- 1State Key Laboratory of Grassland Agro-Ecosystems, Institute of Arid Agroecology, School of Life Sciences, Lanzhou University, Lanzhou, China
- 2Institute of Ecology, School of Applied Meteorology, Nanjing University of Information Science and Technology, Nanjing, China
Land use patterns and vegetation coverage in semi-arid areas of the Loess Plateau have undergone great changes due to the implementation of the “Grain for Green” project. The introduction of legume pasture species, such as alfalfa (Medicago sativa L.) and sweet clover (Melilotus officinalis L.), is one of the most efficient methods of vegetation restoration and reconstruction in this region. However, there is a need for an effective assessment of the root system distribution and its interaction with soil after long-term introduction. An experiment involving the introduction of alfalfa and sweet clover on abandoned farmlands was initiated in 2003 to assess the long-term effects. After 17 years, root and soil samples at depths of 0–20 and 20–60 cm were collected to characterize the root biomass, root carbon (C), nitrogen (N), and phosphorus (P), soil microbial biomass carbon (MBC) and nitrogen (MBN), soil organic carbon (SOC), and soil N and P. The results showed that the root biomass density of alfalfa in the 0–20 and 20–60 cm layers (63.72 and 12.27 kg m–3, respectively) were significantly higher than for sweet clover (37.43 and 8.97 kg m–3, respectively) and under natural abandonment (38.92 and 9.73 kg m–3, respectively). The SOC, total nitrogen (TN), total phosphorus (TP), available phosphorus (AP), nitrate-nitrogen (NO3–-N), MBC and MBN in the 0–20 and 20–60 cm layers were higher after alfalfa introduction compared with sweet clover introduction and natural abandonment, although the ammonia-nitrogen (NH4+-N) concentration in the 0–20 cm layer was lower. There were significantly positive correlations between root biomass density and both soil nutrients and microbial biomass, while there was a negative correlation between the soil NH4+-N and root biomass density. These results indicate that alfalfa root growth improved soil organic matter accumulation and nutrient mineralization. The accumulation and mineralization of soil nutrients also guaranteed root and microorganism growth. Therefore, it was concluded that alfalfa introduction will promote soil nutrients immobilization and mineralization and may enable sustainable land use in the semi-arid region of the Loess Plateau, China.
Introduction
Soil contains the largest carbon (C) pool in the terrestrial ecosystem, being 3.3 times larger than the atmospheric pool and 4.5 times larger than the biotic storage (Lal, 2004). It plays a vital role in regulating climate warming (Melillo et al., 2002; Zhou et al., 2012). In addition, soil nitrogen (N) and phosphorus (P) are essential elements for vegetation growth (Westheimer, 1987; Azziz et al., 2012; Yamashita et al., 2020). The soil C, N, and P cycles are strongly coupled with biological processes (Elser et al., 2000; Reed et al., 2015). Land use changes due to either natural or anthropogenic activities, can induce changes in the C balance and nutrient flow, thereby altering ecosystem functions (Post and Kwon, 2000; Deng et al., 2016). Slight changes in C and N exchanges between the soil ecosystem and atmosphere could have a significant impact on global climate change (Classen et al., 2015). Therefore, it is essential to assess the effects of land use on soil biogeochemical cycles in the future under global climate change.
The Loess Plateau in China is an ecologically fragile zone, with serious soil erosion issues (Fu, 1989; Chen et al., 2007; Zhao et al., 2013), and is also sensitive to climate change (He et al., 2006; Liu and Sang, 2013; Miao et al., 2016; Fang et al., 2017; Fang et al., 2018; Gong et al., 2020). Revegetation of degraded land is one of the principal strategies used to control soil erosion and recover ecosystem function, which has had significant effects on soil quality (Yuan et al., 2019), and even on socioeconomic development (Fu et al., 2011; Zhao et al., 2013). The “Grain for Green” project (converting steep farmlands to forest or grassland) was initiated by the Chinese government in 1999 to undertake ecological restoration (Feng et al., 2005; Deng et al., 2014). In this process, legumes such as alfalfa, sweet clover, and Caragana species are widely used as pioneer species to restore damaged or degenerated ecosystems due to their strong environmental adaptability, N fixation benefits, and rapid formation of continuous vegetation cover (An et al., 2013; Yuan et al., 2016b; Yao et al., 2020). Vegetation succession may affect the input and decomposition processes of soil C or N; thus, affecting its storage (Fujii et al., 2020). Carbon sequestration requires a large amount of N, resulting in the soil N concentration being one of the limiting factors for soil C storage (van Groenigen et al., 2017). Legumes have the potential to increase the N input into ecosystems, while promoting C and N accumulation in vegetation and soil (Vitousek et al., 2013; Hu et al., 2016). Phosphorus is considered to be a stable element in the soil ecosystem (Vitousek et al., 2010); thus, the process of P fixation and mineralization should also be considered when assessing the introduction of legumes. Vegetation change has a profound impact on the soil biogeochemical cycles of ecosystems at regional and global scales (Yu et al., 2018). In addition, there is a lag effect in the response of soil to vegetation restoration (Knops and Tilman, 2000). Therefore, it is essential to assess the long-term effects of land use change on soil C, N, and P in this region.
How the aboveground biomass responds to the extent of vegetation restoration or stand age has been extensively studied (Liu et al., 2015; Zhang et al., 2017). Land use change can affect the functional composition of plant communities (Hoelzle et al., 2012; Turner et al., 2019), and would finally influence the chemical characteristics of roots and root biomass in the long-term (Mokany et al., 2006; Qiu et al., 2012). However, there have been few studies of the changes in belowground biomass. Land-use changes could alter the magnitude of C inputs into soil by changing the biomass of plant residues, including both aboveground and belowground biomass (Frasier et al., 2019). The input of aboveground litter, fine root turnover, and root exudates increases the C, N, and P concentration in the surface soil, while roots and root exudates are the main sources of organic matter in the deep soil (Rumpel and Kogel-Knabner, 2011; Schmidt et al., 2011). However, roots store SOC and N more efficiently than shoots (Kong and Six, 2010; Jackson et al., 2017; Sokol and Bradford, 2019) and a specific relationship has been observed between root biomass and SOC accumulation (Fornara and Tilman, 2008). In addition, roots not only directly affect the SOC concentration, but also form aggregates with the soil particles (Gale et al., 2000), driving the physical-chemical stabilization of SOC (Six et al., 2000; Six et al., 2004). Although P is derived from the weathering of parent material and ecosystems exist with a constant P content (Walker and Syers, 1976), root exudates can directly or indirectly affect P availability in the rhizosphere (Schilling et al., 1998; Gransee, 2001). Legume introduction extends the rhizosphere in both the horizontal and vertical directions, leading to a change in the distribution of soil C, N, and P (Pransiska et al., 2016). However, exactly how roots regulate soil C, N, and P after legume introduction still remains unclear.
Land-use change is the main factor that affects plant litter input, root turnover, and exudation, all of which make a large contribution to microbial activity and community composition (Campbell et al., 1997; Pandey et al., 2010; Singh and Ghoshal, 2014). Soil microorganisms are the main source of soil enzymes, which have a key role in C, N, and P transformation, playing an important part in the biogeochemical cycles of terrestrial ecosystems (Kiss et al., 1975). Microbial associations with roots in the soil are complex and can enhance the ability of plants to acquire nutrients from soil through the turnover of microbial biomass within the rhizosphere (Vanveen et al., 1989; Boyrahmadi and Raiesi, 2018). It is therefore necessary to consider the root-microorganism relationship in the process of revegetation.
The impact of land use change on soil properties, root systems, and soil microorganisms is of great importance in ecosystem management and policy making. Therefore, an experiment was conducted in the semi-arid region of the Loess Plateau to determine the long-term effects of legume introduction on soil properties [SOC, total nitrogen (TN), total phosphorus (TP), microbial biomass carbon (MBC) and nitrogen (MBN), mineral N, available phosphorus (AP)] at depths of 0–20 and 20–60 cm and their inter-relationship. We hypothesized that: (1) legume introduction increases the root biomass of communities and microorganisms (i.e., the MBC and MBN), leading to an increase in the concentrations of mineral nutrients; and (2) legume introduction improves soil C and N storage, but has no significant effect on the TP concentration due to its stability.
Materials and Methods
The Study Area
The study was conducted at the Gansu Dryland Agroecology Observation and Research Station on the Loess Plateau (36°02′N, 104°25′E, 2,400 m above sea level), Lanzhou University, where a long-term revegetation experiment was established in 2003. The station is located in the northern mountain region of Yuzhong County, Gansu Province, China. This region is characterized by a semi-arid climate, with a mean annual temperature and precipitation of 7.5°C and 378.4 mm, respectively, during the experiment (2003–2019). Most of the precipitation occurred in June to September. The soil had a mean soil bulk density of 1.28 g cm–3, pH of 8.2, and calcium carbonate (CaCO3) concentration of 146 g kg–1. The soil was classified as a Heima [a calcic Kastanozems, according to the World Reference Base for Soil Resources (WRB)], with a field water holding capacity of 23% and a permanent wilting point of 4.5% (Shi et al., 2003). This area is subjected to both wind and soil erosion.
To address the pressure caused by the expanding human population since the 1950s, cropland became the main land use in this region, even in sloping areas. After livelihood conditions improved, croplands with a slope greater than 15° were converted into grasslands as part of the “Grain for Green” project, which was beneficial to both the environment and animal husbandry.
Experimental Settings and Design
In April 2003, three hillside fields were selected for revegetation, following the cultivation of spring wheat. The three fields had different landscape aspects, one was north–east facing, with a slope of 10–14°, and the other two were south-east facing with slopes of 12–16° and 4–8°, respectively. The distance between each site was less than 1,000 m and they all had the same elevation. We divided each field into three plots. The size of each plot was 35 m × 45 m, and they were located next to each other. The three plots were treated randomly with: (i) fallow conditions, (ii) alfalfa (Medicago sativa L.) (perennial legume species) introduced at a seed density of 22.5 kg ha–1, and iii) sweet clover (Mentha suaveolens L.) (biennial legume species) introduced at a seed density of 11.3 kg ha–1. These densities were optimal for planting based on local farming practices. The plant community succeeded naturally with no further management practices, such as grazing (fencing), tillage, fertilization, or harvesting after legume introduction (Yuan et al., 2016a). A plant community survey was conducted in August every year, taking care to avoid disturbance (Yuan et al., 2015).
Root and Soil Sampling
After 17 years of vegetation restoration, root biomass was measured in the most vigorous vegetation-growing period in August 2019. Root biomass was measured using a root drill (70 mm inner diameter; 100 mm height, Kezheng Instrument Co. Ltd., Shangyu, Shaoxing). The sample points were 3 m from the margin so avoiding “Edge effects.” Three random samplings were conducted at the upper, middle, and lower sites in each sample plot, with sampling increments at soil depths of 0–20 and 20–60 cm. We got 3 soil samples and mixed them as a sample each site. So, 54 root samples were obtained (3 replication, 3 treatments, 3 landscapes, and 2 soil layers). A root mesh bag, with a 0.15 mm mesh size, was used to wash the soil attached to the roots. Then, the roots were dried to a constant mass at 75°C and root biomass was measured.
In April 2019, i.e., the initiation of the growing season, soil samples were collected in the 0–20 and 20–60 cm soil layers using a soil auger, with an inner diameter of 4 cm. The sampling principle is consistent with the root sample. Three soil cores were collected and mixed together in each plot. All the visible litter and roots were removed by hand, soil samples were sieved through a 2-mm aperture screen (plant residue on the sieve was discarded). Each soil sample was then divided into two parts. One was stored at 4°C to determine soil moisture, MBC and MBN, and inorganic N at the field moisture level. The other was air dried for the determination of SOC, TN, and P.
The Determination of Root and Soil Properties
The dried plant samples were crushed by an ultra-centrifugal mill to enable the determination of C, N, and P in the root system. The root C was determined by the potassium dichromate oxidation method, and root N and P were measured after digestion in a H2SO4-H2O2 mixture using the Auto-Kjeldahl and the molybdenum-antimony spectrophotometry methods (Thomas et al., 1967).
Soil samples were soaked in a 2 M KCl solution, shaken in a concentrator at 200 rpm for 1 h and then filtered into centrifugal tubes. An auto-flow injection system (Skalar, Breda, Netherlands) was then used to measure nitrate-N (NO-N) and ammonium-N (NH-N). The fumigation—extraction method was used to determine MBC and MBN. The soil samples were extracted by 0.5 M K2SO4, shaking 1 h at 200 rpm. And then, MBC and MBN were directly measured using a CHN Analyzer (LECO CHN–1000, Michigan, United States). The difference between total organic C and TN extracted with 0.5 M K2SO4 was determined through chloroform-fumigated and non-fumigated soil samples. Factors of 0.45 and 0.54 were applied to adjust the recovery of MBC and MBN (Brookes et al., 1985; Beck et al., 1997). The values were calculated based on air-dry soil.
Air-dry samples (<0.15 mm) were used for the measurement of SOC by the Walkley and Black dichromate oxidation method (Nelson and Sommers, 1982), TN by the K2SO4–CuSO4–Se (100:10:1) distillation method (Bremner and Mulvaney, 1982), and TP by a colorimetric method at 700 nm (UV-1800, Mapada, Shanghai, China) after soil digestion in an HClO4-H2SO4 mixture (O’Halloran and Cade-Menun, 2006). Available phosphorus (<2.00 mm) was extracted with NaHCO3 according to the Olsen method (Olsen, 1954).
Statistical Analyses
Before analysis, the data were analyzed for normal distribution by the Shapiro–Wilk test. If not normally distributed, the data were transformed by log10 before analysis. A one-way analysis of variance (ANOVA, in randomized blocks) was applied to test the differences in root biomass density, root C, N, and P, MBC and MBN, SOC, TN, and TP among the fallow, alfalfa, and sweet clover treatments using the Genstat18.0 software (VSN International, Hemel Hempstead, United Kingdom). The landscape was set as a block. Significant differences were compared by Tukey test at P < 0.05. A Pearson’s correlation analysis was used to evaluate the correlations among root biomass density and soil nutrients. Principal Component Analysis (PCA) was conducted using CANOCO 4.5 (Plant Research International, Wageningen, Netherlands). Structural equation modeling (SEM) was performed using AMOS 21.0 (Amos Development Corporation, Chicago, IL, United States) to quantify the relative importance of the potential direct and indirect pathways, which could affect root biomass based on the Pearson’s correlation analysis, conceptual modeling (Supplementary Figure A), the goodness of model fit, and logical reasoning. The Origin 9.0 was used to drawn all graphs (OriginLab OriginPro 2015, United States).
Results
The C, N, and P Concentrations in the Root Biomass
Both the root biomass density and its C, N, and P concentration varied throughout the soil profile among the revegetation methods (Figures 1A–D). The concentrations of all three elements decreased with soil depth. Seventeen years after legume introduction, the root biomass density in the alfalfa field was significantly higher than in the fallow and sweet clover fields (Figure 1A). In the 0–20 cm layer, root C and N in the alfalfa field were significantly higher than in the fallow and sweet clover fields (Figures 1B,C). The root P concentration was lowest in the alfalfa field, but the differences in the 0–20 cm layer were not significant (Figure 1D). In the 20–60 cm layer, the root N concentration in the alfalfa field showed no differences from the fallow field, but were much higher than in the sweet clover field (Figure 1B). The root C and P concentrations in the 20–60 cm layer had intermediate values and displayed the same tendency as N (Figures 1A,C), but the root C concentration in the alfalfa and sweet clover fields was much lower than in the fallow field. There were no differences in the root P concentration in the 0–20 cm layer in the alfalfa field compared with the fallow and sweet clover fields (Figure 1D).
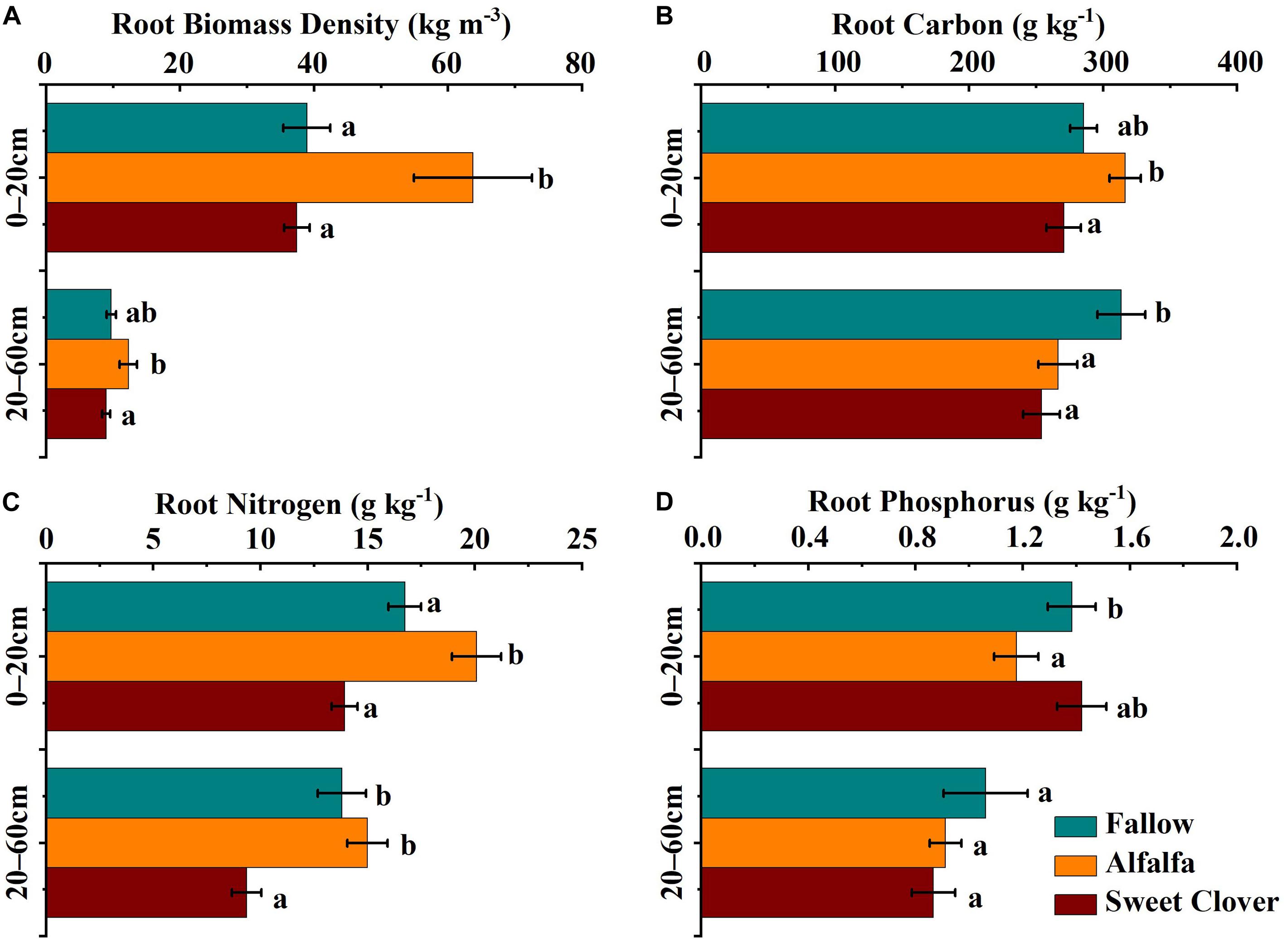
Figure 1. Root biomass density (A), carbon (B), nitrogen (C), and phosphorus (D) distributions at different soil depths under different revegetation treatments in 2019 (17 years after revegetation). Data represents the mean value ± standard error (n = 9), and the different letters indicate significant differences at the p < 0.05 level.
The SOC, TN, TP, Nitrate-Nitrogen (NO3–-N), Ammonia-Nitrogen (NH4+-N), and AP Concentrations in Soils
The SOC, TN, and TP concentrations generally declined with soil depth. The highest SOC concentration in the 0–20 and 20–60 cm layers were observed in the alfalfa field, with average values of 12.38 and 9.77 g kg–1, respectively, followed by fallow (9.73 and 6.01 g kg–1, respectively) and sweet clover (10.76 and 4.94 g kg–1, respectively) (Figure 2A). The tendency of TN was similar to that of SOC (Figure 2B). There was little change in TP compared with SOC and TN, but the concentration in the alfalfa field was significantly higher than in the other two treatments (Figure 2C).
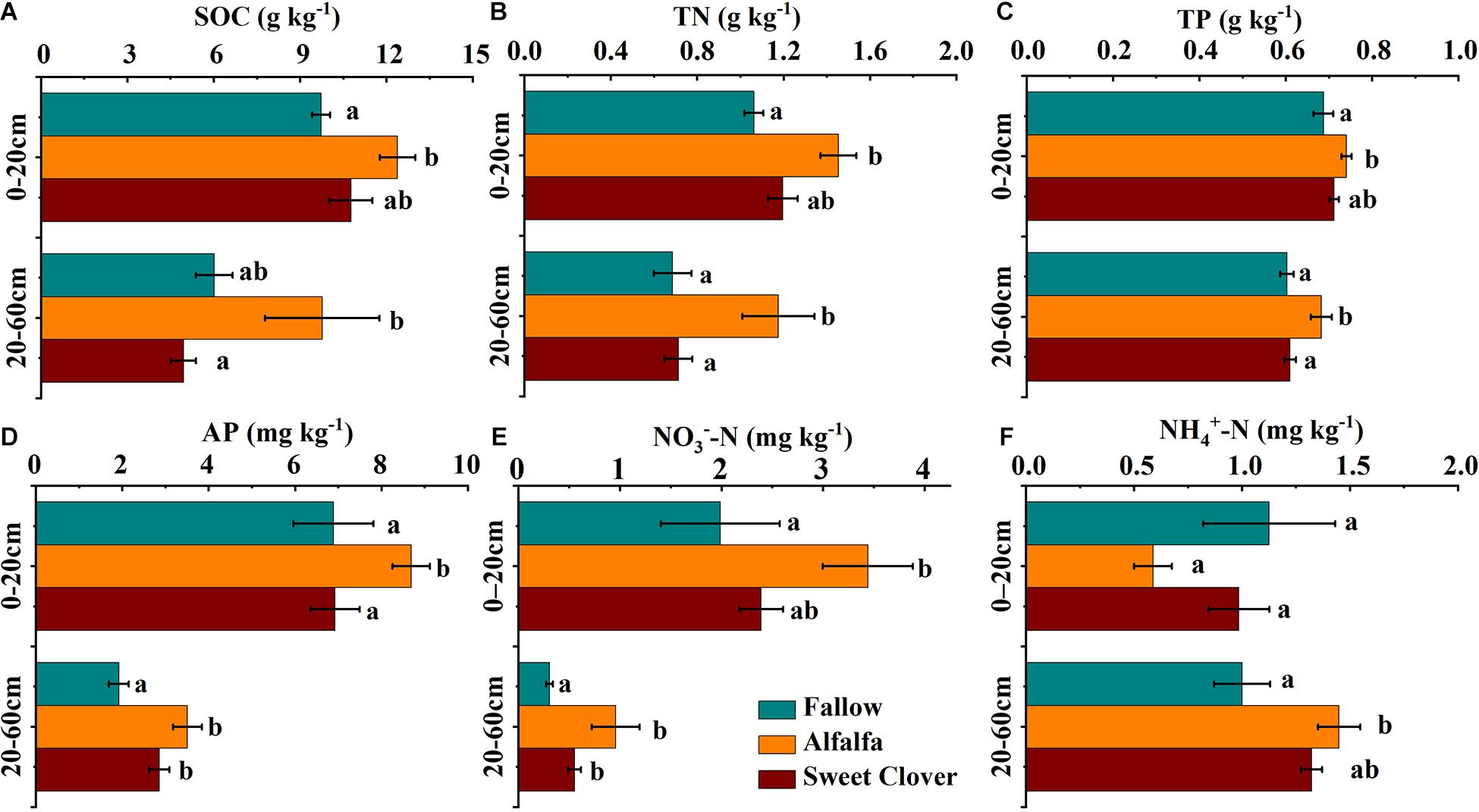
Figure 2. Soil organic carbon (SOC) (A), TN (B), TP (C), AP (D), NO3–-N (E), and NH4+-N (F) distributions at different soil depths under different revegetation treatments in 2019 (17 years after revegetation). Data represents the mean value ± standard error (n = 9), and the different letters indicate significant differences at the p < 0.05 level. (SOC, soil organic carbon; TN, total nitrogen; TP, total phosphorus; AP, available phosphorus; NO3–-N, nitrate nitrogen; NH4+-N, ammonium nitrogen).
The changes in the NO3–-N and NH4+-N concentrations in the 0–20 and 20–60 cm layers are presented in Figures 2E,F. The lowest NH4+-N concentration was observed in the 0–20 cm layer in the alfalfa field and increased with soil depth. Total inorganic nitrogen (TIN: NO3–-N + NH4+-N) in the alfalfa field was still significantly high. Compared with the fallow field, the AP concentration in the 0–20 and 20–60 cm layers also increased after legume introduction (Figure 2D). Alfalfa introduction resulted in an increase in the AP concentration in both soil layers, while sweet clover introduction had little effect.
Soil Microbial Properties
The highest MBC and MBN values were observed in the 0–20 cm layer in the alfalfa field (402.72 and 48.32 mg kg–1, respectively). There was no significant difference in MBC or MBN between the fallow and sweet clover fields. Both MBC and MBN decreased with soil depth in all three treatments (Figures 3A,B).
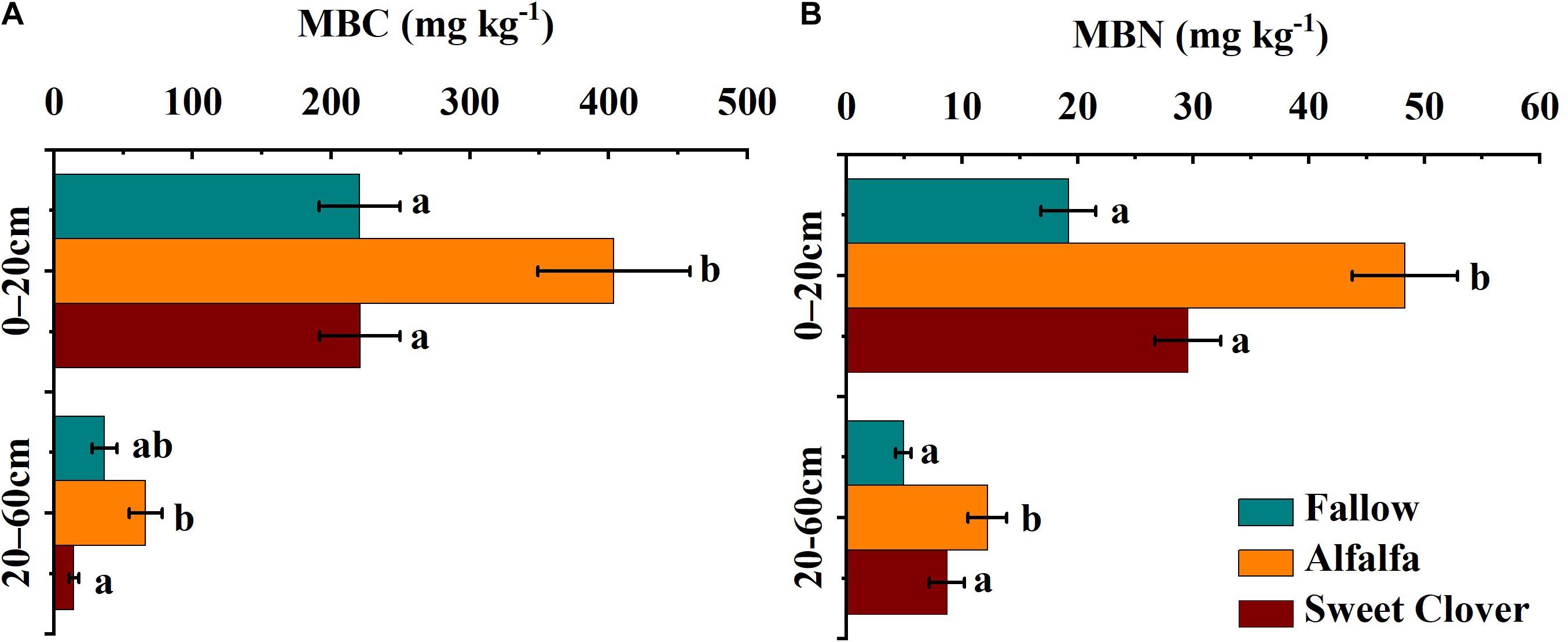
Figure 3. MBC (A), and MBN (B) distributions at different soil depths under different revegetation methods in 2019 (17 years after revegetation). Data represents the mean value ± standard error (n = 9), and the different letters indicate significant differences at the p < 0.05 level. (MBC, microbial biomass carbon; MBN, microbial biomass nitrogen).
The Relationship Between Root Biomass and Soil Properties
There were significant positive correlations between soil nutrients and root biomass density (p < 0.05), while there was a negative correlation between soil NH4+-N and root biomass density (r = −0.25, p = 0.07) (Figure 4). The SEM showed that the organic matter of the soil substrates could affect the available nutrients (AP and TIN) and further affect TN and TP by affecting the MBC and MBN. On the other hand, the AP and TIN directly affected the belowground biomass. Together, these indicators explained 44% of the belowground variance in root biomass. In addition, MBN had a direct effect on the root N concentration. MBC indirectly affected the belowground root biomass by affecting AP, further affecting root C and N concentrations (Figure 5).
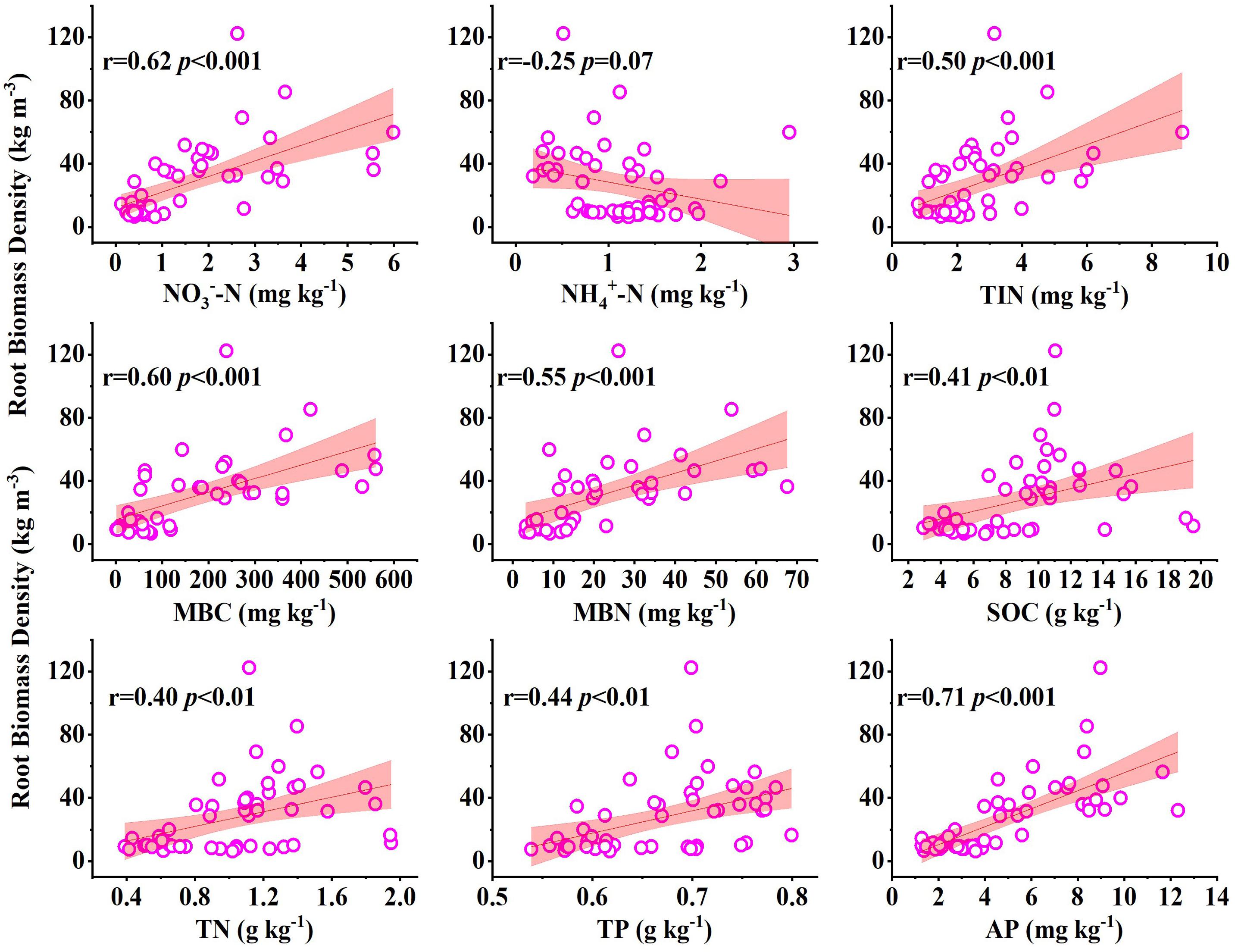
Figure 4. Relationships of root biomass density with soil properties (data were collected in 2019, 17 years after revegetation). NO3–-N, nitrate nitrogen; NH4+-N, ammonium nitrogen; TIN, total inorganic nitrogen; MBC, microbial biomass carbon; MBN, microbial biomass nitrogen; SOC, soil organic carbon; TN, total nitrogen; TP, total phosphorus; AP, available phosphorus.
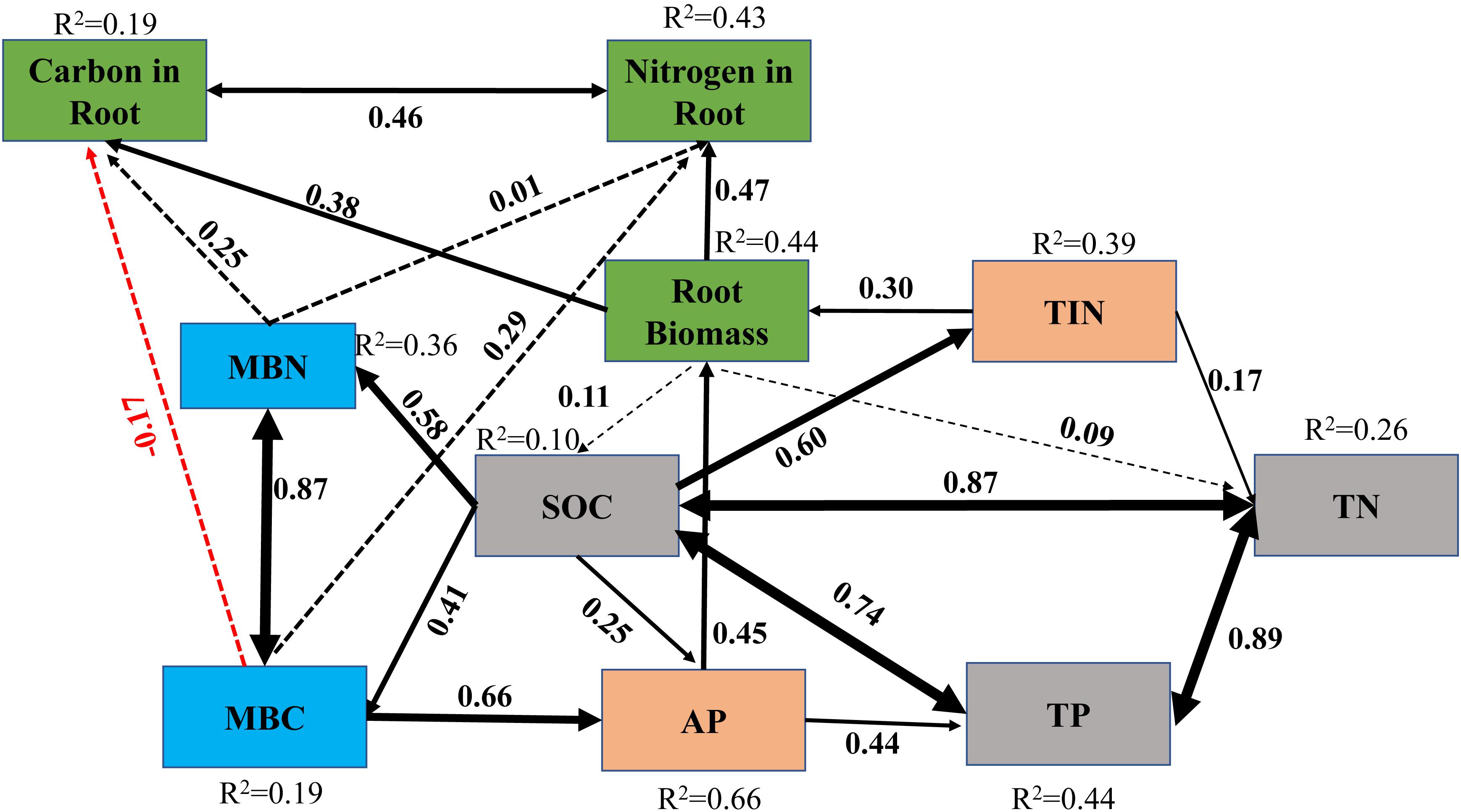
Figure 5. Structural equation model (SEM) showing the relationship between soil total nutrients, mineralized nutrients, microbial composition, and root characteristics (Df = 23, NFI = 0.952, CFI = 0.999, RMSEA = 0.022, p = 0.427). All variables are observed variables. Solid and dashed lines represent significant and non-significant pathways, respectively. Single-headed arrows represent the direction of causality and double-headed arrows indicate correlations between variables. The numbers adjacent to the arrows are standardized path coefficients. The proportion of variance explained (R2) is shown alongside each response variable (data were collected in 2019, 17 years after revegetation). TIN, total inorganic nitrogen; MBC, microbial biomass carbon; MBN, microbial biomass nitrogen; SOC, soil organic carbon; TN, total nitrogen; TP, total phosphorus; AP, available phosphorus.
Discussion
Our first hypothesis that legume introduction would increase the root biomass of communities and the soil MBC and MBN concentrations, leading to an increase in the TIN and AP concentrations was supported by the results under alfalfa fields. After 17 years, alfalfa introduction significantly increased root biomass, which was in line with previous reports of aboveground biomass (Yuan et al., 2016a). The root system plays an essential role in changing SOC and N storage following land use change (Fornara and Tilman, 2008; Cong et al., 2015). Alfalfa is a perennial leguminous plant, with a large root biomass density (Figure 1A) (Honde et al., 2020), increasing the C input to soil. Soil organic matter is mineralized by microorganisms to form mineral nutrients (Pearce et al., 2015). In semi-arid areas, organic matter is beneficial to water retention and soil permeability, which could provide more suitable environmental conditions for microbial activities. Available phosphorus and TIN were the main factors affecting root biomass (Figure 5). In addition, there was competition between microorganisms and plant roots for the acquisition of soil nutrients (Hodge et al., 2000; Winkler et al., 2010), which could affect the root C and N concentrations. Microorganisms could decompose plant roots (Kusliene et al., 2014; Lupascu et al., 2014), releasing nutrients into the soil (de Kroon et al., 2012). An increase in the supply of organic substrates may alter the competitive relationship between different microbial functional groups (Zak et al., 2003; Waldrop et al., 2006). In this experiment, the highest MBC and MBN was observed in the alfalfa field (Figures 3A,B), indicating that the microorganisms in the alfalfa field were more active. There was a significant positive correlation between the microbial index (MBC and MBN) and soil properties (SOC and TN) (Figure 6), implying that microbial biomass is one of the sources of soil nutrient elements. Therefore, microbial activity is the driving factor that mediates the plant-soil relationship in this region and regulates the soil biogeochemical cycling process. It plays an important role in the mineralization of soil C and N.
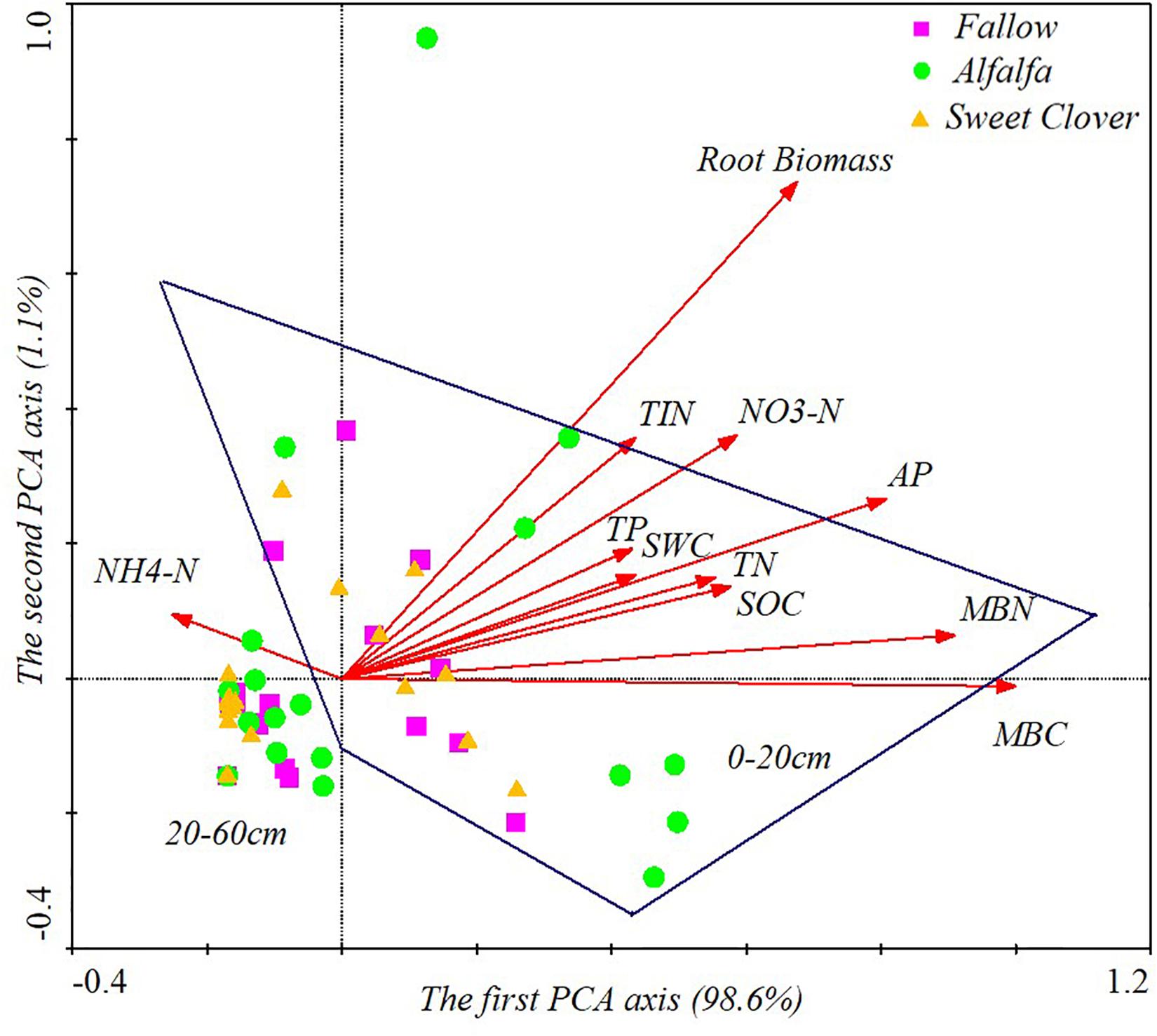
Figure 6. Principal component analysis (PCA) results, showing the relationships for root biomass with soil properties in different soil layers (data were collected in 2019, 17 years after revegetation). NO3–-N, nitrate nitrogen; NH4+-N, ammonium nitrogen; TIN, total inorganic nitrogen; MBC, microbial biomass carbon; MBN, microbial biomass nitrogen; SOC, soil organic carbon; TN, total nitrogen; TP, total phosphorus; AP, available phosphorus.
The second hypothesis that legume introduction would promote soil C and N accumulation was supported in alfalfa fields, and legumes introduction have no effect on soil TP was supported by the results only in sweet clover fields. Consistent with our hypothesis, the introduction of legumes (alfalfa) increased the SOC and TN concentrations, which was in agreement with previous research results (Post and Kwon, 2000; Guo and Gifford, 2002; Wang et al., 2015; Han et al., 2018). In our study, the highest SOC concentration was observed in both the 0–20 and 20–60 cm layers after alfalfa introduction, followed by sweet clover and fallow (Figure 2A). Revegetation after farmland abandonment can rapidly improve vegetation coverage (Zheng et al., 2019), which is beneficial to soil erosion control on sloping cropland. Vegetation restoration can also promote the input of litter and root exudates (Zhang et al., 2011), which are conducive to an increase in soil C storage. A “priming effect” on the respiration of microorganisms has been observed with an increase in root biomass; thus, promoting the decomposition of the old SOC (Kuzyakov et al., 2000; Fontaine et al., 2003; Kuzyakov, 2010). In this experiment, the “priming effect” was not taken into consideration, and it should therefore be further studied. The increase in root biomass could also lead to a higher root respiration rate (Luo et al., 1996; Pregitzer et al., 2008), inducing a decline in soil C storage. The combined effect of these changes in the belowground C cycle eventually led to more C storage in soils following alfalfa introduction (Figure 2A), which implies that the loss of SOC caused by the “priming effect” and respiration may be compensated for by the C input. Changes in SOC may therefore reflect the balance of accumulation and degradation rates (Bevivino et al., 2014).
The highest TN was also observed in both the 0–20 and 20–60 cm layers in the alfalfa field, followed by the sweet clover and fallow fields. Both alfalfa and sweet clover are legume species, capable of N-fixation (Sinclair and Serraj, 1995; Carlsson and Huss-Danell, 2003); therefore, improving N storage. However, sweet clover is a biennial plant and would therefore disappear from the plant communities after its 2-year lifespan (Li et al., 1996). Compared with the perennial alfalfa, the N-fixation effect of sweet clover may be lower in the long-term. This explains the lower TN concentration that was observed in the sweet clover fields (Figure 2B). The increase in the amount of N-fixing microorganisms could lead to a high TN concentration in alfalfa fields, alleviating the N limitation of the ecosystem (Wei et al., 2019). Alfalfa roots had a high N concentration (Figure 1C), and when they decompose it would be rapidly released (McLaren and Turkington, 2010; Makkonen et al., 2012). The belowground environment in alfalfa fields could provide opportunities for decomposers to obtain water, organic matter, and mineralized N, which would increase the release of NO3–-N and NH4+-N by decomposition of the root system (Silver and Miya, 2001; Mulder et al., 2002; Mueller et al., 2013). The alfalfa field had a higher soil NO3–-N concentration in the 0–20 and 20–60 cm layers than the other two treatments (Figure 2E), but there was a lower NH4+-N concentration in the 0–20 cm layer (Figure 2F). On the one hand, the low soil moisture content and high porosity in the semi-arid area of the study site provided a strong aerobic environment for the conversion of NH4+-N to NO3–-N through nitrification (Wei et al., 2019). On the other hand, the roots in the 0–20 cm layer had a high mineralized N utilization rate, which was mainly NH4+-N (Geisseler et al., 2010). Therefore, legume introduction would significantly increase soil NO3–-N, but not soil NH4+-N. The large root biomass in the alfalfa field would be beneficial to soil C input and N fixation, reducing the net N mineralization rate, but the available N released by fine roots could reduce the inhibitory effect on the N cycle induced by the increased root biomass (Fornara et al., 2009). Furthermore, the fine root turnover of leguminous plants is higher than that of C4 grasses (Fornara and Tilman, 2008). Therefore, alfalfa may have the potential to improve the N cycle.
The TP concentration increased in the 0–20 and 20–60 cm layers in the alfalfa field (Figure 2C). This result does not support our second hypothesis. This may be associated with P utilization in deep soil layers (He and Li, 2016; Zhou et al., 2018). Alfalfa has a strong and deep root system, which can utilize P even more than 5 m belowground. In addition, the root could be considered a “nutrient pump” (Farley and Kelly, 2004; Jobbagy and Jackson, 2004), promoting the transfer of P in the deep soil layer to the surface soil layer through residue inputs in the alfalfa field. Soil microorganisms and plant roots can therefore effect P transformation and then influence the P availability (Richardson, 2001). Microorganisms not only store immobilized P but can also provide inorganic or organic forms of P for plant roots by dissolving and mineralizing soil TP (Richardson, 2001). Phosphorus in humic-metal complexes could be mobilized by organic anions, inducing an increase in soil P availability (Gerke, 1993). Microorganisms play an important role in rapidly metabolizing various organic anions or releasing them through their own traits (Jones, 1998). Roots can release acid substances and decrease the soil pH (Phillips et al., 2004; Gu et al., 2018). Microorganisms, such as fungi, could enhance the area of plant roots, which is beneficial to the release of acid substances and uptake of mobilized P (Solaiman and Abbott, 2003). In alfalfa grassland, the soil calcium carbonate concentration is low (Gu et al., 2018), therefore the release of acidic substances by plants and microorganisms would lead to low pH which is conducive to the activation of TP. Thus, the increased AP concentration after alfalfa introduction might be due to rhizosphere acidification.
Conclusion
The long-term (17-years) effects of legume introduction on the root biomass and soil C, N, and P cycles were investigated. After 17 years, the soil C, N, and P concentrations were enriched in the 0–20 and 20–60 cm layers of the alfalfa field compared with sweet clover and fallow fields. This was induced by the increase in root biomass and soil microbial biomass. Alfalfa introduction promoted the accumulation of soil organic matter and soil organic matter mineralization. Therefore, Alfalfa introduction is a low-cost and rapid revegetation method to enhance the soil C, N, and P pool, enabling sustainable land use in the semi-arid areas of the Loess Plateau.
Data Availability Statement
The original contributions generated for this study are included in the article/Supplementary Material, further inquiries can be directed to the corresponding author.
Author Contributions
XS, CF, and F-ML designed the experiments. XS performed the experiments. XS, CF, Z-QY, and F-ML jointly analyzed data and wrote the manuscript. All authors contributed to the article and approved the submitted version.
Funding
This work was supported by the National Natural Science Foundation of China (31470496) and the ‘111’ Programme (BP0719040).
Conflict of Interest
The authors declare that the research was conducted in the absence of any commercial or financial relationships that could be construed as a potential conflict of interest.
Acknowledgments
We thank International Science Editing (http://www.internationalscienceediting.com) for editing this manuscript.
Supplementary Material
The Supplementary Material for this article can be found online at: https://www.frontiersin.org/articles/10.3389/fenvs.2021.649346/full#supplementary-material
References
An, S. S., Cheng, Y., Huang, Y. M., and Liu, D. (2013). Effects of revegetation on soil microbial biomass, enzyme activities, and nutrient cycling on the Loess Plateau in China. Restor. Ecol. 21, 600–607. doi: 10.1111/j.1526-100X.2012.00941.x
Azziz, G., Bajsa, N., Haghjou, T., Taule, C., Valverde, A., Igual, J. M., et al. (2012). Abundance, diversity and prospecting of culturable phosphate solubilizing bacteria on soils under crop-pasture rotations in a no-tillage regime in Uruguay. Appl. Soil Ecol. 61, 320–326. doi: 10.1016/j.apsoil.2011.10.004
Beck, T., Joergensen, R. G., Kandeler, E., Makeschin, F., Nuss, E., Oberholzer, H. R., et al. (1997). An inter-laboratory comparison of ten different ways of measuring soil microbial biomass C. Soil Biol. Biochem. 29, 1023–1032. doi: 10.1016/S0038-0717(97)00030-8
Bevivino, A., Paganin, P., Bacci, G., Florio, A., Pellicer, M. S., Papaleo, M. C., et al. (2014). Soil bacterial community response to differences in agricultural management along with seasonal changes in a Mediterranean region. PLoS One 9:e105515. doi: 10.1371/journal.pone.0105515
Boyrahmadi, M., and Raiesi, F. (2018). Plant roots and species moderate the salinity effect on microbial respiration, biomass, and enzyme activities in a sandy clay soil. Biol. Fertil. Soils 54, 509–521. doi: 10.1007/s00374-018-1277-6
Bremner, J. M., and Mulvaney, C. S. (1982). “Methods of soil analysis,” in Nitrogen-Total., Part 2, Chemical and Microbiological Properties, eds A. L. Page, R. H. Millar, and D. R. Keneey, (Wisconsin, WI: Madison), 595–624.
Brookes, P. C., Landman, A., Pruden, G., and Jenkinson, D. S. (1985). Chloroform fumigation and the release of soil-nitrogen - a rapid direct extraction method to measure microbial biomass nitrogen in soil. Soil Biol. Biochem. 17, 837–842. doi: 10.1016/0038-0717(85)90144-0
Campbell, C. D., Grayston, S. J., and Hirst, D. J. (1997). Use of rhizosphere carbon sources in sole carbon source tests to discriminate soil microbial communities. J. Microbiol. Methods 30, 33–41. doi: 10.1016/S0167-7012(97)00041-9
Carlsson, G., and Huss-Danell, K. (2003). Nitrogen fixation in perennial forage legumes in the field. Plant Soil 253, 353–372. doi: 10.1023/A:1024847017371
Chen, L. D., Wei, W., Fu, B. J., and Lu, Y. H. (2007). Soil and water conservation on the Loess Plateau in China: review and perspective. Prog. Phys. Geogr. 31, 389–403. doi: 10.1177/0309133307081290
Classen, A. T., Sundqvist, M. K., Henning, J. A., Newman, G. S., Moore, J. A. M., Cregger, M. A., et al. (2015). Direct and indirect effects of climate change on soil microbial and soil microbial-plant interactions: what lies ahead? Ecosphere 6:130.
Cong, W. F., Hoffland, E., Li, L., Six, J., Sun, J. H., Bao, X. G., et al. (2015). Intercropping enhances soil carbon and nitrogen. Glob. Change Biol. 21, 1715–1726. doi: 10.1111/gcb.12738
de Kroon, H., Hendriks, M., van Ruijven, J., Ravenek, J., Padilla, F. M., Jongejans, E., et al. (2012). Root responses to nutrients and soil biota: drivers of species coexistence and ecosystem productivity. J. Ecol. 100, 6–15. doi: 10.1111/j.1365-2745.2011.01906.x
Deng, L., Liu, G. B., and Shangguan, Z. P. (2014). Land-use conversion and changing soil carbon stocks in China’s ‘Grain-for-Green’ program: a synthesis. Glob. Change Biol. 20, 3544–3556. doi: 10.1111/gcb.12508
Deng, L., Wang, G. L., Liu, G. B., and Shangguan, Z. P. (2016). Effects of age and land-use changes on soil carbon and nitrogen sequestrations following cropland abandonment on the Loess Plateau. China. Ecol. Eng. 90, 105–112. doi: 10.1016/j.ecoleng.2016.01.086
Elser, J. J., Fagan, W. F., Denno, R. F., Dobberfuhl, D. R., Folarin, A., Huberty, A., et al. (2000). Nutritional constraints in terrestrial and freshwater food webs. Nature 408, 578–580. doi: 10.1038/35046058
Fang, C., Li, F.-M., Pei, J., Ren, J., Gong, Y., Yuan, Z., et al. (2018). Impacts of warming and nitrogen addition on soil autotrophic and heterotrophic respiration in a semi-arid environment. Agricul. Forest Meteorol. 248, 449–457. doi: 10.1016/j.agrformet.2017.10.032
Fang, C., Ye, J.-S., Gong, Y., Pei, J., Yuan, Z., Xie, C., et al. (2017). Seasonal responses of soil respiration to warming and nitrogen addition in a semi-arid alfalfa-pasture of the Loess Plateau, China. Sci. Total Environ. 59, 729–738. doi: 10.1016/j.scitotenv.2017.03.034
Farley, K. A., and Kelly, E. F. (2004). Effects of afforestation of a paramo grassland on soil nutrient status. Agric. For. Meteorol. 195, 281–290. doi: 10.1016/j.foreco.2003.12.015
Feng, Z. M., Yang, Y. Z., Zhang, Y. Q., Zhang, P. T., and Li, Y. Q. (2005). Grain-for-green policy and its impacts on grain supply in West China. Land Use Pol. 22, 301–312. doi: 10.1016/j.landusepol.2004.05.004
Fontaine, S., Mariotti, A., and Abbadie, L. (2003). The priming effect of organic matter: a question of microbial competition? Soil Biol. Biochem. 35, 837–843. doi: 10.1016/S0038-0717(03)00123-8
Fornara, D. A., and Tilman, D. (2008). Plant functional composition influences rates of soil carbon and nitrogen accumulation. J. Ecol. 96, 314–322. doi: 10.1111/j.1365-2745.2007.01345.x
Fornara, D. A., Tilman, D., and Hobbie, S. E. (2009). Linkages between plant functional composition, fine root processes and potential soil N mineralization rates. J. Ecol. 97, 48–56. doi: 10.1111/j.1365-2745.2008.01453.x
Frasier, I., Quiroga, A., Fernandez, R., Alvarez, C., Gomez, F., Scherger, E., et al. (2019). Soil type, land-use and -management as drivers of root-C inputs and soil C storage in the semi-arid pampa region. Argentina. Soil Tillage Res. 192, 134–143. doi: 10.1016/j.still.2019.05.010
Fu, B. J. (1989). Soil-erosion and its control in the Loess Plateau of China. Soil Use Manage. 5, 76–82. doi: 10.1111/j.1475-2743.1989.tb00765.x
Fu, B. J., Liu, Y., Lu, Y. H., He, C. S., Zeng, Y., and Wu, B. F. (2011). Assessing the soil erosion control service of ecosystems change in the Loess Plateau of China. Ecol. Complex. 8, 284–293. doi: 10.1016/j.ecocom.2011.07.003
Fujii, K., Sukartiningsih, Hayakawa, C., Inagaki, Y., and Kosaki, T. (2020). Effects of land use change on turnover and storage of soil organic matter in a tropical forest. Plant Soil 446, 425–439. doi: 10.1007/s11104-019-04367-5
Gale, W. J., Cambardella, C. A., and Bailey, T. B. (2000). Root-derived carbon and the formation and stabilization of aggregates. Soil Sci. Soc. Am. J. 64, 201–207. doi: 10.2136/sssaj2000.641201x
Geisseler, D., Horwath, W. R., Joergensen, R. G., and Ludwig, B. (2010). Pathways of nitrogen utilization by soil microorganisms - a review. Soil Biol. Biochem. 42, 2058–2067. doi: 10.1016/j.soilbio.2010.08.021
Gerke, J. (1993). Solubilization of Fe(Iii) from Humic-Fe complexes, Humic Fe-Oxide Mixtures and from poorly ordered Fe-Oxide by Organic-Acids - consequences for P-Adsorption. Zeitschrift Fur Pflanzenernahrung Und Bodenkunde 156, 253–257. doi: 10.1002/jpln.19931560311
Gong, Y. H., Zhao, D. M., Ke, W. B., Fang, C., Pei, J. Y., Sun, G. J., et al. (2020). Legacy effects of precipitation amount and frequency on the aboveground plant biomass of a semi-arid grassland. Sci. Total Environ. 705:135899. doi: 10.1016/j.scitotenv.2019.135899
Gransee, A. (2001). “Effects of root exudates on nutrient availability in the rhizosphere,” in Plant Nutrition. Developments in Plant and Soil Sciences, Vol. 92, ed. W. J. Horst, (Dordrecht: Springer), doi: 10.1007/0-306-47624-X_303
Gu, Y. J., Han, C. L., Fan, J. W., Shi, X. P., Kong, M., Shi, X. Y., et al. (2018). Alfalfa forage yield, soil water and P availability in response to plastic film mulch and P fertilization in a semi-arid environment. Field Crop. Res. 215, 94–103. doi: 10.1016/j.fcr.2017.10.010
Guo, L. B., and Gifford, R. M. (2002). Soil carbon stocks and land use change: a meta analysis. Glob. Change Biol. 8, 345–360. doi: 10.1046/j.1354-1013.2002.00486.x
Han, C. L., Gu, Y. J., Kong, M., Hu, L.-W., Jia, Y., Li, F. M., et al. (2018). Responses of soil microorganisms, carbon and nitrogen to freeze thaw cycles in diverse land-use types. Appl. Soil Ecol. 124, 211–217. doi: 10.1016/j.apsoil.2017.11.012
He, J. L., and Li, X. G. (2016). Potentilla fruticosa has a greater capacity to translocate phosphorus from the lower to upper soils than herbaceous grasses in an alpine meadow. Agric. Ecosyst. Environ. 228, 19–29. doi: 10.1016/j.agee.2016.04.021
He, J. S., Fang, J., Wang, Z., Guo, D., Flynn, D. F., and Geng, Z. (2006). Stoichiometry and large-scale patterns of leaf carbon and nitrogen in the grassland biomes of China. Oecologia 149, 115–122. doi: 10.1007/s00442-006-0425-0
Hodge, A., Robinson, D., and Fitter, A. (2000). Are microorganisms more effective than plants at competing for nitrogen? Trends Plant Sci. 5, 304–308. doi: 10.1016/S1360-1385(00)01656-3
Hoelzle, T. B., Jonas, J. L., and Paschke, M. W. (2012). Twenty-five years of sagebrush steppe plant community development following seed addition. J. Appl. Ecol. 49, 911–918. doi: 10.1111/j.1365-2664.2012.02154.x
Honde, S., Thivierge, M. N., Fort, F., Belanger, G., Chantigny, M. H., Angers, D. A., et al. (2020). Root growth and turnover in perennial forages as affected by management systems and soil depth. Plant Soil 451, 371–387. doi: 10.1007/s11104-020-04532-1
Hu, G. Z., Liu, H. Y., Yin, Y., and Song, Z. L. (2016). The role of legumes in plant community succession of degraded grasslands in Northern China. Land Degrad. Dev. 27, 366–372. doi: 10.1002/ldr.2382
Jackson, R. B., Lajtha, K., Crow, S. E., Hugelius, G., Kramer, M. G., and Pineiro, G. (2017). The ecology of soil carbon: pools, vulnerabilities, and biotic and abiotic controls. Ann. Rev. Ecol. Evol. Systemat. 48, 419–445. doi: 10.1146/annurev-ecolsys-112414-054234
Jobbagy, E. G., and Jackson, R. B. (2004). The uplift of soil nutrients by plants: biogeochemical consequences across scales. Ecology 85, 2380–2389. doi: 10.1890/03-0245
Jones, D. L. (1998). Organic acids in the rhizosphere - a critical review. Plant Soil 205, 25–44. doi: 10.1023/A:1004356007312
Kiss, S., Dragan-Bularda, M., and Radulescu, D. (1975). Biological significance of enzymes accumulated in soil. Adv. Agron. 27, 25–87. doi: 10.1016/S0065-2113(08)70007-5
Knops, J. M. H., and Tilman, D. (2000). Dynamics of soil nitrogen and carbon accumulation for 61 years after agricultural abandonment. Ecology 81, 88–98.
Kong, A. Y. Y., and Six, J. (2010). Tracing root vs. residue carbon into soils from conventional and alternative cropping systems. Soil Sci. Soc. Am. J. 74, 1201–1210. doi: 10.2136/sssaj2009.0346
Kusliene, G., Rasmussen, J., Kuzyakov, Y., and Eriksen, J. (2014). Medium-term response of microbial community to rhizodeposits of white clover and ryegrass and tracing of active processes induced by C-13 and N-15 labelled exudates. Soil Biol. Biochem. 76, 22–33. doi: 10.1016/j.soilbio.2014.05.003
Kuzyakov, Y. (2010). Priming effects: interactions between living and dead organic matter. Soil Biol. Biochem. 42, 1363–1371. doi: 10.1016/j.soilbio.2010.04.003
Kuzyakov, Y., Friedel, J. K., and Stahr, K. (2000). Review of mechanisms and quantification of priming effects. Soil Biol. Biochem. 32, 1485–1498. doi: 10.1016/S0038-0717(00)00084-5
Lal, R. (2004). Soil carbon sequestration impacts on global climate change and food security. Science 304, 1623–1627. doi: 10.1126/science.1097396
Li, R., Volenec, J. J., Joern, B. C., and Cunningham, S. M. (1996). Seasonal changes in nonstructural carbohydrates, protein, and macronutrients in roots of alfalfa, red clover, sweetclover, and birdsfoot trefoil. Crop Sci. 36, 617–623. doi: 10.2135/cropsci1996.0011183X003600030016x
Liu, B., Zhao, W. Z., Liu, Z. L., Yang, Y. T., Luo, W. C., Zhou, H., et al. (2015). Changes in species diversity, aboveground biomass, and vegetation cover along an afforestation successional gradient in a semi-arid desert steppe of China. Ecol. Eng. 81, 301–311. doi: 10.1016/j.ecoleng.2015.04.014
Liu, W., and Sang, T. (2013). Potential productivity of the Miscanthus energy crop in the Loess Plateau of China under climate change. Environ. Res. Lett. 8:044003. doi: 10.1088/1748-9326/8/4/044003
Luo, Y. Q., Jackson, R. B., Field, C. B., and Mooney, H. A. (1996). Elevated CO2 increases belowground respiration in California grasslands. Oecologia 108, 130–137. doi: 10.1007/Bf00333224
Lupascu, M., Welker, J. M., Seibt, U., Xu, X., Velicogna, I., Lindsey, D. S., et al. (2014). The amount and timing of precipitation control the magnitude, seasonality and sources (C-14) of ecosystem respiration in a polar semi-desert, northwestern Greenland. Biogeosciences 11, 4289–4304. doi: 10.5194/bg-11-4289-2014
Makkonen, M., Berg, M. P., Handa, I. T., Hattenschwiler, S., van Ruijven, J., van Bodegom, P. M., et al. (2012). Highly consistent effects of plant litter identity and functional traits on decomposition across a latitudinal gradient. Ecol. Lett. 15, 1033–1041. doi: 10.1111/j.1461-0248.2012.01826.x
McLaren, J. R., and Turkington, R. (2010). Plant functional group identity differentially affects leaf and root decomposition. Glob. Change Biol. 16, 3075–3084. doi: 10.1111/j.1365-2486.2009.02151.x
Melillo, J. M., Steudler, P. A., Aber, J. D., Newkirk, K., Lux, H., Bowles, F. P., et al. (2002). Soil warming and carbon-cycle feedbacks to the climate system. Science 298, 2173–2176. doi: 10.1126/science.1074153
Miao, C. Y., Sun, Q. H., Duan, Q. Y., and Wang, Y. F. (2016). Joint analysis of changes in temperature and precipitation on the Loess Plateau during the period 1961-2011. Clim. Dyn. 47, 3221–3234. doi: 10.1007/s00382-016-3022-x
Mokany, K., Raison, R. J., and Prokushkin, A. S. (2006). Critical analysis of root: shoot ratios in terrestrial biomes. Glob. Change Biol. 12, 84–96. doi: 10.1111/j.1365-2486.2005.001043.x
Mueller, K. E., Hobbie, S. E., Tilman, D., and Reich, P. B. (2013). Effects of plant diversity, N fertilization, and elevated carbon dioxide on grassland soil N cycling in a long-term experiment. Glob. Change Biol. 19, 1249–1261. doi: 10.1111/gcb.12096
Mulder, C. P. H., Jumpponen, A., Hogberg, P., and Huss-Danell, K. (2002). How plant diversity and legumes affect nitrogen dynamics in experimental grassland communities. Oecologia 133, 412–421. doi: 10.1007/s00442-002-1043-0
Nelson, D. W., and Sommers, L. E. (1982). “Methods of soil analysis,” in Part 2. Total Carbon, Organic Carbon and Organic Matter, eds A. L. Page, R. H. Miller, and D. R. Keeney, (Wisconsin: Madison), 539–579.
O’Halloran, I. P., and Cade-Menun, B. J. (2006). “Total and organic phosphorus,” in Soil Sampling and Methods of Analysis, 2nd Edn, eds M. R. Carter, and E. G. Gregorich, (Boca Raton FL: Canadian Society of Soil Science/CRC Press).
Olsen, S. R. (1954). Estimation of Available Phosphorus in Soils by Extraction with Sodium Bicarbonate. Washington, D.C: US Department of Agriculture.
Pandey, C. B., Singh, G. B., Singh, S. K., and Singh, R. K. (2010). Soil nitrogen and microbial biomass carbon dynamics in native forests and derived agricultural land uses in a humid tropical climate of india. Plant Soil 333, 453–467. doi: 10.1007/s11104-010-0362-x
Pearce, A. R., Rastetter, E. B., Kwiatkowski, B. L., Bowden, W. B., Mack, M. C., and Jiang, Y. (2015). Recovery of arctic tundra from thermal erosion disturbance is constrained by nutrient accumulation: a modeling analysis. Ecol. Appl. 25, 1271–1289. doi: 10.1890/14-1323.1
Phillips, D. A., Fox, T. C., King, M. D., Bhuvaneswari, T. V., and Teuber, L. R. (2004). Microbial products trigger amino acid exudation from plant roots. Plant Physiol. 136, 2887–2894. doi: 10.1104/pp.104.044222
Post, W. M., and Kwon, K. C. (2000). Soil carbon sequestration and land-use change: processes and potential. Glob. Change Biol. 6, 317–327. doi: 10.1046/j.1365-2486.2000.00308.x
Pransiska, Y., Triadiati, T., Tjitrosoedirjo, S., Hertel, D., and Kotowska, M. M. (2016). Forest conversion impacts on the fine and coarse root system, and soil organic matter in tropical lowlands of Sumatera (Indonesia). For. Ecol. Manag. 379, 288–298. doi: 10.1016/j.foreco.2016.07.038
Pregitzer, K. S., Burton, A. J., King, J. S., and Zak, D. R. (2008). Soil respiration, root biomass, and root turnover following long-term exposure of northern forests to elevated atmospheric Co-2 and tropospheric O-3. New Phytol. 180, 153–161. doi: 10.1111/j.1469-8137.2008.02564.x
Qiu, L. P., Wei, X. R., Zhang, X. C., Cheng, J. M., Gale, W., Guo, C., et al. (2012). Soil organic carbon losses due to land use change in a semi-arid grassland. Plant Soil 355, 299–309. doi: 10.1007/s11104-011-1099-x
Reed, S. C., Yang, X. J., and Thornton, P. E. (2015). Incorporating phosphorus cycling into global modeling efforts: a worthwhile, tractable endeavor. New Phytol. 208, 324–329. doi: 10.1111/nph.13521
Richardson, A. E. (2001). Prospects for using soil microorganisms to improve the acquisition of phosphorus by plants. Aus. J. Plant Physiol. 28, 897–906. doi: 10.1071/Pp01093
Rumpel, C., and Kogel-Knabner, I. (2011). Deep soil organic matter-a key but poorly understood component of terrestrial C cycle. Plant Soil 338, 143–158. doi: 10.1007/s11104-010-0391-5
Schilling, G., Gransee, A., Deubel, A., Lezovic, G., and Ruppel, S. (1998). Phosphorus availability, root exudates, and microbial activity in the rhizosphere. Zeitschrift Fur Pflanzenernahrung Und Bodenkunde 161, 465–478. doi: 10.1002/jpln.1998.3581610413
Schmidt, M. W. I., Torn, M. S., Abiven, S., Dittmar, T., Guggenberger, G., Janssens, I. A., et al. (2011). Persistence of soil organic matter as an ecosystem property. Nature 478, 49–56. doi: 10.1038/nature10386
Shi, Z., Liu, W., Guo, S. L., and Li, F. M. (2003). Moisture properties in soil profiles and their relation to landform at Zhonglianchuan small watershed. Agricul. Res. Arid Areas 21, 101–104.
Silver, W. L., and Miya, R. K. (2001). Global patterns in root decomposition: comparisons of climate and litter quality effects. Oecologia 129, 407–419. doi: 10.1007/s004420100740
Sinclair, T. R., and Serraj, R. (1995). Legume nitrogen-fixation and drought. Nature 378, 344–344. doi: 10.1038/378344a0
Singh, M. K., and Ghoshal, N. (2014). Variation in soil microbial biomass in the dry tropics: impact of land-use change. Soil Res. 52, 299–306. doi: 10.1071/SR13265
Six, J., Bossuyt, H., Degryze, S., and Denef, K. (2004). A history of research on the link between (micro)aggregates, soil biota, and soil organic matter dynamics. Soil Tillage Res. 79, 7–31. doi: 10.1016/j.still.2004.03.008
Six, J., Elliott, E. T., and Paustian, K. (2000). Soil macroaggregate turnover and microaggregate formation: a mechanism for C sequestration under no-tillage agriculture. Soil Biol. Biochem. 32, 2099–2103. doi: 10.1016/S0038-0717(00)00179-6
Sokol, N. W., and Bradford, M. A. (2019). Microbial formation of stable soil carbon is more efficient from belowground than aboveground input. Nat. Geosci. 12, 46–53. doi: 10.1038/s41561-018-0258-6
Solaiman, M. Z., and Abbott, L. K. (2003). Phosphorus uptake by a community of arbuscular mycorrhizal fungi in jarrah forest. Plant Soil 248, 313–320. doi: 10.1023/A:1022372228545
Thomas, R. L., Sheard, R. W., and Moyer, J. R. (1967). Comparison of conventional and automated procedures for nitrogen, phosphorus, and potassium analysis of plant material using a single digestion. Agron. J. 59, 240–243. doi: 10.2134/agronj1967.00021962005900030010x
Turner, B. L., Zemunik, G., Laliberte, E., Drake, J. J., Jones, F. A., and Saltonstall, K. (2019). Contrasting patterns of plant and microbial diversity during long-term ecosystem development. J. Ecol. 107, 606–621. doi: 10.1111/1365-2745.13127
van Groenigen, J. W., van Kessel, C., Hungate, B. A., Oenema, O., Powlson, D. S., and van Groenigen, K. J. (2017). Sequestering soil organic carbon: a nitrogen dilemma. Environ. Sci. Technol. 51, 4738–4739. doi: 10.1021/acs.est.7b01427
Vanveen, J. A., Merckx, R., and Vandegeijn, S. C. (1989). Plant and soil related controls of the flow of carbon from roots through the soil microbial biomass. Plant Soil 115, 179–188. doi: 10.1007/Bf02202586
Vitousek, P. M., Menge, D. N. L., Reed, S. C., and Cleveland, C. C. (2013). Biological nitrogen fixation: rates, patterns and ecological controls in terrestrial ecosystems. Philos. Trans. R. Soc. B-Biol. Sci. 368:20130119. doi: 10.1098/rstb.2013.0119
Vitousek, P. M., Porder, S., Houlton, B. Z., and Chadwick, O. A. (2010). Terrestrial phosphorus limitation: mechanisms, implications, and nitrogen-phosphorus interactions. Ecol. Appl. 20, 5–15. doi: 10.1890/08-0127.1
Waldrop, M. P., Zak, D. R., Blackwood, C. B., Curtis, C. D., and Tilman, D. (2006). Resource availability controls fungal diversity across a plant diversity gradient. Ecol. Lett. 9, 1127–1135. doi: 10.1111/j.1461-0248.2006.00965.x
Walker, T. W., and Syers, J. K. (1976). The fate of phosphorus during pedogenesis. Geoderma 15, 1–19. doi: 10.1016/0016-7061(76)90066-5
Wang, Z., Guo, S., Sun, Q., Li, N., Jiang, J., Wang, R., et al. (2015). Soil organic carbon sequestration potential of artificial and natural vegetation in the hilly regions of Loess Plateau. Ecol. Eng. 82, 547–554. doi: 10.1016/j.ecoleng.2015.05.031
Wei, X. R., Reich, P. B., and Hobbie, S. E. (2019). Legumes regulate grassland soil N cycling and its response to variation in species diversity and N supply but not CO2. Glob. Change Biol. 25, 2396–2409. doi: 10.1111/gcb.14636
Westheimer, F. H. (1987). Why nature chose phosphates. Science 235, 1173–1178. doi: 10.1126/science.2434996
Winkler, J. B., Dannenmann, M., Simon, J., Pena, R., Offermann, C., Sternad, W., et al. (2010). Carbon and nitrogen balance in beech roots under competitive pressure of soil-borne microorganisms induced by girdling, drought and glucose application. Funct. Plant Biol. 37, 879–889. doi: 10.1071/Fp09309
Yamashita, H., Sonobe, R., Hirono, Y., Morita, A., and Ikka, T. (2020). Dissection of hyperspectral reflectance to estimate nitrogen and chlorophyll contents in tea leaves based on machine learning algorithms. Sci. Rep. 10:17360. doi: 10.1038/s41598-020-73745-2
Yao, G.-Q., Nie, Z.-F., Turner, N. C., Li, F.-M., Gao, T.-P., Fang, X.-W., et al. (2020). Combined high leaf hydraulic safety and efficiency provides drought tolerance in Caragana species adapted to low mean annual precipitation. New Phytol. 229, 230–244. doi: 10.1111/nph.16845
Yu, Z. P., Wang, M. H., Huang, Z. Q., Lin, T. C., Vadeboncoeur, M. A., Searle, E. B., et al. (2018). Temporal changes in soil C-N-P stoichiometry over the past 60 years across subtropical China. Glob. Change Biol. 24, 1308–1320. doi: 10.1111/gcb.13939
Yuan, Z. Q., Epstein, H., and Li, F. M. (2015). Factors affecting the recovery of abandoned semi-arid fields after legume introduction on the Loess Plateau. Ecol. Eng. 79, 86–93. doi: 10.1016/j.ecoleng.2015.03.012
Yuan, Z.-Q., Fang, C., Zhang, R., Li, F.-M., Javaid, M. M., and Janssens, I. A. (2019). Topographic influences on soil properties and aboveground biomass in lucerne-rich vegetation in a semi-arid environment. Geoderma 344, 137–143. doi: 10.1016/j.geoderma.2019.03.003
Yuan, Z. Q., Yu, K. L., Epstein, H., Fang, C., Li, J. T., Liu, Q. Q., et al. (2016a). Effects of legume species introduction on vegetation and soil nutrient development on abandoned croplands in a semi-arid environment on the Loess Plateau, China. Sci. Total Environ. 541, 692–700. doi: 10.1016/j.scitotenv.2015.09.108
Yuan, Z. Q., Yu, K. L., Guan, X. K., Fang, C., Li, M., Shi, X. Y., et al. (2016b). Medicago sativa improves soil carbon sequestration following revegetation of degraded arable land in a semi-arid environment on the Loess Plateau, China. Agric. Ecosyst. Environ. 232, 93–100. doi: 10.1016/j.agee.2016.07.024
Zak, D. R., Holmes, W. E., White, D. C., Peacock, A. D., and Tilman, D. (2003). Plant diversity, soil microbial communities, and ecosystem function: are there any links? Ecology 84, 2042–2050. doi: 10.1890/02-0433
Zhang, C., Liu, G. B., Xue, S., and Song, Z. L. (2011). Rhizosphere soil microbial activity under different vegetation types on the Loess Plateau, China. Geoderma 161, 115–125. doi: 10.1016/j.geoderma.2010.12.003
Zhang, H., Wang, K. L., Zeng, Z. X., Du, H., and Zeng, F. P. (2017). Biomass and carbon sequestration by Juglans regia plantations in the Karst Regions of Southwest China. Forests 8:103. doi: 10.3390/f8040103
Zhao, G. J., Mu, X. M., Wen, Z. M., Wang, F., and Gao, P. (2013). Soil erosion, conservation, and eco-environment changes in the Loess Plateau of China. Land Degrad. Dev. 24, 499–510. doi: 10.1002/ldr.2246
Zheng, K., Wei, J. Z., Pei, J. Y., Cheng, H., Zhang, X. L., Huang, F. Q., et al. (2019). Impacts of climate change and human activities on grassland vegetation variation in the Chinese Loess Plateau. Sci. Total Environ. 660, 236–244. doi: 10.1016/j.scitotenv.2019.01.022
Zhou, J. Z., Xue, K., Xie, J. P., Deng, Y., Wu, L. Y., Cheng, X. H., et al. (2012). Microbial mediation of carbon-cycle feedbacks to climate warming. Nat. Clim. Chang. 2, 106–110. doi: 10.1038/Nclimate1331
Keywords: legumes, Medicago sativa L., land use change, plant nutrients, root biomass, soil properties
Citation: Song X, Fang C, Yuan Z-Q and Li F-M (2021) Long-Term Growth of Alfalfa Increased Soil Organic Matter Accumulation and Nutrient Mineralization in a Semi-Arid Environment. Front. Environ. Sci. 9:649346. doi: 10.3389/fenvs.2021.649346
Received: 04 January 2021; Accepted: 12 March 2021;
Published: 01 April 2021.
Edited by:
Ivika Ostonen, University of Tartu, EstoniaReviewed by:
Susan Tandy, Rothamsted International, United KingdomWenzhi Liu, Wuhan Botanical Garden, Chinese Academy of Sciences, China
Copyright © 2021 Song, Fang, Yuan and Li. This is an open-access article distributed under the terms of the Creative Commons Attribution License (CC BY). The use, distribution or reproduction in other forums is permitted, provided the original author(s) and the copyright owner(s) are credited and that the original publication in this journal is cited, in accordance with accepted academic practice. No use, distribution or reproduction is permitted which does not comply with these terms.
*Correspondence: Feng-Min Li, Zm1saUBsenUuZWR1LmNu
†These authors have contributed equally to this work and share first authorship