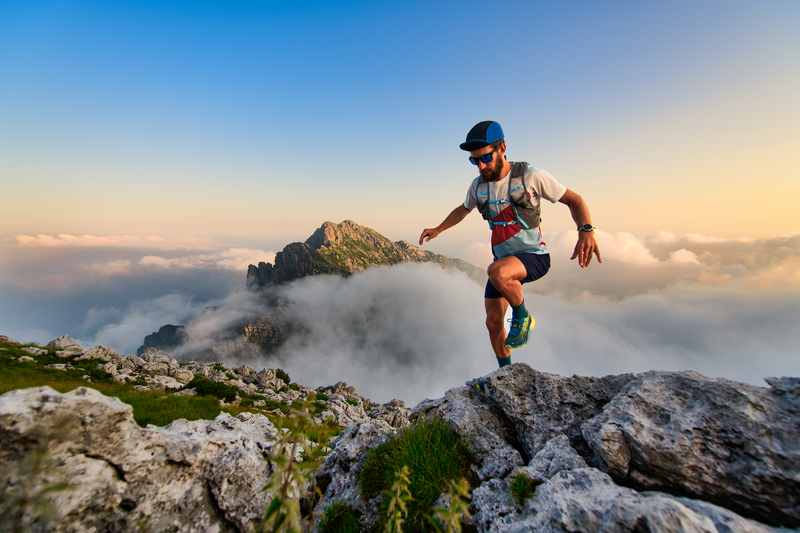
95% of researchers rate our articles as excellent or good
Learn more about the work of our research integrity team to safeguard the quality of each article we publish.
Find out more
ORIGINAL RESEARCH article
Front. Environ. Sci. , 05 July 2021
Sec. Biogeochemical Dynamics
Volume 9 - 2021 | https://doi.org/10.3389/fenvs.2021.660133
This article is part of the Research Topic Nitrogen in the Environment View all 9 articles
Paddy fields are major sources of atmospheric N2O. Soil temperature and moisture strongly affect N2O emissions from rice fields. However, N2O emissions from cold-waterlogged paddy fields (CW), an important kind of paddy soil in China, are not well studied so far. It is unclear whether the N2O emissions from cold-waterlogged paddy fields are the same as normal paddy fields (NW). We investigated the N2O emission characteristics from the CW and NW paddy fields under with (R1) and without (R0) rice in Tuku Village, Baisha Town, Yangxin County (YX site, monitoring in 2013) and Huandiqiao Town, Daye City (DY site, monitoring in 2014); compared the difference and influencing factors between the CW and NW paddy fields at two sites in South China. The results showed that the N2O emissions from NWR0 were 13.4 times higher than from CWR0, and from NWR1 were 10.3 times higher than from CWR1 in the YX site. The N2O emissions from NWR0 were 2.4 times higher than from CWR0, and from NWR1 were 17.3 times higher than from CWR1 in the DY site. The structural equation models (SEMs) showed that the N2O emissions are mainly driven by rice planting and soil moisture in the NW fields at the annual scale, while soil temperature in the CW fields. Overall, N2O emissions from cold waterlogged paddy fields are significantly lower than those of normal paddy fields due to the low temperature and higher water content; however, there are dinitrogen emissions from cold waterlogged paddy fields denitrification should be further examined.
Nitrous oxide (N2O) is the third-largest long-lived greenhouse gas following CO2 and CH4. The lifetime of N2O in the atmosphere is about 121 years, and its greenhouse effect is 265 times that of CO2 on a hundred-year scale (IPCC, 2014). Farmland ecosystems are the primary anthropogenic source of N2O emissions.
The nitrification and denitrification of the soil’s nitrogen cycle can lead to N2O emissions (Spott et al., 2011; Butterbach-Bahl et al., 2013; Hu et al., 2015a). Soil water change, soil aggregate fragmentation, organic matter degradation, and organic nitrogen mineralization regulate N2O emissions (Čuhel et al., 2010; Sheng et al., 2013; Wissing et al., 2013; Zhu et al., 2013; Wang et al., 2014; Weller et al., 2016).
Rice is a staple food and feeds nearly 50% of the global population (Alexandratos and Bruinsma, 2012). Paddy fields are an important source of N2O emissions, and 8–11% of China's agricultural N2O emissions were estimated from rice fields (Zou et al., 2009). A cold-waterlogged paddy field is a major type of low-yield paddy soil in China, accounting for 15.2% of the total paddy fields in this country (Xie et al., 2015). Its main characteristics are higher groundwater levels and lower soil temperature than normal paddy fields (Qiu et al., 2013; Liu et al., 2016). Those environments make strong anaerobic conditions, poor soil structure, high organic matter contents, and low rates of N mineralization (Xie et al., 2015). Those properties of CW fields result in significantly lower rice biomass yields and higher methane emissions than normal paddy fields (NW fields) (Xu et al., 2020).
Soil water content has a decisive influence on the process of nitrification and denitrification (Davidson and Verchot, 2000). Soil water-saturated areas or flooding conditions hinder gas diffusion and form an anaerobic soil environment (Zhu et al., 2013). Alternating wet and dry, the most common water management measures in normal rice fields, causes repeated nitrification and denitrification and results in a large amount of N2O production and emission Hofstra and Bouwman (2005), Hu et al. (2015b), Patrick and Wyatt (1964), Fierer and Schimel (2002), Gaihre et al. (2017), Islam et al. (2018), and N2O emissions from lowland rice fields showed significant spatial and seasonal variations from lowland rice fields (Gaihre et al., 2017). However, due to the high groundwater level, the effects of alternating dry and wet measures in cold-waterlogged paddy fields are far inferior to normal rice fields.
As mentioned above, there are considerable differences in soil water content, soil temperature, soil organic matter content, rice yield, and methane emissions between CW fields and NW field. However, N2O fluxes characteristics, total N2O emissions, and influencing factors of cold-waterlogged paddy fields have not been explored. We hypothesized that the cold-waterlogged paddy fields have lower N2O emissions than normal rice fields. The impact of rice planting on nitrous oxide emissions and the significant effect of nitrous oxide emissions should differ from normal rice fields. Therefore, this study intends to systematically monitor the cold-waterlogged paddy field’s N2O emissions characteristics on an annual scale in two representative regions and analyze the main controlling factors that affect N2O emissions. It’s significant to understand rice fields’ total greenhouse effect, accurately assessing the N2O emissions of China’s rice field system, and reasonably formulate the emission reduction measures of this type of rice field.
The study was conducted at two sites with different climate zones in Huangshi, Hubei Province, China. One belongs to a subtropical climate zone in Tuku Village, Baisha Town, Yangxin County (YX site, 2013), and soil-derived from acid aplite. Another is Huandiqiao Town, Daye City (DY site, 2014), a northern subtropical monsoon climate zone and soil derived from carbonatite. Soil physical and chemical properties of the surface layer soil (0–20 cm) are listed in Table 1. We conducted eight treatments, including NW planted with (NWR1) or without (NWR0) rice and CW planted with (CWR1) or without (CWR0) rice in both sites. The area of each plot with rice was 100 m2 (10 m × 10 m), and the subplot without rice was 3 m2 (1.5 m × 2 m). Each treatment had three replicates. Urea, calcium superphosphate, and potassium chloride were applied as nitrogen, phosphorous, and potassium fertilizers, respectively (N: P2O5: K2O = 180: 90: 120 kg hm−2) at both sites. Specifically, 50% nitrogen, 100% potassium, and 100% phosphorus were applied as basal fertilizer. The remaining 30% nitrogen applied at the jointing stage, and another 20% nitrogen applied ∼15 days after full heading.
TABLE 1. Soil physical and chemical properties (mean ± SD, n = 3) in paddy fields at the two experimental sites in Hubei Province, China.
N2O fluxes were measured using a static chamber technique, as reported previously (Xu et al., 2020). Each static chamber consisted of three parts: a bottom base, a middle chamber, and a top chamber. The chambers were wrapped with a layer of thermal insulation material. The base’s four walls were drilled at 10 cm from the top with two rows of 2-cm-diameter holes to facilitate water and fertilizer flow. The base (42 cm long × 42 cm wide × 20 cm high), with a groove around the top edge, was inserted 20 cm into the soil and remained in situ except for tillage. The middle chambers with a groove around the top edge and top chambers (42 cm long × 42 cm wide × 50 cm high) covered the base (with a volume equal to the sum of middle and top chambers).
At transplanting, we transplanted four rice plants (at the same density as outside of the chamber) in the base. The gas samples are sampled every 7–10 days in the non-rice season. During the rice planting period, gases were collected for five consecutive days; thereafter, the gases were periodically collected at 7-days intervals. For each sampling, the gas within the chamber was collected four times from 8:00–10:00 a.m., using a 30-ml gas-tight syringe at 0, 5, 10, 15, and 20 min. The samples were transported to the laboratory and analyzed within 24 h. Meanwhile, soil temperature at a depth of 5 cm was recorded using an electronic digital thermometer.
The concentrations of N2O in gas samples were analyzed by gas chromatography (Agilent 7890A, United States) equipped with an electron capture (ECD) for N2O concentration analyses at 350°C, and the carrier gas was purified N2. We calculate the N2O fluxes by making a linear regression of the gas concentration.
The N2O fluxes was calculated using the following formula:
Where F is the N2O flux (ug m−2h−1); ρ is the N2O density in the standard state (kg m−3); V is the effective volume of the closed chamber (m3), S is the base area (m2); dC/dt is the change of N2O concentration in the sealed chamber per unit time, and T is the average temperature in the closed section.
The N2O cumulative gas emissions was calculated by interpolation using the following formula (Iqbal et al., 2008):
where Ec is the cumulative emissions (mg m−2), n is the number of observations, Fi (ug m−2h−1), and Fi+1 (ug m−2 h−1) are the fluxes of the i and i+1 sampling, and ti and ti+1 are the i and i+1 sampling date.
Soil temperature near the base frames was measured at a depth of 5 cm in each plot and subplot using an E278 probe-type digital thermometer (Minggao Electronics Ltd., Shenzhen, China). Topsoil samples (0–20 cm) were collected randomly from five points per plot (including the plot and subplot) using a gauge auger (3-cm inner diameter) and transported immediately to the laboratory, and then homogenized and divided into two parts. One part was dried at 105°C for 24 h to determine soil water content by gravimetric. The other part was extracted with 0.5 M K2SO4 solution (soil: water = 1:5) for 1 h shaking and then filtrated to determine soil mineral N (NH4+-N and NO3−-N) and dissolved organic carbon (DOC). The NH4+-N and NO3−-N were analyzed using a flow-injection auto-analyzer. The DOC was measured with a TOC analyzer (Wu et al., 2017).
N2O accumulation emissions are expressed as the mean ± standard deviation (SD) from three replicates. Statistical analysis was conducted using SPSS 24 (IBM SPSS, Somers, United States). The relationship between N2O fluxes and environmental factors was performed in R (v3.6.1) using the “basicTrendline” packages with a single environmental factor as the independent variable and N2O flux as the dependent variable. The model parameter is used to select the fitting function, and the p-value and R2 value are used to determine the final regression model. Finally, SEMs were used to analyse the direct and indirect relationships between environmental factors and the N2O fluxes. The first step in an SEM requires establishing an a priori model based on the known effects and the relationships among the driving variables. The piecewiseSEM package (version 2.1.0) was used to analyze SEMs. We used non-significant (p > 0.05) Fisher’s C values to indicate a good fit (Ochoa-Hueso et al., 2020).
Regardless of rice planting, the mean soil water content of CW fields was significantly higher than that of NW fields during the monitoring period (Figure 1, p < 0.01), and rice planting has no difference at both types of fields at two sites. The average concentration of DOC for the CWR0 and CWR1 was significantly higher than those of the NWR0 and the NWR1 at the DY site (Figure 1, p < 0.01), but no difference at the YX site. The average concentration of NO3−-N for the CWR0 was significantly higher than that for the NWR0 at the DY site (Figure 1, p < 0.01), and the average concentration of NH4+-N of the CWR1 was significantly higher than that of the NWR1 at the DY site (Figure 1, p < 0.01). In the same site, the CW fields’ mean soil temperature was lower than that of the NW fields’ during the entire monitoring period, and the differences were not statistically significant (p > 0.05). However, from July 1, 2013, to September 1, 2013, the average soil temperature of the CW fields (28.45 ± 1.98°C) was significantly lower (p < 0.001) than the NW fields (29.87 ± 1.98°C) (Figure 1 A3), and from July 1, 2014, to September 1, 2014, the average soil temperature of the CW fields (29.97 ± 1.20°C) was significantly lower (p < 0.001) than the NW fields (31.52 ± 1.74°C) (Figure 1 A3).
FIGURE 1. The difference of environmental factors between YX site and DY site (adapted from Xu et al., 2020). Note: CWR0 and CWR1 are cold-waterlogged paddy field without and with rice planting, respectively; NWR0 and NWR1 are normal paddy field without and with rice planting, respectively. The number on the horizontal line indicates the significance of the difference. The points outside the box plot indicate outliers.
The N2O emissions characteristics of CW paddy fields and NW paddy fields are shown in Figure 2. The N2O fluxes at the YX site are between −32.93 −778.98 μg m−2 h−1, and the DY site is between −11.82 −93.42 μg m−2 h−1. The NW rice field of the YX site has three obvious emission peaks without rice. The other three treatments have no emission peaks. All the treatment emission peaks of the DY site are significantly lower than the YX site under the same treatment.
FIGURE 2. Annual N2O emissions from the CW and the NW paddy fields under different treatments. Note: CWR0 and CWR1 are cold-waterlogged paddy field without and with rice planting, respectively; NWR0 and NWR1 are normal paddy field without and with rice planting, respectively. The values are means ± SD (n = 3). BF, SF, and TF are base fertilization, seedling fertilization, and tillering fertilization.
The annual mean N2O fluxes of NWR0 treatment are 35.29 ± 16.17 μg m−2 h−1, and 8.91 ± 3.03 μg m−2 h−1 at YX and DY sites, respectively, and of CWR0 treatment are 4.26 ± 1.72 and 2.10 ± 1.31 μg m−2 h−1 at YX and DY sites, respectively. The mean N2O fluxes from CWR0 treatment was12.1% of that of NWR0 treatment at the YX site and was 23.6% at the DY site, respectively. The mean N2O fluxes of NWR1 treatment was 12.78 ± 2.91 μg m−2 h−1 at the YX site and was 36.00 ± 26.48 μg m−2 h−1 at the DY site, respectively. The mean N2O fluxes of CWR1 treatment was 3.82 ± 2.07 μg m−2 h−1 at the YX site and was 0.43 ± 1.43 μg m−2 h−1 at the DY site, respectively, and mean N2O fluxes from CWR1 treatment was 29.89% of that from NWR1 treatment at the YX site and was 1.20% at DY site, respectively.
The cumulative N2O emissions were calculated by interpolation (Table 2). The results showed that the N2O cumulative emissions from the CWR1 treatment were the lowest at both sites. The highest N2O cumulative emissions were observed in NWR0 treatment at the YX site and in NWR1 treatment at the DY site. Regardless of rice planting, N2O cumulative emissions of the NW fields were significantly higher than that in the CW fields (Table 2, p < 0.05) at both sites. Rice planting significantly reduced the cumulative N2O emissions from the NW field at the YX site but increased dramatically at the DY site. However, rice planting had no significant effect on the cumulative N2O emissions from CW fields at both sites (Table 2).
For the YX site, the N2O fluxes decrease first and then rise with the increase of the soil temperature in the NWR0 treatment (p < 0.001, Figure 3 A3). The N2O fluxes decrease first and then rise with the rise of the soil water content (p < 0.001, Figure 3 B3), and the N2O fluxes decrease first and then rise with the increase of the NH4+-N concentration (p < 0.001, Figure 3 D3) in the NWR1 treatment. For the DY site, the N2O fluxes decrease first and then rise with the increase of the soil DOC concentration (p < 0.05, Figure 4 C1) in the CWR0 treatment. The N2O fluxes decrease first and then rise with the increase of the soil temperature (p < 0.001, Figure 4 B1) in the CWR1 treatment. The N2O fluxes present a trend of first decreasing and then increasing with the increase of the soil temperature (p < 0.05, Figure 4 A4), and N2O fluxed increases with the soil NO3−-N concentration (p < 0.05, Figure 4 E4) in the NWR1 treatment. Other indicators at both sites have no significant relationship with N2O fluxes (Figure 3 and Figure 4).
FIGURE 3. A1, B1, C1, D1, and E1 means the relationships between N2O fluxes and soil temperature, soil water content, DOC contents, NH4+-N contents, and NO3−-N contents in the CWR0, respectively. A2, B2, C2, D2, and E1 means the relationships between N2O fluxes and soil temperature, soil water content, DOC contents, NH4+-N contents, and NO3−-N contents in the CWR1, respectively. A3, B3, C3, D3, and E3 means the relationships between N2O fluxes and soil temperature, soil water content, DOC contents, NH4+-N contents, and NO3−-N contents in the NWR0, respectively. A4, B4, C4, D4, and E4 means the relationships between N2O fluxes and soil temperature, soil water content, DOC contents, NH4+-N contents, and NO3−-N contents in the NWR1, respectively.
FIGURE 4. A1, B1, C1, D1, and E1 means the relationships between N2O fluxes and soil temperature, soil water content, DOC contents, NH4+-N contents, and NO3−-N contents in the CWR0, respectively. A2, B2, C2, D2, and E1 means the relationships between N2O fluxes and soil temperature, soil water content, DOC contents, NH4+-N contents, and NO3−-N contents in the CWR1, respectively. A3, B3, C3, D3, and E3 means the relationships between N2O fluxes and soil temperature, soil water content, DOC contents, NH4+-N contents, and NO3−-N contents in the NWR0, respectively. A4, B4, C4, D4, and E4 means the relationships between N2O fluxes and soil temperature, soil water content, DOC contents, NH4+-N contents, and NO3−-N contents in the NWR1, respectively.
The structural equation model showed that both fields’ N2O fluxes are significantly different between the experiment sites (p < 0.05, Figure 5). Soil temperature directly positively affects N2O fluxes in the CW field (Figure 5 CW). In contrast, other factors, such as soil water content, DOC, NO3−-N, NH4+-N, and rice planting, had no direct effect on N2O fluxes. Rice planting directly affects (p < 0.05, Figure 5 NW) on N2O fluxes at the NW fields. Simultaneously, the soil water content and rice planting directly affected N2O fluxes in the NW fields. Other factors, such as DOC content, NO3−-N content, and NH4+-N, have no direct effects on N2O fluxes in both sites. The DOC concentrations, NO3−-N, and NH4+-N of both type fields were significantly different in both sites (p < 0.005, in Fig. 6CW and Figure 5 NW).
FIGURE 5. Structural equation model. SEMs assessed direct and indirect associations between soil temperature (ST), soil water content (SWC), ammonium nitrogen (NH4+-N), nitrate-nitrogen (NO3−-N), and dissolved organic carbon (DOC) and N2O fluxes. Numbers adjacent to the arrows are standardized path coefficients, analogous to relative regression weights, and indicate the relationship’s effect size. Double-headed arrows indicate covariance between variables; single-headed arrows indicate a one way directed relationship. Solid lines indicate positive associations. Dashed lines indicate a negative association. The width of the arrow is proportional Line width is proportional to the strength of path coefficients. The proportion of variance explained (conditional R2) appears below every response variable in the model.
For NW paddy fields, rice planting has a significant direct positive effect on NO3−-N (p < 0.005, Figure 5 NW) and on NH4+-N (p < 0.005, Figure 5 NW), and a negative effect on DOC (p < 0.005, Figure 5 NW). Simultaneously, soil temperature has a significant direct negative effect on DOC (p < 0.005, Figure 5 NW) and a positive effect on NH4+-N (p < 0.005, Figure 6 NW). For CW paddy fields, rice planting and soil water content have a significant direct negative effect on NO3−-N (p < 0.005, Figure 5 CW), and soil temperature have a significant direct positive effect on NO3−-N (p < 0.05, Figure 5 CW), NH4+-N (p < 0.01, Figure 5 CW), and a significant direct negative effect on DOC (p < 0.005, Figure 5 CW). NO3−-N directly affects NH4+-N (p < 0.01, Figure 5 NW and Figure 5 CW) at both type fields.
Our results demonstrated that the N2O emissions from the CW fields are significantly lower than that of the NW fields, regardless of rice planting (p < 0.05, Table 2). In this study, the soil temperature of the CW fields is significantly lower than that of the NW rice fields during the high air temperature (Figure 6 A3 and B3). However, there is no significant difference on an annual scale. The relationship between soil temperature and N2O emissions is not uniform (Zhou et al., 2018; Wang et al., 2019); this difference is mainly affected by soil moisture (Wu et al., 2013). N2O emissions from soil are affected by the interaction of multiple environmental factors under natural conditions, and the relationship between temperature and water content determines whether to promote N2O emissions. This may be why the relationship between a single factor and N2O is not consistent in our study.
FIGURE 6. Annual variation trend of soil temperature and temperature difference during high temperature period. Note: Buried three temperature recorders in each field. (A) and (B) are the annual variation trend of 5 cm soil temperature during the monitoring period in YX site and DY site, respectively.
The N2O annual cumulative emissions from the NW fields are consistent with the results of Lan et al. (2020) but smaller than those reported by Huang et al. (2019), and the CW fields’ N2O annual emissions are lower than previous studies (Huang et al., 2019; Lan et al., 2020). The possible reason is that the soil water content in Huang’s research is lower than that of the NW fields and the CW fields in this study. In this study, the soil water content of the CW fields is significantly higher than that of NW fields on the annual scale (Figure 1). Soil moisture determines the soil’s redox state (Mei et al., 2011; Blagodatskaya et al., 2014). Previous research had shown that it might reduce 30–80% of N2O in the deep soil layer (anaerobic layer) to N2 before being released into the atmosphere (Clough et al., 2005). The N2 emissions from soil denitrification are considered to be a major gaseous N loss pathway, particularly in flooded paddy fields, where the strictly anaerobic environment promotes the complete reduction of nitrate or nitrite to N2 through the intermediates of N2O and NO (Davidson and Verchot, 2000; Butterbach-Bahl et al., 2013). In our study, the CW rice field has been saturated for a long time and under a strictly anaerobic state (Xu et al., 2020). The strong reduction state may lead to the complete reduction of N2O to N2 (Parton et al., 1996; Zhu et al., 2014). Simultaneously, the rice biomass accumulation is lower in the CW field than in the NW fields, and lower biomass accumulation means less N2O emissions (Xu et al., 2020). The above two points may lead to significantly lower N2O emissions from CW fields.
Rice planting may provide channels for N2O emissions, contributing more than 70% of soil N2O emissions during flooding but less than 20% after drainage (Yu et al., 1997; Yan et al., 2000). In this study, rice planting promoted the N2O accumulative emissions in the NW field at the DY site. However, the N2O emissions from NWR1 were significantly lower than that of NWR0 at the YX site, which may be related to more weeds in the treatment, and weeds (especially Monochoria vaginalis) could lead to a large amount of N2O production and emission. At the same time, it may also be the N2O emissions from NWR0 at the YX site were significantly higher than that of the DY site, which has no weeds. N2O emissions from NWR1 treatments at the DY site were significantly higher than that of the YX site, and this may be related to the lower rice biomass (15,957 kg hm−2 at the DY site and 15,021 kg hm−2 at the YX site) Xu et al. (2020) and the higher soil pH (Table 1), due to the N2O emissions from low-pH soils are significantly higher than those with high-pH soils (Wang et al., 2017).
N2O emissions from paddy fields are affected by various environmental factors (Schaufler et al., 2010; Hu et al., 2015a). Pärn et al. (2018) reported that soil NO3−-N and soil volumetric water content together determine the geographic differentiation of global organic soil N2O emissions (n = 58, R2 = 0.72, p < 0.001), and the relationship between soil temperature and N2O emissions is affected by region (Pärn et al., 2018). In the present study, the structural equation model showed that the N2O emissions of the same type of rice fields are significantly different between the different sites. At the same time, environmental factors have no significant direct effects on N2O emissions. However, there are significant direct or indirect effects between soil environmental factors in each type of paddy field, confirming the cover-up effect of regional differences on environmental factors (Pärn et al., 2018).
The CW fields’ annual N2O cumulative emissions were significantly lower than that of the NW fields under the same climatic conditions and planting systems. N2O emissions from the CW fields are mainly in the flooding period after transplanting, while the NW fields are primarily in the drainage period after flooding. N2O emissions from the CW fields are mainly affected by soil temperature; however, they are mainly affected by rice planting and soil moisture from the NW fields. The CW fields have very low N2O emissions and may have gaseous nitrogen emissions by denitrification. We suggest that follow-up research should study and evaluate the gaseous nitrogen emissions, and this has certain enlightenment for the governance of environmental nitrogen pollution.
The original contributions presented in the study are included in the article/Supplementary Materials, further inquiries can be directed to the corresponding author/s.
XX and JY conceived the idea, XX, MZ, and YX conducted experiment analyzed data, XX, MZ, and MS wrote the manuscript, RH, JY, and MS. reviewed, revised and improved the manuscript.
This study was financially supported by the National Natural Science Foundation of China (No. 41301306), the Scientific and Technological Achievements Cultivation Project of Hubei Academy of Agricultural Sciences, China (2017CGPY01), and the Integration and Demonstration of Key Technologies of Crop Straw Returning in Hubei Province, China.
The authors declare that the research was conducted in the absence of any commercial or financial relationships that could be construed as a potential conflict of interest.
Alexandratos, N., and Bruinsma, J. (2012). World Agriculture towards 2030/2050: The 2012 Revision. Rome: FAO, ESA Working paper.
Blagodatskaya, Е., Zheng, X., Blagodatsky, S., Wiegl, R., Dannenmann, M., and Butterbach-Bahl, K. (2014). Oxygen and Substrate Availability Interactively Control the Temperature Sensitivity of CO2 and N2O Emission from Soil. Biol. Fertil. Soils 50, 775–783. doi:10.1007/s00374-014-0899-6
Butterbach-Bahl, K., Baggs, E. M., Dannenmann, M., Kiese, R., and Zechmeister-Boltenstern, S. (2013). Nitrous Oxide Emissions from Soils: How Well Do We Understand the Processes and Their Controls?. Philosophical Trans. R. Soc. B 368, 1–13. doi:10.1098/rstb.2013.0122
Clough, T. J., Sherlock, R. R., and Rolston, D. E. (2005). A Review of the Movement and Fate of N2O in the Subsoil. Nutr. Cycl Agroecosyst 72, 3–11. doi:10.1007/s10705-004-7349-z
Čuhel, J., Šimek, M., Laughlin, R. J., Bru, D., Chèneby, D., Watson, C. J., et al. (2010). Insights into the Effect of Soil pH on N2O and N2 Emissions and Denitrifier Community Size and Activity. Appl. Environ. Microbiol. 76, 1870–1878.
Davidson, E. A., and Verchot, L. V. (2000). Testing the Hole-In-The-Pipe Model of Nitric and Nitrous Oxide Emissions from Soils Using the TRAGNET Database. Glob. Biogeochem. Cycles 14, 1035–1043. doi:10.1029/1999gb001223
Fierer, N., and Schimel, J. P. (2002). Effects of Drying-Rewetting Frequency on Soil Carbon and Nitrogen Transformations. Soil Biol. Biochem. 34, 777–787. doi:10.1016/s0038-0717(02)00007-x
Gaihre, Y. K., Singh, U., Islam, S. M. M., Huda, A., Islam, M. R., Sanabria, J., et al. (2017). Nitrous Oxide and Nitric Oxide Emissions and Nitrogen Use Efficiency as Affected by Nitrogen Placement in lowland rice fields. Nutr. Cycl Agroecosyst 110, 277–291. doi:10.1007/s10705-017-9897-z
Hofstra, N., and Bouwman, A. F. (2005). Denitrification in Agricultural Soils: Summarizing Published Data and Estimating Global Annual Rates. Nutr. Cycl Agroecosyst 72, 267–278. doi:10.1007/s10705-005-3109-y
Hu, H.-W., Chen, D., He, J.-Z., and van der Meer, J. R. (2015a). Microbial Regulation of Terrestrial Nitrous Oxide Formation: Understanding the Biological Pathways for Prediction of Emission Rates. FEMS Microbiol. Rev. 39, 729–749. doi:10.1093/femsre/fuv021
Hu, H.-W., Macdonald, C. A., Trivedi, P., Holmes, B., Bodrossy, L., He, J.-Z., et al. (2015b). Water Addition Regulates the Metabolic Activity of Ammonia Oxidizers Responding to Environmental Perturbations in Dry Subhumid Ecosystems. Environ. Microbiol. 17, 444–461. doi:10.1111/1462-2920.12481
Huang, R., Wang, Y., Liu, J., Li, J., Xu, G., Luo, M., et al. (2019). Variation in N2O Emission and N2O Related Microbial Functional Genes in Straw- and Biochar-Amended and Non-amended Soils. Appl. Soil Ecol. 137, 57–68. doi:10.1016/j.apsoil.2019.01.010
IPCC (2014). “Summary for Policymakers. Climate Change. Mitigation of Climate Change,” in Contribution of Working Group III to the Fifth Assessment Report of the Intergovernmental Panel on Climate Change. Cambridge, United Kingdom: Cambridge University Press, 1–33. doi:10.1017/CBO9781107415324
Iqbal, J., Ronggui, H., Lijun, D., Lan, L., Shan, L., Tao, C., et al. (2008). Differences in Soil CO2 Flux between Different Land Use Types in Mid-subtropical China. Soil Biol. Biochem. 40, 2324–2333. doi:10.1016/j.soilbio.2008.05.010
Islam, S. M. M., Gaihre, Y. K., Biswas, J. C., Singh, U., Ahmed, M. N., Sanabria, J., et al. (2018). Nitrous Oxide and Nitric Oxide Emissions from lowland rice Cultivation with Urea Deep Placement and Alternate Wetting and Drying Irrigation. Sci. Rep. 8, 17623. doi:10.1038/s41598-018-35939-7
Lan, T., Li, M., Han, Y., Deng, O., Tang, X., Luo, L., et al. (2020). How Are Annual CH4, N2O, and NO Emissions from rice-wheat System Affected by Nitrogen Fertilizer Rate and Type?. Appl. Soil Ecol. 150, 103469. doi:10.1016/j.apsoil.2019.103469
Liu, Y., Lu, H., Yang, S., and Wang, Y. (2016). Impacts of Biochar Addition on rice Yield and Soil Properties in a Cold Waterlogged Paddy for Two Crop Seasons. Field Crops Res. 191, 161–167. doi:10.1016/j.fcr.2016.03.003
Mei, B., Zheng, X., Xie, B., Dong, H., Yao, Z., Liu, C., et al. (2011). Characteristics of Multiple-Year Nitrous Oxide Emissions from Conventional Vegetable fields in southeastern China. J. Geophys. Res. 116, D12113. doi:10.1029/2010jd015059
Ochoa-Hueso, R., Borer, E. T., Seabloom, E. W., Hobbie, S. E., Risch, A. C., Collins, S. L., et al. (2020). Microbial Processing of Plant Remains Is Co-limited by Multiple Nutrients in Global Grasslands. Glob. Chang Biol. 26, 4572–4582. doi:10.1111/gcb.15146
Pärn, J., Verhoeven, J. T. A., Butterbach-Bahl, K., Dise, N. B., Ullah, S., Aasa, A., et al. (2018). Nitrogen-rich Organic Soils under Warm Well-Drained Conditions Are Global Nitrous Oxide Emission Hotspots. Nat. Commun. 9 (1), 1135. doi:10.1038/s41467-018-03540-1
Parton, W. J., Mosier, A. R., Ojima, D. S., Valentine, D. W., Schimel, D. S., Weier, K., et al. (1996). Generalized Model for N2 and N2O Production from Nitrification and Denitrification. Glob. Biogeochem. Cycles 3 (10), 401–412. doi:10.1029/96gb01455
Patrick, W. H., and Wyatt, R. (1964). Soil Nitrogen Loss as a Result of Alternate Submergence and Drying. Soil Sci. Soc. America J. 28, 647–653. doi:10.2136/sssaj1964.03615995002800050021x
Qiu, S., Wang, M., Wang, F., Chen, J., Li, X., Li, Q., et al. (2013). Effects of Open Drainage Ditch Design on Bacterial and Fungal Communities of Cold Waterlogged Paddy Soils. Braz. J. Microbiol. 44 (3), 983–991. doi:10.1590/s1517-83822013000300050
Schaufler, G., Kitzler, B., Schindlbacher, A., Skiba, U., Sutton, M. A., and Zechmeister-Bolternstern, S. (2010). Greenhouse Gas Emissions from European Soils under Different Land Use: Effects of Soil Moisture and Temperature. Eur. J. Soil Sci. 61 (5), 683–696. doi:10.1111/j.1365-2389.2010.01277.x
Sheng, R., Meng, D., Wu, M., Di, H., Qin, H., and Wei, W. (2013). Effect of Agricultural Land Use Change on Community Composition of Bacteria and Ammonia Oxidizers. J. Soils Sediments 13, 1246–1256. doi:10.1007/s11368-013-0713-3
Spott, O., Russow, R., and Stange, C. F. (2011). Formation of Hybrid N2O and Hybrid N2 Due to Codenitrification: First Review of a Barely Considered Process of Microbially Mediated N-Nitrosation. Soil Biol. Biochem. 43, 1995–2011. doi:10.1016/j.soilbio.2011.06.014
Wang, A., Ma, X., Xu, J., and Lu, W. (2019). Methane and Nitrous Oxide Emissions in rice-crab Culture Systems of Northeast China. Aquacult. Fish. 4, 134–141. doi:10.1016/j.aaf.2018.12.006
Wang, H., Guan, D., Zhang, R., Chen, Y., Hu, Y., and Xiao, L. (2014). Soil Aggregates and Organic Carbon Affected by the Land Use Change from rice Paddy to Vegetable Field. Ecol. Eng. 70, 206–211. doi:10.1016/j.ecoleng.2014.05.027
Wang, Y., Guo, J., Vogt, R. D., Mulder, J., Wang, J., and Zhang, X. (2017). Soil pH as the chief modifier for regional nitrous oxide emissions: New evidence and implications for global estimates and mitigation. Global Change Biol. 24, e617–e626.
Weller, S., Janz, B., Jörg, L., Kraus, D., Racela, H. S. U., Wassmann, R., et al. (2016). Greenhouse Gas Emissions and Global Warming Potential of Traditional and Diversified Tropical rice Rotation Systems. Glob. Change Biol. 22, 432–448. doi:10.1111/gcb.13099
Wissing, L., Kölbl, A., Häusler, W., Schad, P., Cao, Z.-H., and Kögel-Knabner, I. (2013). Management-induced Organic Carbon Accumulation in Paddy Soils: The Role of Organo-mineral Associations. Soil Tillage Res. 126, 60–71. doi:10.1016/j.still.2012.08.004
Wu, D., Dong, W., Oenema, O., Wang, Y., Trebs, I., and Hu, C. (2013). N2O Consumption by Low-Nitrogen Soil and its Regulation by Water and Oxygen. Soil Biol. Biochem. 60, 165–172. doi:10.1016/j.soilbio.2013.01.028
Wu, L., Tang, S., He, D., Wu, X., Shaaban, M., Wang, M., et al. (2017). Conversion from rice to Vegetable Production Increases N 2 O Emission via Increased Soil Organic Matter Mineralization. Sci. Total Environ. 583, 190–201. doi:10.1016/j.scitotenv.2017.01.050
Xie, K., Xu, P., Yang, S., Lu, Y., Jiang, R., Gu, W., et al. (2015). Effects of Supplementary Composts on Microbial Communities and rice Productivity in Cold Water Paddy fields. J. Microbiol. Biotechnol. 25, 569–578. doi:10.4014/jmb.1407.07066
Xu, X. Y., Zhang, M. M., Xiong, Y. S., Yuan, J. F., Shaaban, M., Zhou, W., et al. (2020). The Influence of Soil Temperature, Methanogens and Methanotrophs on Methane Emissions from Cold Waterlogged Paddy fields. J. Environ. Manag. 264, 110421. doi:10.1016/j.jenvman.2020.110421
Yan, X., Shi, S., Du, L., and Xing, G. (2000). Pathways of N2O Emission from rice Paddy Soil. Soil Biol. Biochem. 32 (3), 437–440. doi:10.1016/s0038-0717(99)00175-3
Yu, K. W., Wang, Z. P., and Chen, G. X. (1997). Nitrous Oxide and Methane Transport through rice Plants. Biol. Fertil. Soils 24 (3), 341–343. doi:10.1007/s003740050254
Zhou, M., Wang, X., Wang, Y., and Zhu, B. (2018). A Three-Year experiment of Annual Methane and Nitrous Oxide Emissions from the Subtropical Permanently Flooded rice Paddy fields of China: Emission Factor, Temperature Sensitivity and Fertilizer Nitrogen Effect. Agric. For. Meteorol. 250-251, 299–307. doi:10.1016/j.agrformet.2017.12.265
Zhu, K., Bruun, S., Larsen, M., Glud, R. N., and Jensen, L. S. (2014). Spatial Oxygen Distribution and Nitrous Oxide Emissions from Soil after Manure Application: a Novel Approach Using Planar Optodes. J. Environ. Qual. 43 (5), 1809–1812. doi:10.2134/jeq2014.03.0125
Zhu, X., Burger, M., Doane, T. A., and Horwath, W. R. (2013). Ammonia Oxidation Pathways and Nitrifier Denitrification Are Significant Sources of N2O and NO under Low Oxygen Availability. Proc. Natl. Acad. Sci. 110 (16), 6328–6333. doi:10.1073/pnas.1219993110
Keywords: cold-waterlogged paddy field, N2O, soil temperature, nitrate, ammonium
Citation: Xu X, Zhang M, Xiong Y, Shaaban M, Yuan J and Hu R (2021) Comparison of N2O Emissions From Cold Waterlogged and Normal Paddy Fields. Front. Environ. Sci. 9:660133. doi: 10.3389/fenvs.2021.660133
Received: 28 January 2021; Accepted: 18 June 2021;
Published: 05 July 2021.
Edited by:
Fereidoun Rezanezhad, University of Waterloo, CanadaReviewed by:
Alison E. King, Colorado State University, United StatesCopyright © 2021 Xu, Zhang, Xiong, Shaaban, Yuan and Hu. This is an open-access article distributed under the terms of the Creative Commons Attribution License (CC BY). The use, distribution or reproduction in other forums is permitted, provided the original author(s) and the copyright owner(s) are credited and that the original publication in this journal is cited, in accordance with accepted academic practice. No use, distribution or reproduction is permitted which does not comply with these terms.
*Correspondence: Jiafu Yuan, ZnUxNjgyQHNpbmEuY29t
†These authors have contributed equally to this work
Disclaimer: All claims expressed in this article are solely those of the authors and do not necessarily represent those of their affiliated organizations, or those of the publisher, the editors and the reviewers. Any product that may be evaluated in this article or claim that may be made by its manufacturer is not guaranteed or endorsed by the publisher.
Research integrity at Frontiers
Learn more about the work of our research integrity team to safeguard the quality of each article we publish.