growth.
Rare earths, are diversely abbreviated to as rare earths (RE), rare-earth elements (REE), rare-earth metals (REM), rare earth oxides (REO) and total rare-earth oxides (TREO) (Massari and Ruberti, 2013; Mehmood, 2018). Hereinafter referred to as RE are a group of 17 chemically similar elements in the periodic table as defined by International Union of Pure and Applied Chemistry (IUPAC) (Massari and Ruberti, 2013; Balaram, 2019). They are the scandium (Sc) (Z = 21), yttrium (Y) (Z = 39) and 15 lanthanides with numbers successive atomics from 57 to 71 from the periodic table of elements: lanthanum (La), cerium (Ce), praseodymium (Pr), neodymium (Nd), promethium (Pm), samarium (Sm), europium (Eu), gadolinium (Gd), terbium (Tb), dysprosium (Dy), holmium (Ho), erbium (Er), thulium (Tm), ytterbium (Yb), and lutetium (Lu). Scandium and yttrium belonging to group IIIA of the transition metals, are not lanthanides. They are usually included in RE because they occur naturally in the same minerals and have similar physical and chemical properties: e.g., in the crystalline compounds, they generally have the +III oxidation state, despite some can also have a +II or +IV oxidation state, the coordination numbers in compounds are usually greater than six and decrease across the series. RE bind preferably with oxygen or fluorine as strong electronegative elements. All RE except promethium occur in nature (Henderson, 1984; Mehmood, 2018). They have high electrical conductivity and there are very small differences in solubility and complex formation between these metals (Voncken, 2015). In addition, RE are generally similar in their electronic configuration and ionic radius, so that they have a similar chemical and physical properties (Krishnamurthy and Gupta, 2016). Based on their location in the periodic table and their atomic weights, RE are divided into light RE (i.e., those with a lower mean atomic mass, Z = 57 up to 62) and heavy RE [i.e., those with a higher mean atomic mass, Z = 63 up to 71 (Damhus, 2005)]. Heavy RE are different from light RE in that they have “paired electrons” (counter-clockwize spinning electrons) (Voncken, 2015). Yttrium, although light (Z = 39), is included with the heavy RE group because of its similar chemical and physical properties in terms of compressibility at high pressures and the behavior of the ratio of the Wigner–Seitz radius to the ionic radius as a function of pressure (Chakhmouradian and Wall, 2012; USGS, 2014). Some authors include europium and gadolinium within the group of heavy RE but there is no consensus. Generally, the heavy RE are more used and less concentrated because of their lower crustal abundances and limited reserves. In the Earth’s crust, there is a general trend of decreasing abundance of RE with increasing atomic number. (Xiao et al., 2015). From other studies a third class, medium-RE, includes europium and gadolinium and samarium (Hatch, 2011; Zhanheng, 2011). RE do not exist as individual native metals in nature such us a gold. They are extracted from ores such as bastnaesites, monazites and xenotimes (Zhang et al., 2015).
RE were used in many applications in the past and they are becoming more popular in high-tech industry nowadays. Their unique physical and chemical properties (such as unique magnetic and optical properties) have made them essential for a growing number of critical technologies, i.e., those subject to trade disputes such as semiconductors and computers. (Migaszewski and Gałuszka, 2015; Suli et al., 2017; Ganguli and Cook, 2018; Balaram, 2019; Seaman, 2019).
The applications of RE concern traditional and high-tech sectors. Traditional sectors include the metallurgy industries, glass, ceramics, as well as the oil and chemical industry. RE can be in the form of a mixture of metals, oxides or salts. High-tech sectors, which developed over the past 40 years, include the industries of phosphorus, permanent magnets, batteries [hydrogen storage material, nickel-metal hydride (NiMH)], polishing powders and the nuclear industry. For these applications, RE often need to be in the form of high purity oxides (Zhang et al., 2016). The main industrial applications of RE are briefly summarized in Table 1. In addition, RE have been used in the China agriculture for about 20 years as fertilizers. The yield and quality for several crops have been improved (Pang et al., 2002; Balaram, 2019). Zhenhong and Hong (1996) reported that the RE can raise the output of wheat 4–10% every year in a continuous ten-year period after applications of RE of 600 g/ha-yr. Pang et al. (2002) summarized the effects of RE on main crops.
Due to all these applications, the RE demand increased manifold during the last decades and the trend appears to be for a continued increase in the use of RE. Zhou et al. (2017) reviewed current and predicted 10-years market demand for RE. It is reported that RE demand from clean technologies will reach 51.9 thousand metric tons (kt) REO in 2030. Scenarios on future demand for the RE (Nd, Dy, Pr, and Tb) can be found in the literature up to the year 2035 (Marscheider-Weidemann et al., 2016). Worldwide RE demand is growing rapidly. The annual demand for RE doubled up to 125,000 tons in 15 years, and the demand is projected to reach 315,000 tons in 2030, driven by increasing uptake in green technologies, e.g., global wind show a strong growth in demand and their capacity reached 870 GW in 2021 and could grow to greater than 1330 GW by 2026. Though other applications for NdFeB magnets in renewable energy production will become more important, as governments make efforts to meet stringent global warming and emissions standards.
This demand has induced an increase in mining activities and consequently a release of RE into the surrounding environment, causing a potential threat to human health and the environment.
The massive use of RE in various industries has induced serious contaminations of the surrounding environment which could, in turn, cause negative effects on human health. Despite the growing interest raised by RE, the information that has become available over the last two decades regarding RE is relatively recent and scarce, which has led to the current controversy regarding the health benefits at low concentrations vs. toxic effects at high concentrations. RE have previously been considered to be not toxic and to be readily excreted by animals and human after ingestion. The highest RE concentration was measured in the liver of fattening bulls amounted to 22–482 μg/kg DM for La, 37–719 μg/kg DM for Ce and 4–73 μg/kg DM for Pr and the lowest La, Ce and Pr concentrations with 3–5 μg/kg DM, 5–7 μg/kg DM and 0.5–0.7 μg/kg DM, respectively; Schwabe et al., 2012). Nevertheless, recent publications have reported toxic effects of RE in human health (Li et al., 2013; Rim et al., 2013; Gwenzi et al., 2018), in aquatic organisms (Malhotra et al., 2020), in aquatic microbes (Wilde, 2001), animals (Briner et al., 2000; Rim, 2016) and plants (Babula et al., 2009). The determination of RE concentrations reporting non-toxic and toxic effects in living organisms vary according to the total concentration of these elements in the environment and among different species and the bodyweight of individuals, which in turn depends on age. Moreover, it’s depended on short and long-term exposure. The risks of RE pollution due to mining, transportation and processing as well as from the improper disposal of materials containing these compounds could potentially lead to elevated levels within the environment, that may have very serious environmental and occupational risks. These studies show that the solvent extraction used in the RE mining process [e.g., versatic, naphthenic and phosphoric acids (Xie et al., 2014)] have been responsible for the disease and occupational poisoning of workers and local residents, water pollution, and the contamination of agricultural area. The processing of RE rich monazite rocks for the production of phosphate fertilizers and the subsequent applications of these fertilizers could further increase RE soil concentrations, especially in agricultural areas (Turra and Bacchi, 2011). The amount of RE used in Chinese agriculture has been increasing continuously, reaching some thousands of tons per year. A detailed description of the RE contents reported in the worldwide literature for the main phosphate fertilizers and other products of relevant use in agriculture effects on performance parameters is published by Ramos et al. (2016). Li et al. (2013) reported that RE soil pollution due to tailings from an RE processing plant in China can travel up to approximately 7 km before soil concentrations stabilize to natural levels. In addition, most RE deposits contain radioactive materials (thorium and uranium especially), which impose the risk of radioactive dust emissions. Due to the high mobility in tailings, RE readily disperse into surrounding agricultural soil through water flow and wind. Li et al. (2013) indicated that, fortunately, the risk assessment of the consumption of vegetables containing RE should not lead to an excess of the daily intake considered harmful to human health. There is still a lack of knowledge about the large-scale effects of RE in the environment, as well as their spatial distribution in urban contexts, where these areas are potentially most at risk.
They are known as “rare earths” because their history dates back to the end of the 18th century when the name “earth” was used for describing alkaline metal oxides and “rare” because they were found in low concentrations in the earth’s crust, insufficient to allow their exploitation (Migaszewski and Gałuszka, 2015). Yet some RE are relatively abundant in the earth’s crust relative to other metals (Table 2). For comparison, average crustal abundances for gold, silver, lead, and copper are 0.004, 0.075, 14, and 60 mg/kg, respectively (USGS, 2014). Each RE has different abundance in the earth crust. The most abundant element is cerium with concentrations higher than those of copper or lead. Disregarding the extremely rare RE, i.e., promethium, all other elements are more abundant than silver, gold or platinum (Van Gosen et al., 2014). Ce are five times as much as lead, 880 times as much as silver and 16,500 as much as gold.
TABLE 2. List of the rare earth (RE) found in natural deposits, classification and their abundance in the earth’s crust [in parts per million (mg/kg)].
Although most RE are not currently rare in terms of total amounts in the earth’s crust, they are unevenly distributed and rarely occurred in sufficient abundance in a single location for their mining to be economically exploitable (Mehmood, 2018). In addition, they are usually found within other minerals such as phosphates, carbonates, fluorides and silicates and occur especially in pegmatites, granites and related metamorphic igneous rocks (Tyler, 2004), making extraction from the ore and processing both highly difficult and expensive. In addition, due to their similar chemical and physical properties, as mentioned above, it makes difficult to separate RE using common chemical methods relies on either an alkaline process that uses concentrated sodium hydroxide or an acidic process that uses concentrated sulfuric acid, both at high temperatures (Jha et al., 2016; Zhang et al., 2016).
Out of 200 known minerals containing RE (Walters and Lusty, 2011), only three are considered to be the main mineral deposits exploitable for extraction, (Gupta and Krishnamurthy, 2004; Tyler, 2004; Binnemans et al., 2013; Xie et al., 2014) i.e., 1) bastnaesites ((Ce, La) (CO3) F) deposits in China and United States. They generally contain mostly light RE and provide more than 70% w/w of RE oxides, 2) monazites [(Ce, La, Nd, Th) PO4; provide 35–71% w/w of RE oxides] deposits in Australia, Brazil, China, India, Malaysia, South Africa, Sri Lanka, Thailand, and the United States. They contain mostly heavy RE and a small amount of the light RE phosphate, 3) xenotimes (YPO4; provide 52–67% w/w of RE oxides). Other instances of minerals known to contain RE include secondary monazite, ion absorption clays, spent uranium solutions, apatite, cheralite, eudialyte, loparite, phosphorites (Zhang et al., 2015; Jha et al., 2016).
According to estimates by the U.S. Geological Survey (2018), the worldwide reserves of RE by principal countries such as China, Brazil, Vietnam, Russia, India and Australia (in decreasing order of available reserves) are approximately 132 million metric tons in terms of rare earth oxides. Most of these reserves are located within China, and are estimated at some 44 million metric tons (US Geological Survey, 2018). China represents one third of the total global mining reserves in RE (33.33%), Brazil and Vietnam concentrate 16.67% of world reserves, followed Russia (13.64%), India (5.23%) and Australia (3.40%) (US Geological Survey, 2018; Balaram, 2019). The main sites current operations are in China (Bayan Obo, Inner Mongolia), United States (Mountain Pass, California) and Australia (Mount Weld, Australia-Western). While the United States was the world’s dominant producer through the 1980’s, China’s low production cost for RE since the mid-1990s significantly reduced the prices of RE globally and has become the greatest world supplier which reportedly produces more than 95% of total RE oxide (Rüttinger and Feil, 2010; Zhang, 2013). As a result, many other mines worldwide stopped extracting these elements (Stone, 2009).
Currently, to protect its industry and to decrease the environmental impacts that may result from RE mining, China has reduced its production and restricted the supply of RE through quotas (Golev et al., 2014; Langkau and Erdmann, 2020). Thus, RE are currently placed on the list of “critical” resource for high technology industrial applications due to importance for strategic sectors of the economy and risks of supply shortage (Massari and Ruberti, 2013; Grabas et al., 2014).
The risk of missing RE led many countries to require secondary resources to meet their needs.
Currently, several secondary resources have been identified as having high RE concentrations including recycling of RE from manufacturing RE scrap and residues (Binnemans et al., 2013; Peelman et al., 2016). Also, recycling of RE from end-of-life products containing RE such us NiMH batteries, permanent magnets, fluorescent lamp phosphors, Fe-Nd-B magnets, Sm-Co magnets, voice coil motors and computer monitors (Binnemans et al., 2013; Zhang et al., 2015). In addition, the extraction of RE from mine waste or industrial landfill by-products (apatite rock, phosphogypsum waste, red mud, RE mineral mine tailings metallurgical slags, coal ash, incinerator ash and waste water streams) has been the focus of a number of studies (Binnemans et al., 2013; Hammas-Nasri et al., 2016; Antonick et al., 2019; Gasser et al., 2019; Masmoudi-Soussi et al., 2019; Jalali et al., 2020a).
RE can also be extracted from trace RE associated with minerals such as uranium ore, fluorite, sea sediments, and phosphate rocks (Chen and Graedel, 2015; Wu et al., 2018). Another way to manage the balance problem is to researching out new RE ore deposits or reopening pre-existing RE mine (Long et al., 2012). However, as above mentioned, RE are very dispersed due to their geochemical characteristics and not usually found concentrated as rare earth minerals in economically exploitable ore deposits (Balaram, 2019). Substitutes of RE in most cases either are inferior alternatives or still undiscovered.
Usually, the treatment of RE minerals involves two operation steps, i.e., a physical treatment and a chemical treatment: 1) Physical processing, also known as mineral enrichment, consists of separating the RE minerals from the gangue minerals to produce a RE concentrate, 2) The chemical treatment (Lin et al., 2017), which includes the extraction and separation processes, converts the RE mineral concentrate into a compound which is either a final or an intermediate product for the subsequent production of individual RE or other compounds. RE extraction processes use one or more regents to decompose the minerals and leach the RE into solution. For the RE separation, several processes can be used such as solvent extraction, ion exchange or chemical precipitation to produce mixed or individual RE oxides (REO). Separation of mixed REO into the individual RE can partially be achieved by multiple recrystallization as double salts, and ultimately by multi-stage solvent extraction (Zhang et al., 2013; Haque et al., 2014).
RE production process must go through a series of complex separation processes to produce each individual element. Although there are several costs associated with RE, it is the separation process that largely dictates the cost of RE production. Recycling of RE from secondary resources offers great opportunities for countries with no primary resources. The different RE processes developed are based on acid or basic leaching stages depending on the type of resource treated, followed by a precipitation step to produce a solid of RE usable for various applications. It is clear that in addition to mitigating some supply risks, recycling of RE can minimize environmental problems related to their extraction and processing. Innovative and profitable technologies, which reduce considerably the environmental impact of all recycling streams compared to the primary extraction route, should be developed.
The costliest processes for primary RE mining route are physical separation and chemical leached of minerals (EPA, 2012), similar processes are also required for many recycling methods (Tanaka et al., 2013). However, the recycling can offer an opportunity to recover the desired elements, specifically targeting products with high concentration of certain valuable RE, on the contrary, with the mining where all the RE elements have to be extracted. Another advantage of RE recycling vs. mining is likely to be associated with the treatment and disposal of radioactive wastes arising from the processing of ore raw materials. Even though there has been minimal study to assess the real significance of radioactive pollution arisen from RE processing (Schmidt, 2013), it is evident that taking into account this issue would position recycling as more advantageous (EPA, 2012).
Recycling, cannot replace primary extraction of minerals and satisfy growing RE demand alone, due in particular to the delay induced by end-of-life products behind current requirements. Consequently, any global analysis of mineral raw material supply should consider both types of resources (Grosse, 2010). In addition, the advantages of producing RE concentrates as a secondary resource are that mining operating costs are low. This processing provides also environmental benefits by neutralizing acid producing steps and reducing the volume of waste storage. However, currently, most of these secondary resources are restricted to academic research due to the difficulties of collection, technique and economy (Borra et al., 2015; Davris et al., 2016).
As above mentioned, the total RE contents of soils vary considerably, depending on the type of soil and of the parent rock from which they are issued (Liu et al., 1988; Xin et al., 2006). In addition, other RE sources in soils are atmospheric depositions (rainwater, atmospheric particles and snow) and spreadings (fertilizers, waste especially phosphogypsum, irrigation and sewage waters (Aubert et al., 2002). The RE content in various components of the environment are shown in Table 3.
The effectiveness of phytoextraction is highly dependent on the availability of metals for plant uptake. In general, a higher availability of RE in soil induce a higher RE uptake by plants, as for all trace elements. The RE availability in soils is related to the water soluble and exchangeable fractions of RE, and thus is dependent on physico-chemical of soil properties such as pH, redox potential (Eh), cation exchange capacity (CEC), and clay content (Shan et al., 2002). For instance, the desorption of RE decreased with increasing equilibrium solution pH (Shan et al., 2002). The adsorption capacity of RE is significantly correlated with the CEC of soil (Shan et al., 2002). Johannesson and Lyons (1995) pointed out the important role of complexing RE with various ligands at different pH levels. RE complexation with carbonate ions predominates in the majority of natural waters with moderate to high pH where carbonates are the main component. Thus RE carbonate complexes dominate and typically account for more than 99% of all RE complexes in solution. At neutral pH, the speciation of the RE in complexes with carbonate includes LnHCO32+, LnCO3+, and Ln(CO3)2-, and that with phosphate LnH2PO42+, LnHPO4+, Ln(HPO4)2-, and LnPO4. In water with a acidic pH, free metal ions dominate the speciation of HRE (Ln3+ account for 60% of total Ln and dominate the LnS04+ complexes) (Johannesson et al., 1996). RE release increases as redox potential and pH decrease (Jones, 1997; Cao et al., 2001). The mobilization of La, Gd, and Y depend mainly upon pH value, whereas the mobilization of Ce depends both upon pH and Eh. RE mobilization is positively correlated with that of Fe and Mn (Cao et al., 2001). Due to their higher mobility in soils, LRE are more easily absorbed and accumulated by plants than heavy RE since the latter form much more stable complexes in soil solution (Brioschi et al., 2013; Grosjean et al., 2020). Indeed, roots preferentially absorb free ions rather than dissolved complexes (Brioschi et al., 2013).
The decontamination of soils enriched with RE still requires costly techniques that sometimes generate associated pollution (use of acids). Phytotechnologies are a relevant alternative, suitable for in situ depollution, making possible to preserve the properties of soils which can then regain their vocation as a support for food production or at least to support plantations. Phytotechnologies go beyond the simple “soft” remediation function of plants for risk management and are more broadly integrated into the concept of phytomanagement (Cundy et al., 2013; Song et al., 2019), which brings together all the benefits that humans can derive from plants. This may involve maintaining or even improving biodiversity in the soil (fauna, micro-organisms), as well as preserving the integrity of the environment (fauna, flora and micro-organisms of concern), the physical integrity of the soil, e.g., by limiting erosion through revegetation. Plant biomass can be harvested and incinerated to recover target metals or to be converted into renewable energy such as biofuel, biochar, charcoal, and essential oils. Plants help to sequester CO2, to regulate its concentration in the atmosphere and limit global warming. Finally, plant management integrates cultural services with the possible reuse of vacant areas up to the creation of vegetated recreational spaces.
RE are not known to be essential for plants (Grosjean et al., 2019) but they can compete with calcium in a number of calcium-mediated biological processes, which could explain for some of the toxicity to plants (Brown et al., 1990). Due to their divalent charges and thus lesser charge density, Ca can probably be displaced by the trivalent RE (Ce, Eu, Gd, Ho, La, Nd, Pr, Tb and Y) at Ca-binding sites in biological molecules. Generally, RE toxicity is considered low. Therefore, at present there are no maximum permissible concentrations or threshold limits in the literature (Thomas et al., 2014).
In general, like other minerals, RE exhibit stimulating action on plant growth at low doses and their toxicity increases with concentration. The concentration of RE to become toxic vary according to the elements (Emmanuel et al., 2010; Zhang et al., 2013; Soudek et al., 2017). In order to evaluate the effect and toxicity of RE on plant growth, the literature provides a certain amount of information concerning the physiological effects of RE on germination and plant growth (Table 4). A greater number of studies have been performed under hydroponic conditions that are not necessarily representatives of real conditions in soil (Thomas et al., 2014).
The main route of RE absorption, as most trace metals (TE), is mainly through root absorption of free ionic forms. After dissolving metal in the rhizosphere, two transport mechanisms of metals within plant have been identified. These are either through the apoplastic (passive diffusion) or symplastic pathways (active transport) (Shan et al., 2003). RE are transferred later to the erial parts (AP) in soluble form. The concentrations and distribution patterns are controlled by wall adsorption cell and phosphate precipitation of RE in the roots or at the roots surface (Ding et al., 2005). This process also partly determines the fractionation of RE in AP. The transfer of metal ions to AP is mainly carried out through the xylem (Marschner et al., 2012). Xylem cells have strong cation exchange capacities. Therefore, the combination with a ligand (organic acids and amino acids such as aspartic acid, glutamic acid, citric acid, malic acid and histidine) promotes the RE absorption and translocation from roots to erial parts. Aspartic acid, asparagine and glutamic acid stimulate the lanthanum and yttrium transport in the xylem of Phytolacca americana (Wu et al., 2009). Histidine increases LRE (such us La, Ce Nd, and Pr) desorption from soil, their uptake into the soil solution and their transport to the upper parts of Dicranopteri dichotoma (Shan et al., 2003). Zygophylum album a wild plant growing in soils in the vicinity to PG stockpiles in Tunisia was shown to be a promising candidate in RE phytoextraction, notably for La (Translocation Factor of 6.12) (Jalali et al., 2019). While the uptake and transport of RE in plants have attracted attention during the last decade, the available information on the functioning of the soil-plant system enriched with these elements is scarce (Hu et al., 2004).
The uptake of RE may be facilitated by increased levels of nitrogen and potassium fertilization. Hypotheses supporting the increase in RE uptake in the presence of Na and K maybe due to direct competition between RE and Ca2+ at uptake sites or perhaps soil interactions prior to uptake but is more probably due to increased plant growth. While there was no such effect in the presence of phosphate fertilization, Brown et al. (1990).
Originally, the main objective of phytoextraction was the depollution of contaminated soils allowing polluted sites and soils rehabilitation (salt et al., 1995). Currently, the domain is more interested in cultivating hyperaccumulators plants to recover accumulated economically valuable metals (Rodrigues et al., 2016). These economic and environmental issues conduct to the creation of a new concept called “phytomining.” It is a phytotechnology that aims to rehabilitate contaminated or naturally metal rich soils by recovering metals of high economic value such us nickel or gold, from cultivated hyperaccumulator plants, that can absorb these elements and transfer them to their AP where they are accumulated, and then to reutilize these metals for industrial purposes (Van der Ent et al., 2015). Phytomining aims to develop a chain of an environmentally friendly process, as it is based on plant growth and recovery that minimizes the impacts on resources (Rodrigues et al., 2016). Initially, this chain consists of developing new agronomic practices to select the best hyperaccumulators plants allowing to optimize the extraction yields of valuable metals from the soil and to maximize the economic income (Brooks, 1998; Barbaroux et al., 2012; Van der Ent et al., 2015). Secondly, hydrometallurgical processes are developed and optimized to transform biomass into marketable metal compounds. The phytomining effectiveness depends on the amount of metals that accumulate in harvested biomass as well as the biomass yield that can be cultivated per year or per season.
To date, phytomining technology has been applied intensively in Ni phytoextraction (Barbaroux et al., 2012; Ye-Tao et al., 2012; Bani et al., 2018), but less attention has been paid to the study of accumulation RE mechanisms and phytomining potential of these elements. It is thus possible to consider the possibility of applying this concept for RE, in particular for the development of hydrometallurgical processes for the recovery and upgrading of metals from biomass. The first discovery of the RE accumulation in plants was reported by Robinson in 1943 in the leaves of Carya cathayensis that reached 2,296 mg/kg DW of total RE (Robinson, 1943). Later, high concentrations of RE have been reported in different plant species. There are difficulties in applying the concept of hyperaccumulator thresholds to the RE (Van der Ent et al., 2021), some study in the literature reported concentrations of total RE whereas others focus on individual elements. Nevertheless, some publications have referred to hyperaccumulation of RE based on a criterion of 0.1 wt% [i.e., 1,000 mg/kg of dry matter (DM)] for the sum of all 17 RE of the AP (Wang et al., 1997; Shan et al., 2003). According to this criterion, to date, 10 species belonging to five families have been identified worldwide as RE hyperaccumulators: Blechnaceae, Gleicheniaceae, Juglandaceae, Phytolaccaceae and Thelypteridaceae (Table 5). In another research, the threshold concentration for RE hyperaccumulators could potentially be lowered to 100 mg/kg DM. Moreover, they are two indexes that describe respectively the metal accumulation ability from soil to plant and the metal transfer ability from roots to AP (Brooks, 1998): The Bioconcentration Factor (BCF) and the Translocation Factor (TF) whereby BCF is the RE concentration in the erial plant parts (in mg/kg of DM) relative to that in the soil or culture solution (mg/kg DM), while TF is the RE concentration in the above-ground parts (mg/kg DM) relative to that in the root part concentration (mg/kg DM). Plant species are considered to exhibit removal potential for an element when the BCF value exceeds 1. Through field investigations, approximately 24 RE hyperaccumulator and potential hyperaccumulator species have been reviewed by Liu et al. (2018) and Van der Ent et al. (2021); these species occur across 10 families all of which are ferns: Adiantaceae, Athyriaceae, Aspleniaceae, Blechnaceae, Dryopteridaceae, Gleicheniaceae, Juglandaceae, Lindsaeaceae, Phytolaccaceae and Thelypteridaceae (Table 5).
TABLE 5. Concentrations of rare earth element (RE) in hyperaccumulator plants (mg/kg)–A hyperaccumulation threshold of 1,000 and 100 mg/kg was adopted for the first 10 species and three species respectively.
Although hyperaccumulators of MTE have been widely studied, less attention has been paid to studying the mechanisms of RE hyperaccumulation and potential for RE phytomining. We suggest that further research is necessary in order to confirm that this is an appropriate hyperaccumulator thresholds to the RE.
The fern species displaying the highest RE accumulation potential is Phytolacca icosandra (13,000 mg/kg DW of total RE; Grosjean et al., 2019), Dicranopteris linearis (Up to 7,000 mg/kg DW of total RE; Shan et al., 2003) and Dicranopteris dicthotoma (3,358 mg/kg DW of Ce, La, Pr, and Nd mixture; Wang et al., 1997). To a lesser extent, other ferns could also accumulate RE, such as Carya tomentosa (1,350 mg/kg DW of total RE; Thomas, 2011), Pronephrium simplex (1,234 mg/kg DW of total RE; Lai et al., 2005), Pronephrium triphyllum (1,027 mg/kg DW of total RE; Xue, 2009), Blechnum orientale (1,022 mg/kg DW of La, Ce, Nd, Sm, Eu, Tb, Yb and Lu mixture; Xiao et al., 2003) and Carya spp. (859 mg/kg DW of total RE; Wood and Grauke, 2011). Phytolacca americana is a highbiomass plant that is naturalized worldwide, and is able to accumulate RE up to 1,040 mg/kg of total RE in dry leaves, (Yuan et al., 2018).
As for most trace elements, the RE distribution among the main organs of vascular plants differs considerably. The roots generally accumulate the most RE. For example, the roots of Zea mays accumulate La concentrations 20–150 times higher than the leaves (Diatloff et al., 1999). Many studies have shown a decrease in RE concentrations in the order: root > leaf > stem > grain or fruits in a variety of crops such as corn, wheat, rice and paprika. In trees like those that produce, e.g., citrus fruits, the highest concentrations of RE are generally found in roots too. The same is true in seven species of tropical trees (Jureima-branca (Piptadenia stipulacea), Faveleira (Cnidoscolum phyllacanthus), Jureima-preta (Mimosa hostilis), Caatingueira (Caesalpinia pyramidalis), Manisoba (Mannihoteae glaziovii), Burra-leiteira (Sapium cicatricosum), and Umbu (Phytolacea dioica L.), where RE are also accumulated in the roots except cerium that tends to be concentrated in the bark (Nakanishi et al., 1997; Tyler, 2004). Among the plants identified, the natural perennial fern D. dichotoma (syn. D. linearis) grown in acidic soil in southern China, is the one that accumulates the most RE in mining areas of China. Li et al. (2010), first reported the accumulation of RE in this plant species in southern Jiangxi province in China. For many years, this plant has proven to be an ideal material for the study of RE in plants (Fa-Shui et al., 1999; Liang et al., 2014). In this plant, the Ce, Dy, La and Nd concentrations are in the order leaf > root > stem while those of Eu, Gd, Ho, Pr, Sm, and Y are in the order root > leaf > stem (Fa-Shui et al., 1999).
Hyperaccumulators such as D. dichotoma often adopt different mechanisms such a defense strategy against metal stress, e.g., compartmentation of metals in vacuoles (Lasat et al., 1998; Yuan et al., 2001), complexing of metal ions with organic ligands (Lee and Kim, 1997) and coordination with histidine (Krämer et al., 1996). The identification of new fern species that are able to accumulate RE is of great interest. A recent study identified new accumulating species RE using a screening method for ferns grown on RE contaminated substrate. Three species reached more than 200 mg/kg: Onoclea sensibilis, Athyrium othophorum and Athyrium filix-femina (Grosjean et al., 2020).
For non-hyperaccumulating plants, i.e., bioindicator plants, whose accumulation level depends on the pool of RE in the soil solution, one way to improve phytoextraction performances consists in promoting the mobilization of RE with the help of microorgnaisms to increase its pool in solution. In addition to that, microorganisms, in particular the Plant Growth Promoting Rizobacteria (PGPR), may improve the plant growth and health.
Microorganisms are involved in all geochemical cycles of TE including RE. While lanthanides can substitute for Ca2+ in some enzymes and tissues, because their similarities in terms of ionic radius (Lim and Franklin, 2006; Pagano et al., 2015), scientists have long considered RE to be inert because of the low solubility of these elements in the environment. Since 2011, it has been discovered that RE such as Ce3+ and La3+ are required for the activity of methanol dehydrogenase enzyme used by some bacteria to oxidize methanol for carbon and energy (Hibi et al., 2011; Pol et al., 2014). Thus, many studies have been undertaken for a better understanding of the interactions between microorganisms and RE, such as RE mobilization from solids through metabolic reactions (Brandl et al., 2016) and RE immobilization from liquids through sorption by biomass. The mobilization of TE by microorganisms from all solid matrix including those containing RE occurs thanks to three mechanisms 1) proton induced solubilization (acidolysis) with the mobilization by formation of organic/inorganic acids, 2) ligand induced solubilization (complexolysis) with the mobilization of RE from mineral surfaces by excretion of complexing agents, and 3) oxidation/reduction reactions (redoxolysis) resulting from the mobilization of RE by oxidative or reductive reactions (Brandl, 2001). Various parameters such as pH, oxidation-reduction conditions, the presence and nature of mineral and organic colloids as well as the presence and activity of microorganisms influence this mobility (Cao et al., 2001).
Since microorganisms have different optimum growth pH, it is essential to identify pH conditions that promote the production of the most beneficial microbial agents for the leaching of RE. For example, under controlled conditions, a fungus isolated (but no identified) from a carbonate rock was shown to be able to mobilize Ce and Eu of a dolomite at acidic pH (Dou and Lian, 2010) while Arthrobacter sp. leads, between pH 6.5 and 7.5, to mobilize all the RE from hornblende (Brantley et al., 2001). In another example, it has been shown that at low pH values of bioleaching cultures (<2) Aspergillus niger produces mainly citric acid, while at higher pH values (>4) it tends to produce gluconic and oxalic acids (Brisson et al., 2016; Rasoulnia and Mousavi, 2016). These acids are well known for their ability to mobilize TE among which RE.
Since most of the RE are found in oxidation state III, they are quickly adsorbed by a large variety of bearing phases in soil, such as oxides and metallic hydroxides. For example, manganese oxides (Mn(III), Mn(IV)) strongly adsorb trivalent RE, in particular Ce(III), much more than La and Pr, and oxidize it to Ce(IV) (Takahashi et al., 2000; Ohta and Kawabe, 2001). These adsorption reactions and oxidation-reduction will also be influenced by the organic matter content and the competition phenomena between ligands. Once mobilized, bacterial interactions with RE will modify their behavior and transfer in the environment. Ohnuki et al. (2015) showed that the adsorption of Ce by P. fluorescens in Ce(III) form prohibited its subsequent oxidation to Ce(IV) by manganese oxides, thus modifying its transfer.
In an attempt to understand the fundamental mechanisms of monazite bioleaching by Enterobacter erogenes, Fathollahzadeh et al. (2018) has demonstrated that the contact of bacteria with minerals can have a significant effect on their capacity to increase mineral dissolution. The ability of phosphate-solubilizing microorganisms to solubilize RE-phosphate minerals can occur by contact mechanisms, non-contact mechanism, or a combination of both. In contact leaching, attached cells solubilize phosphate within a matrix of extracellular polymeric substances and mobilize RE(III) into the solution. Organic acid anions secreted by the cells from organic substrates (e.g., glucose) may complex RE(III). Following proton dissociation of organic acids, free protons also attack the ore surface resulting in further phosphate solubilization. Incorporation of phosphate into the biomass enhances RE(III) solubility. Alternatively (non-contact leaching), attached cells may play a role in organic acid production while suspended cells incorporate phosphate from solution increasing RE(III) solubility.
The potential roles of native bacteria in mobilization of RE from ores, in which the density of these elements is low, are only slightly documented. The extremely varied metabolisms of microorganisms allow them to interact closely with the mineral component of their environment. Microbial extracellular polysaccharides can increase the RE dissolution by complexing the ions of the solution thus lowering the saturation level of the latter (Banfield et al., 1999; Welch et al., 1999). The bacteria can solubilize the metals or increase their bioavailability by the production of siderophores and afterward adsorb the metals on their biomass, on metal-induced outer membrane proteins and by bioprecipitation (Diels et al., 2009). Loges et al. (2012) have shown that siderophores have a very strong affinity for Ce (IV). The formation of RE (La, Sc and Y)-siderophore complexes was observed in Mycobacterium smegmatis by Andrès et al. (1991), as well as the sorption of Eu, La, Th and Yb on the same Bacterial cells (Andrès et al., 1993; Andrès et al., 1995). These same authors observe the sorption of Gd in Bacillus subtilis, Pseudomonas eruginosa, Ralstonia metallidurans CH34 (renamed Cupriavidus metallidurans CH34), M. smegmatis and Saccharomyces cerevisiae (Andrès et al., 2000), La, Eu and Yb in P. eruginosa (Texier et al., 2000; Texier et al., 2002), Ce and Nd in Bacillus cereus, Sc and Sm in Arthrobacter luteolus isolated from an environment rich in RE (Emmanuel et al., 2011; Emmanuel et al., 2012).
Many phosphate solubilizing microorganisms (PSM), such as E. erogenes, Pantoea agglomerans, Pseudomonas putida have demonstrated to grow in the presence of natural RE phosphate minerals, releasing RE, iron and thorium (Corbett et al., 2017). Qu et al. (2019), studied the RE bioleaching performance of chemoheterotrophic bacterium (Acetobacter sp.) from red mud. The results showed that the leaching ratios of Al, Lu, Sc, Th, and Y were 55, 53, 61, 52 and 53% respectively under one-step process at 2% red mud pulp density. Other studies reported that the highest percentages of bioleached total RE from monazite and (Th-U) concentrate directly by Aspergillus ficuum, are found to be 75.4 and 63.8% at a pulp density 0.6 and 1.2% (w/v), respectively, after 9 days and 63.5 and 52.6% by Pseudomonas eruginosa after 8 days (Hassanien et al., 2014). Also, fungal strains able to solubilize phosphate minerals such as A. niger have shown potential for RE bioleaching from monazite (Castro et al., 2020). Several other fungal strains have been also used to leach monazite releasing RE to the aqueous phase such us Aspergillus terreus, Paecilomyces spp. and Penicillum sp. that can release a total concentration of 12.32 mg/L RE (Ce, La, Nd, and Pr) from the weathered monazite after 8 days (Castro et al., 2020). Another study with A. niger reported that the highest rates of RE from monazite were obtained when the fungus was cultivated on minimal medium with the mineral as the sole phosphorous source.
Concentrations of RE leached by other fungal species, such as A. niger and Aspergillus tubigensis, were 24 times lower than Penicillium sp. (0.44 and 0.43 mg/L, respectively). Bacterial species such as P. agglomerans, E. erogenes, and P. putida released bound RE into solution at also quite low concentrations (1.63, 1.93, and 1.45 mg/L, respectively). While the efficiency of the process is low, other bacteria have demonstrated that they were more able to solubilize Ce and La from monazite ore: ca. 5.7 mg/L of Ce (0.13% of leaching efficiency) and ca. 2.8 mg/L of La (0.11%) were leached by Acetobacter aceti, and Azospirillum brasilense, Azospirillum lipoferum, Pseudomonas rhizosphaerae and Mesorhizobium cicero leached 0.5–1 mg/L of both Ce and La (Shin et al., 2015).
In addition, anaerobic bacteria have been applied for the mobilization of RE from solid waste such us phosphogypsum. Almost 80% of Yttrium was mobilized from phosphogypsum in a fixed-bed reactor by Desulfovibrio desulfuricans (Dudeney and Sbai, 1993). Total RE was mobilized from gibbsite samples by Acidithiobacillus ferrooxidans with efficiencies of 67.6% (Brandl et al., 2016).
The combination of native consortia presents on the ore with the inoculation of a PSM leached a greater amount of RE than a single PSM or than the native population alone, due to a syntrophic effect between populations (Corbett et al., 2018). In addition, PSM produce both alkaline and acid phosphatases, either intracellular or secreted extracellularly, enable the hydrolysis of phosphoric compounds and the release of RE into the leachate. The combination action of acid phosphatase activity and organic acids increase RE solubilization in monozite (Corbett et al., 2018). More information for microbial RE mobilization from solid matrices were reviewed by Brandl et al. (2016).
The rhizosphere contains 10–100 times more microorganisms per gram of soil than the non-rhizospheric soil, due to the release of carbon and nitrogen compounds belonging to rhizodeposits (Cunningham and Ow, 1996). Thus, plants have close interaction with rhizospheric microbes, some of which being able to improve their growth and development by increasing the absorption of nutrients and their tolerance to telluric pathogens and various other stresses (Lebeau et al., 2008). Rhizospheric microorganisms can also modify the mobility and availability of metals present in the soil (Abou-Shanab et al., 2003; Bani et al., 2007; Lebeau et al., 2008). PGPR (which stands for Plant Growth-Promoting Rhizobacteria) play a key role in stimulating plant growth and improving the tolerance to abiotic stresses (Dimkpa et al., 2009; Aransiola et al., 2019). Generally, PGPR isolated from the rhizosphere belong to different bacterial families, including Achromobacter, Arthrobacter, Azotobacter, Azospirillum, Bacillus, Burkholderia, Enterobacter, Micrococcus, Pseudomonas, Rhizobium and Serratia (Gray and Smith, 2005; De Zelicourt et al., 2013). These bacteria possess the capacity to increase the plant biomass and/or to mobilize the soil metals. Processes such as chelation and protonation are the main drivers for the mobilization of TE to which RE belong (Kidd et al., 2009; Cabello-Conejo et al., 2014). Some bacteria produce organic acids (oxalate, malate and citrate…) capable to dissolving silicates, thus making metal more available (Khan, 2005; Lebeau et al., 2008; Braud et al., 2009). Thus, the interactions between plant and microorganisms influence the RE mobility and their availability for plants (Sessitsch et al., 2013), promoting the transfer of RE from rhizosphere to plant and therefore contribute to improving phytoextraction performance.
The stimulation of plant biomass results from two actions, the first of which being the production of phytohormones like indole acetic acid (IAA) (Patten and Glick, 2002). A small concentration of IAA in the rhizosphere stimulates the elongation of primary roots (within the young shoots), whereas stronger concentrations inhibit this elongation while promoting a lengthening of the lateral and adventive roots (Xie et al., 1996). The second justification entails the production of enzymes like 1-aminocyclopropane-1-carboxylate (ACC) deaminase. Many PGPR are capable of splitting the ACC produced by plants, a precursor of ethylene, a phytohormone involved in modulating the growth and cellular metabolism of plants (Ping and Boland, 2004). Hence ACC deaminase contribute to reduce the ethylene content for plants subjected to stress, e.g., TE-related stress (Belimov et al., 2005). On average, the use of PGPR reduces the rate of ACC and ethylene by a factor of 2 up to 4 (Belimov et al., 2005).
Modifying metal mobility in the soil can be explained by microbial complexation with microbial metabolites, such as siderophores, some small molecules with a molecular weight ranging from 150 to 2,000 Da. These siderophores are known for their very high affinity for iron from 1023 up to 1052 M−1 (Hernlem et al., 1996; Hider and Kong, 2010; Khan et al., 2018). They are produced by various microorganisms when iron is in short supply [<10 μM (De Villegas et al., 2002; Braud et al., 2009)] for the purpose of solubilizing and acquiring this vital metal of limited bioavailability. The roots can thus incorporate the iron from bacterial siderophore-iron complexes by a mechanism of either chelator degradation accompanying the iron release or a direct incorporation of these complexes (Rajkumar et al., 2010). The siderophores, like pyoverdine and pyochelin, can also complex other metals, e.g., copper (Cu) and nickel (Ni) (Braud et al., 2009).
The slowness of phytoextraction constitutes its main drawback, which is sometimes prohibitive. Among various solutions to increase the phytoextraction rates, the association of microorganisms (bioaugmentation) with plants (phytoextraction), in particular PGPR has been the subject of growing interest for the past ten years (Lebeau et al., 2008; Sessitsch et al., 2013; Xu et al., 2014). In both natural and anthropized ecosystems, bacteria associated with plants, in addition to stimulating their growth, play a key role in the adaptation of their hosts to changing environments (Ma et al., 2011). Different direct mechanisms (solubilization of phosphorus and potassium, nitrogen fixation, iron sequestration by siderophores, hormone production) or indirect (reduction in the number of pathogens by the production of antibiotics as well as the iron available in the rhizosphere for phytopathogens) are involved (Glick, 1995; Lucy et al., 2004). The production of phytohormones by bacteria associated with plants, such as auxin (indole-3-acetic acid, AIA), cytokinins and gibberellins, frequently leads to stimulation of germination, growth and reproduction while by protecting the plant from both biotic and abiotic stresses (Taghavi et al., 2009). Although several conditions, including stimulation of plant growth, accumulation and tolerance to metals, can effectively enhance phytoextraction rates, the concentration of metals available in the rhizosphere greatly influences the amount of metals accumulated in the plants. Indeed, a significant proportion of TE is generally related to organic or inorganic constituents in polluted soils and their phytoavailability is closely linked to their chemical speciation (McBride, 1989). Nevertheless, the metabolites released by bacteria in the rhizosphere (siderophores, acids organic, plant growth regulators) can promote or, on the contrary, alter the uptake by the plant. According to the literature, in a contaminated medium the plant/bacteria association can increase, in 60% of studies, the biomass of the plant by 1.2–4 times and/or the metal concentration in the leaves from 1.1 to 3.1 times (Lebeau et al., 2008). The microorganisms used in bioaugmentation can result from selections made from environmental samples (soil, sediment, waste, sludge, etc.) (Vogel, 1996). The use of bacteria capable of mobilizing TE and/or stimulating plant development (PGPR properties) may serve to increase the amount of TE extracted in this manner and hence the efficiency of phytoextraction. The microorganisms used for the bioaugmentation of metal-contaminated soil may be sourced using selections among environmental samples (Bois et al., 2011; Lebeau, 2011). They are then cultivated and inoculated in either their native environment or another environment. For RE, Jalali et al. (2020a) selected bacteria derived from contaminated soils in the vicinity of phosphogypsum stockpiles for TE including RE (Ce, La, Nd and Y) mobilization (siderophore production) and plant growth promotion (IAA, ACC deaminase production). Attention has also been paid to the use of genetically modified microorganisms for bioaugmentation, but their introduction into the environment must be carefully controlled (Sayler and Ripp, 2000).
Despite the growing concern on the environmental impact of an increasing use of RE in agriculture and their effects on plant growth and productivity (Reddy et al., 2001; Tyler, 2004; Balaram, 2019), only a few studies have been devoted to extraction RE using coupled bioaugmentation-phytoextraction which have taken into account, only the effect of bacteria on the biomass. Treatment with Arbuscular mycorrhizal fungi significantly increase N, P, and K content in shoots and roots of Astragalus sinicus and growth of Zea mays and Sorghum bicolor in soil enriched with RE (Chen and Zhao, 2009; Guo et al., 2013). The dry weight biomass of the investigated plants increase by 211% up to 387% in shoots and roots as compared with the non-inoculated treatment. Trifi et al. (2017) revealed that Pantoea sp. BRM17, an isolated bacterium of Tunisian phosphgypsum, produces siderophores, IAA and ACC deaminase. Its addition to a soil amended with 2% PG has enabled increasing the biomass of the stems and roots of cultivated Brassica napus. A recent study by Jalali et al. (2020a, 2020b) reported that Bacillus as PGPR promoted barley seedlings growth mainly via production of organic acid, ACC deaminase and the association of Trifolium pratense, with Bacillus sp. stimulate the growth of this plant (i.e., factors of 3.7 for in AP and 3.1 in roots) and while increasing the Cd, Sr and RE (Ce, La, Nd, Sr and Y) concentrations respectively by factors of 6.4, 0.6, 0.93, 0.58 and 5.5 in AP. Also, Bacillus sp. strain is especially promising, in association with Helianthus annuus, for Ce, La, Nd and Y, with plant concentrations being raised by factors of 4.4, 38.3, 3.4 and 21, respectively in the AP. A major increase was also observed in the AP biomass of H. annuus by factors of 3.36. In addition, the translocation factor was increased for all MTE and is ranged between 1.1 for Sr and 6.8 for Y.
Conventional extraction and recovery of RE by physico-chemical methods has three major limitations: 1) extraction of RE is via chemical treatment using strong acidic and alkali solutions at high temperature, 2) RE are found mixed in the ore, and thus chemical separation of each element leads to an overall inefficient recovery, and 3) the extraction efficiency is dependent on ores containing high concentrations of RE, which prevents the recovery of less concentrated sources of RE. Green and efficient extraction techniques are receiving increasing attention. Bioaugmentation-enhanced RE phytomining is an innovative technology that offers an alternative to physico-chemical methods of recycling RE, which could contribute to a more sustainable world and help to avoid risks of supply disruption and market dependencies.
Based on the limited reports of the association of bioaugmentation with phytoextraction for the treatment of RE, the review highlights the need for more detailed studies on this process. It has been shown the phytoextraction potential of various plants, but from a more fundamental point of view, the study of the mechanisms involved in its tolerance to RE could be investigated more in depth. It would especially be relevant to study more accurately the mobilization of RE by bacteria. Their ability to survive, colonize the soil, and maintain their PGPR and RE mobilization activities must be specified. From an application point of view of the process associating bioaugmentation with phytoextraction, several studies can be undertaken, in particular the identification of local spontaneous plant species with high biomass capable of accumulating RE and implementing technical routes (from seed collection to sowing and harvesting). Also, the use of wild plant growing in soils containing RE co-cultures with plants commonly used in phytoextraction of theis elements could increase the efficiency of phytoextraction.
As the main factor of failure of bioaugmentation is the stress to which the microorganisms inoculated in the soil or the growing medium are subjected, the optimization of the survival of these microorganisms and their colonization of the rhizosphere is of paramount importance. Inoculation modalities could be tested such as the inoculation technique (free or immobilized microorganisms); the direct coating of the seeds would also be tested.
A final perspective would be to develop and optimize processes for valuing the plant biomass collected and recovering RE, like what has been developed for nickel (phytoming, agromining and recovery of Ni sulfate).
JJ wrote the first draft of the review. TL has initiated this review, helped structure it, proofread it, correct and complete it.
This research was funded by the Region of Pays de la Loire. The funding includes the open access publication fees.
The authors declare that the research was conducted in the absence of any commercial or financial relationships that could be construed as a potential conflict of interest.
Abou-Shanab, R. A., Angle, J. S., Delorme, T. A., Chaney, R. L., Van Berkum, P., Moawad, H., et al. (2003). Rhizobacterial Effects on Nickel Extraction from Soil and Uptake by Alyssum Murale. New Phytol. 158 (1), 219–224. doi:10.1046/j.1469-8137.2003.00721.x
Andrès, Y., MacCordick, H. J., and Hubert, J.-C. (1993). Adsorption of Several Actinide (Th, U) and Lanthanide (La, Eu, Yb) Ions by Mycobacterium Smegmatis. Appl. Microbiol. Biotechnol. 39, 413–417. doi:10.1007/BF00192103
Andrès, Y., MacCordick, H. J., and Hubert, J.-C. (1991). Complexes of Mycobactin fromMycobacterium Smegmatis with Scandium, Yttrium and Lanthanum. Biol. Met. 4 (4), 207–210. doi:10.1007/BF01141182
Andrès, Y., MacCordick, H. J., and Hubert, J. C. (1995). Selective Biosorption of Thorium Ions by an Immobilized Mycobacterial Biomass. Appl. Microbiol. Biotechnol. 44, 271–276. doi:10.1007/s002530050553
Andrès, Y., Thouand, G., Boualam, M., and Mergeay, M. (2000). Factors Influencing the Biosorption of Gadolinium by Micro-organisms and its Mobilisation from Sand. Appl. Microbiol. Biotechnol. 54, 262–267. doi:10.1007/s002530000368
Antonick, P. J., Hu, Z., Fujita, Y., Reed, D. W., Das, G., Wu, L., et al. (2019). Bio- and mineral Acid Leaching of Rare Earth Elements from Synthetic Phosphogypsum. The J. Chem. Thermodynamics 132, 491–496. doi:10.1016/j.jct.2018.12.034
Aransiola, S. A., Ijah, U. J. J., Abioye, O. P., and Bala, J. D. (2019). Microbial-aided Phytoremediation of Heavy Metals Contaminated Soil: a Review. Eur. J. Biol. Res. 9 (2), 104–125. doi:10.5281/zenodo.3244176
Aubert, D., Stille, P., Probst, A., Gauthier-Lafaye, F., Pourcelot, L., and Del nero, M. (2002). Characterization and Migration of Atmospheric REE in Soils and Surface Waters. Geochimica et Cosmochimica Acta 66 (19), 3339–3350. doi:10.1016/S0016-7037(02)00913-4
Babula, P., Adam, V., Opatrilova, R., Zehnalek, J., Havel, L., and Kizek, R. (2009). “Uncommon Heavy Metals, Metalloids and Their Plant Toxicity: a Review,” in Organic Farming, Pest Control and Remediation of Soil Pollutants, 275–317. doi:10.1007/978-1-4020-9654-9_14
Balaram, V. (2019). Rare Earth Elements: A Review of Applications, Occurrence, Exploration, Analysis, Recycling, and Environmental Impact. Geosci. Front. 10 (4), 1285–1303. doi:10.1016/j.gsf.2018.12.005
Banfield, J. F., Barker, W. W., Welch, S. A., and Taunton, A. (1999). Biological Impact on mineral Dissolution: Application of the Lichen Model to Understanding mineral Weathering in the Rhizosphere. Proc. Natl. Acad. Sci. 96 (7), 3404–3411. doi:10.1073/pnas.96.7.3404
Bani, A., Echevarria, G., Pavlova, D., Shallari, S., Morel, J. L., and Sulçe, S. (2018). “Element Case Studies: Nickel,” in Agromining: Farming for Metals (Cham: Springer), 221–232. doi:10.1007/978-3-319-61899-9_12
Bani, A., Echevarria, G., Sulçe, S., Morel, J. L., and Mullai, A. (2007). In-situ Phytoextraction of Ni by a Native Population of Alyssum Murale on an Ultramafic Site (Albania). Plant Soil 293 (1), 79–89. doi:10.1007/s11104-007-9245-1
Barbaroux, R., Plasari, E., Mercier, G., Simonnot, M. O., Morel, J. L., and Blais, J. F. (2012). A New Process for Nickel Ammonium Disulfate Production from Ash of the Hyperaccumulating Plant Alyssum Murale. Sci. Total Environ. 423, 111–119. doi:10.1016/j.scitotenv.2012.01.063
Belimov, A. A., Hontzeas, N., Safronova, V. I., Demchinskaya, S. V., Piluzza, G., Bullitta, S., et al. (2005). Cadmium-tolerant Plant Growth-Promoting Bacteria Associated with the Roots of Indian Mustard (Brassica Juncea L. Czern.). Soil Biol. Biochem. 37 (2), 241–250. doi:10.1016/j.soilbio.2004.07.033
Binnemans, K., Jones, P. T., Blanpain, B., Van Gerven, T., Yang, Y., Walton, A., et al. (2013). Recycling of Rare Earths: a Critical Review. J. Clean. Prod. 51, 1–22. doi:10.1016/j.jclepro.2012.12.037
Bois, P., Huguenot, D., Norini, M.-P., Ul Haque, M. F., Vuilleumier, S., and Lebeau, T. (2011). Herbicide Degradation and Copper Complexation by Bacterial Mixed Cultures from a Vineyard Stormwater basin. J. Soils Sediments 11 (5), 860–873. doi:10.1007/s11368-011-0354-3
Borra, C. R., Pontikes, Y., Binnemans, K., and Van Gerven, T. (2015). Leaching of Rare Earths from bauxite Residue (Red Mud). Minerals Eng. 76, 20–27. doi:10.1016/j.mineng.2015.01.005
Brandl, H., Barmettler, F., Castelberg, C., and Fabbri, C. (2016). Microbial Mobilization of Rare Earth Elements (REE) from mineral Solids: a Mini Review. AIMS Microbiol. 3 (2), 190–204. doi:10.1007/s12275-014-3532-3
Brandl, H. (2001). Microbial Leaching of Metals. Biotechnology 10, 191–224. doi:10.1002/9783527620999.ch8k
Brantley, S. L., Liermann, L., Bau, M., and Wu, S. (2001). Uptake of Trace Metals and Rare Earth Elements from Hornblende by a Soil Bacterium. Geomicrobiology J. 18 (1), 37–61. doi:10.1080/01490450151079770
Braud, A., Hoegy, F., Jezequel, K., Lebeau, T., and Schalk, I. J. (2009). New Insights into the Metal Specificity of thePseudomonas Aeruginosapyoverdine-Iron Uptake Pathway. Environ. Microbiol. 11 (5), 1079–1091. doi:10.1111/j.1462-2920.2008.01838.x
Briner, W., Rycek, R. F., Moellenberndt, A., and Dannull, K. (2000). Neurodevelopmental Effects of Lanthanum in Mice. Neurotoxicology and teratology 22 (4), 573–581. doi:10.1016/s0892-0362(00)00075-1
Brioschi, L., Steinmann, M., Lucot, E., Pierret, M. C., Stille, P., Prunier, J., et al. (2013). Transfer of Rare Earth Elements (REE) from Natural Soil to Plant Systems: Implications for the Environmental Availability of Anthropogenic REE. Plant Soil 366 (1-2), 143–163. doi:10.1007/s11104-012-1407-0
Brisson, V. L., Zhuang, W.-Q., and Alvarez-Cohen, L. (2016). Bioleaching of Rare Earth Elements from Monazite Sand. Biotechnol. Bioeng. 113 (2), 339–348. doi:10.1002/bit.25823
Brooks, R. R. (1998). Plants that Hyperaccumulate Heavy Metals: Their Role in Phytoremediation, Microbiology. Archaeology 380. doi:10.1002/bit.25823
Brown, P. H., Rathjen, A. H., Graham, R. D., and Tribe, D. E. (1990). Chapter 92 Rare Earth Elements in Biological Systems. Handbook Phys. Chem. rare earths 13, 423–452. doi:10.1016/S0168-1273(05)80135-7
Cabello-Conejo, M. I., Becerra-Castro, C., Prieto-Fernández, A., Monterroso, C., Saavedra-Ferro, A., Mench, M., et al. (2014). Rhizobacterial Inoculants Can Improve Nickel Phytoextraction by the Hyperaccumulator Alyssum Pintodasilvae. Plant Soil 379 (1), 35–50. doi:10.1007/s11104-014-2043-7
Cao, X., Chen, Y., Wang, X., and Deng, X. (2001). Effects of Redox Potential and pH Value on the Release of Rare Earth Elements from Soil. Chemosphere 44 (4), 655–661. doi:10.1016/S0045-6535(00)00492-6
Castro, L., Blázquez, M. L., González, F., and Muñoz, J. A. (2020). Bioleaching of Phosphate Minerals Using Aspergillus niger: Recovery of Copper and Rare Earth Elements. Metals 10 (7), 978. doi:10.3390/met10070978
Chakhmouradian, A. R., and Wall, F. (2012). Rare Earth Elements: Minerals, Mines, Magnets (And More). Elements 8 (5), 333–340. doi:10.2113/gselements.8.5.333
Challaraj Emmanuel, E. S., Vignesh, V., Anandkumar, B., and Maruthamuthu, S. (2011). Bioaccumulation of Cerium and Neodymium by Bacillus Cereus Isolated from Rare Earth Environments of Chavara and Manavalakurichi, India. Indian J. Microbiol. 51 (4), 488–495. doi:10.1007/s12088-011-0111-8
Chen, M., and Graedel, T. E. (2015). The Potential for Mining Trace Elements from Phosphate Rock. J. Clean. Prod. 91, 337–346. doi:10.1016/j.jclepro.2014.12.042
Chen, X.-H., and Zhao, B. (2009). Arbuscular Mycorrhizal Fungi Mediated Uptake of Nutrient Elements by Chinese Milk Vetch (Astragalus sinicus L.) Grown in Lanthanum Spiked Soil. Biol. Fertil. Soils 45 (6), 675–678. doi:10.1007/s00374-009-0379-6
Corbett, M. K., Eksteen, J. J., Niu, X.-Z., Croue, J.-P., and Watkin, E. L. J. (2017). Interactions of Phosphate Solubilising Microorganisms with Natural Rare-Earth Phosphate Minerals: a Study Utilizing Western Australian Monazite. Bioproc. Biosyst Eng 40 (6), 929–942. doi:10.1007/s00449-017-1757-3
Corbett, M. K., Eksteen, J. J., Niu, X.-Z., and Watkin, E. L. J. (2018). Syntrophic Effect of Indigenous and Inoculated Microorganisms in the Leaching of Rare Earth Elements from Western Australian Monazite. Res. Microbiol. 169 (10), 558–568. doi:10.1016/j.resmic.2018.05.007
Cundy, A. B., Bardos, R. P., Church, A., Puschenreiter, M., Friesl-Hanl, W., Müller, I., et al. (2013). Developing Principles of Sustainability and Stakeholder Engagement for “Gentle” Remediation Approaches: The European Context. J. Environ. Manage. 129, 283–291. doi:10.1016/j.jenvman.2013.07.032
Cunningham, S. D., and Ow, D. W. (1996). Promises and Prospects of Phytoremediation. Plant Physiol. 110 (3), 715–719. doi:10.1104/pp.110.3.715
d'Aquino, L., De Pinto, M. C., Nardi, L., Morgana, M., and Tommasi, F. (2009). Effect of Some Light Rare Earth Elements on Seed Germination, Seedling Growth and Antioxidant Metabolism in Triticum Durum. Chemosphere 75 (7), 900–905. doi:10.1016/j.chemosphere.2009.01.026
Damhus, T., Hartshorn, R. M., and Hutton, A. T. (2005). Nomenclature of Inorgaic Chemistry: IUPAC Recommendations 2005. Northampton, United Kingdom: Chemistry International.
Davris, P., Balomenos, E., Panias, D., and Paspaliaris, I. (2016). Selective Leaching of Rare Earth Elements from bauxite Residue (Red Mud), Using a Functionalized Hydrophobic Ionic Liquid. Hydrometallurgy 164, 125–135. doi:10.1016/j.hydromet.2016.06.012
De Villegas, M. E. D., Villa, P., and Frías, A. (2002). Evaluation of the Siderophores Production by Pseudomonas aeruginosa PSS. Revista Latinoamericana de Microbiologia 44 (3-4), 112–117. doi:10.1590/S1517-83822012000200028
De Zelicourt, A., Al-Yousif, M., and Hirt, H. (2013). Rhizosphere Microbes as Essential Partners for Plant Stress Tolerance. Mol. Plant 6 (2), 242–245. doi:10.1093/mp/sst028
Diatloff, E., Asher, C. J., and Smith, F. W. (1999). “The Effects of Rare Earth Elements on the Growth and Nutrition of Plants,” in Materials Science Forum (Switzerland: Trans Tech Publications Ltd), 354–360. doi:10.4028/www.scientific.net/msf.315-317.354
Diatloff, E., Smith, F. W., and Asher, C. J. (2008). Effects of Lanthanum and Cerium on the Growth and mineral Nutrition of Corn and Mungbean. Ann. Bot. 101 (7), 971–982. doi:10.1093/aob/mcn021
Diels, L., Van Roy, S., Taghavi, S., and Van Houdt, R. (2009). From Industrial Sites to Environmental Applications with Cupriavidus Metallidurans. Antonie Van Leeuwenhoek 96 (2), 247–258. doi:10.1007/s10482-009-9361-4
Dimkpa, C. O., Merten, D., Svatoš, A., Büchel, G., and Kothe, E. (2009). Siderophores Mediate Reduced and Increased Uptake of Cadmium byStreptomyces tendaeF4 and sunflower (Helianthus Annuus), Respectively. J. Appl. Microbiol. 107 (5), 1687–1696. doi:10.1111/j.1365-2672.2009.04355.x
Ding, S., Liang, T., Zhang, C., Yan, J., and Zhang, Z. (2005). Accumulation and Fractionation of Rare Earth Elements (REEs) in Wheat: Controlled by Phosphate Precipitation, Cell wall Absorption and Solution Complexation. J. Exp. Bot. 56 (420), 2765–2775. Epub 2005 Aug 30. doi:10.1093/jxb/eri270
Dou, C. W., and Lian, B. (2010). Role of Rock-Inhabiting Fungi in Dissolution, Migration and Concentration of Rare Earth Elements in Dolomite. Bull. Mineralogy Petrol. Geochem. 29, 57–62. doi:10.1080/11263504.2013.877533
Dudeney, A. W. L., and Sbai, M. L. (1993). “Bioleaching of Rare-Earth-Bearing Phosphogypsum,” in Biohydrometallurgical Technologies. Editors A. E. Torma, J. E. Wey, and V. L. Lakshmanan (Jackson Hole: The Minerals, Metals, and Materials Society), 39–47.
Emmanuel, E. C., Ananthi, T., Anandkumar, B., and Maruthamuthu, S. (2012). Accumulation of Rare Earth Elements by Siderophore-Forming Arthrobacter Luteolus Isolated from Rare Earth Environment of Chavara, India. J. Biosci. 37 (1), 25–31. doi:10.1007/s12038-011-9173-3
Emmanuel, E. S., Ramachandran, A. M., Ravindran, A., Natesan, M., and Maruthamuthu, S. (2010). Effect of Some Rare Earth Elements on Dry Matter Partitioning, Nodule Formation and Chlorophyll Content in Arachis hypogaea L. Plants. Aust. J. Crop Sci. 4 (9), 670. doi:10.3316/informit.859297716662769
EPA (2012). Rare Earth Elements, A Review of Production, Processing, Recycling, and Associated Environmental Issues.
Fa-Shui, H. O. N. G., Zheng-Gui, W. E. I., Ye, T. A. O., Shou-Kang, W. A. N., Yue-Tao, Y. A. N. G., Xin-De, C. A. O., et al. (1999). Distribution of Rare Earth Elements and Structure Characterization of Chlorophyll-Lanthanum in a Natural Plant Fern Dicranopteris Dichotoma. J. Integr. Plant Biol. 41 (8).
Fathollahzadeh, H., Hackett, M. J., Khaleque, H. N., Eksteen, J. J., Kaksonen, A. H., and Watkin, E. L. (2018). Better Together: Potential of Co-culture Microorganisms to Enhance Bioleaching of Rare Earth Elements from Monazite. Bioresour. Tech. Rep. 3, 109–118. doi:10.1016/j.biteb.2018.07.003
Fu, Y., Li, F., Xu, T., Cai, S., Chu, W., Qiu, H., et al. (2014). Bioaccumulation, Subcellular, and Molecular Localization and Damage to Physiology and Ultrastructure in Nymphoides Peltata (Gmel.) O. Kuntze Exposed to Yttrium. Environ. Sci. Pollut. Res. 21 (4), 2935–2942. doi:10.1007/s11356-013-2246-0
Ganguli, R., and Cook, D. R. (2018). Rare Earths: A Review of the Landscape. MRS Energy & Sustainability 5. doi:10.1557/mre.2018.7
Gasser, M. S., Ismail, Z. H., Abu Elgoud, E. M., Abdel Hai, F., Ali, O. I., and Aly, H. F. (2019). Process for Lanthanides-Y Leaching from Phosphogypsum Fertilizers Using Weak Acids. J. Hazard. Mater. 378, 120762. doi:10.1016/j.jhazmat.2019.120762
Glick, B. R. (1995). The Enhancement of Plant Growth by Free-Living Bacteria. Can. J. Microbiol. 41, 109–117. doi:10.1139/m95-015
Golev, A., Scott, M., Erskine, P. D., Ali, S. H., and Ballantyne, G. R. (2014). Rare Earths Supply Chains: Current Status, Constraints and Opportunities. Resour. Pol. 41, 52–59. doi:10.1016/j.resourpol.2014.03.004
Grabas, K., Pawełczyk, A., Ostrowski, A., Stręk, S., Stręk, W., Hreniak, D., et al. (2014). “September. Recovery of Lanthanides from Apatite Phosphogypsum,” in COM 2014-Conference of Metallurgists Proceedings. doi:10.13140/2.1.1697.5360
Gray, E. J., and Smith, D. L. (2005). Intracellular and Extracellular PGPR: Commonalities and Distinctions in the Plant-Bacterium Signaling Processes. Soil Biol. Biochem. 37 (3), 395–412. doi:10.1016/j.soilbio.2004.08.030
Greenwood, N. N., and Earnshaw, A. (1984). Chemistry of the Elements. Oxford: Pergamon Press. doi:10.1002/ange.19850970833
Grosjean, N., Blaudez, D., Chalot, M., Gross, E. M., and Le Jean, M. (2020). Identification of New hardy Ferns that Preferentially Accumulate Light Rare Earth Elements: a Conserved Trait within Fern Species. Environ. Chem. 17 (2), 191–200. doi:10.1071/en19182
Grosjean, N., Le Jean, M., Berthelot, C., Chalot, M., Gross, E. M., and Blaudez, D. (2019). Accumulation and Fractionation of Rare Earth Elements Are Conserved Traits in the Phytolacca Genus. Sci. Rep. 9 (1), 1–12. doi:10.1038/s41598-019-54238-3
Grosse, F. (2010). Is Recycling “Part of the Solution”? the Role of Recycling in an Expanding Society and a World of Finite Resources. SAPI EN. S. Surv. Perspect. Integrating Environ. Soc. 3 (1).
Guo, W., Zhao, R., Zhao, W., Fu, R., Guo, J., Bi, N., et al. (2013). Effects of Arbuscular Mycorrhizal Fungi on maize (Zea mays L.) and Sorghum (Sorghum Bicolor L. Moench) Grown in Rare Earth Elements of Mine Tailings. Appl. Soil Ecol. 72, 85–92. doi:10.1016/j.apsoil.2013.06.001
Gupta, C. K., and Krishnamurthy, N. (2004). Extractive Metallurgy of Rare Earths. Trombay, Bombay: CRC Press. doi:10.1179/imr.1992.37.1.197
Guyonnet, D., Planchon, M., Rollat, A., Escalon, V., Tuduri, J., Charles, N., et al. (2015). Material Flow Analysis Applied to Rare Earth Elements in Europe. J. Clean. Prod. 107, 215–228. doi:10.1016/j.jclepro.2015.04.123
Gwenzi, W., Mangori, L., Danha, C., Chaukura, N., Dunjana, N., and Sanganyado, E. (2018). Sources, Behaviour, and Environmental and Human Health Risks of High-Technology Rare Earth Elements as Emerging Contaminants. Sci. Total Environ. 636, 299–313. doi:10.1016/j.scitotenv.2018.04.235
Hammas-Nasri, I., Horchani-Naifer, K., Férid, M., and Barca, D. (2016). Rare Earths Concentration from Phosphogypsum Waste by Two-step Leaching Method. Int. J. Mineral Process. 149, 78–83. doi:10.1016/j.minpro.2016.02.011
Haque, N., Hughes, A., Lim, S., and Vernon, C. (2014). Rare Earth Elements: Overview of Mining, Mineralogy, Uses, Sustainability and Environmental Impact. Resources 3 (4), 614–635. doi:10.3390/resources3040614
Hassanien, W. A. G., Desouky, O. A. N., and Hussien, S. S. E. (2014). Bioleaching of Some Rare Earth Elements from Egyptian Monazite Using Aspergillus Ficuum and Pseudomonas aeruginosa. Walailak J. Sci. Tech. (Wjst) 11 (9), 809–823. doi:10.14456/WJST.2014.85
Hatch, G. P. (2011). Critical Rare Earths: Global Supply and Demand Projections and the Leading Contenders for New Sources of Supply. Carpentersville: Technology Metals Research, LLC. doi:10.2118/140391-ms
He, Y.-W., and Loh, C.-S. (2000). Cerium and Lanthanum Promote floral Initiation and Reproductive Growth of Arabidopsis thaliana. Plant Sci. 159 (1), 117–124. doi:10.1016/S0168-9452(00)00338-1
Henderson, P. (1984). “Rare Earth Element Geochemistry,” in Developments in Geochemistry (Amsterdam: Elsevier), 510.
Hernlem, B. J., Vane, L. M., and Sayles, G. D. (1996). Stability Constants for Complexes of the Siderophore Desferrioxamine B with Selected Heavy Metal Cations. Inorg. Chim. Acta 244 (2), 179–184. doi:10.1016/0020-1693(95)04780-8
Hibi, Y., Asai, K., Arafuka, H., Hamajima, M., Iwama, T., and Kawai, K. (2011). Molecular Structure of La3+-Induced Methanol Dehydrogenase-like Protein in Methylobacterium Radiotolerans. J. Biosci. Bioeng. 111 (5), 547–549. doi:10.1016/j.jbiosc.2010.12.017
Hider, R. C., and Kong, X. (2010). Chemistry and Biology of Siderophores. Nat. Prod. Rep. 27 (5), 637–657. doi:10.1039/B906679A
Hu, X., Ding, Z., Chen, Y., Wang, X., and Dai, L. (2002). Bioaccumulation of Lanthanum and Cerium and Their Effects on the Growth of Wheat (Triticum aestivum L.) Seedlings. Chemosphere 48 (6), 621–629. doi:10.1016/s0045-6535(02)00109-1
Hu, Z., Richter, H., Sparovek, G., and Schnug, E. (2004). Physiological and Biochemical Effects of Rare Earth Elements on Plants and Their Agricultural Significance: a Review. J. Plant Nutr. 27 (1), 183–220. doi:10.1081/PLN-120027555
Ichihashi, H., Morita, H., and Tatsukawa, R. (1992). Rare Earth Elements (REEs) in Naturally Grown Plants in Relation to Their Variation in Soils. Environ. Pollut. 76 (2), 157–162. doi:10.1016/0269-7491(92)90103-h
Jalali, J., Gaudin, P., Ammar, E., and Lebeau, T. (2020b). Bioaugmentation Coupled with Phytoextraction for the Treatment of Cd and Sr, and Reuse Opportunities for Phosphogypsum Rare Earth Elements. J. Hazard. Mater. 399, 122821. doi:10.1016/j.jhazmat.2020.122821
Jalali, J., Gaudin, P., Capiaux, H., Ammar, E., and Lebeau, T. (2019). Fate and Transport of Metal Trace Elements from Phosphogypsum Piles in Tunisia and Their Impact on Soil Bacteria and Wild Plants. Ecotoxicology Environ. Saf. 174, 12–25. doi:10.1016/j.ecoenv.2019.02.051
Jalali, J., Gaudin, P., Capiaux, H., Ammar, E., and Lebeau, T. (2020a). Isolation and Screening of Indigenous Bacteria from Phosphogypsum-Contaminated Soils for Their Potential in Promoting Plant Growth and Trace Elements Mobilization. J. Environ. Manage. 260, 110063. doi:10.1016/j.jenvman.2020.110063
Jha, M. K., Kumari, A., Panda, R., Rajesh Kumar, J., Yoo, K., and Lee, J. Y. (2016). Review on Hydrometallurgical Recovery of Rare Earth Metals. Hydrometallurgy 165, 2–26. doi:10.1016/j.hydromet.2016.01.035
Johannesson, K. H., and Lyons, W. B. (1995). Rare-earth Element Geochemistry of Colour Lake, an Acidic Freshwater lake on Axel Heiberg Island, Northwest Territories, Canada. Chem. Geology. 119 (1-4), 209–223. doi:10.1016/0009-2541(94)00099-T
Johannesson, K. H., Stetzenbach, K. J., Hodge, V. F., and Lyons, W. B. (1996). Rare Earth Element Complexation Behavior in Circumneutral pH Groundwaters: Assessing the Role of Carbonate and Phosphate Ions. Earth Planet. Sci. Lett. 139 (1-2), 305–319. doi:10.1016/0012-821X(96)00016-7
Jones, D. L. (1997). Trivalent Metal (Cr, Y, Rh, La, Pr, Gd) Sorption in Two Acid Soils and its Consequences for Bioremediation. Eur. J. Soil Sci. 48, 697–702. doi:10.1046/j.1365-2389.1997.00116.x
Khan, A. G. (2005). Role of Soil Microbes in the Rhizospheres of Plants Growing on Trace Metal Contaminated Soils in Phytoremediation. J. trace Elem. Med. Biol. 18 (4), 355–364. doi:10.1016/j.jtemb.2005.02.006
Khan, A., Singh, P., and Srivastava, A. (2018). Synthesis, Nature and Utility of Universal Iron Chelator - Siderophore: A Review. Microbiol. Res. 212-213, 103–111. doi:10.1016/j.micres.2017.10.012
Kidd, P., Barceló, J., Bernal, M. P., Navari-Izzo, F., Poschenrieder, C., Shilev, S., et al. (2009). Trace Element Behaviour at the Root-Soil Interface: Implications in Phytoremediation. Environ. Exp. Bot. 67 (1), 243–259. doi:10.1016/j.envexpbot.2009.06.013
Krämer, U., Cotter-Howells, J. D., Charnock, J. M., Baker, A. J. M., and Smith, J. A. C. (1996). Free Histidine as a Metal Chelator in Plants that Accumulate Nickel. Nature 379 (6566), 635–638. doi:10.1038/379635a0
Krishnamurthy, N., and Gupta, C. K. (2016). Extractive Metallurgy of Rare Earths. 2nd Edition. Boca Raton: CRC Press.
Lai, Y., Wang, Q., Yan, W., Yang, L., and Huang, B. (2005). Preliminary Study of the Enrichment and Fractionation of REEs in a Newly Discovered REE Hyperaccumulator Pronephrium Simplex by SEC-ICP-MS and MALDI-TOF/ESI-MS. J. Anal. Spectrom. 20 (8), 751–753. doi:10.1039/B501766A
Langkau, S., and Erdmann, M. (2020). Environmental Impacts of the Future Supply of Rare Earths for Magnet Applications. J. Ind. Ecol., 1–17. doi:10.1111/jiec.13090
Lasat, M. M., Baker, A. J. M., and Kochian, L. V. (1998). Altered Zn Compartmentation in the Root Symplasm and Stimulated Zn Absorption into the Leaf as Mechanisms Involved in Zn Hyperaccumulation in Thlaspi Caerulescens. Plant Physiol. 118 (3), 875–883. doi:10.1104/pp.118.3.875
Lebeau, T. (2011). “Bioaugmentation for In Situ Soil Remediation: How to Ensure the success of Such a Process,” in Bioaugmentation, Biostimulation and Biocontrol (Berlin, Heidelberg: Springer), 129–186. doi:10.1007/978-3-642-19769-7_7
Lebeau, T., Braud, A., and Jézéquel, K. (2008). Performance of Bioaugmentation-Assisted Phytoextraction Applied to Metal Contaminated Soils: a Review. Environ. Pollut. 153 (3), 497–522. doi:10.1016/j.envpol.2007.09.015
Lee, W. J., and Kim, K. Y. (1997). Taxonomic Study of Dictyota Dichotoma (Phaeophyta) Based on Morphological Variations of Four Local Populations from Korea. Algae 12 (2), 83–91.
Li, J., Hong, M., Yin, X., and Liu, J. (2010). Effects of the Accumulation of the Rare Earth Elements on Soil Macrofauna Community. J. Rare Earths 28 (6), 957–964. doi:10.1016/S1002-0721(09)60233-7
Li, X., Chen, Z., Chen, Z., and Zhang, Y. (2013). A Human Health Risk Assessment of Rare Earth Elements in Soil and Vegetables from a Mining Area in Fujian Province, Southeast China. Chemosphere 93, 1240–1246. doi:10.1016/j.chemosphere.2013.06.085
Liang, T., Li, K., and Wang, L. (2014). State of Rare Earth Elements in Different Environmental Components in Mining Areas of China. Environ. Monit. Assess. 186 (3), 1499–1513. doi:10.1007/s10661-013-3469-8
Lim, S., and Franklin, S. J. (2006). Engineered Lanthanide-Binding Metallohomeodomains: Designing Folded Chimeras by Modular Turn Substitution. Protein Sci. 15 (9), 2159–2165. doi:10.1110/ps.062365506
Lin, R., Howard, B. H., Roth, E. A., Bank, T. L., Granite, E. J., and Soong, Y. (2017). Enrichment of Rare Earth Elements from Coal and Coal By-Products by Physical Separations. Fuel 200, 506–520. doi:10.1016/j.fuel.2017.03.096
Liu, C., Yuan, M., Liu, W.-S., Guo, M.-N., Huot, H., Tang, Y.-T., et al. (2018). “Element Case Studies: Rare Earth Elements,” in Agromining: Farming for Metals (Cham: Springer), 297–308. doi:10.1007/978-3-319-61899-9_19
Liu, M., and Hasenstein, K. H. (2005). La3+ Uptake and its Effect on the Cytoskeleton in Root Protoplasts of Zea mays L. Planta 220 (5), 658–666. doi:10.1007/s00425-004-1379-2
Liu, Z., Guo, B. S., Zhu, W. M., Xiong, B. K., Ji, Y. J., Liu, Z., et al. (1988). “Rare Earth Elements in Soil,” in Rare Earth Elements in Agriculture (in Chinese) (Beijing: China Agricultural Science and Technology Press), p. 22–44.
Loges, A., Wagner, T., Barth, M., Bau, M., Göb, S., and Markl, G. (2012). Negative Ce Anomalies in Mn Oxides: The Role of Ce4+ Mobility during Water-mineral Interaction. Geochimica et Cosmochimica Acta 86, 296–317. doi:10.1016/j.gca.2012.03.017
Long, K. R., Van Gosen, B. S., Foley, N. K., and Cordier, D. (2012). “The Principal Rare Earth Elements Deposits of the United States: A Summary of Domestic Deposits and a Global Perspective,” in Non-Renewable Resource Issues: Geoscientific and Societal Challenges. Editors R. Sinding-Larsen, and F-W. Wellmer (Netherlands: Springer), 131–155. doi:10.1007/978-90-481-8679-2_7
Lucy, M., Reed, E., and R. Glick, B. (2004). Applications of Free Living Plant Growth-Promoting Rhizobacteria. Antonie van leeuwenhoek 86 (1), 1–25. doi:10.1023/b:anto.0000024903.10757.6e
Ma, Y., Rajkumar, M., Luo, Y., and Freitas, H. (2011). Inoculation of Endophytic Bacteria on Host and Non-host Plants-Effects on Plant Growth and Ni Uptake. J. Hazard. Mater. 195, 230–237. doi:10.1016/j.jhazmat.2011.08.034
Malhotra, N., Hsu, H.-S., Liang, S.-T., Roldan, M. J. M., Lee, J.-S., Ger, T.-R., et al. (2020). An Updated Review of Toxicity Effect of the Rare Earth Elements (REEs) on Aquatic Organisms. Animals 10 (9), 1663. doi:10.3390/ani10091663
Marscheider-Weidemann, F., Langkau, S., Hummen, T., Erdmann, L., Tercero Espinoza, L., Angerer, G., et al. (2016). Summary | Raw Materials for Emerging Technologies 2016. Berlin: DERA Rohstoffi nformationen, 13.
Marschner, H. (2012). Marschner‘s mineral Nutrition of Higher Plants, 3e éd. London, Waltham: Petra Marschner, 672.
Masmoudi-Soussi, A., Hammas-Nasri, I., Horchani-Naifer, K., and Férid, M. (2019). Study of Rare Earths Leaching after Hydrothermal Conversion of Phosphogypsum. Chem. Africa 2 (3), 415–422. doi:10.1007/s42250-019-00048-z
Massari, S., and Ruberti, M. (2013). Rare Earth Elements as Critical Raw Materials: Focus on International Markets and Future Strategies. Resour. Pol. 38 (1), 36–43. doi:10.1016/j.resourpol.2012.07.001
McBride, M. B. (1989). “Reactions Controlling Heavy Metal Solubility in Soils,” in Advances in Soil Science (New York, NY: Springer), 1–56. doi:10.1007/978-1-4613-8847-0_1
Mehmood, M. (2018). Rare Earth Elements-A Review. J. Ecol. Nat. Resour. 2, 1–6. doi:10.23880/jenr-16000128
Migaszewski, Z. M., and Gałuszka, A. (2015). The Characteristics, Occurrence, and Geochemical Behavior of Rare Earth Elements in the Environment: a Review. Crit. Rev. Environ. Sci. Technol. 45 (5), 429–471. doi:10.1080/10643389.2013.866622
Nakanishi, T. M., Takahashi, J., and Yagi, H. (1997). Rare Earth Element, Al, and Sc Partition between Soil and Caatinger wood Grown in north-east Brazil by Instrumental Neutron Activation Analysis. Biol. Trace Elem. Res. 60 (3), 163–174. doi:10.1007/BF02784437
Ohnuki, T., Jiang, M., Sakamoto, F., Kozai, N., Yamasaki, S., Yu, Q., et al. (2015). Sorption of Trivalent Cerium by a Mixture of Microbial Cells and Manganese Oxides: Effect of Microbial Cells on the Oxidation of Trivalent Cerium. Geochimica et Cosmochimica Acta 163, 1–13. doi:10.1016/j.gca.2015.04.043
Ohta, A., and Kawabe, I. (2001). REE(III) Adsorption onto Mn Dioxide (δ-MnO2) and Fe Oxyhydroxide: Ce(III) Oxidation by δ-MnO2. Geochimica et Cosmochimica Acta 65, 695–703. doi:10.1016/S0016-7037(00)00578-0
Ozaki, T., Enomoto, S., Minai, Y., Ambe, S., and Makide, Y. (2000). A Survey of Trace Elements in Pteridophytes. Bter 74 (3), 259–274. doi:10.1385/bter:74:3:259
Pagano, G., Guida, M., Tommasi, F., and Oral, R. (2015). Health Effects and Toxicity Mechanisms of Rare Earth Elements-Knowledge Gaps and Research Prospects. Ecotoxicology Environ. Saf. 115, 40–48. doi:10.1016/j.ecoenv.2015.01.030
Pang, X., Li, D., and Peng, A. (2002). Application of Rare-Earth Elements in the Agriculture of China and its Environmental Behavior in Soil. Environ. Sci. Pollut. Res. 9 (2), 143–148. doi:10.1007/BF02987462
Patten, C. L., and Glick, B. R. (2002). Role of Pseudomonas Putida Indoleacetic Acid in Development of the Host Plant Root System. Aem 68 (8), 3795–3801. doi:10.1128/AEM.68.8.3795-3801.2002
Peelman, S., Sun, Z. H. I., Sietsma, J., and Yang, Y. (2016). “Leaching of Rare Earth Elements,” in Rare Earths Industry (Elsevier Inc. Process, 149), 78–83.
Ping, L., and Boland, W. (2004). Signals from the Underground: Bacterial Volatiles Promote Growth in Arabidopsis. Trends Plant Science 9 (6), 263–266. doi:10.1016/j.tplants.2004.04.008
Pol, A., Barends, T. R. M., Dietl, A., Khadem, A. F., Eygensteyn, J., Jetten, M. S. M., et al. (2014). Rare Earth Metals Are Essential for Methanotrophic Life in Volcanic Mudpots. Environ. Microbiol. 16 (1), 255–264. doi:10.1111/1462-2920.12249
Qu, Y., Li, H., Wang, X., Tian, W., Shi, B., Yao, M., et al. (2019). Bioleaching of Major, Rare Earth, and Radioactive Elements from Red Mud by Using Indigenous Chemoheterotrophic Bacterium Acetobacter Sp. Minerals 9 (2), 67. doi:10.3390/min9020067
Rajkumar, M., Ae, N., Prasad, M. N. V., and Freitas, H. (2010). Potential of Siderophore-Producing Bacteria for Improving Heavy Metal Phytoextraction. Trends Biotechnology 28 (3), 142–149. doi:10.1016/j.tibtech.2009.12.002
Ramos, S. J., Dinali, G. S., Oliveira, C., Martins, G. C., Moreira, C. G., Siqueira, J. O., et al. (2016). Rare Earth Elements in the Soil Environment. Curr. Pollut. Rep 2 (1), 28–50. doi:10.1007/s40726-016-0026-4
Rasoulnia, P., and Mousavi, S. M. (2016). Maximization of Organic Acids Production by Aspergillus niger in a Bubble Column Bioreactor for V and Ni Recovery Enhancement from Power Plant Residual Ash in Spent-Medium Bioleaching Experiments. Bioresour. Tech. 216, 729–736. doi:10.1016/j.biortech.2016.05.114
Reddy, N., Maheswaran, J., Meehan, B., and Peverill, K. (2001). “The Application of Rare Earth Elements in Enhancement of Crop Production in Australia. Part 2,” in Proceedings of the 4th International Conference on Rare Earth Development and Application.
Rico, C. M., Morales, M. I., Barrios, A. C., McCreary, R., Hong, J., Lee, W.-Y., et al. (2013). Effect of Cerium Oxide Nanoparticles on the Quality of rice (Oryza Sativa L.) Grains. J. Agric. Food Chem. 61 (47), 11278–11285. doi:10.1021/jf404046v
Rim, K.-T. (2016). Effects of Rare Earth Elements on the Environment and Human Health: a Literature Review. Toxicol. Environ. Health Sci. 8 (3), 189–200. doi:10.1007/s13530-016-0276-y
Rim, K. T., Koo, K. H., and Park, J. S. (2013). Toxicological Evaluations of Rare Earths and Their Health Impacts to Workers: a Literature Review. Saf. Health work 4 (1), 12–26. doi:10.5491/SHAW.2013.4.1.12
Robinson, W. O. (1943). The Occurrence of Rare Earths in Plants and Soils. Soil Sci. 56 (1), 1–6. doi:10.1097/00010694-194307000-00001
Robinson, W. O., Whetstone, R., and Scribner, B. F. (1938). The Presence of Rare Earths in hickory Leaves. Science 87 (2264), 470. doi:10.1126/science.87.2264.470
Rodrigues, J., Houzelot, V., Ferrari, F., Echevarria, G., Laubie, B., Morel, J.-L., et al. (2016). Life Cycle Assessment of Agromining Chain Highlights Role of Erosion Control and Bioenergy. J. Clean. Prod. 139, 770–778. doi:10.1016/j.jclepro.2016.08.110
Rüttinger, L., and Feil, M. (2010). Sustainable Prevention of Resource Conflicts: New Risks from Raw Materials for the Future? Case Study and Scenarios for China and Rare Earths. Adelphi, Berlin: Adelphi. http://www.adelphi.de/filles/de/news/application/pdf/rohkon_report_3.4_china.pdf (Accessed September 27, 2011).
Salt, D. E., Blaylock, M., Kumar, N. P. B. A., Dushenkov, V., Ensley, B. D., Chet, I., et al. (1995). Phytoremediation: a Novel Strategy for the Removal of Toxic Metals from the Environment Using Plants. Nat. Biotechnol. 13 (5), 468–474. doi:10.1038/nbt0595-468
Sayler, G. S., and Ripp, S. (2000). Field Applications of Genetically Engineered Microorganisms for Bioremediation Processes. Curr. Opin. Biotechnol. 11 (3), 286–289. doi:10.1016/S0958-1669(00)00097-5
Schmidt, G. (2013). Description and Critical Environmental Evaluation of the REE Refining Plant LAMP Near Kuantan/Malaysia: Radiological and Non-radiological Environmental Consequences of the Plant's Operation and its Wastes. ÖkoInstitut eV Darmstadt. Kuantan/Malaysia: Prepared on behalf of NGO “Save Malaysia, Stop Lynas” (SMSL).
Schwabe, A., Meyer, U., Grün, M., Voigt, K. D., Flachowsky, G., and Dänicke, S. (2012). Effect of Rare Earth Elements (REE) Supplementation to Diets on the Carry-Over into Different Organs and Tissues of Fattening Bulls. Livestock Sci. 143 (1), 5–14. doi:10.1016/j.livsci.2011.08.010
Seaman, J. (2019). “Rare Earths and China,” in A Review of Changing Criticality in the New Economy. doi:10.4324/9781315649139
Sessitsch, A., Kuffner, M., Kidd, P., Vangronsveld, J., Wenzel, W. W., Fallmann, K., et al. (2013). The Role of Plant-Associated Bacteria in the Mobilization and Phytoextraction of Trace Elements in Contaminated Soils. Soil Biol. Biochem. 60, 182–194. doi:10.1016/j.soilbio.2013.01.012
Shan, X.-q., Lian, J., and Wen, B. (2002). Effect of Organic Acids on Adsorption and Desorption of Rare Earth Elements. Chemosphere 47 (7), 701–710. doi:10.1016/S0045-6535(02)00032-2
Shan, X., Wang, H., Zhang, S., Zhou, H., Zheng, Y., Yu, H., et al. (2003). Accumulation and Uptake of Light Rare Earth Elements in a Hyperaccumulator Dicropteris Dichotoma. Plant Sci. 165 (6), 1343–1353. doi:10.1016/S0168-9452(03)00361-3
Shannon, R. D. (1976). Revised Effective Ionic Radii and Systematic Studies of Interatomic Distances in Halides and Chalcogenides. Acta Cryst. Sect A. 32, 751–767. doi:10.1107/s0567739476001551
Shin, D., Kim, J., Kim, B.-s., Jeong, J., and Lee, J.-c. (2015). Use of Phosphate Solubilizing Bacteria to Leach Rare Earth Elements from Monazite-Bearing Ore. Minerals 5, 189–202. doi:10.3390/min5020189
Song, Y., Kirkwood, N., Maksimović, Č., Zheng, X., O'Connor, D., Jin, Y., et al. (2019). Nature Based Solutions for Contaminated Land Remediation and brownfield Redevelopment in Cities: A Review. Sci. Total Environ. 663, 568–579. doi:10.1016/j.scitotenv.2019.01.347
Soudek, P., Petrová, Š., Landa Lekuberria, J., Tomášková, I., and Vaněk, T. (2017). “Ecotoxicity of Rare Earth Elements,” in 15th International Conference on Environmental Science and Technology.
Stone, R. (2009). As China's Rare Earth R&D Becomes Ever More Rarefied, Others Tremble. Science 325, 1336–1337. doi:10.1126/science.325_1336
Suli, L. M., Ibrahim, W. H. W., Aziz, B. A., Deraman, M. R., and Ismail, N. A. (2017). A Review of Rare Earth mineral Processing Technology. Chem. Eng. Res. Bull. 19, 20–35. doi:10.3329/cerb.v19i0.33773
Taghavi, S., Garafola, C., Monchy, S. ½., Newman, L., Hoffman, A., Weyens, N., et al. (2009). Genome Survey and Characterization of Endophytic Bacteria Exhibiting a Beneficial Effect on Growth and Development of poplar Trees. Appl. Environ. Microbiol. 75 (3), 748–757. doi:10.1128/AEM.02239-08
Takahashi, Y., Shimizu, H., Usui, A., Kagi, H., and Nomura, M. (2000). Direct Observation of Tetravalent Cerium in Ferromanganese Nodules and Crusts by X-ray-absorption Near-Edge Structure (XANES). Geochimica et Cosmochimica Acta 64 (17), 2929–2935. doi:10.1016/S0016-7037(00)00403-8
Tanaka, M., Oki, T., Koyama, K., Narita, H., and Oishi, T. (2013). “Recycling of Rare Earths from Scrap,” in Handbook on the Physics and Chemistry of Rare Earths, 43 (Elsevier), 159–211. doi:10.1016/B978-0-444-59536-2.00002-7
Texier, A.-C., Andrès, Y., and Le Cloirec, P. (2000). Selective Biosorption of Lanthanide (La, Eu, Yb) Ions by an Immobilized Bacterial Biomass. Water Sci. Technol. 42 (5-6), 91–94. doi:10.2166/wst.2000.0500
Texier, A. C., Andrès, Y., Faur-Brasquet, C., and Le Cloirec, P. (2002). Fixed-bed Study for Lanthanide (La, Eu, Yb) Ions Removal from Aqueous Solutions by Immobilized Pseudomonas aeruginosa: Experimental Data and Modelization. Chemosphere 47 (3), 333–342. doi:10.1016/s0045-6535(01)00244-2
Thomas, P. J., Carpenter, D., Boutin, C., and Allison, J. E. (2014). Rare Earth Elements (REEs): Effects on Germination and Growth of Selected Crop and Native Plant Species. Chemosphere 96, 57–66. doi:10.1016/j.chemosphere.2013.07.020
Thomas, W. A. (2011). Accumulation of Rare Earths and Circulation of Cerium by Mockernut hickory Trees. Can. J. Bot. 53, 1159–1165. doi:10.1139/b75-139
Trifi, H., Ben Salem, I., Benzina, K., Fourati, A., Costa, M. C., Achouak, W., et al. (2017). Effectiveness of the Plant Growth-Promoting Rhizobacterium Pantoea Sp. BRM17 in Enhancing Brassica Napus Growth in Phosphogypsum-Amended Soil. Sidi Thabet, Tunisia: Pedosphere. doi:10.1016/S1002-0160(17)60454-5
Turra, C., and Bacchi, M. A. (2011). Evaluation on Rare Earth Elements of Brazilian Agricultural Supplies. J. Environ. Chem. Ecotoxicology 3 (4), 86–92. doi:10.5897/JECE.9000024
Tyler, G. (2004). Rare Earth Elements in Soil and Plant Systems - A Review. Plant Soil 267 (1-2), 191–206. doi:10.1007/s11104-005-4888-2
U.S. Geological Survey (2018). Mineral Commodity Summaries 2018. Reston, Virginia: U.S. Geological Survey, 132–133.
USGS (2014). United States Geological Survey, Mineral Commodity Summaries 2014. Reston, Virginia: U.S. Geological Survey., 196.
Van der Ent, A., Baker, A. J. M., Reeves, R. D., Chaney, R. L., Anderson, C. W. N., Meech, J. A., et al. (2015). Agromining: Farming for Metals in the Future?. Environ. Sci. Technol. 49, 4773–4780. doi:10.1021/es506031u
Van der Ent, A., Echevarria, G., Baker, A. J., and Morel, J. L. (2021). Agromining: Farming for metalsAgromining: Farming for Metals. Cham, Switzerland: Springer, 75–92. doi:10.1007/978-3-030-58904-2
Van Gosen, B. S., Verplanck, P. L., Long, K. R., Gambogi, J., and Seal, R. R. (2014). The Rare-Earth Elements: Vital to Modern Technologies and Lifestyles. Reston: US Geological Survey. doi:10.3133/fs20143078
Vogel, T. M. (1996). Bioaugmentation as a Soil Bioremediation Approach. Curr. Opin. Biotechnol. 7 (3), 311–316. doi:10.1016/S0958-1669(96)80036-X
Von Tucher, S., Goy, C., and Schmidhalter, U. (2001). “Effect of Lanthanum on Growth and Composition of mineral Nutrients of Phaseolus vulgaris L Var. Nanus and Zea mays L. Conv. Saccharata,” in Plant Nutrition (Dordrecht: Springer), 524–525. doi:10.1007/0-306-47624-X_254
Voncken, J. H. L. (2015). Physical and Chemical Properties of the Rare Earths. Delft: The Rare Earth Elements, 53–72. doi:10.1007/978-3-319-26809-5_3
Walters, A., and Lusty, P. (2011). Rare Earth Elements - Commodity Profile. November): British Geological Survey, 54.
Wang, Y. Q., Sun, J. X., Chen, H. M., and Guo, F. Q. (1997). Determination of the Contents and Distribution Characteristics of REE in Natural Plants by NAA. J. Radioanal. Nucl. Chem. 219 (1), 99–103. doi:10.1007/BF02040273
Wang, Z. G., Yu, X. Y., and Zhao, Z. H. (1989). The Geochemistry of Rare Earth Elements. Beijing:Science Press, p. 321.
Welch, S. A., Barker, W. W., and Banfield, J. F. (1999). Microbial Extracellular Polysaccharides and Plagioclase Dissolution. Geochimica et Cosmochimica Acta 63 (9), 1405–1419. doi:10.1016/S0016-7037(99)00031-9
Wilde, E. W., Berry, C. J., and Goli, M. B. (2002). Toxicity of Gadolinium to Some Aquatic Microbes (No. WSRC-MS-2000-00638). Savannah River Site (US), 420–427. doi:10.1007/s001280271
Wood, B. W., and Grauke, L. J. (2011). The Rare-Earth Metallome of Pecan and Other Carya. J. Amer. Soc. Hort. Sci. 136 (6), 389–398. doi:10.21273/jashs.136.6.389
Wu, J.-l., Wei, Z.-g., Zhao, H.-y., Li, H.-x., and Hu, F. (2009). The Role of Amino Acids in the Long-Distance Transport of La and Y in the Xylem Sap of Tomato. Biol. Trace Elem. Res. 129 (1-3), 239–250. doi:10.1007/s12011-008-8277-6
Wu, S., Wang, L., Zhao, L., Zhang, P., El-Shall, H., Moudgil, B., et al. (2018). Recovery of Rare Earth Elements from Phosphate Rock by Hydrometallurgical Processes - A Critical Review. Chem. Eng. J. 335, 774–800. doi:10.1016/j.cej.2017.10.143
Xiao, H. Q., Zhang, Z. Y., Li, F. L., and Chai, Z. F. (2003). Study on Contents and Distribution Characteristics of REE in Fern by NAA. Nucl. Technol. 26, 421–423.
Xiao, Y.-f., Chen, Y.-y., Feng, Z.-y., Huang, X.-w., Huang, L., Long, Z.-q., et al. (2015). Leaching Characteristics of Ion-Adsorption Type Rare Earths Ore with Magnesium Sulfate. Trans. nonferrous met. Soc. China 25 (11), 3784–3790. doi:10.1016/S1003-6326(15)64022-5
Xie, F., Zhang, T. A., Dreisinger, D., and Doyle, F. (2014). A Critical Review on Solvent Extraction of Rare Earths from Aqueous Solutions. Minerals Eng. 56, 10–28. doi:10.1016/j.mineng.2013.10.021
Xie, H., Pasternak, J. J., and Glick, B. R. (1996). Isolation and Characterization of Mutants of the Plant Growth-Promoting Rhizobacterium Pseudomonas Putida GR12-2 that Overproduce Indoleacetic Acid. Curr. Microbiol. 32 (2), 67–71. doi:10.1007/s002849900012
Xin, H. U., Xiao-Rong, W. A. N. G., and Chao, W. A. N. G. (2006). Bioaccumulation of Lanthanum and its Effect on Growth of maize Seedlings in a Red Loamy Soil. Pedosphere 16 (6), 799–805. doi:10.1016/S1002-0160(06)60116-1
Xu, Y., Sun, G.-D., Jin, J.-H., Liu, Y., Luo, M., Zhong, Z.-P., et al. (2014). Successful Bioremediation of an Aged and Heavily Contaminated Soil Using a Microbial/plant Combination Strategy. J. Hazard. Mater. 264, 430–438. doi:10.1016/j.jhazmat.2013.10.071
Xue, W. A. N. G., Yousheng, L. I. N., Dongwu, L. I. U., Hengjian, X. U., Tao, L. I. U., and Fengyun, Z. H. A. O. (2012). Cerium Toxicity, Uptake and Translocation in Arabidopsis thaliana Seedlings. J. Rare Earths 30 (6), 579–585. doi:10.1016/S1002-0721(12)60094-5
Xue, Y. (2009). Studies of the Hyperaccumulation Ability of Pronephrium Simplex and Pronephrium Triphyllum to Rare Earth Elements and Their Binding Peptides. BSc Thesis: Xiamen University, 99. doi:10.1039/B501766A
Ye-Tao, T. A. N. G., Teng-Hao-Bo, D. E. N. G., Qi-Hang, W. U., Shi-Zhong, W. A. N. G., Rong-Liang, Q. I. U., Ze-Bin, W. E. I., et al. (2012). Designing Cropping Systems for Metal-Contaminated Sites: a Review. Pedosphere 22 (4), 470–488. doi:10.1016/S1002-0160(12)60032-0
Yuan, D., Shan, X.-q., Huai, Q., Wen, B., and Zhu, X. (2001). Uptake and Distribution of Rare Earth Elements in rice Seeds Cultured in Fertilizer Solution of Rare Earth Elements. Chemosphere 43 (3), 327–337. doi:10.1016/s0045-6535(00)00142-9
Yuan, M., Guo, M.-N., Liu, W.-S., Liu, C., van der Ent, A., Morel, J. L., et al. (2017). The Accumulation and Fractionation of Rare Earth Elements in Hydroponically Grown Phytolacca Americana L. Plant Soil 421 (1), 67–82. doi:10.1007/s11104-017-3426-3
Yuan, M., Liu, C., Liu, W.-S., Guo, M.-N., Morel, J. L., Huot, H., et al. (2018). Accumulation and Fractionation of Rare Earth Elements (REEs) in the Naturally Grown Phytolacca Americana L. In Southern China. Int. J. Phytoremediation 20 (5), 415–423. doi:10.1080/15226514.2017.1365336
Zhang, C., Li, Q., Zhang, M., Zhang, N., and Li, M. (2013). Effects of Rare Earth Elements on Growth and Metabolism of Medicinal Plants. Acta Pharmaceutica Sinica B 3 (1), 20–24. doi:10.1016/j.apsb.2012.12.005
Zhang, J., and Edwards, C. (2013). Mineral Decomposition and Leaching Processes for Treating Rare Earth Ore Concentrates. Can. Metallurgical Q. 52 (3), 243–248. doi:10.1179/1879139513Y.0000000084
Zhang, J., Zhao, B., and Schreiner, B. (2016). Separation Hydrometallurgy of Rare Earth Elements. Switzerland: Springer International Publishing, 259.
Zhang, S. (2013). Problems and Countermeasures of Rare Earth Industry in China. Can. Soc. Sci. 9 (3), 9–14. doi:10.3968/j.css.1923669720130903.Z1013
Zhang, W., Rezaee, M., Bhagavatula, A., Li, Y., Groppo, J., and Honaker, R. (2015). A Review of the Occurrence and Promising Recovery Methods of Rare Earth Elements from Coal and Coal By-Products. Int. J. Coal Preparation Utilization 35 (6), 295–330. doi:10.1080/19392699.2015.1033097
Zhanheng, C. H. E. N. (2011). Global Rare Earth Resources and Scenarios of Future Rare Earth Industry. J. rare earths 29 (1), 1–6. doi:10.1016/S1002-0721(10)60401-2
Zhenhong, L., and Hong, W. (1996). Present Status and Future Prospect of Rare Earth Application in China [J]. Chin. Rare Earths 6.
Keywords: bioaugmentation, bioavailability, hyperaccumulator, phytomanagement, phytomining, rare earth elements, soil
Citation: Jalali J and Lebeau T (2021) The Role of Microorganisms in Mobilization and Phytoextraction of Rare Earth Elements: A Review. Front. Environ. Sci. 9:688430. doi: 10.3389/fenvs.2021.688430
Received: 31 March 2021; Accepted: 31 May 2021;
Published: 15 June 2021.
Edited by:
Michel Chalot, Université Bourgogne Franche-Comté, FranceReviewed by:
Agnieszka Klimkowicz-Pawlas, Institute of Soil Science and Plant Cultivation, PolandCopyright © 2021 Jalali and Lebeau. This is an open-access article distributed under the terms of the Creative Commons Attribution License (CC BY). The use, distribution or reproduction in other forums is permitted, provided the original author(s) and the copyright owner(s) are credited and that the original publication in this journal is cited, in accordance with accepted academic practice. No use, distribution or reproduction is permitted which does not comply with these terms.
*Correspondence: Thierry Lebeau, thierry.lebeau@univ-nantes.fr
Disclaimer: All claims expressed in this article are solely those of the authors and do not necessarily represent those of their affiliated organizations, or those of the publisher, the editors and the reviewers. Any product that may be evaluated in this article or claim that may be made by its manufacturer is not guaranteed or endorsed by the publisher.
Research integrity at Frontiers
Learn more about the work of our research integrity team to safeguard the quality of each article we publish.