- Systemic Physiological and Ecotoxicological Research, Department of Biology, University of Antwerp, Antwerp, Belgium
In recent years, biodegradable polymers have been hailed as one of the potential solutions to the plastic pollution problem, due to their ability to fully degrade rather than break down in smaller pieces over time. However, complete degradation of biodegradable polymers is often achievable only under strictly controlled conditions (i.e. increased temperature and pressure), which are not found in the natural environment – particularly in aquatic and marine habitats. This study aims to compare the degradation performance of plastic films made of two different biodegradable polymers – polylactic acid (PLA) and polyhydroxyalkanoates (PHA) – to that of low-density polyethylene (LDPE) films, in a simulated marine environment. Plastic films of the three chosen polymers, of equal dimensions, were exposed to natural sunlight within a novel setup - which simulated the sea surface - for six months. Films were chosen as they are among the most frequently reported type of plastic litter in coastal environments worldwide, and because of the increasing adoption on the market of biodegradable films for packaging. Results showed that, after six months, no consistent degradation could be observed on any of the films–not even the biodegradable ones. Between PLA and PHA films, the latter weathered slightly more than the former, but not at a significant level. Interestingly, differences were reported among the different polymer films in terms of type and extent of biofouling, brittleness, surface charge and surface microstructural changes. Overall, this work suggests that biodegradable plastic behaves rather similarly to traditional plastic in the marine environment over a half-year span. Albeit further experiments on even longer timescales are needed, this study provides evidence that, unless properly disposed of in an industrial composter facility, biodegradable plastic may only contribute to the very problem it was intended to solve.
Introduction
Due to their favorable characteristics, plastics are employed worldwide in a variety of applications (Lebreton et al., 2017). In 2019, the global plastic production almost reached 370 million tons (PlasticsEurope, 2020), the majority of this production being employed for packaging (Geyer et al., 2017; Chen et al., 2021), which is almost always immediately discarded after a single use (United Nations Environment Programme, 2018). World population growth, access to a better quality of life, and the dynamics of modern consumerism bolstered the demand for single-use plastics, and thus contributed greatly to increase plastic waste across the globe (Rhodes, 2019; Abalansa et al., 2020; Oliveira et al., 2020). The ongoing Covid-19 pandemic has only made matters worse: production and use of plastic disposable personal protection devices (PPE) has skyrocketed, generating a tide of discarded PPE no country is ready to properly manage (Adyel, 2020). Even before this unprecedented amount of litter, plastic waste management has long represented an issue: landfill run-off, and improper or illegal disposal cause plastic litter to end in waterways and marine environments (Schmidt et al., 2017). Plastic waste in aquatic environments is aesthetically unpleasant and directly harmful to biota and humans; moreover, biological and physical agents degrade this waste to microscopic particles, known as microplastics (MPs) (Derraik, 2002; Galloway and Lewis, 2016).
Among the strategies currently considered to solve the plastic waste problem, the introduction of products made of biodegradable plastic has been hailed by some as a game-changing approach (Heidbreder et al., 2019; Luyt and Malik, 2019). Biodegradation is a biological process in which organic matter is completely or partially converted in water, CO2, methane, energy, and new biomass, by hydrolysis, photodegradation, and/or microbial degradation (Kershaw and United Nations Environment Programme, 2015). In the imagination of many, including some lawmakers, biodegradable plastics are supposed to simply dissolve in a fortnight when discarded in the environment, in a process akin to 21st-century witchcraft. The reality is that biodegradable plastics, to successfully degrade, need to be treated in an industrial composting facility, at temperatures between 50 and 60°C and with a concentrated microbial community (Folino et al., 2020). These parameters are far away from those found in uncontrolled environments. Hence, biodegradable plastics discarded in the marine environment, such as carrier bags, degrade significantly slower and with less success than in the composter, or in pure water (Accinelli et al., 2012; Deroiné et al., 2014). The adoption of biodegradable plastic has not been supported by a thorough information campaign of consumers on how to properly dispose of these items; many countries lack the necessary collection schemes and composting infrastructure (Zhu and Wang, 2020). Biodegradable plastic producers can apply for certifications offered by private entities, which are based on regional standards such as the ASTM D7473-12, 2021 and ASTM D6691-17, 2017 for degradation in pelagic marine environments. However, the available standards fail to describe the variety of marine environments (i.e. salt marshes, intertidal zones, and more), and employ unrealistic exposure parameters (Harrison et al., 2018). Moreover, a cloud of ambiguity surrounds the definition of biodegradable as an (often unsupported) product claim. This cloud shrouds some less-than-legitimate practices, such as greenwashing: companies exploit certain labels (such as “green”, “environmentally friendly”, “bio-based”) as a marketing ploy, without following their words with a sound commitment on the environmental level (Delmas and Burbano, 2011; Viera et al., 2020). An example of the reigning confusion among biodegradability is the commercialisation of oxo-degradable products, consisting in conventional plastic polymers mixed with UV-degrading additives (Thomas et al., 2012). This leads to fragmentation of the polymeric matrix in micro-particles of non-degradable material, thus increasing microplastic pollution, while effectively sweeping the (plastic) dust under the carpet (European Bioplastics, 2015).
Due to the lack of transparency around biodegradability certifications, especially those pertaining to aquatic and marine environments, the role of the scientific community is to provide clear, reliable data on the behavior of biodegradable plastics. The standards on which biodegradability tests are based (e.g. ASTM 6691) are based on parameters which, albeit easily achievable in a laboratory setting, are quite unrealistic when applied to the environment (e.g. temperatures ≥30°C). In turn, certain important environmental characteristics are difficultly reproducible to a realistic level in the laboratory, namely solar UV radiation, mechanical forces both by abiotic (e.g. currents, waves) and biotic agents (e.g. biofouling, accidental ingestion), and the presence of a diverse marine microbial community. As an example, xenon arc lamp and LED-based solar simulators available on the market are presently not capable of perfectly emulating the Sun’s spectrum; this limitation may alter the degradation process in a laboratory setting, as solar UV radiation plays a capital role in photodegradation (Leary, 2016). Moreover, factors such as a more realistic dynamic set-up versus a classic static one when performing biodegradation tests can change quite drastically the results obtained (Thellen et al., 2008). Although laboratory tests maintain their informational value within a comprehensive assessment strategy, field tests are of utter importance. However, the latter type of experiment does not come without disadvantages: they are liable to tampering by macrofauna and humans alike, difficult to check upon, and biofouling can disrupt the exposure of the plastic samples (Lott et al., 2020). A balanced solution could be found in hybrid test systems, allowing exposure to natural sunlight, and incorporating stirring/movement, with natural or inoculated seawater - but contained vessels to avoid tampering, and easier accessibility to the experiment, for control purposes.
This experiment aimed to compare the degradation and the chemical and physical surface changes of films made by two types of biodegradable polymer, polylactic acid (PLA) and polyhydroxyalkanoates (PHA), to those of films made by a conventional polymer, low-density polyethylene (LDPE) after a six-month exposure to a simulated marine environment with natural sunlight. We visually inspected the films to determine the percentage of surface degraded after exposure; furthermore, we investigated topographical and chemical surface changes to assess hydrolysis of the polymers. PHA and PLA were chosen as biodegradable polymers due to their ubiquitous presence on the market, especially in packaging applications (M Kolybaba et al., 2003; Xu and Guo, 2010; Jambunathan and Zhang, 2016; Sangroniz et al., 2019). Conversely, LDPE was selected because it is one of the most employed polymers in packaging (PlasticsEurope, 2020) and it is not degradable in marine environments, acting as negative control.
Materials and Methods
Experimental Setup
The experimental setup was placed on the roof of an eight-story building, in order to achieve maximal solar irradiation, uninterrupted by obstacles casting shadows, throughout the day–similar to the conditions found on the average sea surface. The setup consisted of an aluminum table, where the table surface was a grid to allow air circulation, and an overlying structure which could be opened by a lid, to check upon the experimental vessels (see Figure 1). The structure was lined with UV-transparent greenhouse foil stretched on the frames, to protect the experiment inside from external tampering (such as strong winds, or birds). Inside the structure, 13 glass tanks were placed, and filled with 9 L each of artificial seawater, made of milli-Q water and hw Marinemix® professional salts (Wiegandt GmbH, Krefeld, Germany) at a salinity of 35 PSU. A plastic film square was placed in 12 of the containers, aside for one tank, which was left empty and acted as control.
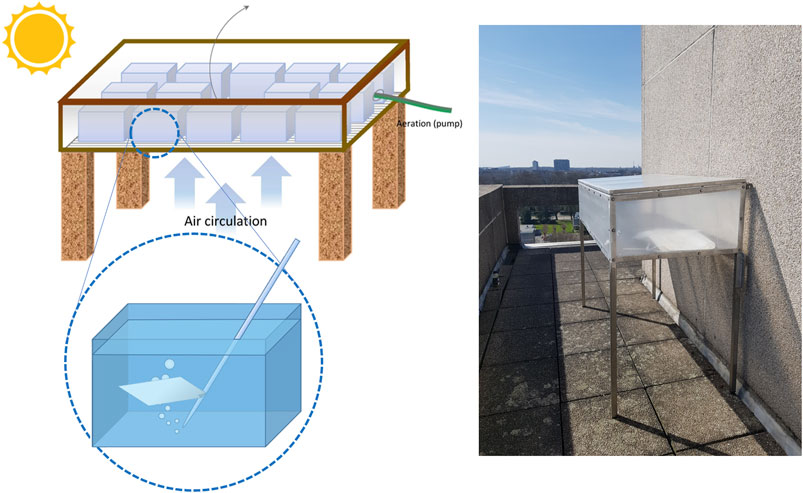
FIGURE 1. Experimental setup. On the top left, diagram of the greenhouse-table; the top and sides are made of UV-transparent foil, and the lid opens upwards as shown by the arrow. On the bottom left, detailed diagram of an experimental vessel with the glass pipette. On the right, picture of the setup after installation on the top of the roof, before the experimental vessels were placed inside the table.
The 12 plastic film squares were 150 × 150 mm in area and had a thickness of 0.05 mm; four were made of low-density polyethylene (LDPE), four of a polyhydroxyalkanoate (PHA) blend (polyhydroxybutyrate/polyhydroxyvalerate 8% (PHB-co-PHV)), and finally, four of poly-l-lactic acid (PLA); all films were acquired from Goodfellow GmbH (Friedberg, Germany). The PHA and PLA films are defined by the producer as “biodegradable”: specifically, the PHB-co-PHV blend was reported as being degradable in conditions such as incubation in compost for 80 days at 58°C–achievable in an industrial composter but not under typical use of injection molded or extruded articles. The biodegradation rate of PLA was indicated to be dependent on ambient temperature: this rate can vary between years, in soil (average temperature between 8–15°C), to weeks, in industrial composting facilities (50–65°C). This data is echoed by findings reported in literature: PHB-co-PHV was found to degrade almost completely after 39 days in a pilot-scale composter, at temperatures ranging from 40 to 70°C (Weng et al., 2010). In a different study, PHB-co-PHV samples were fully mineralized after 70–90 days at 58°C in an industrial composter setting. PLA has been reported to completely degrade in a time span of three to six weeks (depending on polymer crystallinity and object size) in a composting environment (Madhavan Nampoothiri et al., 2010). In an aquatic degradation test, up to 90% of PLA mineralized after 120 days at 60°C; the mineralization process was faster in anaerobic conditions, reaching 60% after 40 days at 52°C (Itävaara et al., 2002).
The experiment ran continuously for six months, from april to October 2018. To provide aeration and stirring to the vessels, air was delivered through glass pipettes connected to a pump and placed in each tank, including the control. In order to account for water loss from the experimental vessels due to evaporation, ultrapure water was added periodically; salinity was checked daily by using a handheld refractometer and maintained at 35 PSU throughout the experiment.
Weather and Irradiance Data
Weather data, particularly over air temperature (°C), and average irradiance (W m−2), was collected by a weather station located at N 51° 9′ 24.368″ E 4° 26′ 37.908″, within the Mesodrome facilities of the University of Antwerp, Belgium. The experimental site is located at a distance of 2.52 km from the weather station; therefore, the data collected are reasonably applicable to the experimental site.
Degradation, Chemical, and Surface Analysis
After exposure, the films were taken out from the experimental vessels, rinsed with ultrapure water, and left to air dry in a laminar flow cabinet for two days, taking care to avoid prolonged exposure to both artificial and natural light. The films were weighed and subsequently stored in between two glass plates in a cool, dry place. Degradation analysis was performed with a stereo microscope (Leica S8 APO) connected to a Leica MC190 HD camera (Leica Microsystems Belgium, Machelen, Belgium). The images were processed using the public domain ImageJ software. Subsamples (n = 10) of an approximate area of 1 mm2 were cut out, using a scalpel, in random points along an imaginary diagonal crossing each film. They were imaged through scanning electron microscopy to analyze their surface on a microscopic scale, using a FEI Quanta 250 FEG, in environmental (ESEM) mode, operating under high vacuum and with an electron beam of 5 kV. Furthermore, films spectra were obtained with a Fourier-Transform Infrared (FT-IR) spectrometer (LUMOS II, Bruker, Kontich, Belgium) in transmission mode.
Results
Weather Data
Over the six-months experimental period, the data collected by the weather station showed that the average air temperature was 17.7°C, and the average irradiance 193 W m−2. The highest temperature recorded, 37.8°C, occurred on the July 26, 2018; whilst the highest irradiance, 1043 W m−2, was registered on the June 3, 2018.
Degradation
The films were imaged under a stereomicroscope to assess surface loss and thus degradation. None of the three types of films showed significant surface loss (higher than 1%). Two out of the four PHA films had holes, of a combined area of 18 mm2 for film 1 (0.08% of total surface, Figure 2) and 0.141 mm2 for film 2 (0.006% of total surface, Figure 2); all PHA films presented cracks, some of them longer than 10 mm. No surface loss was observed on LDPE and PLA films, but the latter type of film consistently showed cracks, such as the 6 mm one shown in Figure 2. No significant change in thickness was observed between reference and exposed films; on the contrary, a slight (less than 1% of the original weight, data not shown) weight difference was recorded, explained by the presence of biofouling on the exposed films.
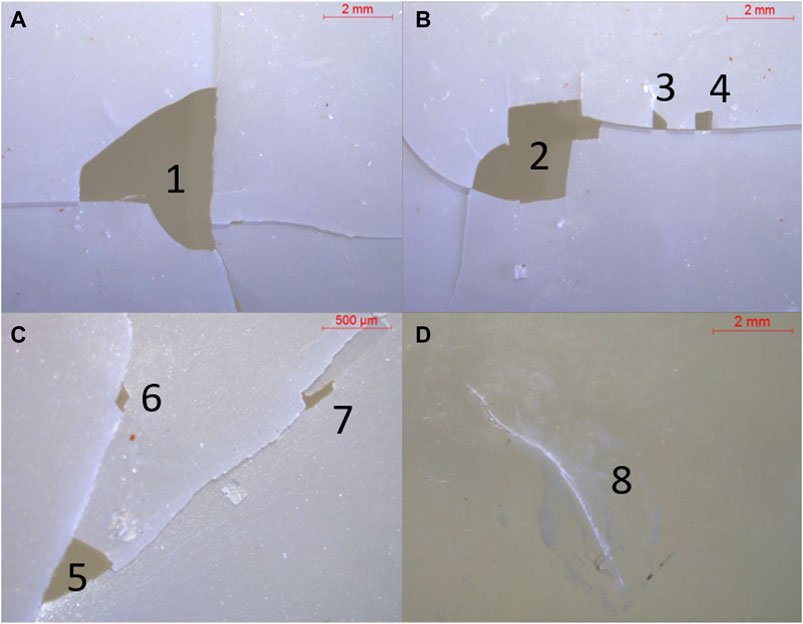
FIGURE 2. Stereo microscope images of surface loss and changes in PHA (A-C) and PLA (D) films after exposure (hole sizes in mm2: 1=11.011, 2=6.703, 3=0.130, 4=0.230, 5=0.115, 6=0.008, 7=0.018).
Chemical Analysis
FT-IR spectra were obtained for referenced and exposed films; whilst no significant difference was observed between the spectra of reference and exposed PLA and PHA films, all exposed LDPE films showed sharp peaks located in the area around 1,650 cm−1, and a broad peak in the area around 3,300 cm−1, which were not present in the reference ones (Figure 3).
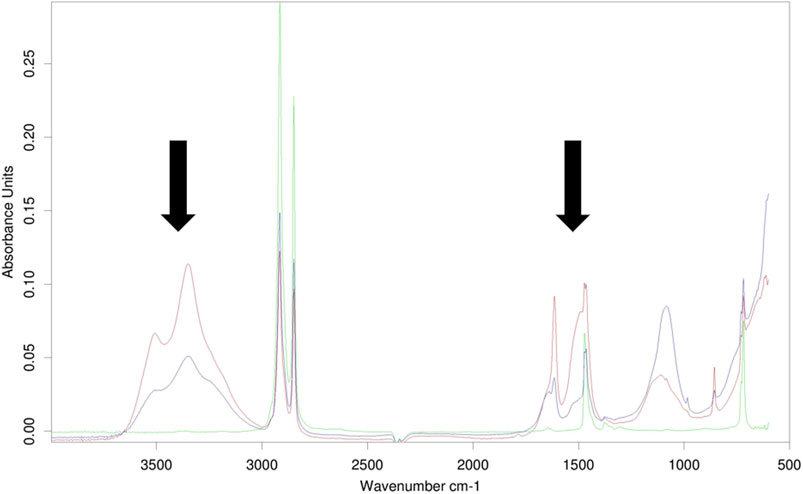
FIGURE 3. FT-IR spectral comparison of reference and exposed LDPE films. The green line represents the reference spectrum, while the red and black lines depict the spectra of LDPE films after exposure. Peaks around 1,650 cm−1 and 3,300 cm−1 are highlighted by arrows.
Surface Analysis
Comparison of pictures taken of reference and exposed films through the E-SEM microscope showed different effects of the six-month exposure on the three types of plastic tested in this study. As for LDPE films, on the reference ones the relatively smooth texture was interrupted by artifacts appearing as tracks, probably left during film production (Figure 4A, red arrow); and small production faults (Figure 4B, blue arrow). After a six-month exposure, the tracks were deepened (Figure 4C), and the surface presented remarkable indentations (Figure 4D). What was visible by visual inspection, a consistent biofouling by green algae of the LDPE films after exposure, was confirmed by E-SEM imaging: thick, multi-layered “mats” of filamentous algae were present in seven out of 10 of the subsamples analyzed, often associated with groups of oval-shaped microorganisms, each of them around 1 µm in length (Figures 4E,F).
Reference PLA films showed the same tracks left during production (Figures 5A,B); interestingly, after exposure these tracks did not deepen, unlike in the LDPE films (Figure 5C). The surface texture weathered to what could be described as a puckered, “orange-peel” appearance (Figures 5D,E), and deep, round surface indentations characterized a majority of the subsamples for this film type (Figure 5E). Biofouling was present in four out of the ten subsamples, and the algal filaments did not create multi-layered “mats” such as those observed on LDPE films (Figure 5F).
In contrast with the other film types considered in this study, reference PHA films presented a completely smooth surface, devoid of tracks and seemingly of other production artifacts - to the point that imaging the surface was challenging due to the lack of topography landmarks (Figures 6A,B). After exposure, these films developed a coarse, brittle surface, different from the puckering imaged on PLA films (Figure 6C). The surface was also characterized by deep, isolated cuts, which created a loss of material (Figures 6D,E). Biofouling was registered on three out of the 10 subsamples, and, as in the case of PLA films, was not thick and multi-layered, consisting often of isolated patches (Figure 6F).
Discussion
In this study, we assessed degradation, surface structure and chemical properties of LDPE, PLA, and PHA films, after a six-month exposure to a simulated seawater environment. No significant degradation, defined as surface loss, was observed in any of the films tested, including the biodegradable polymer ones. Interestingly, the chemical and physical structure of the films surface after weathering, as well as the rate of biofouling, were different for each of the polymers tested.
Polymer degradation is influenced by the temperature of the surrounding aqueous medium, and, when considering field conditions, by the irradiance of the location where the degradation is undergoing. Laboratory studies conducted in accordance with standardized test protocols such as the American Society for Testing Materials (ASTM) D6691 rely on a temperature of 30°C for the test medium (ASTM D6691-17, 2017), accelerating, or, in some case, reaching complete degradation within the experimental timeframe: Mayer (1990) reported that PHA films lost 48% of their original weight after a 12 weeks exposure to seawater at 30°C; a study commissioned by the California Department of Resources Recycling and Recovery (CalRecycle) described how films made of the proprietary PHAs Mirel 4100 and Mirel 2200 underwent a 45 and 38% degradation respectively (measured as carbon-to-CO2 conversion) after a six-month exposure to seawater at 30°C (California State University, Greene, J., and Chico Research Foundation, 2012). The temperature conditions employed in such laboratory tests are highly unlikely to be found in natural aquatic environments (Haider et al., 2019; Chamas et al., 2020), particularly for a six-month period–therefore, products made with commercial polymers which obtained marine biodegradability certifications (based on standards such as the ASTM D6691) may end up not fully degrading in natural seawater conditions. On the other end, among field studies conducted in the Tropics, significant degradation of exposed biodegradable plastic is frequently observed - due obviously to a higher average sea surface temperature and daily irradiance than that of temperate areas. Imam et al. (1999) described a 0.1% average weight loss per day of PHA films submerged in the coastal sea of Puerto Rico, where the average air temperature is 25.6°C; similarly, Boyandin et al. (2012) exposed PHA films to the South China Sea in Vietnam and measured a 46% loss in mass after 160 days–the average sea temperature during the experimental period was 28.75°C (Volova et al., 2011), close to the temperature employed during standardized laboratory testing. In the aforementioned CalRecycle report, a successive experiment was performed, exposing PHA and PLA bottles for 12 months to seawater ranging from 21 to 25°C, representative of the temperatures of Half Moon Bay (San Francisco, United States): no discernible degradation was observed after the exposure, even after an experimental duration double than that of the 30°C test, highlighting the effect of temperature over degradation of biodegradable polymers in seawater. Bagheri et al. (2017) executed a laboratory experiment exposing PLA and PHA films to seawater, under fluorescent light, at 25°C, for a period of 12 months: PLA films showed no significant degradation, and PHA films lost a mere 8.5% of their initial mass. One recently published work compared LDPE and PHA (Mirel P5001) film degradation after exposure to a temperate versus a tropical marine environment: films submerged for 22 months in a pelagic setting in the Mediterranean Sea (Elba Island, Italy) showed no surface loss, while films placed for the same amount of time in the Indonesian sea experienced a ∼90% surface loss (Lott et al., 2020). Overall, these studies seem to demonstrate a proportional relationship between degradation rate and temperature and irradiance. Whilst the average temperatures in the tropical regions range between 25–28°C, the average air temperature during the experimental period of our study was 17.7°C. The average irradiance recorded during this study was 193 W m−2; as an example, the average yearly irradiance in Malaysia is ∼1744 W m−2 (Shavalipour et al., 2013), nearly 700 W m−2 more than the highest irradiance we reported, 1043 W m−2. Nonetheless, within literature there are examples of studies conducted in temperate regions which showed consistent biodegradable polymer degradation after seawater exposure: Mergaert et al. (1995) placed PHA films in the waters at Zeebrugge, Belgium for nine months, and observed a mass loss between 49 and 52%. More recently, Rutkowska et al. (2008) described a mass loss of 60% in PHA films submerged in the Baltic Sea for one and a half months. These findings hint towards other factors being involved in kickstarting and maintaining the degradation process, such as polymer properties.
Although both biodegradable polymers, PHA and PLA possess very different properties, which in turn influence the degradation success in seawater. Min and co-authors introduced a model predicting that glass transition temperature Tg to be inversely proportional to degradation rate; hence, polymers owning a Tg lower than the ocean temperature tend to degrade faster (Min et al., 2020). As for the biodegradable polymers considered in this work, PHA possess a Tg < ocean temperature, between -4.6 and 30°C (Thellen et al., 2008; Wang et al., 2021), whereas PLA has a Tg ranging between 44 and 60°C (Becker et al., 2010; Min et al., 2020), well above the ocean temperature: consequently, the degradation of PHA will occur at a faster rate than that of PLA in the same exposure conditions. Additionally, polymers which absorb a very low quantity of water (such as PLA) can experience changes in Tg after a prolonged presence in an aquatic environment (Auras et al., 2004). An exception to the Min and co-authors’ model is PE, which, albeit owning a very low Tg (-110°C), as other polyolefins is scarcely degradable (Min et al., 2020). Conversely, a higher crystallinity implies less availability of functional groups within the polymer, and thus slower hydrolysis processes (Chamas et al., 2020). At temperatures above 30°C, in aqueous media, PLA, a semi-crystalline polymer, experiences hydrolysis of the ester linkages in the amorphous regions, thus exposing carboxylic acid ends, which, by lowering the pH, self-catalyze the complete hydrolysis of the polymer. High temperatures close to Tg are extremely improbable to occur in the marine environment; the main mechanisms of degradation become photo-oxidation and enzymatic hydrolysis (Martin et al., 2014; Chamas et al., 2020; Kliem et al., 2020; Wang et al., 2021). In fact, to increase biodegradability of PLA in seawater, Martin et al. created a PLA derivative polymer, modifying its chain targeting the acetal functional group. Various authors have described the lack of degradation of PLA after long-term seawater exposures in natural conditions (Tsuji and Suzuyoshi, 2002; Deroiné et al., 2014; Bagheri et al., 2017; Haider et al., 2019; Gexia et al., 2020). Similarly, in our study no discernible surface loss was detected; FT-IR analysis of the reference and exposed PLA films spectra showed no difference in peaks, demonstrating no chemical surface change. This finding is consistent with the results of a study by Nazareth et al. (2019), where the spectra of PLA carrier bags exposed to natural seawater for six months did not significantly differ from the reference spectrum. On a topographic level, the “orange peel” pattern witnessed in this work on the surface of exposed PLA films may represent an initial stage of hydrolysis. This pattern recalls the surface changes imaged by Rodriguez et al. (2016): PLA films incubated in neutral aqueous medium at 70°C present characteristics reminiscent of those observed in our study, although the pores we imaged were less deep–a consequence of the extreme weathering conditions (pH, temperature) employed in the study. Wang and co-authors (2021) published a micrograph of a PLA film surface after 13 months exposure in seawater, which depicts a rounded indentation not unlike those seen during our analysis (compare with Figure 5D).
In our study, FT-IR characterization of PHA films spectra after seawater exposure showed no difference with the reference spectrum, in contrast with Briassoulis et al. (2019), who, after six months of exposure in natural seawater of PHA films, detected a broad peak around 3,300 cm−1. This phenomenon was interpreted by the authors as proof of the presence of OH− groups, created by enzymatic hydrolysis of the polymer. SEM imaging of the exposed PHA films depicted a coarse, brittle surface, a finding compatible with other works in literature (Woolnough et al., 2010; Volova et al., 2011; Deroiné et al., 2014, 2015; Bagheri et al., 2017), although there the brittleness is more pronounced and leads to deeper cracks. Overall, the surface structure changes of exposed PLA and PHA films that we detected are consistent with previous works and may thus point to an initial development of enzymatic hydrolysis catalyzed by the microbial community spontaneously developed in the test vessels by aerial deposition. In further experiments, it will be fundamental to characterize the microbiota, and add to the seawater an inoculum supplemented with appropriate medium for growth (as in i.e. Lott et al., 2020), to reach conditions overlapping with those of real seawater surface.
LDPE films were chosen as negative control, and not expected to degrade, as extensively reported in literature (see e.g. Roy et al., 2011). However, FT-IR analysis of the exposed LDPE films showed definite peaks when compared with the reference spectrum: a broad peak at ∼3,350 cm−1, consistent with OH− functional groups, and two narrow peaks at 1,600 and 1,100 cm−1, which could indicate the presence of biologically related amides. Interestingly, these types of peaks were observed in other studies, such as Briassoulis et al. (2019), for biodegradable plastic spectra, and interpreted as evidence of biofouling-induced chemical surface changes. The micrographs of exposed LDPE films taken in our study depict a topographical change in the surface, which appears more indented than the reference; these type of films presented the highest degree of biofouling compared to PHA and PLA, observed both on a micro- and macroscopic scale. These phenomena suggest that LDPE films in our study after six month of seawater exposure were colonized by microbiota at a higher degree than biodegradable films, which in turn caused consistent physical and chemical surface changes. This increased fouling on LDPE films may have been prompted by increased surface brittleness and free carbonyl group ends, perhaps due to photodegradation (Albertsson et al., 1987; Orr Gilan et al., 2004).
This work demonstrated that PHA and PLA films, although marketed as biodegradable plastics, do not significantly degrade after six months in a temperate marine environment. The PHA degradation reported in previous temperate seas studies was probably not observed to such a great extent after our analysis due to experimental limitations - the lack of microbial inoculum/growth medium. Furthermore, in future trials, increased degradation times (12–24 months) should be explored, to uncover potential longer-term effects of exposure.
PHA items have potential to degrade in seawater, albeit on increased timescales when compared with soil or industrial composter degradation. Nonetheless, producing PHA for widespread applications is challenging, both because of its increased production cost in respect to PLA and conventional polyesters, and for the brittleness of the unblended polymer (Jambunathan and Zhang, 2016). These factors have prioritized PLA as a competitor in the biodegradable plastics race (Philp et al., 2013). Hence, the majority of marine biodegradable plastic litter will be constituted by PLA, which is not degradable in the marine environment. Unknowing consumers may contribute to this issue by selecting PLA products over other polymers, in the wrong belief of adopting an environmentally friendly behavior. Even to a careful consumer, the lack of international certifications defining precise biodegradability requirements make labels and product claims difficult to interpret–leaving space for greenwashing (Viera et al., 2020). There is undeniable confusion within end-users on biodegradable polymer waste disposal, and not all countries/regions are equipped with appropriate infrastructure (Zhu and Wang, 2020).
These arguments render the adoption of biodegradable polymers as a substitute for conventional ones in single-use applications potentially harmful, if not accompanied by strict international standards for biodegradability, adequate waste stream management, and consumer education on proper disposal. Biodegradable polymers have the potential to act as a steppingstone towards solving the plastic litter problem; nevertheless, embracing this new technology mindlessly will plainly create a new class of persistent pollutants in the marine environment–similarly to its conventional counterpart.
Data Availability Statement
The raw data supporting the conclusions of this article will be made available by the authors, without undue reservation.
Author Contributions
CCC conceived, designed, and carried out the experiment and subsequent analysis. CCC wrote the first manuscript draft. RB revised the manuscript. All authors contributed to manuscript revision, read, and approved the submitted version.
Funding
This study was funded by the Research Foundation–Flanders (FWO) Strategic Research (SB) doctoral grant number 1S15417N, awarded to CCC.
Conflict of Interest
The authors declare that the research was conducted in the absence of any commercial or financial relationships that could be construed as a potential conflict of interest.
Publisher’s Note
All claims expressed in this article are solely those of the authors and do not necessarily represent those of their affiliated organizations, or those of the publisher, the editors and the reviewers. Any product that may be evaluated in this article, or claim that may be made by its manufacturer, is not guaranteed or endorsed by the publisher.
Acknowledgments
The authors would like to thank Gilles Van Loon and his team for constructing the experimental setup; Tine Derez for her invaluable help with ESEM imaging; Freddy Dardenne for providing the weather data; and finally Ali Pilehvar, Giovanni Castaldo, and Elvio D. Amato for their assistance with experiment maintenance.
References
Abalansa, S., El Mahrad, B., Vondolia, G. K., Icely, J., and Newton, A. (2020). The Marine Plastic Litter Issue: A Social-Economic Analysis. Sustainability 12, 8677. doi:10.3390/su12208677
Accinelli, C., Saccà, M. L., Mencarelli, M., and Vicari, A. (2012). Deterioration of Bioplastic Carrier Bags in the Environment and Assessment of a New Recycling Alternative. Chemosphere 89, 136–143. doi:10.1016/j.chemosphere.2012.05.028
Adyel, T. M. (2020). Accumulation of Plastic Waste during COVID-19. Science 369, 1314–1315. doi:10.1126/science.abd9925
Albertsson, A. C., Andersson, S. O., and Karlsson, S. (1987). The Mechanism of Biodegradation of Polyethylene. Polym. Degrad. Stab. 18, 73–87. doi:10.1016/0141-3910(87)90084-X
ASTM D6691-17 (2017). Test Method for Determining Aerobic Biodegradation of Plastic Materials in the Marine Environment by a Defined Microbial Consortium or Natural Sea Water Inoculum. West Conshohocken: ASTM International. doi:10.1520/D6691-17
ASTM D7473-12 (2021). Test Method for Weight Attrition of Plastic Materials in the Marine Environment by Open System Aquarium Incubations. West Conshohocken: ASTM International. doi:10.1520/D7473-12
Auras, R., Harte, B., and Selke, S. (2004). An Overview of Polylactides as Packaging Materials. Macromol. Biosci. 4, 835–864. doi:10.1002/mabi.200400043
Bagheri, A. R., Laforsch, C., Greiner, A., and Agarwal, S. (2017). Fate of So-Called Biodegradable Polymers in Seawater and Freshwater. Glob. Challenges. 1, 1700048. doi:10.1002/gch2.201700048
Becker, J. M., Pounder, R. J., and Dove, A. P. (2010). Synthesis of Poly(lactide)s with Modified Thermal and Mechanical Properties. Macromol. Rapid Commun. 31, 1923–1937. doi:10.1002/marc.201000088
Boyandin, A. N., Rudnev, V. P., Ivonin, V. N., Prudnikova, S. V., Korobikhina, K. I., Filipenko, M. L., et al. (2012). Biodegradation of Polyhydroxyalkanoate Films in Natural Environments. Macromol. Symp. 320, 38–42. doi:10.1002/masy.201251004
Briassoulis, D., Pikasi, A., Briassoulis, C., and Mistriotis, A. (2019). Disintegration Behaviour of Bio-Based Plastics in Coastal Zone marine Environments: A Field experiment under Natural Conditions. Sci. Total Environ. 688, 208–223. doi:10.1016/j.scitotenv.2019.06.129
California State University, Greene, J., and Chico Research Foundation (2012). PLA and PHA Biodegradation in the Marine Environment. Sacramento, CA: Department of Resources Recycling and Recovery.
Chamas, A., Moon, H., Zheng, J., Qiu, Y., Tabassum, T., Jang, J. H., et al. (2020). Degradation Rates of Plastics in the Environment. ACS Sustainable Chem. Eng. 8, 3494–3511. doi:10.1021/acssuschemeng.9b06635
Chen, Y., Awasthi, A. K., Wei, F., Tan, Q., and Li, J. (2021). Single-use Plastics: Production, Usage, Disposal, and Adverse Impacts. Sci. Total Environ. 752, 141772. doi:10.1016/j.scitotenv.2020.141772
Delmas, M. A., and Burbano, V. C. (2011). The Drivers of Greenwashing. Calif. Management Rev. 54, 64–87. doi:10.1525/cmr.2011.54.1.64
Deroiné, M., César, G., Le Duigou, A., Davies, P., and Bruzaud, S. (2015). Natural Degradation and Biodegradation of Poly(3-Hydroxybutyrate-Co-3-Hydroxyvalerate) in Liquid and Solid Marine Environments. J. Polym. Environ. 23, 493–505. doi:10.1007/s10924-015-0736-5
Deroiné, M., Le Duigou, A., Corre, Y. M., Le Gac, P. Y., Davies, P., César, G., et al. (2014). Accelerated Ageing of Polylactide in Aqueous Environments: Comparative Study between Distilled Water and Seawater. Polym. Degrad. Stab. 108, 319–329. doi:10.1016/j.polymdegradstab.2014.01.020
Derraik, J. G. B. (2002). The Pollution of the marine Environment by Plastic Debris: a Review. Mar. Pollut. Bull. 44, 842–852. doi:10.1016/S0025-326X(02)00220-5
European Bioplastics (2015). “OXO-BIODEGRADABLE” PLASTICS AND OTHER PLASTICS WITH ADDITIVES FOR DEGRADATION. Berlin, Germany: European Bioplastics e.V. Available at: https://docs.european-bioplastics.org/2016/publications/bp/EUBP_bp_additive-mediated_plastics.pdf.
Folino, A., Karageorgiou, A., Calabrò, P. S., and Komilis, D. (2020). Biodegradation of Wasted Bioplastics in Natural and Industrial Environments: A Review. Sustainability 12, 6030. doi:10.3390/su12156030
Galloway, T. S., and Lewis, C. N. (2016). Marine Microplastics Spell Big Problems for Future Generations. Proc. Natl. Acad. Sci. USA. 113, 2331–2333. doi:10.1073/pnas.1600715113
Gexia, W., Dan, H., Wei, Z., and Junhui, J. I. (2020). Degradation Performance of Typical Biodegradable Polyesters in Seawater. 功能高分子学报 33, 492–499. doi:10.14133/j.cnki.1008-9357.20191015001
Geyer, R., Jambeck, J. R., and Law, K. L. (2017). Production, Use, and Fate of All Plastics Ever Made. Sci. Adv. 3, e1700782. doi:10.1126/sciadv.1700782
Haider, T. P., Völker, C., Kramm, J., Landfester, K., and Wurm, F. R. (2019). Plastics of the Future? the Impact of Biodegradable Polymers on the Environment and on Society. Angew. Chem. Int. Ed. 58, 50–62. doi:10.1002/anie.201805766
Harrison, J. P., Boardman, C., O'Callaghan, K., Delort, A. M., and Song, J. (2018). Biodegradability Standards for Carrier Bags and Plastic Films in Aquatic Environments: a Critical Review. R. Soc. Open Sci. 5, 171792. doi:10.1098/rsos.171792
Heidbreder, L. M., Bablok, I., Drews, S., and Menzel, C. (2019). Tackling the Plastic Problem: A Review on Perceptions, Behaviors, and Interventions. Sci. Total Environ. 668, 1077–1093. doi:10.1016/j.scitotenv.2019.02.437
Imam, S. H., Gordon, S. H., Shogren, R. L., Tosteson, T. R., Govind, N. S., and Greene, R. V. (1999). Degradation of Starch-Poly(β-Hydroxybutyrate-Co-β-Hydroxyvalerate) Bioplastic in Tropical Coastal Waters. Appl. Environ. Microbiol. 65, 431–437. doi:10.1128/AEM.65.2.431-437.1999
Jambunathan, P., and Zhang, K. (2016). Engineered Biosynthesis of Biodegradable Polymers. J. Ind. Microbiol. Biotechnol. 43, 1037–1058. doi:10.1007/s10295-016-1785-z
Kershaw and United Nations Environment Programme (2015). Biodegradable Plastics & marine Litter Misconceptions, Concerns and Impacts on marine Environments. Nairobi: UNEPAvailable at: http://unep.org/gpa/documents/publications/BiodegradablePlastics.pdf (Accessed August 1, 2016).
Kliem, S., Kreutzbruck, M., and Bonten, C. (2020). Review on the Biological Degradation of Polymers in Various Environments. Materials 13, 4586. doi:10.3390/ma13204586
Kolybaba, M., Tabil, L. G., Panigrahi, S., Crerar, W. J., Powell, T., and Wang, B. (2003). Biodegradable Polymers: Past, Present, and Future. ASABE/CSBE North Central Intersectional Meeting, Fargo, North Dakota, October 3–4, 2003. American Society of Agricultural and Biological Engineers. doi:10.13031/2013.41300
Leary, G. P. (2016). Comparison of Xenon Lamp-Based and LED-Based Solar Simulators. Bozeman, MT: Montana State University, 1–47. Available at: https://scholarworks.montana.edu/xmlui/handle/1/9837 (Accessed January 21, 2021). doi:10.1109/pvsc.2016.7750227
Lebreton, L. C. M., van der Zwet, J., Damsteeg, J. W., Slat, B., Andrady, A., and Reisser, J. (2017). River Plastic Emissions to the World's Oceans. Nat. Commun. 8, 15611. doi:10.1038/ncomms15611
Lott, C., Eich, A., Unger, B., Makarow, D., Battagliarin, G., Schlegel, K., et al. (2020). Field and Mesocosm Methods to Test Biodegradable Plastic Film under marine Conditions. Plos One 15, e0236579. doi:10.1371/journal.pone.0236579
Luyt, A. S., and Malik, S. S. (2019). “Can Biodegradable Plastics Solve Plastic Solid Waste Accumulation?,” in Plastics To Energy Plastics Design Library. Editor S. M. Al-Salem (Norwich, NY: William Andrew Publishing), 403–423. doi:10.1016/B978-0-12-813140-4.00016-9
Martin, R. T., Camargo, L. P., and Miller, S. A. (2014). Marine-degradable Polylactic Acid. Green. Chem. 16, 1768–1773. doi:10.1039/C3GC42604A
Mayer, J. M. (1990). Degradation Kinetics of Polymer Films in Marine and Soil Systems under Accelerated Conditions. in Novel Biodegradable Microbial Polymers NATO ASI Series. Editor E. A. Dawes (Dordrecht: Springer Netherlands), 469–471. doi:10.1007/978-94-009-2129-0_49
Mergaert, J., Wouters, A., Swings, J., and Anderson, C. (1995). In Situ biodegradation of Poly(3-Hydroxybutyrate) and Poly(3-Hydroxybutyrate-Co-3-Hydroxyvalerate) in Natural Waters. Can. J. Microbiol. 41 (Suppl. 1), 154–159. doi:10.1139/m95-182
Min, K., Cuiffi, J. D., and Mathers, R. T. (2020). Ranking Environmental Degradation Trends of Plastic marine Debris Based on Physical Properties and Molecular Structure. Nat. Commun. 11, 1–11. doi:10.1038/s41467-020-14538-z
Nazareth, M., Marques, M. R. C., Leite, M. C. A., and Castro, Í. B. (2019). Commercial Plastics Claiming Biodegradable Status: Is This Also Accurate for marine Environments?. J. Hazard. Mater. 366, 714–722. doi:10.1016/j.jhazmat.2018.12.052
Oliveira, J., Belchior, A., da Silva, V. D., Rotter, A., Petrovski, Ž., Almeida, P. L., et al. (2020). Marine Environmental Plastic Pollution: Mitigation by Microorganism Degradation and Recycling Valorization. Front. Mar. Sci. 7. doi:10.3389/fmars.2020.567126
Orr Gilan, I., Hadar, Y., and Sivan, A. (2004). Colonization, Biofilm Formation and Biodegradation of Polyethylene by a Strain of Rhodococcus Ruber. Appl. Microbiol. Biotechnol. 65, 97–104. doi:10.1007/s00253-004-1584-8
Philp, J. C., Bartsev, A., Ritchie, R. J., Baucher, M.-A., and Guy, K. (2013). Bioplastics Science from a Policy Vantage point. New Biotechnol. 30, 635–646. doi:10.1016/j.nbt.2012.11.021
PlasticsEurope, (2020). Plastics - the Facts 2020. Available at: https://www.plasticseurope.org/en/resources/publications/4312-plastics-facts-2020 (Accessed January 21, 2021).
Rhodes, C. J. (2019). Solving the Plastic Problem: From Cradle to Grave, to Reincarnation. Sci. Prog. 102, 218–248. doi:10.1177/0036850419867204
Rodriguez, E. J., Marcos, B., and Huneault, M. A. (2016). Hydrolysis of Polylactide in Aqueous media. J. Appl. Polym. Sci. 133. doi:10.1002/app.44152
Roy, P. K., Hakkarainen, M., Varma, I. K., and Albertsson, A. C. (2011). Degradable Polyethylene: Fantasy or Reality. Environ. Sci. Technol. 45, 4217–4227. doi:10.1021/es104042f
Rutkowska, M., Krasowska, K., Heimowska, A., Adamus, G., Sobota, M., Musioł, M., et al. (2008). Environmental Degradation of Blends of Atactic Poly[(R,S)-3-hydroxybutyrate] with Natural PHBV in Baltic Sea Water and Compost with Activated Sludge. J. Polym. Environ. 16, 183–191. doi:10.1007/s10924-008-0100-0
Sangroniz, A., Zhu, J. B., Tang, X., Etxeberria, A., Chen, E. Y. X., and Sardon, H. (2019). Packaging Materials with Desired Mechanical and Barrier Properties and Full Chemical Recyclability. Nat. Commun. 10, 1–7. doi:10.1038/s41467-019-11525-x
Schmidt, C., Krauth, T., and Wagner, S. (2017). Export of Plastic Debris by Rivers into the Sea. Environ. Sci. Technol. 51, 12246–12253. doi:10.1021/acs.est.7b02368
Shavalipour, A., Hakemzadeh, M. H., Sopian, K., Mohamed Haris, S., and Zaidi, S. H. (2013). New Formulation for the Estimation of Monthly Average Daily Solar Irradiation for the Tropics: A Case Study of Peninsular Malaysia. Int. J. Photoenergy 2013, 1e174671–6. doi:10.1155/2013/174671
Thellen, C., Coyne, M., Froio, D., Auerbach, M., Wirsen, C., and Ratto, J. A. (2008). A Processing, Characterization and Marine Biodegradation Study of Melt-Extruded Polyhydroxyalkanoate (PHA) Films. J. Polym. Environ. 16, 1–11. doi:10.1007/s10924-008-0079-6
Thomas, N. L., Clarke, J., McLauchlin, A. R., and Patrick, S. G. (2012). Oxodegradable Plastics: Degradation, Environmental Impact and Recycling. Proc. Inst. Civil Eng. - Waste Resource Management 165, 133–140. doi:10.1680/warm.11.00014
Tsuji, H., and Suzuyoshi, K. (2002). Environmental Degradation of Biodegradable Polyesters 1. Poly(ε-Caprolactone), poly[(R)-3-hydroxybutyrate], and poly(L-Lactide) Films in Controlled Static Seawater. Polym. Degrad. Stab. 75, 347–355. doi:10.1016/S0141-3910(01)00240-3
United Nations Environment Programme, (2018). Single-use Plastics, a Roadmap for Sustainability. Available at: https://www.unenvironment.org/resources/report/single-use-plastics-roadmap-sustainability (Accessed January 21, 2021).
Viera, J. S. C., Marques, M. R. C., Nazareth, M. C., Jimenez, P. C., and Castro, Í. B. (2020). On Replacing Single-Use Plastic with So-Called Biodegradable Ones: The Case with Straws. Environ. Sci. Pol. 106, 177–181. doi:10.1016/j.envsci.2020.02.007
Volova, T. G., Boyandin, A. N., Vasil’ev, A. D., Karpov, V. A., Kozhevnikov, I. V., Prudnikova, S. V., et al. (2011). Biodegradation of Polyhydroxyalkanoates (PHAs) in the South China Sea and Identification of PHA-Degrading Bacteria. Microbiology 80, 252–260. doi:10.1134/S0026261711020184
Wang, G. X., Huang, D., Ji, J. H., Völker, C., and Wurm, F. R. (2021). Seawater‐Degradable Polymers-Fighting the Marine Plastic Pollution. Adv. Sci. 8, 2001121. doi:10.1002/advs.202001121
Woolnough, C. A., Yee, L. H., Charlton, T., and Foster, L. J. R. (2010). Environmental Degradation and Biofouling of ‘green' Plastics Including Short and Medium Chain Length Polyhydroxyalkanoates. Polym. Int. 59, 658–667. doi:10.1002/pi.2746
Xu, J., and Guo, B. H. (2010). “Microbial Succinic Acid, its Polymer Poly(butylene Succinate), and Applications,” in Plastics From Bacteria: Natural Functions And Applications Microbiology Monographs. Editor G. G. Q. Chen (Berlin, Heidelberg: Springer), 347–388. doi:10.1007/978-3-642-03287-5_14
Keywords: polyethylene, polyhydroxyalkanoates, poly l lactic acid, saltwater, biofouling, fragmentation
Citation: Catarci Carteny C and Blust R (2021) Not Only Diamonds Are Forever: Degradation of Plastic Films in a Simulated Marine Environment. Front. Environ. Sci. 9:662844. doi: 10.3389/fenvs.2021.662844
Received: 01 February 2021; Accepted: 02 July 2021;
Published: 02 August 2021.
Edited by:
Jastin Samuel, Lovely Professional University, IndiaReviewed by:
Mamtesh Singh, University of Delhi, IndiaSreejoyee Ghosh, University of Texas MD Anderson Cancer Center, United States
Copyright © 2021 Catarci Carteny and Blust. This is an open-access article distributed under the terms of the Creative Commons Attribution License (CC BY). The use, distribution or reproduction in other forums is permitted, provided the original author(s) and the copyright owner(s) are credited and that the original publication in this journal is cited, in accordance with accepted academic practice. No use, distribution or reproduction is permitted which does not comply with these terms.
*Correspondence: Camilla Catarci Carteny, camilla.catarcicarteny@uantwerpen.be