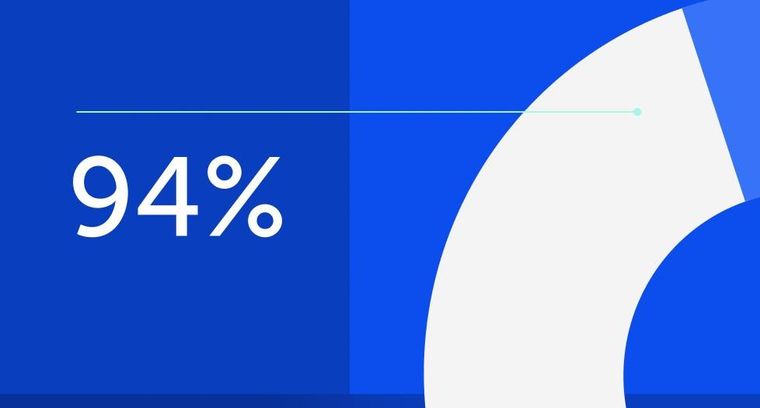
94% of researchers rate our articles as excellent or good
Learn more about the work of our research integrity team to safeguard the quality of each article we publish.
Find out more
ORIGINAL RESEARCH article
Front. Environ. Sci., 12 August 2021
Sec. Freshwater Science
Volume 9 - 2021 | https://doi.org/10.3389/fenvs.2021.671556
This article is part of the Research TopicFreshwater Ecosystems in Arid and Semiarid Zones Facing Multiple Stressors: Human Disturbances, Climate Change, and Dryland River ConservationView all 9 articles
In dryland rivers, flow intermittency means fish populations are often subjected to drought disturbance. The viability of these fish populations depends on the availability of waterhole refuges for individuals to survive drought (resistance) and the ability of surviving fish to repopulate the rivers by recruitment and dispersal once flow returns (resilience). In this study we combined remote-sensed mapping of the locations of waterholes that lasted through an extreme drought in the northern Murray Darling Basin, Australia, with an assessment of the impacts of in-stream barriers on limiting the opportunities for fish to move and repopulate after drought. We found that at the peak of this 2018–2020 drought, the worst on record for some rivers and the most spatially synchronous recorded across the region, waterholes were few and generally small – representing only 11% of the total river channel network. All the fish in the region that survived the drought were concentrated into this limited waterhole refuge habitat. Even small instream structures, such as minor weirs, caused large reductions in the opportunities for fish to move between river segments when there is flow. Almost all the 104 instream structures assessed reduced long-term fish movement opportunities, measured as days with discharge greater than calculated barrier drown out thresholds, by more than 70% and up to 100%, when compared to opportunities for movement if the barrier was not present. This large impact from small instream barriers is a consequence of flow intermittency and is likely to reduce fish population resilience and impact the capacity of fish populations to recover after drought. Combining information on the risks posed by limited refuge habitat availability during drought and from reduced movement opportunity following drought allowed us to identify river segments where these combined threats are the greatest risk to viability of local fish populations. Considering the spatial arrangements of these risks provides a means to systematically prioritize mitigation measures such as weir removal to improve fish movement opportunities and local management of key waterholes to increase drought resistance. The approach used here provides a guide for assessing and prioritizing the management of fish population viability risks from drought and fragmentation by barriers in any non-perennial river setting.
Dryland rivers, those where annual evaporation exceeds annual precipitation, drain more than 50% of the world’s land area and support more than 20% of its human population (Nanson et al., 2002). Fish are an important component of their ecosystems, but the harsh and variable conditions that characterize dryland rivers pose challenges to fish populations (Arthington et al., 2005; Balcombe and Arthington, 2009; Vardakas et al., 2017; Rubalcaba et al., 2020). The relatively low fish species richness of dryland rivers (Romaní et al., 2017) is due to their extreme hydrological variability (see Puckridge et al., 1998), which drives considerable variability in their abiotic environments and the intensity of biotic interactions such as competition, predation and disease (Magoulick, 2000). Subsequently, only habitat generalist species with broad environmental tolerances are able to persist in these rivers (Horwitz, 1978).
Paramount amongst these harsh conditions, fish populations in many dryland rivers are subject to frequent hydrological drought disturbances. Flow stops, and most of the river channels dry, with remaining water restricted to deeper, in-channel features, commonly termed waterholes (Knighton and Nanson, 2000). During drought events, dryland river fish populations resist biophysical disturbance by utilizing waterholes as drought refugia (Sedell et al., 1990; Sheldon et al., 2010), as nearly all lack traits to allow aestivation or other means of survival without water (Romaní et al., 2017). After drought breaks with the return of flow, fish dispersal, reproduction and recruitment allow populations to recolonize denuded river reaches, making their regional populations resilient. The high prevalence of drought disturbances in dryland rivers means that fish populations frequently cycle through periods of resistance conferred by refugia and resilience conferred by movement, dispersal, reproduction and recruitment (Balcombe, 2005; Balcombe and Arthington, 2009; Arthington and Balcombe, 2011; Kerezsy et al., 2011; Kerezsy et al., 2013; Marshall et al., 2016). This cycling has been referred to as “boom and bust ecology” and is characteristic of dryland river ecosystems (Bunn et al., 2006; Sternberg et al., 2011). Both resistance and resilience are prerequisite for continuing fish population viability in these systems (Magoulick and Kobza, 2003; Crook et al., 2010).
The longer a drought continues, the fewer waterholes persist, as first the shallowest, and then progressively deeper waterholes dry (Hamilton et al., 2005). Hence, regional fish population resistance diminishes over time as waterhole refugia fail and local populations are progressively extirpated. Theoretically, the fewer the refuge waterholes available to support regional populations of fish during drought, the greater is the risk of regional extinctions (Bond et al., 2015). Under such circumstances, fish population resilience may rely on longer distance dispersal of individual fish from remote refugia (Kerezsy et al., 2013).
When drought-breaking flow events occur, dryland river fishes typically respond rapidly to move out of drought refugia (Kerezsy et al., 2013; Marshall et al., 2016). However, inter- and intra-species movement behaviour is highly variable. For example, studies from Australian dryland rivers have found that short lived, “extreme colonizing species” can move many hundreds of kilometers into previously dry river reaches, whereas longer lived “conservative colonizing species” tend to move shorter distances, stay within or closer to drought refuges, and often display philopatry to the waterhole where they resisted a previous drought (Kerezsy et al., 2013; Marshall et al., 2016). Within species there is considerable individual variability in response to flow, with many individuals remaining within a refuge waterhole (i.e., “partial migation,” sensuChan, 2001), random choice of upstream or downstream initial movement direction and variable philopatry over the initial or subsequent flow events (Marshall et al., 2016). Movement of individual fish out of refugia provides both adaptive advantages, such as access to under-utilised food and other resources and spawning locations (Labbe and Fausch, 2000), but also confers considrable risks, as movement itself uses energy and exposes fish to elevated predation (Brodersen et al., 2008), and stranding away from refugia as flow recedes represents almost certain death in systems with intermittent flow (Albanese et al., 2004; Vardakas et al., 2017). Evidence suggests that movement behaviour of dryland river fishes represents evolved adaptations to balance these risks and advantages, as introduced, invasive species and hybrid individuals adopt maladaptive behaviours in comparison to native species (Pärssinen et al., 2020). Variability in intraspecific behaviour, particularly philopatry and partial migration, are characteristic of this adaptation and are likely associated with the highly variable and unpredictable hydrology of dryland rivers, whereby different behaviours are advantagous at different times (Chan, 2001; Marshall et al., 2016). Superimposed on this is the role of individual experience and spatial memory, as fish that have experienced past drought and flow cycles behave in ways that increase survival more than do novice individuals (Chapman and Kramer, 1991).
Support for the critical importance of movement and dispersal for maintaining viable dryland river fish populations comes from several lines of evidence. Firstly, on occasion all fish are extirpated from dryland river tributaries through drying, and studies have found recolonisation occurs, facilitated by hydrological connection over varying time scales (Kerezsy et al., 2013; Gido et al., 2015). Secondly, loss of connectivity has been attributed as a cause of dramatic declines in the range and abundance of dryland river fish species (Gido et al., 2015; Perkin et al., 2015). For example, the silver perch (Bidyanus bidyanus (Mitchell, 1838), Terapontidae) once the predominant large-bodied species in the northern dryland rivers of the Australian Murray Darling Basin (Scott, 2005), is now critically endangered and functionally extinct in these rivers, largely due to the limitation of dispersal by dams and weirs (Threatened Species Scientific Committee, 2013; Romaní et al., 2017). Finally, the population genetics of dryland river fishes retain traces of local bottlenecks tempered by gene flow, further suggesting that dispersal is of paramount importance (Huey et al., 2011). Despite the importance of movement, in-channel structures fragment many dryland rivers by disrupting hydrological connectivity and limiting fish movement (Gido et al., 2015), as is the case in most rivers (Grill et al., 2019). Barriers restrict long distance dispersal, with the impact greater for movement in upstream rather than downstream directions and varying with species and fish size (Stuart and Sharpe, 2020; Hermoso et al., 2021). Fragmentation of dryland river fish populations by instream barriers that limit dispersal and recolonisation greatly increases the probability of extinctions, with the risk increasing with the degree of fragmentation as populations become smaller (Smith, 1997; Fagan, 2002; Fagan et al., 2002; Liermann et al., 2012; Gido et al., 2015).
Metapopulation theory (Levins, 1969) provides a framework for assessing the consequences of fragmentation for dryland river fish populations (Gido et al., 2015). Metapopulations are comprised of the network of connected local populations that in dryland rivers can be readily identified as fish in spatially explicit waterholes. But these are unable to exchange migrants by dispersal except when they are connected via river channels by discrete flow events (Marshall et al., 2016). Over time, some local populations go extinct due to drying or stochastic water quality failures, but the movement of individuals during periods of connectivity is sufficient to recolonise these extirpated waterholes, maintaining collective metapopulation viability (Huey et al., 2011; Bond et al., 2015). However, metapopulations can survive only if the recolonisation rate of waterholes is at least equal to their extinction rate, suggesting that metapopulation scale extinctions can occur even when some suitable habitat remains (Datry et al., 2017). If extinction rate increases, for example due to increasing drought severity or waterhole habitat degradation, or if recolonisation rate reduces as a consequence of barriers to dispersal or flow regime change, metapopulations of fish may be driven extinct (Datry et al., 2017). Fragmentation that reduces recolonisation of local populations is predicted to lower the genetic diversity of the metapopulation (e.g., Cole et al., 2016) in proportion to the extent that barriers limit the geographic range of recolonisation, with minimum viable fragment sizes varying between fish species based on their dispersal capabilities (Gido et al., 2015; Murphy et al., 2015; Tonkin et al., 2018). Adverse effects on fish populations are more likely to occur in small fragments, isolated with impermeable barriers, in rivers with frequent disturbance events (Gido et al., 2015), suggesting dryland river fish may be particularly vulnerable.
Here we consider how the processes of habitat loss from drought and river fragmentation by barriers pose risks to the viability of fish populations in an Australian dryland river setting.
The dryland rivers of the northern Murray-Darling Basin (MDB), (Queensland, Australia), were subject to a severe hydrological drought from ca.2018-early 2020 that was one of the longest periods, (in some rivers the longest), without river flow on record. Record low rainfall, high rates of evaporative water loss associated with unprecedented extreme temperatures (World Meteorological Organization, 2020) and the prolonged lack of inflow, combined to limit the availability of waterholes as drought refugia. Furthermore, the quality of remaining refugia was compromised by hypoxic to anoxic conditions, exacerbating as they shallowed with increasing drought duration (Queensland Government, unpublished data), further threatening fish population resistance (Hopper et al., 2020), and causing widely publicized mass fish kills (Vertessy et al., 2019). From mid-January 2020 flow returned to these rivers following widespread rainfall, ending the long hydrological drought. However, before the onset of sustained flow, many locations experienced small “blackwater event” inflows (sensu King et al., 2012) that caused additional multi-day anoxia in persisting waterholes (Queensland Government, unpublished data). In combination, the limited number of waterholes, their poor water quality during the drought, and the impact of the first flush blackwater event suggest that few fish may have resisted this drought in some rivers of the northern MDB, and it is likely to have caused local to regional scale fish extinctions. Under such circumstances, movement of surviving fish and their progeny is critical for the recolonisation of extirpated habitat patches, yet there are over 100 instream barriers in these rivers that potentially limit this process.
Here we aim to identify both habitat loss from drought and river fragmentation by barriers in the northern MDB region. We then aim to use this information to assess the risk that these combined threats pose to the viability of the region’s fish populations. To address these aims, we combined mapping of the locations and extents of persistent waterholes at the time of extreme drought, with hydrological evaluations of barrier effects on opportunities for fish movement to facilitate recolonisation. This indicates river segments, defined by the locations of the barriers, where local populations of fishes are at greatest risk from the combined influences of drought on population resistance, and barrier effects on their drought resilience.
Given that future climate projections forecast increased prevalence and duration of extreme droughts for dryland regions, including those in Australia (Wang, 2005; Kirono et al., 2020), a better understanding of threats to resistance and resilience will greatly enhance capacity for sustainable management of fish populations in dryland rivers. The spatial distribution of this risk provides an important input into identifying risk mitigation priorities such as improvements to fish passage past barriers, waterhole habitat restoration and flow event management.
This assessment was conducted in the headwaters of Australia’s largest drainage basin, the Murray-Darling Basin (Fielder et al., 2011). The Queensland portions of all Murray Darling Basin rivers plus the Lower Balonne system in New South Wales downstream to its junctions with the Barwon River were included in the study (Figure 1). Henceforth, for convenience, the region is termed the Queensland Murray Darling Basin (QMDB).
FIGURE 1. Map of the study area showing the river network (blue lines), catchment boundaries (light grey lines), the border between the Australian states of Queensland (QLD) and New South Wales (NSW – dark grey line), with numbered red circles the locations and identities (as listed in Supplementary Table S2) of instream barriers, and black triangles representing the locations of stream gauging stations associated with instream barriers. Insert map shows the location of the study area within Australia.
The QMDB drains an area of approximately 260,000 km2 (Newham et al., 2018), and includes catchments of the Border Rivers, Moonie, Condamine–Balonne, Warrego and Paroo Rivers, which flow south into the Darling River system.
With the exception of a small temperate climate zone along the Great Dividing Range at the south-east margin, the area is subtropical and dry (Biggs et al., 2013). Annual rainfall declines, and climatic variability and evaporation increase along a gradient from east to west (Marshall et al., 2006; Sternberg et al., 2008; Fielder et al., 2011). The average annual rainfall at Toowoomba in the east of the region is 952 mm per year, while that of Eulo, on the Paroo River in the west, is 331 mm (Commonwealth of Australia, Bureau of Meteorology, 2021). The topography is generally flat, with low river channel slope which results in fine sediment accumulation and high turbidity (averaging more than 500 NTU), increasing with distance from the headwaters (Moffatt and Voller, 2002; Fielder et al., 2011; Marshall et al., 2019), and extensive floodplains and wetland complexes in some areas (Kingsford, 1999; Marshall et al., 2019).
Most rivers in the region are rainfall-runoff, as opposed to groundwater-fed, and, as rainfall tends to occur as sporadic large-scale events, their hydrology is typified by frequent periods without flow punctuated by unpredictable but well-defined flow events. For example, the Moonie River flows only 35 per cent of the time and usually exists as a series of disconnected waterholes (Sternberg et al., 2008). The duration and frequency of no-flow periods increases from east to west.
The fish fauna of the study region is relatively uniform throughout the dryland parts of the catchments, being dominated in terms of biomass and abundance by three species; golden perch [Macquaria ambigua (Richardson 1845), Percichthyidae], bony bream [Nematalosa erebi (Günther, 1868), Clupeidae] and the invasive, alien common carp [Cyprinus carpio (Linnaeus, 1758), Cyprinidae] (Prior et al., 2005; Negus et al., 2015a; Negus et al., 2019). An additional ten native species are also widespread but sporadic in occurrence throughout the region: Murray cod [Maccullochella peelii (Mitchell, 1838), Percichthyidae], silver perch [Bidyanus bidyanus (Mitchell, 1838), Terapontidae], spangled perch [Leiopotherapon unicolor (Günther, 1859), Terapontidae], tandan [Tandanus tandanus (Mitchell, 1838), Plotosidae], Hyrtl’s catfish (Neosilurus hyrtlii Steindachner, 1867; Plotosidae), Australian smelt [Retropinna semoni (Weber, 1895), Retropinnidae], Murray River rainbowfish [Melanotaenia fluviatilis (Castelnau, 1878), Melanotaeniidae], unspecked hardyhead [Craterocephalus fluvis (Günther, 1867), Atherinidae], olive perchlet (Ambassis agassizii Steindachner, 1867; Ambassidae), western carp gudgeon [Hypseleotris klunzingeri (Ogilby, 1898), Eleotridae]; as are two additional alien species: goldfish [Carassius auratus (Linnaeus, 1758), Cyprinidae] and eastern gambusia [Gambusia holbrooki (Girard, 1859); Poeciliidae]. The assemblage composition of fishes in refuge waterholes during periods without flow comprises of the three dominant species plus nested subsets of the additional regional assemblage (Balcombe et al., 2006). Within the study area there are also several other fish species that are either very rare or spatially restricted and which are not typical elements of dryland river refuge waterhole assemblages: Rendahl’s catfish [Porochilus rendahli (Whitley, 1928), Plotosidae] known from several smaller tributary systems of the Condamine-Balonne System, dwarf flathead gudgeon [Philypnodon macrostomus (Hoese and Reader, 2006); Eleotridae] known from few specimens collected in the mid Condamine River between Dalby and Surat, and three species that are restricted to localized, headwater regions: southern purple spotted gudgeon [Mogurnda adspersa (Castelnau, 1878), Eleotridae], mountain galaxias [Galaxias olidus (Günther, 1866); Galaxiidae] and river blackfish [Gadopsis marmoratus (Richardson, 1848); Percichthyidae], (Balcombe et al., 2006; Negus et al., 2015b; Marshall et al., 2019).
The severity of the 2018–2020 drought was compared to previous droughts in the region by determining drought durations as consecutive days without flow across the stream gauges of the QMDB (Queensland Govrnment, 2020; Water NSW, 2020). Droughts at each gauge were ranked from longest to shortest to determine the rank of the recent drought.
Spatial synchrony of drought across the catchments in the study area was also considered based on these gauge records. Previous studies have identified that nearly all waterholes in the region are less than 3 m deep when flow ceases and they are full, and that they lose water, mainly through evaporative loss, at a rate of 1 m per 170 days (Lobegeiger, 2010; DSITI, 2015). Based upon this, Queensland Government have adopted 180 days as a drought duration threshold beyond which broad-scale waterhole persistence and quality are at risk, and therefore local fish populations are threatened by drought. Recently, risk at stream gauges throughout the QMDB has been assessed using this threshold and summarized at the Basin scale to inform emergency fish management priorities (MDBA, 2019). We applied this threshold to the records of all QMDB gauges to develop time-series of the proportion of stream gauges in each river catchment that were at risk each day between 1973 and 2020 (chosen to provide representation of gauges across all rivers). We adopted a definition of catchment drought as when 50% or more of gauges in a catchment simultaneously exceeded the 180 days no flow threshold. This was applied to the Condamine Balonne system, divided into sections with recognized differences in flow regime and water management (DES, 2018b); (the upper Condamine River, mid-Condamine River, Maranoa River and Lower Balonne system); and the Moonie, Warrego and Paroo Rivers. For the Border Rivers, 25% of gauges was adopted to define catchment drought because flow regimes of some parts of this system are much less intermittent (DES, 2018a). If all these regions were in simultaneous drought there would be a count of eight as the highest possible simultaneous drought score. This identified patterns of catchment-scale drought synchrony across the QMDB and permitted comparison of the 2018–2020 drought with previous regional droughts within the broader stream gauge records.
Dates for peak drought were identified as the last day before flow events broke the drought based on stream gauge records (Queensland Govrnment, 2020; Water NSW, 2020). Because of the large spatial area of the QMDB and asynchrony of rainfall across it, a conservative date of January 15, 2020 was selected when none of the gauges in the area had yet experienced any post-drought flow. However, in the Condamine Balonne catchment there was a major release of water from Beardmore Dam into the lower Balonne distributary system in May 2019 (DNRME, 2019), which in effect artificially broke the drought in the Lower Balonne rivers.
Sentinel-2 satellite images from the European Space Agency’s Copernicus program were sourced to cover the area on dates as close to and before January 15, 2020 as possible, resulting in 50 images collected between 1–13 January 2020. An additional set of seven images from 13–16 April 2019 were analyzed for the Lower Balonne region. The 2015 water index (2015WI), with clouds removed using its cloud and cloud-shadow mask (Fisher et al., 2016), was used to identify water on the landscape at 10 m resolution for each of these images. The data were intersected with field validation photographic point data of waterhole presence/absence collected by Queensland Government at this time (Figure 2) to calibrate the water index thresholds for the QMDB. The water classification was clipped to the Australian Geofabric Hydrological Network Surface Network layer (BoM, 2014), with a buffer of 100 m. Data were stitched together for each catchment. Individual waterholes were defined as polygons consisting of either individual, isolated water-pixels, or clusters of connected pixels, with an area attribute for each polygon.
FIGURE 2. The presence or absence of water in rivers of the QMDB was used to calibrate the accurate identification of water from satellite images (A). As an example, the two site photos here represent sites 70 (B) and 71 (C) on the calibrated remote sensed water data.
For the Lower Balonne region, comparison was made of waterholes present in the 2019 and 2020 datasets with the expectation of more water in 2020 following the Beardmore Dam release (DNRME, 2019). This was largely substantiated, but there were some areas that were wet in 2019 but dry in 2020, which was not consistent with the 2019 data representing peak drought conditions. Thus, for the Lower Balonne region waterholes were represented as locations that were wet in both 2019 and 2020 datasets.
To represent the proportion of the river channel network that was wet, the length of waterholes within river catchments were expressed as a percentage of the catchment total channel length derived from the Australian Geofabric Hydrological Network Surface Network layer (BoM, 2014).
The locations, heights and drown out thresholds for all known instream barriers in the QMDB Rivers (Supplementary Table S2) were collated from Queensland Government records (Kerr et al., 2018) and from previous use in modelling (DSITIA, 2013; DES, 2018b). In this context the drown out threshold of a barrier is defined as the river discharge at which the downstream water level rises to a height where it begins to affect flow over a barrier, which is the discharge at which fish have opportunity to move upstream past the barrier (Koehn and Crook, 2013). Many of the drown out values for these barriers are estimates that have not been validated with specific field assessments, but those that have been validated (as per Keller et al., 2012) generally indicate estimates are reasonable at approximating these characteristics (Kerr et al., 2018).
Each barrier was next associated with the most relevant active QMDB stream gauge (Queensland Govrnment, 2020; Water NSW, 2020) to enable hydrological assessment of fish movement opportunities for each barrier. Five barriers on minor tributary streams were excluded from subsequent analysis because there were no stream gauges representing their hydrology, leaving 104 barriers included in analyses.
Empirical information on the movement behaviour of QMDB fish in relation to discharge (Marshall et al., 2016; DES, 2021) was used to determine 0.058 m3 s−1 (5 ML day−1) as an approximation of the minimum discharge threshold to instigate fish movement out of isolated refuge waterholes when flow commences following no-flow periods, in the absence of artificial barriers. For the gauge associated with each barrier and using the entire daily gauge records, calculations were made to identify firstly, the days when discharge exceeded 0.058 m3 s−1 and secondly, the days when it exceeded the barrier drown out threshold. The sum of these represented the number of days in the gauge record where there was opportunity respectively for fish passage without and with the barrier in place. Because the various gauges had different periods of flow record, these opportunities for fish movement were expressed as the percentage of total days in each gauge record to standardize the data between gauges. We also calculated the duration of maximum spells between fish movement opportunities for each barrier based on associated stream gauge records using the same with and without barrier flow thresholds.
Loss of long-term movement opportunity for fish due to the presence of each barrier was calculated as the percentage reduction in opportunities with the barrier present relative to without, termed here barrier risk. Risk categories were defined based on the magnitude of loss of movement opportunity as extreme risk (100% loss), high risk (70–100% loss), medium risk (50–70% loss), low risk (0–50% loss). Each barrier was assigned to a risk category on this basis as a representation of risk to post drought resilience.
To better understand resilience to the 2018–2020 QMDB drought, the gauge records (Queensland Govrnment, 2020; Water NSW, 2020) were also analyzed to determine if drought breaking flows in early 2020 were of sufficient magnitude to provide fish movement opportunities by drowning out each of the barriers.
Barrier locations were used to divide the QMDB river network into river segments using the major rivers data from Australian Geofabric Hydrological Network Surface Network layer (BoM, 2014), with a buffer of 100 m. This was achieved by using the cutting tool in ArcGIS version 10.7.1 (ESRI) applied perpendicular to the orientation of the river at the walls of downstream and upstream defining barriers for each segment. For conventional linear river segments this was straightforward resulting in segments with one barrier at the downstream extent which defined the name and fish passage opportunities for the segment, and one barrier at the upstream extent defining the upstream boundary. However, some segments of the QMDB river network are highly anastomosed with multiple anabranches and flood channels leaving and re-joining the main channels which can result in more than one downstream barrier defining the segment. Some segments also had barriers on more than one upstream tributary defining the upstream segment boundaries. These complexities resulted in 96 river segments defined by the 104 barriers. In cases with multiple downstream defining barriers, segment fish passage opportunities were defined by the barrier that drowned out more/most frequently.
The waterhole data were intersected with the river segments using ArcGIS and spatial statistics summarized from the resulting attribute database (dbf) files via pivot tables in Microsoft Excel as the count and total area of waterholes present per segment at the peak of the 2018–2020 drought.
Risk to drought resistance of fish populations per river segment was determined based on the number and area of waterholes present in the segment at peak drought, termed here waterhole risk. Risk categories were defined as extreme risk (no waterholes present), high risk (fewer than 10 waterholes or less than 1 ha of waterhole habitat present), medium risk (fewer than 50 waterholes or less than 5 ha of waterhole habitat present) and low risk (more than 50 waterholes or greater than 5 ha of waterhole habitat present). Each river segment was assigned to a risk category on this basis, as a representation of drought resistance risk.
Drought risk to local fish populations is the combined risks of resistance to drought in refuge waterholes and resilience following drought. To represent this, both the barrier risk and the waterhole risk for segments were given a score (1.5 for extreme risk, 1 for high risk, 0.5 for medium risk and 0 for low risk) and then these were summed to give an indication of overall drought risk for each segment. Scoring was consistent for the two contributing risks, in effect conferring them equal weighting in overall risk.
Spatial, patch-based graphs (Eros et al., 2012) were developed to represent both peak drought waterhole availability and fish movement opportunity per segment and so represent combined resistance and resilience risks to local fish populations. This was done for both long-term fish movement opportunities and for opportunities following the 2018–2020 drought.
Gauge records confirm that the 2018–2020 drought was both severe and widespread across the QMDB. The drought duration averaged 354 days without any flow (range 43–1,006 days) across the stream gauges of the QMDB. Compared to average drought durations, this drought was between 4.7 and 8.1 times longer, depending on river, but at some individual gauges it was up to 25 times longer than average (Table 1, Supplementary Table S1). At many stream gauges the 2018–2020 drought was the longest drought on record, and it was amongst the longest three droughts at most stream gauges. This was the case across all QMDB catchments (Table 1, Supplementary Table S1).
TABLE 1. Summary drought statistics for each QMDB river based on available stream gauge data for long term records and the 2018–2020 drought. For this summary, droughts are identified as consecutive days with zero flow recorded in gauge records, with means and ranges (minimum-maximum) of values for all gauges in each catchment (indicated as n). The rank order of the 2018–2020 drought is based on its duration in comparison to all other droughts in each gauge record. Data for individual gauges are presented in Supplementary Table S1.
Between 1973–2020 at least one river was in drought on 34% of days. On five previous occasions (in 1994, 1995, 2002, 2003, 2006), there were short periods when five of the eight rivers were simultaneously in drought. The maximum number of rivers in simultaneous drought was six and this occurred on only one occasion and for 35 days (0.06% of days) during the 2018–2020 drought (Figure 3), confirming this drought as having the greatest spatial synchrony across the QMDB of all recorded droughts.
FIGURE 3. Drought synchrony across the rivers of the Queensland Murray-Darling Basin between 1973–2020 showing that the 2018–2020 drought had the greatest synchrony. (A) shows the number of rivers in drought on each day and (B) shows the sum of days with different number of rivers simultaneously in drought, with zero rivers excluded.
At the peak of the 2018–2020 drought remote sensing identified 13,504 individual waterholes with a total combined surface area of 8,410 ha across the region. Waterholes were sparse and occupied on average only 11% of the river channel network at the peak of the drought (Table 2), with this scaling in an east to west gradient so that the highest proportion of the channel that was wet was the Border Rivers and the lowest the Paroo River.
TABLE 2. The percentage of the river channel network that retained surface water in waterholes (‘wet’) at the peak of the 2018–2020 drought in each QMDB river system.
Most barriers were small with a mean height of 2.7 m (range 0.5–12.1 m) and with 88% of all barriers being less than 5 m high (Figure 4) and only three barriers (120 Coolmunda Dam, 121 E. J. Beardmore Dam and 122 Glenlyon Dam) over 10 m. Barrier drown out flow thresholds averaged 100 m3 s−1 (range 1–1,051 m3 s−1) but specific thresholds could not be derived for the three largest barriers that are known to never drown out (120 Coolmunda Dam, 121 E. J. Beardmore Dam and 122 Glenlyon Dam). Despite their typically short stature, barriers had a profound and wide-spread impact on reducing opportunities for fish movement in the QMDB (Figure 5; Table 3, Supplementary Table S2). In the hypothetical absence of barriers, the average opportunities for fish passage occurred on 44% of days (range 11–98% of days). The presence of instream barriers reduced this average to only 5% of days (range 0–73% of days). The average loss of fish movement opportunities due to individual barriers was 87% of those hypothetical opportunities available without barriers (range 19–100%). Furthermore, twelve segments lost 100% of without-barrier movement opportunities, only eight segments lost less than 70% and of those only four segments lost less than 50% of movement opportunities without barriers. Barriers also increased the duration of maximum spells between fish movement opportunities from a mean of 492 days (range 109–54,160 days) to a mean of 2,619 days (range 160–280,202 days, excluding the barriers that never drown out).
FIGURE 4. Frequency histogram of the height of instream barriers in the QMDB with height bins of 1 m.
FIGURE 5. Histograms showing the frequency with which flow at the locations of QMDB instream barriers provide fish movement opportunities, expressed as the percentage of days in their associated stream gauge records, in bins of 10%. (A) applying a flow threshold of 0.058 m3 s−1 (5 Ml day−1) in the hypothetical absence of instream barriers, (B) with existing barrier drown out flow thresholds applied, representing the current case.
TABLE 3. Numbers of QMDB river segments per risk category for 1) peak 2018–2020 drought waterhole habitat availability, 2) long-term effects of barriers in reducing fish movement opportunities, 3) long term drought risk as the sum of waterhole and long-term barrier risks and the number of river segments within each waterhole risk category that were 4) drowned out providing fish passage opportunities, or 5) not drowned out preventing fish passage, but the drought breaking flows of 2020. Risk categories are defined in the Methods text and results for each river segment are presented in Supplementary Table S3.
When the river network was divided into local fish populations in 96 river segments defined by barrier locations, there was variability in peak drought waterhole habitat between segments (Table 3, Supplementary Table S3). The number of remaining waterholes per segment varied from 0–2,487 with an average of 140 and the area of persistent waterhole habitat per segment varied from 0 to 4,220 ha with an average of 88 ha. Seven segments had zero persistent waterholes, and an additional 20 had less than 10 ha of persistent waterhole habitat (Figure 6).
FIGURE 6. Spatial, patch-based graph representing both peak drought waterhole availability per river segment as the size of the circles, and magnitude of long-term fish movement opportunities past segment-defining barriers as the thickness of arrows (or crosses for impermeable barriers), both in the hypothetical absence of barriers (black arrows) and with barriers (red arrows and crosses). Red circles indicate river segments isolated by barriers. This illustrates the combined, long-term resistance and resilience risks to local fish populations across the QMDB region.
When the risks from long-term barrier impacts on movement opportunities (barrier risk) and peak-drought availability of drought refuge waterholes (waterhole risk) were combined (Figure 7; Table 3, Supplementary Table S3), fish populations were found to be at extreme risk from drought in six river segments and at high risk in further 76 segments, representing most of the region. All the extreme risk segments had no waterhole habitat and greater than 70% reduction in fish movement opportunities because of barriers (Figures 6, 7).
FIGURE 7. Map of the study area showing river segments, defined by barrier locations, colour coded to indicate the spatial distribution of the combined risks to local fish populations from drought limitation of refuge waterhole habitat availability and long-term barrier impacts on movement opportunities. Labels indicate the segment ID numbers listed in Supplementary Table S3.
The drought breaking floods in January–February 2020 provided fish passage opportunities to many river segments where in the long-term opportunities are severely limited by barriers (Table 3, Supplementary Table S3). However, there were still 22 segments, including some in every river catchment except the Warrego and Paroo, where movement opportunities were not provided by this flooding. Some of these segments had little or no refuge water habitat remaining at the end of the drought (Figure 8) and it is these segments with poor drought resistance and no opportunity for fish movement to facilitate drought resilience where local fish populations were at greatest risk from the 2018–2020 drought (Table 3, Supplementary Table S3).
FIGURE 8. Spatial, patch-based graph representing both peak drought waterhole availability per river segment as the size of the circles, and provision of fish movement opportunities past segment-defining barriers from the drought breaking flows in 2020. Arrows indicate opportunity and crosses lack of opportunity. Red circles indicate river segments isolated by barriers. This illustrates the combined resistance and resilience risks to local fish populations across the QMDB region from the 2018–2020 drought.
This study has shown that the twin impacts of drought and fragmentation by barriers pose profound risks to the long-term viability of dryland river fish populations. Drought was shown to threaten survival by eliminating the majority of habitat and was likely to also have degraded the quality of the small amount of shrinking waterhole habitat that remained by impacting water quality, limiting food quality and quantity and by enhancing biotic interactions such as competition, predation and disease (Arthington et al., 2005; Bunn et al., 2006; Sternberg et al., 2008; Sheldon et al., 2010). Then, the potential for population recovery following drought was severely compromised by the impacts of numerous in-stream barriers which individually and collectively acted to severely limit opportunities for fish to move and repopulate depleted habitat patches. While our study focuses on dryland rivers of the northern portion of Australia’s Murray Darling Basin, reliance of fish populations upon refugia and movement for their viability are characteristic of non-perennial rivers everywhere, with a recent estimate such rivers constitute up to 60% of the global river network (Messager et al., 2021). Therefore, our results have global relevance.
As expected, given the extreme climatic conditions experienced by the QMDB over the period 2018–2020 (World Meteorological Organization, 2020), we found waterhole habitat throughout the region to be very limited and highly fragmented by the drought, with the catchment-scale availability mirroring the east to west climatic gradient of increasing landscape aridity. This likely put pressure on the capacity of fish populations to resist the drought, particularly when combined with degradation of waterhole quality by anoxic conditions. Monitoring of dissolved oxygen profiles in several of the persistent waterholes over this period (Queensland Government, unpublished data) showed many episodes where dissolved oxygen concentrations fell below the 2–3 mg L−1 threshold at which native fishes generally suffer high mortality rates (Small et al., 2014). With only 11% of river habitat intact and that with poor water quality in remaining waterholes, it seems certain that regional population abundances of fishes were severely reduced. For example, results of regular, standardized sampling conducted in 2019–2020 for the Murray Darling Basin Authority (MDBA) found abundances of Murray cod in the Border Rivers dropped to 10% of pre-drought levels [Greg Ringwood (MDBA), pers comm].
When disturbances such as drought are asynchronous “portfolio effects” mean the dynamics of system components (here local fish populations in different QMDB rivers), correlate weakly or negatively with each other through space and time. This results in the dynamics of many biological systems being less variable than the individual components they are composed of, which confers system stability and reduces risk of overall system failure (Schindler et al., 2015). With synchronous drought across a region, such as occurred during the 2018–2020 drought, local fish populations in rivers fluctuate in unison and their metapopulations are at greater risk of collapse (Stier et al., 2020). Our results documenting widespread and synchronous loss of waterhole habitat during the recent drought suggests that it posed greater risks of regional fish extinctions than did other known historical QMDB droughts. However, this may have been somewhat mitigated by parts of the Condamine-Balonne system where hydrological drought was less severe, due to local water management activities such as managed dam releases and treated wastewater inputs, and which represent exceptions to this broad synchrony of drought. Without these inputs of water, it is likely that the synchrony of the 2018–2020 drought and its resulting risks to viability of fish metapopulations would have been even greater.
Climate change projections are for a doubling or more of global land area under extreme drought by 2100 (Pokhrel et al., 2021) and for increasing prevalence and severity of extreme heatwaves (Perkins-Kirkpatrick et al., 2016; Arguez et al., 2020). Regional forecasts for the study area are likewise for increasing frequency and severity of drought and heatwave conditions (The Long Paddock, 2020), putting additional pressure on the persistence of refuge waterholes (Hamilton et al., 2005) and increasing the prevalence of drought synchrony. Additionally, catchment land use has increased the supply of fine sediment beyond river transport capacity within parts of the QMDB, resulting in waterhole shallowing (Lobegeiger, 2010; Reid et al., 2017), which reduces their persistence time during droughts (Lobegeiger, 2010; Negus et al., 2015a). At catchment scales, aridification associated with climate change is also altering geomorphological processes in dryland rivers, further limiting waterhole habitat (Larkin et al., 2020a; Larkin et al., 2020b). So, although the 2018–2020 drought studied here was severe, future risks are predicted to be even greater in these and other global dryland rivers. Simulations have confirmed that loss of waterhole refuge habitat is a threat to fish population viability in dryland rivers, with extinction probability increasing with the number of waterholes lost (i.e., drought intensity) and the frequency of droughts (Bond et al., 2015). Patterns in the population genetics of these fishes indicate that historical extirpations have occurred (Huey et al., 2011), probably as a result of habitat loss during past droughts more severe than those known from the instrumental record (see Ault et al., 2014). Critically however, following past extirpations populations have recolonized from surviving locations by movement and dispersal, with signatures remaining of this process as genetic bottlenecks and founder effects (Huey et al., 2011). Unlike on these past occasions, movement in the QMDB is now severely constrained by the anthropogenic loss of opportunity imposed by instream barriers constructed over the past 50–150 years.
We found the magnitude of connectivity loss in the QMDB due to the influence of instream barriers to be large, with some barriers identified as impermeable and almost all limiting fish movement opportunities by 70% or more in the long term. An additional threat imposed by these barriers is their effect of increasing the duration of spells between fish movement opportunities. Our result that they increase the average maximum such spell from just over 1 year to over 7 years implies they have the potential to reduce the frequency of movement opportunities beyond the longevity of many QMDB fishes, allowing individuals no movement opportunities within their lifetimes, thus further impacting population viability. For example, the critically endangered silver perch requires opportunities for upstream migration to form spawning aggregations, yet this species rarely exceeds 7 years of age in the wild (Koehn et al., 2020). This threat may be even greater for shorter lived species because they have a lower probability of movement opportunity occurring during their lifespans.
River fragmentation is one of the major anthropogenic drivers of changes to global freshwater fish faunas and is a major threat to the survival of many freshwater species, both globally and in Australia (Hughes, 2021; Su et al., 2021). Of the barriers considered in this study, only three were higher than 10 m with the median height being just 2.7 m. Despite their diminutive size, we found them to fragment rivers resulting in severe limitation of fish movement opportunities. This is consistent with other recent studies that highlight the importance of small instream barriers in fragmenting rivers and curtailing fish movement (e.g., Couto et al., 2021). Owing to their high abundance and broad geographical extent, the wide-spread proliferation of small barriers may be an important, yet underappreciated, threat to the persistence of lotic fishes, particularly in the setting of dryland rivers with intermittent flow regimes.
Despite emerging recognition of the importance of small barriers in fragmenting rivers, the impact they had here on limiting fish movement opportunities was larger than we initially expected. This is because the highly intermittent hydrology of these rivers is dominated by small flow events, adequate to permit fish passage in the absence of instream structures, but insufficient to drown out even small barriers. This means that naturally (i.e., without artificial barriers) most days that these rivers flowed permitted fish passage, but now many flow-days do not, as larger events that drown out barriers are comparatively rare. Water resource development may have exacerbated this effect by reducing the occurrence of flows sufficient to allow passage, particularly for some of the larger weirs, but it is the influence of physical barriers imposed by the weirs themselves that is the major driver of the reduced fish movement opportunities we have documented for the QMDB. In a broader context, the average loss of fish connectivity of 87% observed here in a dryland river setting is much greater than the average 24% reduction in connectivity simulated to result from tens of thousands of small hydroelectric dams on Brazilian rivers (Couto et al., 2021). Although different methods were used to assess connectivity, this difference indicates the inherent and magnified threat that even small barriers pose to fish movement in rivers with intermittent flow regimes.
Barriers have greatest impact on fish movement when flows are low. At higher flows, barriers can potentially be passable when drowned out, though some fish may still avoid them despite opportunity to pass (Koehn and Crook, 2013). When flow provides opportunity for adult fish in QMDB waterholes to move, those that do move upstream and downstream in approximately equal proportions (Marshall et al., 2016; Harding, 2020; DES, 2021), so they encounter barriers in both upstream and downstream directions. In general, instream barriers have greater impact upon fish movement in upstream rather than downstream directions (Stuart and Sharpe, 2020). This is demonstrated for the QMDB by a study of fish passage in the Moonie River (Marshall et al., 2016). Golden perch were recorded moving both downstream and upstream past in-stream barriers. Upstream passage occurred only during drown out conditions and when flow height was at least 0.2 m higher than the weir wall. Most but not all downstream passage also occurred when the weir was drowned out (Queensland Government, unpublished data). Similar results have been found for golden perch and Murray cod in the Condamine River (Harding, 2020) and for golden perch, Hyrtl’s tandan and common carp in the Narran and Culgoa Rivers (DES, 2020). While opportunities to move downstream may be less constrained, downstream passage over barriers can be hazardous for fish. Changes in pressure associated with downstream passage of adult fish, larvae and eggs over weirs can severely limit survival and thus dispersal success (Boys et al., 2016a; Boys et al., 2016b; Pflugrath et al., 2018). No data on these impacts have been collected for QMDB barriers, so the significance of this “barotrauma” as a constraint to downstream passage is uncertain.
It is also well recognized that different species of fishes and different sized individuals of the same species have different capabilities in passing barriers when there are opportunities provided by flow (Hermoso et al., 2021). Australian freshwater fishes have a reduced swimming capacity in comparison to species on other continents, but swimming performance data for Australian species is scarce (Watson et al., 2019 in review). In a recent multi-species study, body length explained most of the interspecific variation in swimming performance of Australian freshwater species, including several that are ubiquitous throughout the QMDB (Watson et al., 2019 in review). This study also found that swimming endurance and success in traversing a distance decreased with increasing flow velocity, with golden perch as an example having a sprint swim speed (i.e., maximum speed) of less than 0.5 m s−1. This swimming performance, typical of others from the study area, helps explain why QMDB fishes require barrier drown out for upstream passage, as without drown out there is a rapid increase in flow velocity across the weir which exceeds their swimming capability to pass.
While the detrimental effects of barriers on river fishes are well documented, they can also have potentially beneficial effects on waterhole habitat persistence by artificially increasing water depth (Sheldon et al., 2010). This is the primary purpose of most of the barriers in our study where they increase the availability of water during drought for use by riparian properties as a stock and domestic water source. The benefits of this for fish are uncertain (Sheldon et al., 2010), as there is insufficient information on the relative quality of habitat in ponded areas associated with barriers in comparison to natural waterholes. Barriers can also protect upstream fish populations from invasive, alien species expanding from downstream introductions (e.g., Koehn et al., 2000). The impacts of invasive common carp on native fishes and other ecosystem components have been demonstrated to be substantial in the study region (Marshall et al., 2019). However, barriers cannot prevent this damage, as the three invasive species present, common carp, goldfish and eastern gambusia, are already pervasive throughout these rivers (Negus et al., 2015a; Negus et al., 2019), possibly because they invaded and dispersed widely prior to the construction of barriers. However, there is potential that the existing network of barriers may limit or slow the advance of future invasions.
Considering the distribution of QMDB waterhole habitat at peak-drought, in relation to barrier locations and their impacts of fish movement opportunities, allowed us to identify river segments where fish populations are at greatest risk from their combined impacts. In the long-term, this risk was particularly high at 30% of the river segments which had little or no peak-drought waterhole habitat, and fish movement opportunities reduced by barriers by more than 70%. At these segments, located in the Condamine-Balonne, Moonie and Border Rivers catchments, extirpations of local fish populations are most likely, with a low probability, or at best slow rates, of natural recolonisation. As the 2020 drought-breaking flows were historically large and widespread (Queensland Government, unpublished data), fish passage opportunities from this event were much greater than the long-term averages, resulting in only 6% of river segments being at high risk. These were again distributed across the Condamine-Balonne, Moonie and Border Rivers catchments. The spatial distribution of this risk and its underlying processes of resistance and resilience has informed site selection for an ongoing project investigating abundance, movement and recruitment of local fish populations as they recover from the 2018–2020 drought.
Framing these dryland fish populations as metapopulations clarifies the ramifications of drought and fragmentation. Local populations are extirpated by drought as waterholes dry or become inhospitable, and connectivity during flow events, permitting dispersal, allows local population recolonisation, so maintaining metapopulation viability. However, as metapopulations can survive only if the recolonisation rate of local populations is at least equal to their extinction rate (Datry et al., 2017), dispersal plays a fundamental role in maintaining populations in patchy environments (Zhang et al., 2021) such as fish in dryland rivers. This implies that if the extirpation of local fish populations increases, as predicted under the influences of drought, climate change and other stressors to waterhole refuge persistence and function outlined above, metapopulations would remain viable only if there is an equivalent rate of recolonisation maintained. Our results showing fragmentation and large reductions in movement opportunities imposed by barriers cast doubt that this will be the case. However, vulnerability of different fish species to metapopulation extinction will depend on a complex interplay between fragment sizes and interactions of their life history traits such as longevity and dispersal capability with hydrology and barrier hydraulics (Gido et al., 2015; Carvajal-Quintero et al., 2017). Some fish species that were historically abundant in QMDB rivers (Scott, 2005) are already extinct or functionally extinct in places, including silver perch throughout the region, southern purple spotted gudgeon throughout the region (apart from some headwater reaches), eel-tailed catfish in the Paroo River and Australian smelt and Murray river rainbowfish in the Moonie River (Koehn et al., 2020; MDBA, 2020; Queensland Government, 2020). The roles of drought and fragmentation in these extirpations is unclear but given the risks we have identified it is reasonable to assume they have played a role.
As metapopulation extinctions from drought and fragmentation are expected to be preceded by losses of genetic diversity (Cole et al., 2016), trends in genetic diversity in dryland fish metapopulations, using metrics such as effective population size (Hughes et al., 2012), should be monitored to forewarn of impending extinctions. Future work should also be undertaken to cross-check fish distributional records with the risk indexes presented in this study to assess if barriers impact different species of fishes differently. However, the general homogenous fish fauna of the region (Prior et al., 2005; Negus et al., 2015b; Marshall et al., 2019; Negus et al., 2019), with individual sites representing nested subsets of the regional fauna (Balcombe et al., 2006), suggests that all refugia are important and that networks of multiple, connected waterholes are required to maintain regional metapopulations of all species. This renders systematic spatial prioritization approaches to mitigation of barriers (Hermoso and Filipe, 2021) less useful here than in situations with stronger spatial structure to regional fish assemblage composition.
Results of this study are directly relevant to informing the management of native fish in the QMDB, and the approach is applicable to dryland rivers and others with intermittent flow regimes elsewhere. Sustainable management of native fish populations in the QMDB is a core objective of management and policy at multiple jurisdictional scales: at the federal scale via the Basin Wide Environmental Watering Strategy of the Murray-Darling Basin Plan (Murray-Darling Basin Authority, 2019), at the Queensland state scale via the Water Act 2000 and subsidiary catchment Water Plans (The State of Queensland, 2017; The State of Queensland, 2019a; The State of Queensland, 2019b), and at local scales via initiatives such as catchment restoration (Southern Queensland Landscapes, 2021) and native fish habitat improvement (OzFish, 2021). The spatial arrangement and locations of refuge waterholes at peak drought mapped here provide obvious targets for focusing local management of stressors to resident fish within waterholes (Negus et al., 2015a; Negus et al., 2015b), such as habitat augmentation (e.g., artificial watering or “re-snagging” with large wood), riparian vegetation restoration, mitigation of fine sediment accumulation, stock and feral mammal exclusion fencing, invasive carp control and management of poor water quality. Superimposing the barrier effects on this landscape-scale habitat template permits prioritization of works to improve fish passage past barriers, such as by weir removal, addition of suitable fish passage devices or by managing flow events to achieve barrier drown out for the best benefit to fish populations at nested spatial scales. To this effect, recommendations based upon this study were provided by the Queensland Government to the Australian Government to aid their prioritization of such measures under an AUS$180 million programme of works to complement the benefits of water buy-backs for native fish in the northern Murray Darling Basin (Australian Government, 2020). Ideally, all unnecessary barriers should be removed or modified to facilitate fish passage, but such considerations require a detailed and strategic cost benefit analysis that has not presently been undertaken. Such an analysis should consider the socio-economic and ecological benefits of each barrier versus the risks it imposes to fish populations (Couto et al., 2021). The results of this study provide the necessary information for the QMDB on the ecological costs of existing barriers and the benefits that passage mitigation would proffer to native fish populations. Small barriers, such as those that are characteristic of our study region, have been shown to be the easiest and most economically feasible to remove (Poff and Hart, 2002), giving hope that, with suitable policy and funding, the risks we identify here could be mitigated. Finally, the river segments with the greatest combined risk (i.e., extreme risk segments on Figure 7 and in Supplementary Table S3) are the locations where extirpations of local populations of fishes are most likely with the least likelihood of recolonisation. To maintain local populations throughout the QMDB, these six segments are the top priority for mitigation measures. There are also 47 high risk segments, with some in every river except the Paroo and including all the segments of the Moonie River, where consideration should also be given to measures that would reduce risk to local populations of fishes.
The original contributions presented in the study are included in the article/Supplementary Material, further inquiries can be directed to the corresponding author.
JM conceived of the research and its design, and led data analysis and the writing of the manuscript. JL assisted with design, analysis and writing. AS undertook the remote sensing spatial analyses for mapping of waterholes and assisted with writing.
This work was jointly funded by the Queensland Government and the Commonwealth Environmental Water Office.
Author AS was employed by the company Ozius Pty Ltd.
The remaining authors declare that the research was conducted in the absence of any commercial or financial relationships that could be construed as a potential conflict of interest.
All claims expressed in this article are solely those of the authors and do not necessarily represent those of their affiliated organizations, or those of the publisher, the editors and the reviewers. Any product that may be evaluated in this article, or claim that may be made by its manufacturer, is not guaranteed or endorsed by the publisher.
There are many people who assisted with this work in many ways. Thanks to Andrea Prior (DNRME) for providing data to assist with calibrating the identification of water by remote sensing. Thanks to Neal Foster, Michael Peat and Lindsay White from the Commonwealth Environmental Water Office (CEWO) for funding and encouragement to undertake this work. Thanks to Mark Jacobs, Paul Lawrence and Andrew Moss from Queensland Department of Environment and Science (DES) for funding the waterhole mapping. Thanks to Peter Brownhalls from Queensland Department of Natural Resources, Mines and Energy (DNRME) for advice and data used to identify peak drought dates and understand the release of water from Beardmore Dam in 2019 and Paul Harding (DES) for providing 2020 flow statistics. Copernicus Sentinel satellite images were sourced from the Queensland Government’s Remote Sensing Centre (and Joint Remote Sensing Research Program). Thanks to Norbert Menke, Ryan Woods (DES), Andrea Prior, Tess Mullins and Janice Kerr (DNRME) for providing barrier locations and drown out estimates. Thanks to Kate Hodges and Ryan Woods (DES) for providing advice on fish movement behaviour in the Condamine-Balonne system, and to Craig Franklin and Rebecca Cramp for providing advice and access to their in review study of Australian freshwater fish swimming capabilities. Thanks to Myriam Raymond and Brad Mayger (DES) for their tremendous support with GIS spatial analyses to define river segments and calculate waterhole statistics for the segments and to and Joanna Blessing (DES) for producing the map for Figures 1, 7. Thanks to John Tibby, Peter Negus, Bill Senior and two reviewers for critically considering earlier drafts of this manuscript. We are very grateful for all these inputs!
The Supplementary Material for this article can be found online at: https://www.frontiersin.org/articles/10.3389/fenvs.2021.671556/full#supplementary-material
Albanese, B., Angermeier, P. L., and Dorai-Raj, S. (2004). Ecological Correlates of Fish Movement in a Network of Virginia Streams. Can. J. Fish. Aquat. Sci. 61, 857–869. doi:10.1139/F04-096
Arguez, A., Hurley, S., Inamdar, A., Mahoney, L., Sanchez-Lugo, A., Yang, L., et al. (2020). Should We Expect Each Year in the Next Decade (2019-28) to Be Ranked Among the Top 10 Warmest Years Globally?. Bull. Am. Meteorol. Soc. 101, E655–E663. doi:10.1175/BAMS-D-19-0215.1
Arthington, A. H., and Balcombe, S. R. (2011). Extreme Flow Variability and the 'boom and Bust' Ecology of Fish in Arid-Zone Floodplain Rivers: a Case History with Implications for Environmental Flows, Conservation and Management. Ecohydrol. 4, 708–720. doi:10.1002/eco.221
Arthington, A. H., Balcombe, S. R., Wilson, G. A., Thoms, M. C., and Marshall, J. (2005). Spatial and Temporal Variation in Fish-Assemblage Structure in Isolated Waterholes during the 2001 Dry Season of an Arid-Zone Floodplain River, Cooper Creek, Australia. Mar. Freshw. Res. 56, 25–35. doi:10.1071/MF04111
Ault, T. R., Cole, J. E., Overpeck, J. T., Pederson, G. T., and Meko, D. M. (2014). Assessing the Risk of Persistent Drought Using Climate Model Simulations and Paleoclimate Data. J. Clim. 27, 7529–7549. doi:10.1175/JCLI-D-12-00282.1
Australian Government (2020). Delivering Environmental Works and Measures for the Northern Basin. Available at: https://www.agriculture.gov.au/water/mdb/basin-plan/commitments/environmental-works-measures (Accessed February 3, 2021).
Balcombe, S. R., Arthington, A. H., Foster, N. D., Thoms, M. C., Wilson, G. G., and Bunn, S. E. (2006). Fish Assemblages of an Australian Dryland River: Abundance, Assemblage Structure and Recruitment Patterns in the Warrego River, Murray - Darling Basin. Mar. Freshw. Res. 57, 619–633. doi:10.1071/MF06025
Balcombe, S. R., and Arthington, A. H. (2009). Temporal Changes in Fish Abundance in Response to Hydrological Variability in a Dryland Floodplain River. Mar. Freshw. Res. 60, 146–159. doi:10.1071/MF08118
Balcombe, S. (2005). The Role of Refugia in Maintaining Biodiversity in Dryland Rivers. Canberra ACT Australia: CRC for Freshwater Ecology.
Biggs, A. J. W., Silburn, D. M., and Power, R. E. (2013). Catchment Salt Balances in the Queensland Murray-Darling Basin, Australia. J. Hydrol. 500, 104–113. doi:10.1016/j.jhydrol.2013.07.027
BoM (2014). Geofabric Surface Network V2.1.1. Available at: https://data.gov.au/data/dataset/australian-hydrological-geospatial-fabric-geofabric-surface-network-v2-x (Accessed July 15, 2020).
Bond, N. R., Balcombe, S. R., Crook, D. A., Marshall, J. C., Menke, N., and Lobegeiger, J. S. (2015). Fish Population Persistence in Hydrologically Variable Landscapes. Ecol. Appl. 25, 901–913. doi:10.1890/14-1618.1
Boys, C. A., Robinson, W., Miller, B., Pflugrath, B., Baumgartner, L. J., Navarro, A., et al. (2016a). A Piecewise Regression Approach for Determining Biologically Relevant Hydraulic Thresholds for the protection of Fishes at River Infrastructure. J. Fish. Biol. 88, 1677–1692. doi:10.1111/jfb.12910
Boys, C. A., Robinson, W., Miller, B., Pflugrath, B., Baumgartner, L. J., Navarro, A., et al. (2016b). How Low Can They Go when Going with the Flow? Tolerance of Egg and Larval Fishes to Rapid Decompression. Biol. Open 5, 786–793. doi:10.1242/bio.017491
Brodersen, J., Nilsson, P. A., Hansson, L.-A., Skov, C., and Brönmark, C. (2008). Condition-dependent Individual Decision-Making Determines Cyprinid Partial Migration. Ecology 89, 1195–1200. doi:10.1890/07-1318.1
Bunn, S. E., Thoms, M. C., Hamilton, S. K., and Capon, S. J. (2006). Flow Variability in Dryland Rivers: Boom, Bust and the Bits in between. River Res. Applic. 22, 179–186. doi:10.1002/rra.904
Carvajal-Quintero, J. D., Januchowski-Hartley, S. R., Maldonado-Ocampo, J. A., Jézéquel, C., Delgado, J., and Tedesco, P. A. (2017). Damming Fragments Species' Ranges and Heightens Extinction Risk. Conservation Lett. 10, 708–716. doi:10.1111/conl.12336
Chan, K. (2001). Partial Migration in Australian Landbirds: A Review. Emu - Austral Ornithology 101, 281–292. doi:10.1071/MU00034
Chapman, L. J., and Kramer, D. L. (1991). The Consequences of Flooding for the Dispersal and Fate of Poeciliid Fish in an Intermittent Tropical Stream. Oecologia 87, 299–306. doi:10.1007/bf00325270
Cole, T. L., Hammer, M. P., Unmack, P. J., Teske, P. R., Brauer, C. J., Adams, M., et al. (2016). Range-wide Fragmentation in a Threatened Fish Associated with post-European Settlement Modification in the Murray-Darling Basin, Australia. Conserv. Genet. 17, 1377–1391. doi:10.1007/s10592-016-0868-8
Commonwealth of Australia, Bureau of Meteorology (2021). Climate Statistics for Australian Sites. web page. Available at: http://www.bom.gov.au/climate/averages/tables/ca_qld_names.shtml (Accessed February 2, 2021).
Couto, T. B. A., Messager, M. L., and Olden, J. D. (2021). Safeguarding Migratory Fish via Strategic Planning of Future Small Hydropower in Brazil. Nat. Sustain. 4, 409–416. doi:10.1038/s41893-020-00665-4
Crook, D. A., Reich, P., Bond, N. R., McMaster, D., Koehn, J. D., and Lake, P. S. (2010). Using Biological Information to Support Proactive Strategies for Managing Freshwater Fish during Drought. Mar. Freshw. Res. 61, 379–387. doi:10.1071/MF09209
Datry, T., Corti, R., Heino, J., Hugueny, B., Rolls, R. J., and Ruhí, A. (2017). “Habitat Fragmentation and Metapopulation, Metacommunity, and Metaecosystem Dynamics in Intermittent Rivers and Ephemeral Streams,” in Intermittent Rivers and Ephemeral Streams, Ecology and Management. Editors T. Datry, N. Bonada, and A. Boulton (Academic Press), 377–403. doi:10.1016/b978-0-12-803835-2.00014-0
DES (2021). Fish Movement Behaviour in the North Murray – Darling Basin: Report on the Closure of the Murray – Darling Basin. Queensland Government, Brisbane: Department of Environment and Science.
DES (2020). Pioneer Valley Water Resource Planning Area — Facts and Maps. WetlandInfo. Available at: https://wetlandinfo.des.qld.gov.au/wetlands/facts-maps/water-resource-planning-area-pioneer-valley/ (Accessed January 13, 2020).
DES (2018a). Review of Water Plan (Border Rivers) 2003 and Resource Operations Plan: Environmental Assessment Report. Queensland Government, Brisbane: Department of Environment and Science.
DES (2018b). Review of Water Plan (Condamine and Balonne) 2004 and Resource Operations Plan: Environmental Assessment Report. Queensland Government, Brisbane: Department of Environment and Science.
DNRME (2019). Lower Balonne Flow Report April and May 2019. Toowoomba, Australia: Queensland Government, Department of Natural Resources Mines and Energy.
DSITI (2015). Waterhole Refuge Mapping and Persistence Analysis in the Lower Balonne and Barwon-Darling Rivers. Brisbane, Australia: Department of Science, Information Technology and Innovation, Queensland Government. Available at: https://www.mdba.gov.au/sites/default/files/pubs/FINAL Report_Waterholes Project northern Basin WEB OPTIMISED .pdf (Accessed February 8, 2019).
DSITIA (2013). Review of Water Resource (Warrego, Paroo, Bulloo and Nebine) Plan 2003 and Resource Operations Plan: Environmental Risk Assessment for Selected Ecological Assets. Brisbane, Australia, AustraliaInformation Technology, Innovation and the Arts: State of Queensland; Department of Science.
Eros, T., Olden, J. D., Schick, R. S., Schmera, D., and Fortin, M. J. (2012). Characterizing Connectivity Relationships in Freshwaters Using Patch-Based Graphs. Landsc. Ecol. 27, 303–317. doi:10.1007/s10980-011-9659-2
Fagan, W. F. (2002). Connectivity, Fragmentation, and Extinction Risk in Dendritic Metapopulations. Ecology 83, 3243–3249. doi:10.1890/0012-9658(2002)083[3243:cfaeri]2.0.co;2
Fagan, W. F., Unmack, P. J., Burgess, C., and Minckley, W. L. (2002). Rarity, Fragmentation, and Extinction Risk in Desert Fishes. Ecology 83, 3250–3256. doi:10.1890/0012-9658(2002)083[3250:rfaeri]2.0.co;2
Fielder, D. P., Davidson, W., and Barratt, P. J. (2011). Aquatic Conservation Assessments (ACA), Using AquaBAMM, for the Wetlands of the Murray-Darling Basin. Queensland Government, Brisbane QLD Australia: Department of Environment and Resource Management.
Fisher, A., Flood, N., and Danaher, T. (2016). Comparing Landsat Water index Methods for Automated Water Classification in Eastern Australia. Remote Sensing Environ. 175, 167–182. doi:10.1016/j.rse.2015.12.055
Gido, K. B., Whitney, J. E., Perkin, J. S., and Turner, T. F. (2015). Fragmentation, Connectivity and Fish Species Persistence in Freshwater Ecosystems,” In Conservation of Freshwater Fishes. Editors G. P. Closs, M. Krkosek, and J. D. Olden (Cambridge, United Kingdom and New York, NY: Cambridge University Press), 292–323.
Grill, G., Lehner, B., Thieme, M., Geenen, B., Tickner, D., Antonelli, F., et al. (2019). Mapping the World's Free-Flowing Rivers. Nature 569, 215–221. doi:10.1038/s41586-019-1111-9
Hamilton, S. K., Bunn, S. E., Thoms, M. C., and Marshall, J. C. (2005). Persistence of Aquatic Refugia between Flow Pulses in a Dryland River System (Cooper Creek, Australia). Limnol. Oceanogr. 50, 743–754. doi:10.4319/lo.2005.50.3.0743
Harding, D. (2020). Acoustic Telemetry Project Update for the Condamine-Balonne Rivers. Queensland Government, Toowoomba: Department of Natural Resources and Mines and Department of Science, Technology and Innovation.
Hermoso, V., Clavero, M., and Filipe, A. F. (2021). An Accessible Optimisation Method for Barrier Removal Planning in Stream Networks. Sci. Total Environ. 752, 141943. doi:10.1016/j.scitotenv.2020.141943
Hermoso, V., and Filipe, A. F. (2021). Offsetting Connectivity Loss in Rivers: Towards a No-Net-Loss Approach for Barrier Planning. Biol. Conservation 256, 109043. doi:10.1016/j.biocon.2021.109043
Hopper, G. W., Gido, K. B., Pennock, C. A., Hedden, S. C., Frenette, B. D., Barts, N., et al. (2020). Nowhere to Swim: Interspecific Responses of Prairie Stream Fishes in Isolated Pools during Severe Drought. Aquat. Sci. 82. doi:10.1007/s00027-020-0716-2
Horwitz, R. J. (1978). Temporal Variability Patterns and the Distributional Patterns of Stream Fishes. Ecol. Monogr. 48, 307–321. doi:10.2307/2937233
Huey, J. A., Schmidt, D. J., Balcombe, S. R., Marshall, J. C., and Hughes, J. M. (2011). High Gene Flow and Metapopulation Dynamics Detected for Three Species in a Dryland River System. Freshw. Biol. 56, 2378–2390. doi:10.1111/j.1365-2427.2011.02666.x
Hughes, J. M., Real, K. M., Marshall, J. C., and Schmidt, D. J. (2012). Extreme Genetic Structure in a Small-Bodied Freshwater Fish, the Purple Spotted Gudgeon, Mogurnda Adspersa (Eleotridae). PLoS One 7. Available at: http://www.scopus.com/inward/record.url?eid=2-s2.0-84863772214&partnerID=40&md5=11198cd5724a6790c9416ab35814f760.
Hughes, K. (2021). The World’s Forgotten Fishes. Gland, Switzerland: WWF International. Available at: https://www.wwf.ch/sites/default/files/doc-2021-02/World%27s Forgotten Fishes %28REPORT FINAL%29.pdf. doi:10.1164/ajrccm-conference.2021.203.1_meetingabstracts.a4893
Keller, R. J., Peterken, C. J., and Berghuis, A. P. (2012). Design and Assessment of Weirs for Fish Passage under Drowned Conditions. Ecol. Eng. 48, 61–69. doi:10.1016/j.ecoleng.2011.06.037
Kerezsy, A., Balcombe, S. R., Arthington, A. H., and Bunn, S. E. (2011). Continuous Recruitment Underpins Fish Persistence in the Arid Rivers of Far-Western Queensland, Australia. Mar. Freshw. Res. 62, 1178–1190. Available at: http://www.scopus.com/inward/record.url?eid=2-s2.0-80053128786&partnerID=40&md5=48bb1594713f885c4a4688d3e7343c0c. doi:10.1071/mf11021
Kerezsy, A., Balcombe, S. R., Tischler, M., and Arthington, A. H. (2013). Fish Movement Strategies in an Ephemeral River in the Simpson Desert, Australia. Austral Ecol. 38, 798–808. doi:10.1111/aec.12075
Kerr, J., Kimball, A., Prior, A., Ellaway, C., and Luke, L. (2018). Barriers to Fish Passage in the Queensland Murray-Darling Basin - A Report for the South-West Queensland Environmental Flows Assessment Program. Queensland Government, Brisbane: Department of Natural Resources and Mines and Department of Science, Technology and Innovation.
King, A. J., Tonkin, Z., and Lieshcke, J. (2012). Short-term Effects of a Prolonged blackwater Event on Aquatic Fauna in the Murray River, Australia: Considerations for Future Events. Mar. Freshw. Res. 63, 576–586. doi:10.1071/MF11275
Kingsford, R. T. (1999). Aerial Survey of Waterbirds on Wetlands as a Measure of River and Floodplain Health. Freshw. Biol. 41, 425–438. doi:10.1046/j.1365-2427.1999.00440.x
Kirono, D. G. C., Round, V., Heady, C., Chiew, F. H. S., and Osbrough, S. (2020). Drought Projections for Australia: Updated Results and Analysis of Model Simulations. Weather Clim. Extremes 30, 100280. doi:10.1016/j.wace.2020.100280
Knighton, A. D., and Nanson, G. C. (2000). Waterhole Form and Process in the Anastomosing Channel System of Cooper Creek, Australia. Geomorphology 35, 101–117. doi:10.1016/S0169-555X(00)00026-X
Koehn, J., Brumley, A., and Gehrke, P. (2000). Managing the Impacts of Carp. Canberra: Bureau of Rural Sciences (Department of Agriculture, Fisheries and Forestry - Australia). Available at: http://data.daff.gov.au/anrdl/metadata_files/pe_brs90000002059.xml. doi:10.1061/40475(278)46
Koehn, J. D., and Crook, D. A. (2013). “Movements and Migration,” Ecology of Australian Freshwater Fishes. Editors P. Humphries, and K. Walker (Melbourne, Australia: CSIRO Publishing), 105–130.
Koehn, J. D., Raymond, S. M., Stuart, I., Todd, C. R., Balcombe, S. R., Zampatti, B. P., et al. (2020). A Compendium of Ecological Knowledge for Restoration of Freshwater Fishes in Australia. Mar. Freshw. Res. 71, 1391–1463. doi:10.1071/MF20127
Labbe, T. R., and Fausch, K. D. (2000). Dynamics of Intermittent Stream Habitat Regulate Persistence of a Threatened Fish at Multiple Scales. Ecol. Appl. 10, 1774–1791. doi:10.1890/1051-0761(2000)010[1774:doishr]2.0.co;2
Larkin, Z. T., Ralph, T. J., Tooth, S., and Duller, G. A. T. (2020a). A Shifting' river of Sand': The Profound Response of Australia's Warrego River to Holocene Hydroclimatic Change. Geomorphology 370, 107385. doi:10.1016/j.geomorph.2020.107385
Larkin, Z. T., Ralph, T. J., Tooth, S., Fryirs, K. A., and Carthey, A. J. R. (2020b). Identifying Threshold Responses of Australian Dryland Rivers to Future Hydroclimatic Change. Sci. Rep. 10, 1–15. doi:10.1038/s41598-020-63622-3
Levins, R. (1969). Some Demographic and Genetic Consequences of Environmental Heterogeneity for Biological Control. Bull. Entomol. Soc. America 15, 237–240. doi:10.1093/besa/15.3.237
Liermann, C. R., Nilsson, C., Robertson, J., and Ng, R. Y. (2012). Implications of Dam Obstruction for Global Freshwater Fish Diversity. Bioscience 62, 539–548. doi:10.1525/bio.2012.62.6.5
Lobegeiger, J. (2010). Refugial Waterholes Project: Research Highlights. Brisbane: Department of Environment and Resource Management, Queensland Government. Available at: http://tinyurl.com/357qnzb.
Magoulick, D. D., and Kobza, R. M. (2003). The Role of Refugia for Fishes during Drought: a Review and Synthesis. Freshw. Biol. 48, 1186–1198. doi:10.1046/j.1365-2427.2003.01089.x
Magoulick, D. D. (2000). Spatial and Temporal Variation in Fish Assemblages of Drying Stream Pools: The Role of Abiotic and Biotic Factors. Aquat. Ecol. 34, 29–41. doi:10.1023/a:1009914619061
Marshall, J. C., Blessing, J. J., Clifford, S. E., Hodges, K. M., Negus, P. M., and Steward, A. L. (2019). Ecological Impacts of Invasive Carp in Australian Dryland Rivers. Aquat. Conserv: Mar Freshw Ecosyst 29, 1870–1889. doi:10.1002/aqc.3206
Marshall, J. C., Menke, N., Crook, D. A., Lobegeiger, J. S., Balcombe, S. R., Huey, J. A., et al. (2016). Go with the Flow: the Movement Behaviour of Fish from Isolated Waterhole Refugia during Connecting Flow Events in an Intermittent Dryland River. Freshw. Biol. 61, 1242–1258. doi:10.1111/fwb.12707
Marshall, J. C., Steward, A. L., and Harch, B. D. (2006). Taxonomic Resolution and Quantification of Freshwater Macroinvertebrate Samples from an Australian Dryland River: The Benefits and Costs of Using Species Abundance Data. Hydrobiologia 572, 171–194. doi:10.1007/s10750-005-9007-0
MDBA (2019). Native Fish Emergency Response Plan 2019-20. Canberra ACT Australia. Available at: https://www.mdba.gov.au/sites/default/files/pubs/native-fish-emergency-response-plan - 2019-20.pdf.
MDBA (2020). NATIVE FISH RECOVERY STRATEGY: Working Together for the Future of Native Fish. Canberra ACT Australia. Available at: https://www.mdba.gov.au/sites/default/files/pubs/Native Fish Recovery Strategy - June 2020.pdf.
Messager, M. L., Lehner, B., Cockburn, C., Lamouroux, N., Pella, H., Snelder, T., et al. (2021). Global Prevalence of Non-perennial Rivers and Streams. Nature 594, 391–397. doi:10.1038/s41586-021-03565-5
Moffatt, D., and Voller, J. (2002). Fish and Fish Habitat of the Queensland Murray-Darling Basin. Brisbane, Australia: Queensland Government, Department of Primary Industries.
Murphy, A. L., Pavlova, A., Thompson, R., Davis, J., and Sunnucks, P. (2015). Swimming through Sand: Connectivity of Aquatic Fauna in Deserts. Ecol. Evol. 5, 5252–5264. doi:10.1002/ece3.1741
Murray-Darling Basin Authority (2019). Basin-wide Environmental Watering Strategy. Canberra ACT Australia.
Nanson, G. C. G. C., Tooth, S., and Knighton, A. D. (2002). “A Global Perspective on Dryland Rivers: Perceptions, Misconceptions and Distinctions,” in Dryland Rivers: Hydrology and Geomorphology of Semi-arid Channels. Editors L. Bull, and M. Kirby (Chichester, U.K.: John Wiley & Sons Limited), 17–54. Available at: https://books.google.gr/books?id=qjHoYZXQee0C.
Negus, P., Blessing, J., Clifford, S., and Steward, A. (2015a). Riverine Assessment in the Warrego, Paroo, Bulloo and Nebine Catchments. Q–Catchments: Technical Report 2012. Queensland Government, Brisbane, Australia: partment of Science, Information Technology and Innovation.
Negus, P., Blessing, J., Steward, A., Clifford, S., Hansen, D., and Hammill, B. (2015b). Ecological Risk Assessment and Threat Prioritisation in Queensland’s Eastern Murray Darling Rivers: Condamine, Balonne and Maranoa; Lower Balonne; Moonie; and Border Rivers, 2012, Q–Catchments Program. Queensland Government, Brisbane: Depaetment of Science Information Technology and Innovation.
Negus, P. M., Blessing, J. J., Clifford, S. E., Steward, A. L., and Lobegeiger, J. S. (2019). Queensland’s Eastern Murray-Darling Riverine Ecosystems: Threats and Condition. Brisbane: Q-catchments Technical Report.
Newham, M., Southwell, B., Thames, D., Moss, A., Moulton, D., and Bennett, L. (2018). Draft Environmental Values and Water Quality Objectives: Queensland Murray Darling Basin. Queensland Government, Brisbane, Australia: Department of Environment and Science.
OzFish (2021). Basin Fish Action Projects. Available at: https://ozfish.org.au/programs/basin-fish-action/ (Accessed February 3, 2021).
Pärssinen, V., Hulthén, K., Brönmark, C., Skov, C., Brodersen, J., Baktoft, H., et al. (2020). Maladaptive Migration Behaviour in Hybrids Links to Predator‐mediated Ecological Selection. J. Anim. Ecol. 89, 2596–2604. doi:10.1111/1365-2656.13308
Perkin, J. S., Gido, K. B., Costigan, K. H., Daniels, M. D., and Johnson, E. R. (2015). Fragmentation and Drying Ratchet Down Great Plains Stream Fish Diversity. Aquat. Conserv: Mar. Freshw. Ecosyst. 25, 639–655. doi:10.1002/aqc.2501
Perkins-Kirkpatrick, S. E., White, C. J., Alexander, L. V., Argüeso, D., Boschat, G., Cowan, T., et al. (2016). Natural Hazards in Australia: Heatwaves. Climatic Change 139, 101–114. doi:10.1007/s10584-016-1650-0
Pflugrath, B. D., Boys, C. A., and Cathers, B. (2018). Predicting Hydraulic Structure-Induced Barotrauma in Australian Fish Species. Mar. Freshw. Res. 69, 1954–1961. doi:10.1071/MF18137
Poff, N. L., and Hart, D. D. (2002). How Dams Vary and Why it Matters for the Emerging Science of Dam Removal. Bioscience 52, 659–668. doi:10.1641/0006-3568(2002)052[0659:hdvawi]2.0.co;2
Pokhrel, Y., Felfelani, F., Satoh, Y., Boulange, J., Burek, P., Gädeke, A., et al. (2021). Global Terrestrial Water Storage and Drought Severity under Climate Change. Nat. Clim. Chang. 11, 226–233. doi:10.1038/s41558-020-00972-w
Prior, A., Marshall, J., Steward, A., Negus, P., McGregor, G., and Marshall, C. (2005). Bioregionalisation of Queensland’s Riverine Ecosystems Provides Management Framework. Brisbane, Australia: Queensland Government, Department of Primary Industries.
Puckridge, J. T., Sheldon, F., Walker, K. F., and Boulton, A. J. (1998). Flow Variability and the Ecology of Large Rivers. Mar. Freshw. Res. 49, 55–72. doi:10.1071/mf94161
Queensland Govrnment (2020). Water Monitoring Information Portal: Queensland Government, Streamflow Data, Open Stations. Available at: https://water-monitoring.information.qld.gov.au/ (Accessed August 10, 2020).
Reid, M. A., Thoms, M. C., Chilcott, S., and Fitzsimmons, K. (2017). Sedimentation in Dryland River Waterholes: a Threat to Aquatic Refugia?. Mar. Freshw. Res. 68, 668–685. doi:10.1071/MF15451
Romaní, A. M., Chauvet, E., Febria, C., Mora-Gómez, J., Risse-Buhl, U., Timoner, X., et al. (2017). The Biota of Intermittent Rivers and Ephemeral Streams: Prokaryotes, Fungi, and Protozoans. Intermittent Rivers and Ephemeral Streams: Ecology and Management. Academic Press, 161–188. doi:10.1016/B978-0-12-803835-2.00009-7
Rubalcaba, J. G., Verberk, W. C. E. P., Hendriks, A. J., Saris, B., and Woods, H. A. (2020). Oxygen Limitation May Affect the Temperature and Size Dependence of Metabolism in Aquatic Ectotherms. Proc. Natl. Acad. Sci. USA 117, 31963–31968. doi:10.1073/pnas.2003292117
Schindler, D. E., Armstrong, J. B., and Reed, T. E. (2015). The Portfolio Concept in Ecology and Evolution. Front. Ecol. Environ. 13, 257–263. doi:10.1890/140275
Scott, A. (2005). Historical Evidence of Native Fish in the Murray-Darling Basin at the Time of European Settlement - from the Diaries of the First Explorers. Canberra: CRC for Freshwater Ecology. Available at: http://freshwater.canberra.edu.au.
Sedell, J. R., Reeves, G. H., Hauer, F. R., Stanford, J. A., and Hawkins, C. P. (1990). Role of Refugia in Recovery from Disturbances: Modern Fragmented and Disconnected River Systems. Environ. Manage. 14, 711–724. doi:10.1007/bf02394720
Sheldon, F., Bunn, S. E., Hughes, J. M., Arthington, A. H., Balcombe, S. R., and Fellows, C. S. (2010). Ecological Roles and Threats to Aquatic Refugia in Arid Landscapes: Dryland River Waterholes. Mar. Freshw. Res. 61, 885–895. doi:10.1071/MF09239
Small, K., Kopf, R. K., Watts, R. J., and Howitt, J. (2014). Hypoxia, Blackwater and Fish Kills: Experimental Lethal Oxygen Thresholds in Juvenile Predatory Lowland River Fishes. PLoS One 9, e94524. doi:10.1371/journal.pone.0094524
Smith, L. C. (1997). Satellite Remote Sensing of River Inundation Area, Stage, and Discharge: A Review. Hydrol. Process. 11, 1427–1439. doi:10.1002/(sici)1099-1085(199708)11:10<1427::aid-hyp473>3.0.co;2-sx
Southern Queensland Landscapes (2021). Flourishing Landscapes Plan 2020-2021. Toowoomba, Australia. Available at: https://irp-cdn.multiscreensite.com/1018ad9f/files/uploaded/SQ Landscapes - Flourishing Landscapes Plan_01042020.pdf.
Sternberg, D., Balcombe, S., Marshall, J., and Lobegeiger, J. (2008). Food Resource Variability in an Australian Dryland River: Evidence from the Diet of Two Generalist Native Fish Species. Mar. Freshw. Res. 59, 137–144. doi:10.1071/MF07125
Sternberg, D., Balcombe, S. R., Marshall, J. C., Lobegeiger, J. S., and Arthington, A. H. (2011). Subtle 'boom and Bust' Response of Macquaria Ambigua to Flooding in an Australian Dryland River. Environ. Biol. Fish. 93, 95–104. doi:10.1007/s10641-011-9895-y
Stier, A. C., Olaf Shelton, A., Samhouri, J. F., Feist, B. E., and Levin, P. S. (2020). Fishing, Environment, and the Erosion of a Population Portfolio. Ecosphere 11, e0328. doi:10.1002/ecs2.3283
Stuart, I. G., and Sharpe, C. P. (2020). Riverine Spawning, Long Distance Larval Drift, and Floodplain Recruitment of a Pelagophilic Fish: A Case Study of golden Perch (Macquaria Ambigua) in the Arid Darling River, Australia. Aquat. Conserv: Mar. Freshw Ecosyst. 30, 675–690. doi:10.1002/aqc.3311
Su, G., Logez, M., Xu, J., Tao, S., Villéger, S., and Brosse, S. (2021). Human Impacts on Global Freshwater Fish Biodiversity. Science 371, 835–838. doi:10.1126/science.abd3369
The Long Paddock (2020). Future Climate Dashboard: Queensland Government. Available at: https://longpaddock.qld.gov.au/qld-future-climate/dashboard/#responseTab5 (Accessed July 15, 2020).
The State of Queensland (2019a). Water Plan (Border Rivers and Moonie) 2019. Australia: Water Act 2000. Available at: https://www.legislation.qld.gov.au/view/pdf/asmade/sl-2019-0012.
The State of Queensland (2019b). Water Plan (Condamine and Balonne) 2019. Australia: Water Act 2000. Available at: https://www.legislation.qld.gov.au/view/pdf/asmade/sl-2019-0011.
The State of Queensland (2017). Water Plan (Warrego, Paroo, Bulloo and Nebine) 2016. Australia: Water Act 2000. Available at: https://www.legislation.qld.gov.au/view/whole/pdf/inforce/current/sl-2016-0006.
Threatened Species Scientific Committee (2013). Conservation Advice Bidyanus Bidyanus (Silver Perch). Canberra ACT Australia. Available at: http://www.environment.gov.au/biodiversity/threatened/species/pubs/76155-conservation-advice.pdf.
Tonkin, J. D., Altermatt, F., Finn, D. S., Heino, J., Olden, J. D., Pauls, S. U., et al. (2018). The Role of Dispersal in River Network Metacommunities: Patterns, Processes, and Pathways. Freshw. Biol. 63, 141–163. doi:10.1111/fwb.13037
Vardakas, L., Kalogianni, E., Economou, A. N., Koutsikos, N., and Skoulikidis, N. T. (2017). Mass Mortalities and Population Recovery of an Endemic Fish Assemblage in an Intermittent River Reach during Drying and Rewetting. fal 190, 331–347. doi:10.1127/fal/2017/1056
Vertessy, R., Barma, D., Baumgartner, L., Mitrovic, S., Sheldon, F., and Bond, N. (2019). Independent Assessment of the 2018-19 Fish Deaths in the Lower Darling. Canberra: Australian Government.
Wang, G. (2005). Agricultural Drought in a Future Climate: Results from 15 Global Climate Models Participating in the IPCC 4th Assessment. Clim. Dyn. 25, 739–753. doi:10.1007/s00382-005-0057-9
Water NSW (2020). Real-time Water Data. Available at: https://realtimedata.waternsw.com.au/water.stm (Accessed August 10, 2020).
Watson, J. R., Goodrich, H. R., Cramp, R. L., Gordos, M. A., Yan, Y., Ward, P. J., et al. (2019). Swimming Performance Traits of Twenty-One Australian Fish Species: a Fish Passage Management Tool for Use in Modified Freshwater Systems. bioRxiv, 861898. doi:10.1101/861898
World Meteorological Organization (2020). WMO Statement on the Status of the Global Climate in 2019. Geneva, Switzerland: World Meteorological Organization. Available at: http://www.wmo.int/pages/mediacentre/press_releases/documents/WMO_1108_EN_web_000.pdf.
Keywords: waterhole refugia, weirs, dams, dispersal, movement, metapopulations, portfolio effects, disturbance synchrony
Citation: Marshall JC, Lobegeiger JS and Starkey A (2021) Risks to Fish Populations in Dryland Rivers From the Combined Threats of Drought and Instream Barriers. Front. Environ. Sci. 9:671556. doi: 10.3389/fenvs.2021.671556
Received: 24 February 2021; Accepted: 22 July 2021;
Published: 12 August 2021.
Edited by:
Eugenia López-López, Instituto Politécnico Nacional de México (IPN), MexicoReviewed by:
Rui Manuel Vitor Cortes, University of Trás-os-Montes and Alto Douro, PortugalCopyright © 2021 Marshall, Lobegeiger and Starkey. This is an open-access article distributed under the terms of the Creative Commons Attribution License (CC BY). The use, distribution or reproduction in other forums is permitted, provided the original author(s) and the copyright owner(s) are credited and that the original publication in this journal is cited, in accordance with accepted academic practice. No use, distribution or reproduction is permitted which does not comply with these terms.
*Correspondence: Jonathan C. Marshall, am9uYXRoYW4ubWFyc2hhbGxAZGVzLnFsZC5nb3YuYXU=
Disclaimer: All claims expressed in this article are solely those of the authors and do not necessarily represent those of their affiliated organizations, or those of the publisher, the editors and the reviewers. Any product that may be evaluated in this article or claim that may be made by its manufacturer is not guaranteed or endorsed by the publisher.
Research integrity at Frontiers
Learn more about the work of our research integrity team to safeguard the quality of each article we publish.