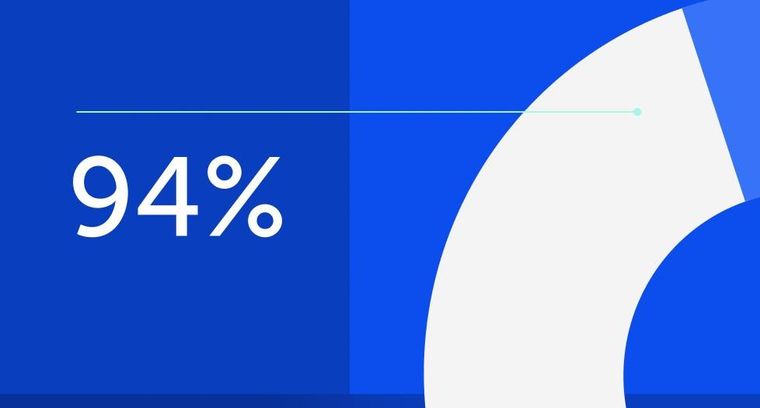
94% of researchers rate our articles as excellent or good
Learn more about the work of our research integrity team to safeguard the quality of each article we publish.
Find out more
REVIEW article
Front. Environ. Sci., 04 May 2021
Sec. Soil Processes
Volume 9 - 2021 | https://doi.org/10.3389/fenvs.2021.643847
This article is part of the Research TopicFrontiers in Environmental Science – Editor’s Picks 2021View all 25 articles
Agricultural pesticide use and its associated environmental harms is widespread throughout much of the world. Efforts to mitigate this harm have largely been focused on reducing pesticide contamination of the water and air, as runoff and pesticide drift are the most significant sources of offsite pesticide movement. Yet pesticide contamination of the soil can also result in environmental harm. Pesticides are often applied directly to soil as drenches and granules and increasingly in the form of seed coatings, making it important to understand how pesticides impact soil ecosystems. Soils contain an abundance of biologically diverse organisms that perform many important functions such as nutrient cycling, soil structure maintenance, carbon transformation, and the regulation of pests and diseases. Many terrestrial invertebrates have declined in recent decades. Habitat loss and agrichemical pollution due to agricultural intensification have been identified as major driving factors. Here, we review nearly 400 studies on the effects of pesticides on non-target invertebrates that have egg, larval, or immature development in the soil. This review encompasses 275 unique species, taxa or combined taxa of soil organisms and 284 different pesticide active ingredients or unique mixtures of active ingredients. We identified and extracted relevant data in relation to the following endpoints: mortality, abundance, biomass, behavior, reproduction, biochemical biomarkers, growth, richness and diversity, and structural changes. This resulted in an analysis of over 2,800 separate “tested parameters,” measured as a change in a specific endpoint following exposure of a specific organism to a specific pesticide. We found that 70.5% of tested parameters showed negative effects, whereas 1.4% and 28.1% of tested parameters showed positive or no significant effects from pesticide exposure, respectively. In addition, we discuss general effect trends among pesticide classes, taxa, and endpoints, as well as data gaps. Our review indicates that pesticides of all types pose a clear hazard to soil invertebrates. Negative effects are evident in both lab and field studies, across all studied pesticide classes, and in a wide variety of soil organisms and endpoints. The prevalence of negative effects in our results underscores the need for soil organisms to be represented in any risk analysis of a pesticide that has the potential to contaminate soil, and for any significant risk to be mitigated in a way that will specifically reduce harm to soil organisms and to the many important ecosystem services they provide.
Soils are arguably the most complex and biodiverse ecosystems on earth, containing nearly a quarter of the planet’s diversity (Ram, 2019). A handful of soil contains an estimated 10 –100 million organisms belonging to over 5,000 taxa (Ramirez et al., 2015), only a small percentage of which have been described (Adams and Wall, 2000). A typical functional soil community is comprised of hundreds to thousands of species of macroinvertebrates and nematodes as well as a vast abundance of microorganisms, including hundreds of fungal and thousands of bacterial species (Bardgett and van der Putten, 2014; Ram, 2019; Singh et al., 2019).
Soil invertebrates perform a variety of different ecosystem services essential for agricultural sustainability. Soil biodiversity enables self-perpetuating ecosystem functions that fuel specialized processes such as soil structure maintenance, nutrient cycling, carbon transformations, and the regulation of pests and diseases (Balvanera et al., 2006; Perrings et al., 2006; Kibblewhite et al., 2008; Chagnon et al., 2015). Burrowing activity by soil organisms modifies soil porosity by increasing aeration, water infiltration and retention, and reducing compaction (Pisa et al., 2015; Ram, 2019). Earthworms alone can construct up to 8,900 km of channels per hectare, decreasing soil erosion by 50% via increased soil porosity and water infiltration (Blouin et al., 2013; Gaupp-Berghausen et al., 2015). Nutrients travel through multiple soil layers by means of foragers, tunnelers, and ground-nesting insects including beetles, ground-nesting bees, ants, and termites (Stork and Eggleton, 1992; Willis Chan et al., 2019), and detritivores like nematodes, springtails, earthworms, millipedes, and woodlice, transform decaying material and minerals into usable forms, cycle nutrients, and increase soil fertility (Stork and Eggleton, 1992; Kibblewhite et al., 2008; Ram, 2019). For example, nematodes and mites enable nitrogen mineralization by feeding on fungal roots and stimulating and regulating microbial activity (Stork and Eggleton, 1992). Dead invertebrates decay and add nitrogen to the soil (Stork and Eggleton, 1992). Soil invertebrates also form up to half of all soil aggregates by breaking down litter and releasing organically rich casts and feces (Stork and Eggleton, 1992). The formation of these large soil aggregates allows for greater soil carbon sequestration, thus these ecosystem engineers play a role in offsetting fossil fuel emissions and combating climate change (Lal, 2004a, b; Lavelle et al., 2006; Dirzo et al., 2014).
Many soil invertebrates also play a role in controlling agricultural pests. Nematodes and mites are used in targeting disease-related bacteria in crops (Stork and Eggleton, 1992; Kibblewhite et al., 2008; Ram, 2019). Predators and parasitoids, such as beetles and parasitic wasps prey on arthropods that interfere with crop production (Stork and Eggleton, 1992; Gill et al., 2016), and herbivorous soil insects can eat the seeds of undesirable plants selectively over crop seeds, reducing the spread of aggressive weeds (Honek et al., 2003).
Increases in land conversion and agricultural intensification accelerate the loss of soil biodiversity and, as a result, have contributed to the reduction of approximately 60% of soil ecosystem services (Díaz et al., 2006; Veresoglou et al., 2015; Singh et al., 2019). Many insects that depend on soil for portions of their life cycle, like ground beetles and ground-nesting bees, as well as terrestrial insects and mites in North America, have declined greatly in recent decades (Forister et al., 2019; Sánchez-Bayo and Wyckhuys, 2019; van Klink et al., 2020; Sullivan and Ozman-Sullivan, 2021). Habitat loss due to agricultural intensification and pollution, primarily from synthetic agricultural pesticides and fertilizers, are thought to be the major driving factors in recent insect declines and are an increasing threat (Hallmann et al., 2017; Forister et al., 2019; Seibold et al., 2019; Sánchez-Bayo and Wyckhuys, 2019; Miličić et al., 2020). In a 2019 survey of member countries of the Food and Agriculture Organization of the United Nations (FAO), overuse of chemical control mechanisms (e.g., pesticides, antibiotics, etc.) was identified as the most impactful practice that has been driving the loss of soil biodiversity in the last 10 years (FAO, 2020).
From 1992 to 2014, DiBartolomeis et al. found that increased use of neonicotinoid insecticides and the environmental persistence of those insecticides drove a 48- and 4-fold increase in oral and contact toxicity load, respectively, for insects in agricultural environments using the European honeybee Apis mellifera L. as a proxy species (DiBartolomeis et al., 2019). The study focused on honeybees because they are the most extensively studied non-target insect within United States agroecosystems; in fact, they are the only terrestrial invertebrate for which the United States Environmental Protection Agency (EPA) requires testing during pesticide registration (Legal Information Institute, 2020). However, the results of this study also signify an increasing threat to soil invertebrates. Neonicotinoid insecticides accounted for 92% of the increase in invertebrate toxicity loading (DiBartolomeis et al., 2019), and 60% of neonicotinoid use is through seed treatments and soil application as of 2011 (Jeschke et al., 2011). Neonicotinoid seed treatments are estimated to be used in over half of soybean acres and nearly all non-organic corn grown in the United States (Douglas and Tooker, 2015; Mourtzinis et al., 2019). Because 80% or more of the active ingredients from neonicotinoid seed treatments remain in the soil (Sur and Stork, 2003; Alford and Krupke, 2017) soil organisms in these systems are likely to be exposed to high doses of these insecticides.
Large-scale use of seed-applied fungicides presents another risk, as almost all United States corn is also treated with seed-applied fungicides (Lamichhane et al., 2019). In addition to the pesticides that are currently on the market, several new seed- and soil-applied pesticide active ingredients are currently going through the registration process in the United States, such as the pyrazolecarboxamide fungicide inpyrfluxam (U.S. EPA, 2020a), the diamide insecticide tetraniliprole (U.S. EPA, 2020d), and the novel insecticide broflanilide (U.S. EPA, 2020c).
The trend toward wider use of soil-applied pesticides — both individually and in combination with other active ingredients — will likely continue to grow. While soil organisms are at particular risk from exposure via direct soil applications, they can also be exposed to pesticides through other routes, such as drift from foliar sprays (Sánchez-Bayo, 2011), inclusion of pesticides in irrigation water (Sánchez-Bayo, 2011), or absorption of pesticides into plant tissue that eventually returns to the soil through senescence of crop residues (Doublet et al., 2009). As this threat to soil organisms increases, it is important to strive for a more complete understanding of the impacts of all pesticides on soil invertebrates.
The word “pesticide” is an umbrella term used to describe an agent that targets a pest — in plant agriculture a pest is defined as an organism that causes harm to crops through direct damage or competition for nutrients and water — and includes insecticides, herbicides, fungicides, and bactericides, among others. Most review papers assessing pesticide impacts to soil organisms have focused on the environmental risks of specific classes of pesticides (Biondi et al., 2012; Pisa et al., 2015; Douglas and Tooker, 2016; Wood and Goulson, 2017), specific taxa affected (da Silva Souza et al., 2014; Pelosi et al., 2014; Römbke et al., 2017), or have analyzed only lab data (Frampton et al., 2006) or field data (Jänsch et al., 2006), making it difficult to identify big-picture trends and hazards that are necessary to inform general pesticide policy. To the best of our knowledge, there has only been one comprehensive overview on the effects of pesticides on a wide variety of soil organisms (Puglisi, 2012), which focused on soil microorganisms, particularly bacteria and fungi. Here we present a review focused on how broad types of pesticides affect the health of soil invertebrates. Given the scope of such a review, special attention is also paid to general effect trends among pesticide classes, taxa, and endpoints, as well as data gaps that should be addressed. Considering the key ecosystem services performed by soil invertebrates and the increase in the use of seed- and soil-applied pesticides, one of our objectives is to understand the overall hazard that pesticides pose to soil invertebrates to inform whether they should be included in regulatory ecotoxicological risk assessment to ensure that environmental harm is adequately estimated.
We conducted a preliminary literature search using Google Scholar to assess the impacts of pesticides on soil organisms. We used the following search terms: (pesticide OR herbicide OR insecticide OR fungicide) AND (soil OR terrestrial OR non-target OR invertebrate) to identify review papers that could be mined for further resources. The soil taxa included in the corresponding bibliographies informed more specific search terms for a second round of literature search combining (pesticide OR herbicide OR insecticide OR fungicide, and the three bactericides approved for agriculture in the United States, streptomycin, kasugamycin, and oxytetracycline, were also used as keywords) with taxa-specific search terms. To ensure an exhaustive search, multiple, diverse strings of keyword searches were used in the following databases and journals between the dates of October 2019 and January 2020; Agricola, Agris, Wiley-Blackwell, Directory of Open Access Journals, Karger, PubMed, Nature, SpringerLink, Environmental Toxicology and Chemistry, Chemosphere, Ecotoxicology and Environmental Safety, Ecotoxicology, Applied Soil Ecology, European Journal of Soil Biology, Environmental Pollution, Soil and Tillage Research, Pesticide Biochemistry and Physiology, Science Direct/Elsevier, Jstor, PlosOne, Frontiers. The title and abstract of each identified paper were briefly scanned for relevance. From this we identified 1,028 studies. After further reviewing all abstracts, the number of studies was reduced to 394 (see Supplemental References). The majority of removed studies did not meet our inclusion criteria (outlined below) and a small number of studies could not be accessed, were not in English, or were not an original study. We separated studies conducted in a laboratory from those conducted in a field setting and present each as such. Some studies were conducted in both the laboratory and the field and, in those cases, the individual tested parameters in each study were designated as being done either in the laboratory or field.
For inclusion in this review, we established the following criteria: (1) The studied organism must be a soil-dwelling terrestrial invertebrate (see definition below) - aquatic organisms or terrestrial microbial organisms, such as bacteria and fungi, were not in the scope of our review; (2) The study must include soil-dwelling organisms that are not target invertebrates or common agricultural pests (for example, thrips, snails, or root feeding beetle larvae); (3) The pesticide studied must not be banned in the United States; and (4) The study must look at pesticide effects on non-target organism(s) with measurable endpoints. We did not include studies that focused on the behavior of pesticides, which includes the topics of sorption, transport, runoff, volatilization, degradation, dissipation, half-life, persistence, and leaching. While these interactions in the soil are necessary to understanding the environmental fate of pesticides, they are beyond the scope of this review. We also did not include studies that dealt with the efficacy of ecotoxicity testing methods, such as species-specific sensitivity, recommendations for test protocols, or new technologies for data collection.
Pesticides were limited to any agricultural insecticide, fungicide, herbicide, or bactericide that is not currently banned in the United States, using (Donley, 2019) to identify banned pesticides. This was done to exclude mainly legacy pesticides that are no longer subject to regulatory review in many parts of the world. We only included data on parent pesticide molecules and not their degradates or metabolites. We included studies done on formulations of pesticides as well as on individual active ingredients. Studies with two or more pesticide active ingredients applied together were categorized as mixtures and included in our analysis. Studies on fertilizers, genetically modified organisms, living pesticidal organisms, antibacterial agents not used in crop production, or any chemical not registered through the United States EPA as a pesticide were outside of the study scope. We only included studies on common veterinary medicines, such as abamectin, if the chemical was applied as a pesticide to plants and not directly to livestock. We included all soil studies on the antibiotics oxytetracycline, kasugamycin, and streptomycin, because they are the only antibiotics currently approved for agricultural application to crops in the United States.
We defined soil organisms as any non-target invertebrate that has egg, larval, or immature development in the soil (Paoletti and Purrington, 1991). We excluded taxa from our data when there was any uncertainty of the location of the egg, larval, and/or immature development of the organism. Many soil-associated invertebrates such as various species of Arachnids, Diptera, or Hymenoptera were therefore not included. In addition, no aquatic organisms or terrestrial microbial organisms such as bacteria and fungi were included. The soil organisms that fit our criteria from the relevant studies are organized by taxa in the Results section and include Oligochaeta (earthworms), Enchytraeidae (potworms), Nematoda (roundworms), Tardigrada (water bears), Acari (mites), Myriapoda (centipedes and millipedes), Isopoda (woodlice), Collembola (springtails), Protura (coneheads), Isoptera (termites), Coleoptera (beetles), Formicidae (ants), Bombus spp. (bumble bees), other ground-nesting bees, and parasitic wasps, as well as various combinations of these taxa studied together.
We identified and extracted the relevant tested parameters in each study. A “tested parameter” was defined as a unique combination of the following variables: pesticide, organism, and endpoint. All tested parameters measured a specific endpoint following exposure of a specific organism to a specific pesticide. For instance, if a study tested how three different pesticides (chlorpyrifos, imidacloprid, and permethrin) affected two endpoints (mortality and DNA damage) on one species (Caenorhabditis elegans), then we would be able to extract six unique tested parameters, any of which could result in a negative effect, positive effect or no significant effect to the tested species. To manageably organize the data, we distilled variables other than “pesticide,” “endpoint,” or “species” into one tested parameter as often as possible. In studies that analyzed multiple substrates, we only entered data from soil tests but also included studies if they only utilized surrogate substrates, such as filter paper. Similarly, if there were multiple time periods utilized to determine LC50 or EC50, we reported data from only the standard 48 h or seven days, whichever was relevant. If there were multiple soil types, temperatures, pesticide concentrations, or moisture contents, we extracted the full data range rather than separating each variable as its own tested parameter. For instance, if three different concentrations of chlorpyrifos were tested on C. elegans mortality, only one tested parameter was recorded. If any of the three concentrations caused a positive or negative effect on C. elegans mortality, then the tested parameter was categorized as having a positive or negative effect. If all three concentrations caused no significant effect, then it was categorized as having no effect.
We considered an effect negative or positive if the authors reported a statistically significant change from that of the control. If a study reported an effect on an endpoint over a time course, we considered the effect positive or negative if there was a significant effect in any of the tested times (e.g., earthworms significantly affected three out of ten days or one out of six years). An effect was considered “positive” if there was an unambiguous benefit to the organism from the associated effect, such as an increase in abundance or reproduction compared to control. All other significant changes were categorized as “negative.” Tested parameters that measured biochemical biomarker endpoints were always considered “negative” if there was any significant change compared to the control. A small minority of studies lacked statistical analyses. For instance, some studies used specific thresholds to determine significance (e.g., < 15% reduction in survival equaled no effect, or exposure exceeding 5% of LD50 was a negative effect). In these rare cases, we scored each tested parameter as “no effect,” “negative effect,” or “positive effect” based on the authors’ conclusions.
We classified tested endpoints into nine major categories that measured the following:
1. Mortality — survival and/or average lifespan.
2. Abundance — number of individuals and/or activity-density.
3. Biomass — weight of organisms.
4. Behavior — behavioral responses, including avoidance, mounding or burrowing activity, litter decomposition, food consumption and predation on or parasitism of target pests, cast or fecal production, locomotor functioning, defensiveness and aggression, and foraging and flight efficiency.
5. Reproduction — fecundity, reproductive anatomy and function, and offspring production, including egg laying rate, hatching rate, juvenile number, larval ejection, ovary development and sperm deformation, sex ratio, brood number and production, sterility, and viable brood cells.
6. Biochemical biomarkers — biochemical or molecular responses from toxic exposure, including oxidative stress (ROS), enzymatic, protein, and lipid activity or content, gene expression, cellular energy allocation or energy available, mitochondrial response, metabolism, neutral red retention time (NRRT), DNA damage, and synapsin levels.
7. Growth — weight and development of individuals, including adults and juveniles, molting rate, larval growth, and cocoon production.
8. Richness and diversity — community structure and composition, including richness, diversity, and evenness.
9. Structural changes — visible, physical histological and/or morphological changes, such as damage to the epidermis.
A total of 394 laboratory and field studies fit our criteria, yielding 2,842 tested parameters representing 275 unique species, taxa, or combined taxa of soil organisms and 284 different pesticide active ingredients or unique mixtures of active ingredients. The majority of studies (257) tested the impact of insecticides on 1,592 tested parameters, followed by herbicides, fungicides, bactericides, and pesticide mixtures with 67, 55, 49, and 2 studies looking at 541, 465, 218, and 26 tested parameters, respectively. We present the negative and positive effect percentages below, and the percentage of parameters with no significant effect can be gleaned from these values, as the total percentage always equals 100%.
Overall, we found that pesticides negatively affected 70.5% and positively affected 1.4% of tested parameters (Table 1). By pesticide type, 74.9% of tested parameters were negatively affected by insecticides, 63.2% by herbicides, 71.4% by fungicides, 57.7% by bactericides, and 56.4% by pesticide mixtures (Table 1). The impact of pesticide mixtures depended on the type; of the 49 mixtures, those consisting of insecticides negatively affected tested parameters 83.7% of the time compared to 61.5, 38.6, and 49.5% caused by herbicide mixtures, fungicide mixtures, and cross pesticide mixtures, respectively.
Table 1. The number of tested parameters (# par.) and the percentage that resulted in negative effects (% neg.) to each soil taxa after exposure to different pesticide types.
Overall, insecticides, herbicides, fungicides, and mixtures each negatively affected a similar number of parameters in the laboratory setting – roughly 80% (Figure 1). Comparatively, studies conducted in the field generally resulted in fewer significant negative impacts, with insecticides negatively impacting about 60% of tested parameters, fungicides 40%, and herbicides 30% (Figure 1). Bactericides generally resulted in fewer negative results in lab studies; however, they were underrepresented compared to other pesticide types.
Figure 1. Percentage of tested parameters showing negative, positive, and no significant effects on soil invertebrates for each pesticide type from laboratory studies, field studies, and total studies.
Organophosphates and neonicotinoids were the most studied classes of insecticides; of herbicides, phosphonoglycines (glyphosate) and triazines; and of fungicides, inorganic compounds such as copper and zinc, as well as conazoles (Supplementary Table 1). Among these, organophosphate, neonicotinoid, pyrethroid and carbamate insecticides, amide/anilide herbicides, and benzimidazole and inorganic fungicides negatively affected soil taxa more than 70% of the time (Figure 2).
Figure 2. Percentage of tested parameters showing negative, positive, and no significant effects on soil invertebrates for all pesticides studied and for individual pesticide types and classes.
Of endpoint categories, structural changes and biochemical biomarkers were the most impacted by pesticides followed by reproduction, mortality, behavior, growth, richness and diversity, abundance, and lastly, biomass (Table 2). Insecticides, fungicides, and pesticide mixtures had a greater than 70% negative effect on mortality endpoints; insecticides and fungicides on reproduction; fungicides on growth; and insecticides on behavior (Table 2). Supplementary Table 2 presents the effects of the most studied classes on the endpoint categories.
Table 2. The number of tested parameters (# par.) and the percentage that resulted in negative effects (% neg.) on each endpoint category tested in soil invertebrates after exposure to different pesticide types.
Overall trends by taxa revealed that Coleoptera (beetles) were more negatively affected by insecticides (79.3% of tested parameters) than herbicides (23.7%) or fungicides (4.6%) (Table 1). Of insecticides, neonicotinoids were the most detrimental to Coleoptera, with 91.5% of tested parameters being negatively affected, while pyrethroids were lowest at 50% (Supplementary Table 1). Eighty-four to 90% of tested parameters in Oligochaetes (earthworms) were negatively affected by the most-studied classes of insecticides, yet insecticides of less-studied classes (“other” category) resulted in a lower proportion of negative effects (55.4%) (Supplementary Table 1). Amide/anilide herbicides and benzimidazole fungicides were especially harmful to earthworms (Supplementary Table 1). Enchytraeids (potworms) were one of the only taxa that were more negatively impacted following exposure to herbicides (87.8% of tested parameters) and fungicides (83.1%) than insecticides (71.1%) (Table 1). Collembola were more negatively affected when exposed to fungicides (82.4%) than insecticides (74.5%) or herbicides (65.2%), at least in lab tests (Table 1).
A total of 281 studies with 1,789 tested parameters conducted in the lab fit our criteria. The endpoints most studied in the lab were biochemical biomarkers (541), mortality (510), reproduction (343), behavior (195), growth (164), and structural changes (36) (Supplementary Table 3). Of taxa, earthworms (Oligochaeta) accounted for 60.4% (1,080) of the tested parameters in lab studies. Collembola, Isopoda, Acari, and Bombus spp. were also well-represented. There were fewer than 30 tested parameters for the remaining taxa – Coleoptera, Formicidae, parasitic wasps, non-Bombus ground-nesting bees, Isoptera, and Nematoda. Pesticides negatively affected 81.0%, positively affected 0.1%, and did not significantly affect 18.9% of tested parameters in laboratory studies (Supplementary Table 3).
A total of 122 studies containing 1,053 tested parameters conducted in a field or semi-field setting fit our criteria. There were 51 studies conducted within Europe, 30 in the United States, eight in Australia, seven in Canada, and five or less in Argentina, Brazil, Cameroon, Columbia, Egypt, India, Japan, Madagascar, Mexico, New Zealand, Sri Lanka, South Africa, and Yemen. The endpoints most studied in field settings were abundance (408), mortality (204), behavior (194), reproduction (66), growth (66), biomass (55), richness and diversity (34), and biochemical biomarkers (26) (Supplementary Table 4). Coleoptera was the most studied taxon in field studies (245 tested parameters), followed closely by Oligochaeta (241 tested parameters). Acari, Collembola, Bombus spp., non-Bombus ground-nesting bees, and Formicidae were also relatively well-represented. There were fewer than 30 tested parameters for the remaining taxa – parasitic wasps, Isopoda, Isoptera, Nematoda, Tardigrada, and Myriapoda. Additionally, 56 tested parameters were associated with mixed organism groups. Pesticides negatively affected 52.6%, positively affected 3.6%, and did not significantly affect 43.8% of the field study tested parameters (Supplementary Table 4).
Enchytraeids (potworms) were analyzed in 38 lab studies with 159 tested parameters, of which pesticides negatively affected 84.3%. Specifically, insecticides negatively affected 75.0%, herbicides 89.6%, fungicides 86.3%, and bactericides 50.0% of tested parameters. Of endpoints, pesticides negatively affected biochemical biomarkers, reproduction, survival, and behavior 81.3%, 87.2%, 84.4%, and 90.9% of the time (Supplementary Table 3). Two tested parameters analyzed growth, with copper oxychloride negatively affecting worm body mass and a mixture of epoxiconazole and dimoxystrobin having no effect (Bart et al., 2017).
All other Oligochaetes, primarily Lumbricidae, but also Eudrilidae, Glossoscolecidae, Megascolecidae, and Moniligastridae were analyzed in 179 lab studies with 1,080 tested parameters. Of these, pesticides negatively affected 85% (Supplementary Table 3). Specifically, insecticides negatively affected 89.0%, herbicides 79.3%, fungicides 82.2%, bactericides 54.5%, and pesticide mixtures 83.3% of tested parameters (Supplementary Table 5). Biochemical biomarkers, which included subcellular events such as enzyme activity, membrane stability, gene expression, metabolism, DNA damage, and general oxidative stress, were the most studied parameters and were negatively affected in 85.5% of 408 tested parameters (Supplementary Table 3). Pesticides negatively impacted earthworm survival, reproduction, growth, and structural changes in 87.7, 82.7, 79.4, and 97.1% of corresponding tested parameters, respectively (Supplementary Table 3). Behavior, which included feeding rate, activity, burrowing, cast production, litter decomposition, avoidance, and respiration, was negatively affected in 80.6% of 98 tested parameters.
Pesticides negatively affected potworms in 43.8% of 16 tested parameters in five field studies and earthworms in 49.8% of 241 tested parameters in 41 field studies. Specifically, fungicides negatively affected 60.0% and pesticide mixtures 33.3% of potworm tested parameters and insecticides negatively affected 54.5%, herbicides 37.5%, fungicides 53.8%, and pesticide mixtures 53.8% of earthworm tested parameters (Supplementary Table 6). Potworm reproduction was negatively affected in six of eight tested parameters and feeding behavior was reduced by imidacloprid and cyfluthrin seed coatings, but not by the fungicide, thiram (Supplementary Table 4) (Larink and Sommer, 2002). Earthworm mortality, abundance, and biomass were negatively affected in 77.8% of 18 tested parameters, 38.2% of 68 tested parameters, and 39.6% of 48 tested parameters, respectively (Supplementary Table 4). Earthworm richness and diversity and behaviors like avoidance of pesticides, surface and cumulative activity, burial of organic matter, cast production, feeding activity, and litter decomposition were also negatively impacted in about half to two-thirds of the tested parameters. Pesticides negatively impacted earthworm reproduction in 81.8% of 11 tested parameters (Supplementary Table 4). Imidacloprid (Kreutzweiser et al., 2008), oxychloride (Eijsackers et al., 2005), and chlorpyrifos (Reinecke and Reinecke, 2007) all reduced earthworm growth, while dimethoate, 2,4-DB, and glyphosate had no effect (Dalby et al., 1995).
Pesticides negatively affected nematodes in 84.6% of 26 tested parameters in eight lab studies. Specifically, insecticides and fungicides negatively affected 100.0% and herbicides 80.0% of tested parameters (Supplementary Table 5). The survival of various nematode species was reduced by the fungicide fludioxonil (Haegerbaeumer et al., 2019), and the herbicides acetochlor (Zhang et al., 2011, 2013) and terbuthylazine (Salminen et al., 1996) (Supplementary Table 3). Nematode reproduction was negatively affected in six of seven tested parameters, by the fungicide fludioxonil (Haegerbaeumer et al., 2019), the insecticides imidacloprid (Gomez-Eyles et al., 2009) and chlorpyrifos (Martin et al., 2009), and the herbicide acetochlor (Zhang et al., 2011, 2013). Biochemical biomarkers were negatively affected in all of six tested parameters (Supplementary Table 3); specifically, oxidative stress response in Caenorhabditis elegans was induced by the herbicides, glyphosate and paraquat (Kronberg et al., 2018), and heat shock protein and B-galactosidase activity were negatively affected by the fungicide, mancozeb (Easton et al., 2001). Growth was negatively affected in all of five tested parameters; acetochlor reduced growth in Acrobeloides nanus, Pristionchus pacificus, and C. elegans (Zhang et al., 2011, 2013), and larval and adult growth of C. elegans was negatively affected by mancozeb (Easton et al., 2001) and glyphosate (Kronberg et al., 2018), respectively.
In seven field studies, pesticides negatively affected nematodes in 46.2% of the 13 tested parameters. Specifically, insecticides negatively affected 50.0%, herbicides 28.6%, and fungicides and pesticide mixtures 100% of the tested parameters (Supplementary Table 6), including nematode mortality, biomass, and abundance (Supplementary Table 4). Carbendazim reduced survival (Burrows and Edwards, 2004), glyphosate reduced biomass (Hagner et al., 2019), and chlormethoxynil (Ishibashi et al., 1983), chloropicrin (Carrascosa et al., 2014), and a mixture of thiobencarb and simetryne (Ishibashi et al., 1983) reduced population abundance, while oxadiazon, paraquat (Ishibashi et al., 1983), azadirachtin (neem), and chlorpyrifos (Stark, 1992) had no effect on nematodes in the field. Nematode diversity was also reduced by 1,3-Dichloropropene, which also was found to negatively affect Tardigrade (water bear) density (Carrascosa et al., 2014).
Pesticides negatively affected mites in 79.6% of 49 tested parameters in 14 lab studies. Specifically, insecticides negatively impacted 78.9%, herbicides 83.3%, and fungicides 80.0% of the tested parameters (Supplementary Table 5). Mite survival and reproduction were negatively affected 79.2% and 73.7% of the time, respectively (Supplementary Table 3). Acari also avoided soils treated with chlorpyrifos, dimethoate, deltamethrin, copper, (Owojori et al., 2014), and spinosad (Rahman et al., 2011), and dimethoate significantly reduced the growth of the mite Hypoaspis aculeifer (Canestrini) (Folker-Hansen et al., 1996).
Pesticides negatively affected mites in 43.7% of 87 tested parameters in 19 field studies; specifically, insecticides negatively affected 53.5%, herbicides 16.7%, fungicides 38.5%, and pesticide mixtures 42.1% of tested parameters (Supplementary Table 6). Pesticide impacts to mite abundance varied between species, but was significantly reduced 44.3% of the time (Supplementary Table 4). Oribatid mite reproduction was negatively impacted in two of five tested parameters and Oribatid mite diversity was negatively impacted by exposure to a combination of mancozeb, copper oxychloride, and metalaxyl (Al-Assiuty et al., 2014). In one tested parameter, glyphosate positively affected Acari by increasing activity by nearly 50% (Al-Daikh et al., 2016).
The insecticide deltamethrin negatively affected Diplopoda in three tested parameters (Supplementary Table 5), causing a significant reduction in survival and neurological functioning, as well as altering millipede behavior by causing agitation, release of defensive secretion, gonopod externalization, and hemolymph leakage (Francisco et al., 2016).
Pesticides had a negative effect in 41.7% and positive effect in 25.0% of 12 tested parameters in seven field studies measuring the combined abundance of Diplopoda, Chilopoda, and Pauropoda (Supplementary Tables 4, 6). Diflubenzuron and mancozeb decreased Myriapod abundance by 73.0% and 43.0%, respectively (Adamski et al., 2009). Carbendazim and lambda-cyhalothrin reduced abundance of the millipede Trigoniulus corallinus (Gervais) (Förster et al., 2006). Monuron reduced overall Diplopod abundance, while atrazine had no significant effect (Fox, 1964). Imidacloprid (Peck, 2009) and a mixed pesticide regimen (Lundgren et al., 2013) had no significant effect on Chilipoda abundance. In pesticide-treated vineyards, the relative abundance of Diplopoda, including Julidae sp., and Pauropoda increased, while Chilopods were not affected (Vaj et al., 2014).
Pesticides negatively affected Isopods in 75.9% of 79 tested parameters in 15 lab studies; specifically, insecticides negatively impacted 80.4%, herbicides 28.6%, and fungicides 81.3% of the tested parameters (Supplementary Table 5). Pesticides negatively affected 80% of 20 behavioral parameters measuring Isopod feeding rate, feces production, avoidance, and locomotion and 85.7% of 35 biochemical biomarkers, including enzymatic activity and metabolism (Supplementary Table 3). Survival of Porcellio scaber, P. dilatatus, and Porcellionides pruinosus was reduced by diazinon (Drobne et al., 2008), dimethoate (Engenheiro et al., 2005; Ferreira et al., 2015), lambda-cyhalothrin (Jänsch et al., 2005), chlorpyrifos (Morgado et al., 2016), and pyrethrins (Zidar et al., 2012). Isopod growth was also negatively impacted in four out of seven tested parameters, reduced by imidacloprid (Drobne et al., 2008), dimethoate (Fischer et al., 1997), chlorpyrifos, and mancozeb (Morgado et al., 2016). Reproduction was negatively affected in two out of three tested parameters by dimethoate (Fischer et al., 1997) and lambda-cyhalothrin (Jänsch et al., 2005), and the epithelial thickness of P. scaber was significantly reduced by imidacloprid (Drobne et al., 2008).
Pesticides negatively affected Isopods in one of four tested parameters from three field studies (Supplementary Table 6). The insecticide lambda-cyhalothrin reduced Circoniscus ornatus abundance at field rates (Förster et al., 2006), while total Isopoda abundance was not significantly affected in pesticide treated vineyards (Nash et al., 2010). Glyphosate did not induce avoidance behavior or reduce reproduction in P. scaber (Niemeyer et al., 2018).
Pesticides negatively affected springtails in 79.1% of 225 tested parameters in 59 lab studies; specifically, insecticides negatively affected 78.1%, herbicides 72.7%, fungicides 96.4%, and bactericides 62.5% of tested parameters (Supplementary Table 5). Collembola mortality was negatively affected in 72.9% and reproduction in 89.2% of corresponding tested parameters. Pesticides negatively impacted 75.0% of 20 parameters measuring avoidance and locomotion behavior, 41.2% of 17 parameters measuring growth, and 88.0% of 25 parameters measuring DNA damage, gene expression, metabolism, gut microbial diversity, and enzymatic activities (Supplementary Table 3).
Pesticides negatively affected springtails in 53.9% of 89 tested parameters in 30 field studies; specifically, insecticides negatively affected 63.3%, herbicides 46.2%, fungicides 16.7%, and pesticide mixtures 47.6% of tested parameters (Supplementary Table 6). Collembola survival was negatively impacted by dimethoate (Joy and Chakravorty, 1991) and chlorpyrifos (Wiles and Frampton, 1996), but not cypermethrin (Wiles and Frampton, 1996) and collembola abundance was reduced 50.7% of the time (Supplementary Table 4). The insecticide ethoprophos caused a total loss in reproduction of Folsomia candida (Willem) (Leitão et al., 2014), while glyphosate had no effect (Niemeyer et al., 2018). Atrazine (Al-Assiuty and Khalil, 1996), and the glyphosate formula, Zapp Qi 620 (Niemeyer et al., 2018) both caused avoidance behavior in Collembola, and fungicide and insecticide seed treatments both significantly increased Sinella curviseta (Brook) surface activity (Zaller et al., 2016). Chlorpyrifos significantly reduced springtail species richness in one study (Fountain et al., 2007), which was not significantly affected by chlorpyrifos or dimethoate in another (Endlweber et al., 2006). Ethoprophos reduced biomass of F. candida by 60% (Leitão et al., 2014), growth was impaired by dimethoate in another (Al-Haifi et al., 2006), and chlorothalonil altered F. candida gene expression in a third tested parameter (Simões et al., 2019). In the only study of another Order of Entognatha — Protura — abundance was unaffected by imidacloprid (Peck, 2009).
One lab study with termites found that streptomycin induced aggressive fighting behavior between individual termites in the same nest (Gao et al., 2018).
Insecticides negatively impacted termites in 58.3% of 12 tested parameters in three field studies (Supplementary Table 6). In one tested parameter, chlorpyrifos significantly reduced termite abundance (De Silva et al., 2010). Fipronil significantly reduced survival and activity in colonies of the termite Coarctotermes clepsydra (Sjöstedt), as 0–30% of termites were active in treated colonies, compared to 100% in untreated colonies (Peveling et al., 2003). Fipronil also reduced wood and cardboard bait consumption in Microcerotermes, Armitermes, and Drepanotermes termites in multiple soil types (Steinbauer and Peveling, 2011). Additionally, the termite Drepanotermes rubriceps (Froggatt) only repaired 19% of damaged mounds when treated with fipronil compared to 53% in unsprayed plots (Steinbauer and Peveling, 2011).
Pesticides negatively affected ground beetles (Carabidae) in 21.1% of 19 tested parameters in four lab studies (Supplementary Table 5). Thiamethoxam seed treatment significantly reduced Coleoptera survival (Douglas et al., 2015) and beetle behavior, including feeding rate was significantly impacted in three of seven tested parameters, while beetle growth was unaffected (Supplementary Table 3).
Beetles, primarily in the family Carabidae, but also Staphylinidae, Latridiidae, Cryptophagidae, Tenebrionidae, Cleridae, Nitidulidae, and Elateridae, were the most represented taxa in field studies and pesticides negatively affected 58.0% of 245 tested parameters across 29 field studies. Specifically, insecticides negatively affected 79.2%, herbicides 28.3%, fungicides 5.0%, and pesticide mixtures 55.9% of tested parameters (Supplementary Table 6). Beetle abundance was reduced in 56.0% and reproduction in 2/3rds of corresponding tested parameters. Beetle behavior, such as feeding rate, predation, avoidance, and locomotory response were negatively affected in 77.4% of 31 tested parameters. Beetle richness and diversity, as well as growth parameters measuring larval development, body size, muscle mass, and lipid mass were negatively affected in two of seven and 16.0% of 25 parameters, respectively. However, beetle enzymatic activity, hemocyte count, and plasmatic basal and phenoloxidase activities, were negatively affected in five out of six tested parameters (Supplementary Table 4).
Of two lab studies with eight tested parameters on ant behavior, insecticides negatively affected seven, or 87.5% (Supplementary Table 5). Environmentally relevant concentrations of imidacloprid impaired Pogonomyrmex occidentalis (Cresson) navigation and foraging success (Sappington, 2018) and the activity and foraging behavior of Lasius flavus F. and L. niger L. (Thiel and Köhler, 2016). Specifically, a significant increase in aggressive behavior in L. flavus toward other nest-mates following exposure to imidacloprid reduced survival probability by 60%, but there was no significant effect on aggression or nest-mate recruitment in L. niger (Thiel and Köhler, 2016).
Pesticides negatively affected ants in 52.9% of 34 tested parameters across eight field/semi-field studies; specifically, insecticides negatively affected 62.5% of 24 tested parameters, pesticide mixtures negatively affected all three tested parameters, and herbicides and fungicides had no significant effects on four and three tested parameters, respectively (Supplementary Table 6). Ant abundance was significantly reduced 51.6% of the time by chlorpyrifos (Armenta et al., 2003), diazinon (Potter et al., 1990; Armenta et al., 2003), deltamethrin (Rodrìguez et al., 2003), fipronil (Steinbauer and Peveling, 2011), a mixture of fipronil and fenitrothion (Walker et al., 2016), and a pesticide mixture containing glyphosate, S-metolachlor, lambda-cyhalothrin and esfenvalerate (Lundgren et al., 2013). Behavior was studied in three tested parameters with two negative effects (Supplementary Table 4); clothianidin and bifenthrin applied together significantly reduced ant predation of black cutworm eggs, and bifenthrin significantly reduced the mound-building activity of L. neoniger (Emery) and Solenopsis molesta (Say) by 90% and 65%, respectively (Larson et al., 2012).
Pesticides negatively affected bumble bees in 56.3% of 128 tested parameters in 18 lab studies. Insecticides negatively impacted 57.6% of 118 tested parameters and four of the remaining 10 tested parameters were negatively impacted by fungicides (Supplementary Table 5). Bumble bee survival was significantly reduced by pesticides in 57.4% of 68 tested parameters and reproduction negatively impacted in 48.0% of 25 tested parameters. Bumble bee behavior and growth were negatively affected by pesticides about 55-60% of the time (Supplementary Table 3). Of the two biochemical biomarker parameters, clothianidin and thiamethoxam altered B. terrestris gene expression (Colgan et al., 2019).
Pesticides negatively affected bumble bees in 52.6% of 190 tested parameters across 23 field studies; specifically, insecticides negatively affected 51.6%, and pesticide mixtures 70.0% of the tested parameters. Herbicides were not studied and fungicides had 85.7% non-significant effects on seven tested parameters (Supplementary Table 6). Neonicotinoids were present in 64.2% of all pesticide treatments tested, 58.1% of which negatively affected bumble bees. Abundance, survival, and reproduction were significantly reduced in 38.5, 53.3, and 48.6% of corresponding tested parameters, respectively (Supplementary Table 4). Nest condition was severely impacted by imidacloprid, while bumble bee biomass was not significantly affected by imidacloprid or chlorpyrifos (Moffat et al., 2015). Pesticides negatively impacted bumble bee behavior in 60.9% of 64 tested parameters, which included the specific measures of pesticide avoidance, foraging efficiency, feeding rate, worker defensive response, flight activity, flower visitation, and flower handling. Pesticides also negatively affected 41.7% of 24 bumble bee growth measurements, including colony development, strength, and mass (Supplementary Table 4). Bumble bee neural function was analyzed in two studies with five tested parameters, of which four (80%) were negatively impacted by pesticide exposure.
Insecticides negatively affected non-Bombus ground-nesting bees in seven (87.5%) of eight tested parameters in two lab studies (Supplementary Table 5). Specifically, survival was significantly reduced in all tests from exposure to permethrin, mexacarbamate, aminocarb, fenitrothion, carbaryl and spinosad (Helson et al., 1994; Mayer et al., 2001). Spinosad had no significant effect on the feeding rate of Nomia melanderi (Cockerell) (Halictidae) (Mayer et al., 2001).
Pesticides negatively affected non-Bombus ground-nesting bees in 75.7% of 37 tested parameters in six field studies; specifically, insecticides and pesticide mixtures negatively affected 80.0% and 66.7% tested parameters, respectively, while other pesticide types were not tested (Supplementary Table 6). Bee survival was negatively affected in 81.8% of 22 tested parameters and abundance in six out of 11 tested parameters (Supplementary Table 4). Both neonicotinoids (Main et al., 2020), and mixed pesticide regimens (Tuell and Isaacs, 2010; Mallinger et al., 2015) negatively impacted richness and diversity of ground nesting bees.
Many parasitic wasps develop in the soil, including Trybliographa rapae (Westwood) (Figitidae), which was analyzed in one lab study with four tested parameters. Although T. rapae was seven times less sensitive to chlorfenvinphos than its host, the agricultural pest cabbage root fly (Delia radicum L.), the wasp’s mortality, longevity, fecundity, and sexual development were all significantly negatively impacted by pesticide exposure (Alix et al., 2001).
Parasitic wasps were analyzed in two field/semi-field studies with 15 tested parameters, of which 73.3% were negatively affected by pesticides. Specifically, insecticides negatively affected 70.0%, fungicides 50%, and herbicides and pesticide mixtures 100% of tested parameters (Supplementary Table 6). The survival of Tiphia vernalis (Rohwer) (Tiphiidae) was reduced by bifenthrin, carbaryl, chlorpyrifos, imidacloprid, oryzalin, pendimethalin, chlorothalonil, thiophanate-methyl, and a mixture of 2,4-D and dicamba, but not halofenozide (Oliver et al., 2006). Imidacloprid reduced T. vernalis egg laying and pest control efficiency, as measured by the ability to locate, recognize, and successfully parasitize its host, the Japanese beetle [Popillia japonica (Newman)] (Rogers and Potter, 2003).
Mixed groups of soil organisms were analyzed in 19 studies with 56 tested parameters, of which pesticides negatively affected 39.3%. Specifically, insecticides negatively affected 40.9%, herbicides 20.0%, fungicides 33.3%, and pesticide mixtures 42.9% of tested parameters (Supplementary Table 6). Soil taxa abundance and richness were negatively affected in 25.8% and 41.7% and positively affected in 19.4% and 8.3% of the 30 and 12 corresponding tested parameters, respectively (Supplementary Table 4). Litter decomposition and feeding rate behaviors were significantly reduced in 69.2% of 13 parameters testing the impact of pesticides on those ecosystem functions.
In reviewing 394 studies on pesticide impacts to soil invertebrates, we found that negative effects dominated, with 70.5% of 2,842 total tested parameters between lab and field studies identifying a negative impact to soil organisms from pesticide exposure. All of the 12 highly studied pesticide classes (Figure 2), other than synthetic auxin herbicides, negatively affected greater than 50% of the tested parameters that were analyzed. These findings indicate that a wide variety of soil-dwelling invertebrates display sensitivity to pesticides of all types and support the need for pesticide regulatory agencies to account for the risks that pesticides pose to soil invertebrates and soil ecosystems.
Insecticides were by far the most-studied pesticide type and, unsurprisingly, because they are designed to target invertebrates, had the largest negative impact on soil invertebrates of any pesticide type analyzed. We found that insecticides consistently negatively affected approximately 60% to 85% of all tested parameters among the taxa studied (Table 1). Bumble bees (Bombus spp.) and mixed soil taxa were the notable exceptions but were still negatively impacted by insecticides 54.2% and 40.9% of the time, respectively. Herbicides and fungicides, however, varied greatly depending on the chemical, taxa, and endpoint studied, resulting in negative impacts on 5% to 100% of tested parameters in the reviewed studies. Some of this variability likely stems from fewer studies on herbicide and fungicide impacts compared to insecticides and, as a result, very few tested parameters for some taxa (Table 1). These results indicate that, in general, soil invertebrates are more variable in their sensitivity to fungicides and herbicides than insecticides. There were too few studies that met our search criteria to identify clear trends regarding the impact of bactericides on soil invertebrates, likely because we did not include research on microorganisms like bacteria or fungi. Negative effect percentages of pesticide mixtures varied between 33.3% and 100%, with most negatively impacting 40–60% of the tested parameters across taxa.
We found that positive effects were rare (1.4% overall) but occurred most often when abundance was measured in field studies with the application of insecticides versus other pesticide types. A positive effect indicates a benefit to one soil organism that may come at the detriment to other soil taxa or soil ecosystem functioning. For example, abundance of certain soil taxa could increase if a pesticide reduces competitors or predators, either through mortality or emigration from the area. Therefore, while certain effects were designated as “positive” to one species or taxa in this analysis, it does not indicate, nor is it likely, that pesticides had a positive effect on the ecosystem as a whole.
Our search criteria only identified three studies that tested the sensitivity of soil invertebrates to commonly used soil fumigants, specifically 1,3-D, dazomet, chloropicrin and metam sodium. Most studies we found on fumigants studied the sensitivity of microbial/fungal organisms or target pests like plant parasitic nematodes and were beyond the scope of this review. Of the nine tested parameters involving fumigants, seven resulted in negative effects to non-target soil invertebrates. The lack of available studies on harm from soil-applied fumigants to non-target invertebrates indicates that this is an area in need of more research.
Few studies measure the effects of pesticide mixtures as opposed to individual active ingredients, though research shows that mixtures of pesticide residues in the soil are the rule rather than the exception (Silva et al., 2019). Nearly all corn grown in the United States is treated with multiple pesticides (Douglas and Tooker, 2015; Lamichhane et al., 2019) and pesticide mixtures have the potential to increase toxicity due to chemical interaction (Sgolastra et al., 2016). We included pesticide mixture studies in our analysis, which surprisingly had fewer overall negative effects than single pesticides. This is likely because mixture studies were overwhelmingly done in a field or semi-field setting rather than a laboratory. However, among only field/semi-field studies, pesticide mixtures had a higher negative effect percentage than all pesticide classes except insecticides (Figure 1). Other variables could also contribute to the overall lower negative effect percentage of mixtures. For instance, mixture studies are often done with concentrations of individual components that are known to not produce an effect individually in order to maximize the ability to identify interactive effects (Kortenkamp, 2007). Furthermore, there was considerable variability in the outcome of mixture experiments based on pesticide type analyzed (see Results Section). Therefore, we caution against comparing the negative effect percentage for mixture studies with those of individual pesticide types in this analysis. Ultimately, considering that environmental exposure to pesticide mixtures is the rule and not the exception, research on pesticide mixtures is a major gap in the literature that we hope receives future focus.
Overall, we found fewer negative impacts of pesticides in field studies compared to laboratory studies. One likely reason for this finding is that the pesticide concentrations used in lab studies were generally higher, while field studies often applied concentrations at or below the recommended use rate. Higher concentrations of pesticides are more often associated with negative effects on soil organisms (Puglisi, 2012). For instance, a study on the effects of pyrimethanil on Enchytraeids conducted across two labs in Portugal and Germany found contrasting results based on the tested pesticide concentration (Bandow et al., 2016). Due to the scope of this review, we did not identify the concentrations expected to be encountered in the environment for every pesticide used in each study. Since pesticide use rates and approved application methods can differ between countries and different areas within a country, a “field-relevant” pesticide concentration in one region may be over- or under-representative of that expected in another region. Therefore, this review is inclusive of the wide variety of exposure concentrations found in the literature and is focused on identifying hazards, not necessarily risks.
In addition, uncontrolled, confounding environmental variables could provide some buffering capacity for pesticide effects in field and semi-field studies. Climatic conditions and various seasonal or yearly variations to the agricultural setting outside of pesticide application, such as cropping system changes or irrigation, make it difficult to fully assess pesticide effects under short-term trials (Ewald et al., 2015; Pelosi et al., 2015). For example, imidacloprid applied to turfgrass without irrigation significantly impacted the pesticide’s effect on foraging bumble bees, while imidacloprid applied with irrigation did not (Gels et al., 2002). Significant differences in the soil microbial community structure were observed when pyrimethanil was applied during heavy rainfalls versus drought (Ng et al., 2014), and moisture increased Collembola sensitivity to lambda-cyhalothrin (Bandow et al., 2014). Different substrates can also determine variation in organism response to pesticide treatment (Velki and Ečimović, 2015), illustrated by the effects of phenmedipham on Enchytraeid reproduction which varied greatly between 18 different tested soils (Amorim et al., 2005a). While laboratory studies often use artificial soil or a standardized natural soil, agricultural soil environments vary widely in factors such as organic matter, water holding capacity, and pH (Amorim et al., 2005b). Additionally, ecotoxicology tests in the lab may use contact filter paper instead of soils, to which soil organisms typically show much higher sensitivity to pesticides. For example, the LC50 for earthworms treated with cypermethrin in artificial soils was 9.83 mg/kg while it was 0.30 mg/kg on contact filter paper, which is only used for testing in laboratory settings (Saxena et al., 2014).
Despite the major advantage of field studies being conducted under more realistic conditions than laboratory studies, there are some downsides to relying on them exclusively. Since the complex logistics and expensive nature of field experiments can lead to lower sample sizes and fewer replicates, they can often lack a high statistical power. This can lead to statistical results that are highly variable between studies even when the overall effects are more-or-less consistent, leading researchers to advise conservative interpretations of non-significant results in low-powered field studies (Douglas and Tooker, 2016). Some endpoints — such as reproduction or individual growth — or organisms that are smaller or less abundant can be difficult to study in the field. Additionally, since pesticides are generally approved for use in different regions with highly variable agricultural practices, geography, precipitation, temperature, air quality, background soil contamination, soil mineral content, pH and organic matter, field studies done in one region may not be representative of effects in another region. The majority of field studies we found took place in Europe and the United States, while very few field studies were conducted in countries from other continents. The disproportionate data from these temperate regions could over- or underestimate the risk of pesticides to soil organisms in other regions of the world, or even subregions in the studied countries. Controlling many of the fluctuating variables found in field tests in a laboratory setting can be useful, and both types of studies should be considered helpful in identifying potential harms that could come from pesticide use in or near soil.
The most sensitive endpoint category was structural changes, followed closely by biochemical biomarkers, then reproduction, mortality, behavior, growth, richness and diversity, abundance, and lastly, biomass (Table 2). All observable effects in a whole organism are preceded by subcellular events which can be measured in biochemical biomarker tests, yet all subcellular events will not necessarily lead to these larger, gross changes. Therefore, having the negative effect percentage of tested parameters gradually decrease from biochemical effects to sublethal effects to lethal effects to more macro-changes (like richness and diversity) was expected. While mortality is largely studied, it is often the least sensitive parameter; for example, acute mortality tests did not provide the most sensitive risk estimates for earthworms in 95% of cases (Frampton et al., 2006). Instead, when an organism is engaged in the detoxification process of pollutants in order to ensure survival, normal functions such as reproduction, growth, and feeding or burrowing behaviors are likely to suffer (Pelosi et al., 2014). For example, earthworms exposed to copper fungicides entered quiescence — a period in which development is suspended — in order to resist contamination, which resulted in a significant reduction of biomass (Bart et al., 2017).
Most studied endpoints for soil organisms provided a clear indication of harm, while others, such as avoidance behavior, inform us of an organism’s response that could indicate other negative effects either to the organism or the ecosystem (Niemeyer et al., 2018). As a test metric, avoidance behavior has high variability and lower sensitivity than other endpoints and has been suggested for better use as a screening evaluation of soil contamination (Loureiro et al., 2005; Natal-da-Luz et al., 2008; Novais et al., 2010). Avoidance represented 35% of behavior endpoints in our analysis, and pesticide exposure led to an increase in avoidance in 77% of 135 tested parameters. Observation of avoidance may explain a reduction in abundance or species richness. However, for taxa that do not avoid pesticide treated soil, reductions in abundance may result from higher mortality. There can also be false negatives in avoidance tests; for example, dimethoate did not cause avoidance behavior in Folsomia candida but did cause stress or paralysis that prevented movement (Pereira et al., 2013). Additionally, certain taxa like Annelids and Isopods possess chemical receptors that allow them to detect and therefore respond to pesticides more readily than other taxa (Loureiro et al., 2005; Amorim et al., 2008; Marques et al., 2009).
Soil toxicology research tends to focus on the impacts of direct exposure and largely ignores the indirect effects on ecosystems when soil organisms are harmed. As such, our review was only able to account for the direct, measured harm to soil organisms and does not account for any additional harm to ecosystems through indirect effects. For instance, herbicides had a greater negative effect on small arthropod population dynamics through changes to surface litter structural complexity, composition, and nutrition than from direct toxicity (House et al., 1987). Additionally, the direct effects of pesticides on soil organisms can have indirect consequences to ecosystem functioning on a larger scale, including contaminating or reducing food sources for terrestrial vertebrates such as birds (Hallmann et al., 2014; Gibbons et al., 2015), and decreasing crop yield by disrupting pollination services (Reilly et al., 2020) and biological control of target pests (Douglas et al., 2015). As an example, slug predation by the beetle Chlaenius tricolor (Dejean) was reduced by 33% following exposure to thiamethoxam, causing a 67% increase in slug activity and density, which resulted in a 19% and 5% decrease in soybean crop density and yield, respectively (Douglas et al., 2015). The importance of these indirect effects of pesticides are underappreciated and, when unaccounted for, can result in an underestimation of risk posed by pesticide use.
Persistence of pesticides in the soil varies greatly across different environmental conditions, like soil type or temperature, and among different pesticides, with particular classes like neonicotinoids (Gibbons et al., 2015) and triazines (Jablonowski et al., 2011) having consistently long soil half-lives. Both burrowing soil taxa and those that develop in the soil are likely to be more vulnerable to the effects of soil-persistent pesticides and conditions that contribute to their persistence.
Some studies in our analysis found that soil invertebrates recovered from negative effects after removal from contaminated soil or following a single pesticide application. We chose not to account for recovery in our data because it would have greatly increased the complexity of our analysis, and its relevance under typical agricultural practices is questionable considering the widespread practice of recurring treatments. For instance, agricultural fields in Great Britain received an average of 17.4 pesticide applications per year in 2015 (Goulson et al., 2018). The United States Department of Agriculture estimates that Washington apples are treated with an average of 51 different pesticides in a total of 6–17 applications per year (USDA, 2016). East coast apples are also treated 15–25 times with pesticides throughout a given year (USDA, 2016). With some pesticides persisting in soil for months or years and the real prospect of recurrent pesticidal applications during the growing season in many fields, soil organisms may not fully recover as they might in the lab or following a single application to a field.
Trends in pesticide application methods are also leading to an increase in the potential for soil contamination. Due to widespread harms associated with pesticide drift, mitigation measures are increasingly being adopted in the United States to cut down on atmospheric presence of pesticides. This includes measures that can increase soil deposition to an area, such as increasing spray droplet size, adding anti-drift adjuvants to formulations and lowering boom height (U.S. EPA, 2016a). In conjunction with other pesticide application methods that have increased considerably, such as pesticide seed treatment (Hitaj et al., 2020), agricultural soils are increasingly being exposed to pesticides at higher levels. The trend away from foliar pesticide application to soil/seed pesticide application will also increase soil exposure throughout the growing season.
It has been suggested that recovery of the soil invertebrate community is slow and can take more than 15 years (Menta, 2012). Therefore, while recovery from some of these sublethal negative effects is possible, it necessarily depends on quick elimination of the soil pesticide followed by a sufficient period for recovery to take place before another application is made. This will likely vary considerably from field to field.
In observing the effects of pesticides on soil organisms, scientists and regulators have tended to focus on a handful of surrogate species that are conducive to studying in a lab or field environment (Frampton et al., 2006; Banks et al., 2014). The selection of soil organisms for ecotoxicology studies usually relies upon an organism’s amenability to the lab and effective use as a bioindicator (Cortet et al., 1999). A review by Jänsch et al. (2006) found that pesticide studies on soil invertebrates are strongly biased toward laboratory testing, and that soil organisms are chosen based upon their ease in testing environments rather than their ecological relevance (Jänsch et al., 2006). Earthworms are the most studied soil organism in ecotoxicology, partially because of their ubiquity in the soil, their pivotal role as ecosystem engineers, and the ease with which they are studied (Luo et al., 1999; Jänsch et al., 2006; Bart et al., 2018). Eisenia spp. were particularly common in studies in this review; however, they are not naturally found in agroecosystems and are often less sensitive to pesticides than other earthworms, like Apporectodea spp. (Pelosi et al., 2013; Bart et al., 2018). Earthworms are less sensitive to pesticides than other soil invertebrates in general (Frampton et al., 2006; Jänsch et al., 2006; Daam et al., 2011), so standardized test methods have been developed for different taxa also amenable to laboratory studies: Folsomia candida (springtail: Collembola), Enchytraeus albidus (potworm: Enchytraeidae), and Hypoaspis aculeifer (mite: Acari) (Kula and Larink, 1997; Frampton et al., 2006; Jänsch et al., 2006).
Field studies typically look at diverse soil communities, often with a focus on beneficial predators like ground beetles (Carabidae) and rove beetles (Staphylinidae); larger taxa that are easier to capture and identify. Still, there is high variability in sensitivity between beetles, often depending on size and seasonal variations of life cycle; as an example, dimethoate applied at lower rates resulted in harm to smaller species like the Carabids, Agonum dorsale (Pontoppidan) and Bembidion sp., and the Staphylinid, Tachyporus hypnorum F., while not affecting larger Carabids like Pterostichus melanarius (Illiger) and Calathus erratus (Sahlberg), and generally having more harmful effects on the beetles in the autumn than in the summer (Gyldenkñrne et al., 2000).
In general, the smaller and more cryptic Protura, Diplura, Pseudoscorpionida, Symphyla, and Pauropoda are the least commonly investigated soil taxa (Menta and Remelli, 2020). In our analysis, Protura, Pauropoda, and Tardigrada were each analyzed in only one study (Peck, 2009; Carrascosa et al., 2014; Vaj et al., 2014), and Symphyla and Diplura were only analyzed in the context of mixed organism groups (Al-Haifi et al., 2006; Atwood et al., 2018). In addition, there were fewer than 20 tested parameters for parasitic wasps, termites, and Myriapods, including millipedes and centipedes.
Bumble bees were commonly represented in the studies in our analysis, yet comparatively less negatively impacted by insecticides than other soil taxa. One potential reason is that there was a higher number of field studies for bumblebees compared to other soil taxa, and, as discussed above, field studies tended to reveal fewer negative effects. Pesticides, dominated by insecticides and, in particular, neonicotinoids, negatively affected about 50-60% of bumble bee tested parameters, including survival, reproduction, neural function, and behavior. Bumble bees are one of the few taxa in our analysis that spend much of their life above ground, sometimes nest above ground, and are eusocial. Thus the exposure potential in bumble bee studies may differ from that of other soil organisms (Gradish et al., 2019). Non-Bombus ground-nesting bees, though less-studied, were negatively impacted by neonicotinoids and other insecticides at a rate more typical to other taxa in our analysis (i.e., 75–90%), suggesting bumble bees are not good surrogates for ground-nesting bees and soil organisms in general.
The European honey bee (Apis mellifera) is the only terrestrial invertebrate for which the U.S. EPA (2018) requires testing for pesticide toxicity, and only on an acute-contact exposure basis (Legal Information Institute, 2020). This is the case even for pesticides that are applied directly to the soil. When harm to pollinators is expected, the agency will often require additional studies on chronic toxicity to honey bees and/or studies on both adult and larval honey bees (U.S. EPA, 2016b). Testing on other bee species (often Bombus terrestris L.) or field or semi-field studies are sometimes, but rarely, requested (U.S. EPA, 2019b). Honey bees have unique life histories and behaviors that result in very different pesticide exposure risk to most invertebrates, even when compared to bumble bees, members of the same taxonomic family that share the very rare trait of being eusocial. While bumble bee colonies often consist of < 500 individuals nesting underground, honey bee colonies of > 10,000 individuals are generally kept in artificial boxes as a domesticated agricultural animal (Gradish et al., 2019). Therefore, pesticide risk to all soil invertebrates in the United States is essentially estimated by harm to a species that generally does not come into contact with the soil and does not share any of the same exposure pathways. For example, most neonicotinoid seed coatings end up in the soil, and concentrations of neonicotinoids can be drastically higher in soil than in pollen (Goulson, 2015; Willis Chan et al., 2019; Dubey et al., 2020; Main et al., 2020).
Not only is A. mellifera a highly specialized species whose sensitivity to chemical stressors is not typically representative of other terrestrial invertebrates (Hardstone and Scott, 2010), other arthropods differ from honey bees in their seasonal timing of emergence, life span, degree of sociality, nesting behavior, and foraging, and are thus subjected to different pesticide exposure routes and levels than honey bees - such as via cuticle contact or soil-covered prey consumption. For example, bumble bees in underground nests experience contact exposure via residues on soils, including as developing larvae and hibernating queens (Gradish et al., 2019). Over 80% of bees, the vast majority of which are solitary, are ground-nesting (Anderson and Harmon-Threatt, 2019) and are at great risk of pesticide exposure, as adult females spend the majority of their life cycle constructing nests in the soil (Willis Chan et al., 2019). Thus, even for soil invertebrates in the same superfamily as honey bees, exposure to, and harm from, pesticide residues in soil and soil cell water are not adequately estimated in current EPA pesticide ecological risk assessments.
From these data it is apparent that, as a set of chemical poisons, pesticides pose a clear hazard to soil invertebrates. A previous review has identified similar hazards to soil microorganisms (Puglisi, 2012). Each individual pesticide will pose a unique risk profile to each soil-dwelling species that is exposed based on exposure potential and sensitivity. This review supports the need for soil health endpoints in regulatory risk assessment of pesticides to assess the probability that pesticide use will negatively impact these ecosystems.
Due to the sheer number of species and endpoints that can be impacted by pesticide use and researchers’ limited capacity to test every permutation, pesticide risk assessment is heavily reliant on surrogate species that are used to generalize toxicity across taxa, and in the case of soil, across entire ecosystems. The level of detachment of soil surrogate species from the taxa they are supposed to represent varies widely across regulatory agencies around the globe. Of the pesticide regulatory agencies whose regulatory process on soil organisms could be easily obtained, none differ as much in their treatment of soil organisms as the European Food Safety Authority (EFSA) and the United States EPA. While the EFSA has testing requirements in place to quantify risk to multiple soil dwelling organisms, the EPA has none.
For pesticides that are likely to contaminate soil, the EFSA currently requires chronic toxicity tests on one earthworm species (Eisenia fetida or Eisenia andrei), one springtail species (Folsomia candida) and one mite species (Hypoaspis aculeifer) (Ockleford et al., 2017). The EFSA also requires a study on nitrogen transformation as a readout of soil microbial activity (Ockleford et al., 2017). If the ratio of exposure to toxicity exceeds a predetermined threshold, higher tier tests, such as field testing, may be required. Additional protection goals have recently been identified, and the EFSA is currently considering strengthening its requirements to include additional exposure pathways and to require tests on Isopods and mycorrhizal fungi (Ockleford et al., 2017).
The EPA’s practice of using only the honey bee as a surrogate for other terrestrial invertebrates underestimates harm to many taxa and can be even more consequential when it comes to identifying mitigation measures for a pesticide. For instance, mitigation measures the EPA has put in place for pesticides that pose a significant risk to the honey bee (which should indicate risk to all terrestrial invertebrates due to its use as a surrogate) are generally designed to reduce risk only to pollinators (U.S. EPA, 2019a, 2020b). Mitigations, like spray restrictions during flower bloom, increasing droplet size to reduce drift, or label language identifying a pollinator hazard, will likely have little impact on soil organism exposure to a pesticide. Of the 219 individual pesticides represented in our review, 74% are currently approved in the United States and 26% are not in current use but could be registered at any time, indicating that our results are highly relevant to pesticide regulation in the United States.
This paper constitutes a comprehensive review of the impacts of agricultural pesticides on soil invertebrates. We found that pesticide exposure negatively impacted soil invertebrates in 70.5% of 2,842 tested parameters from 394 reviewed studies. We also identified several broad trends and directions for future research. Insecticides were the most studied type of pesticide and generally had greater negative impacts on soil invertebrates than herbicides, fungicides and other pesticide types. Herbicides and fungicides still had a high proportion of negative effect findings, however negative effects varied much more widely between different pesticide classes and studied taxa than with insecticides. Fewer studies evaluated the impacts of pesticide mixtures. Given that pesticide mixtures are more commonly found in agricultural soils than individual active ingredients, this is a gap in the literature that should be addressed.
Studies evaluating pesticide impacts often use a narrow range of surrogate species that are easy to rear, identify, or study, while smaller and more cryptic organisms are rarely analyzed. In some cases, the organisms that are the most extensively studied are known to be less sensitive to pesticides than other organisms, suggesting that we have limited knowledge of the extent of harm caused by pesticides.
The prevalence of negative effects in our results underscores the need for soil organisms to be represented in any risk analysis of a pesticide that has the potential to contaminate soil, and for any significant risk to be mitigated in a way that will specifically reduce harm to the soil organisms that sustain important ecosystem services. The United States Environmental Protection Agency does not have sufficient testing requirements or tools in place to quantify risk to soil dwelling organisms. The European honey bee is the only terrestrial invertebrate included in mandatory ecotoxicological testing of pesticides. The practice of using the honey bee as a surrogate underestimates harm to many taxa and often results in narrow efforts to mitigate pesticide impacts solely to honey bees and other pollinators, not soil organisms.
This review presents extensive evidence that pesticides pose a serious threat to soil invertebrates and the essential ecosystem services that they provide. Given the widespread and increasing adoption of seed and soil applied pesticides that pose a particular threat to soil organisms, we strongly support the inclusion of a soil health analysis in the United States pesticide risk assessment process.
TG, TC, and ND conceptualized the project and, with KK, developed the structure for the review. TG researched, compiled data, and prepared an initial manuscript. All authors discussed the results and contributed specific knowledge of the relevant literature. All authors contributed to the editing and finalization of the manuscript and figures.
Publication fees were paid for by The Center for Biological Diversity and Friends of the Earth U.S.
ND, on behalf of his employer, has submitted public comments to the Environmental Protection Agency that have been critical of the agency’s pesticide risk assessments of terrestrial invertebrates and use of honey bees as surrogate species. ND and TC, on behalf of their employer, have expressed public positions on the scientific evidence of harm to terrestrial invertebrates from some pesticides.
The remaining authors declare that the research was conducted in the absence of any commercial or financial relationships that could be construed as a potential conflict of interest.
The Supplementary Material for this article can be found online at: https://www.frontiersin.org/articles/10.3389/fenvs.2021.643847/full#supplementary-material
Adams, G. A., and Wall, D. H. (2000). Biodiversity above and below the surface of soils and sediments: linkages and implications for global change. BioScience 50, 1043–1048.
Adamski, Z., Błoszyk, J., Piosik, K., and Tomczak, K. (2009). Effects of diflubenzuron and mancozeb on soil microarthropods: a long-term study. Biol. Lett. 46, 3–13. doi: 10.2478/v10120-009-0008-y
Al-Daikh, E. B., El-Mabrouk, A., and El Roby, A. S. M. H. (2016). Effect of glyphosate herbicide on the behavior of soil arthropods in non-organic tomato system. Adv. Agric. Biol. 5, 14–19. doi: 10.15192/PSCP.AAB.2016.5.1.1419
Al-Haifi, M. A., Khan, M. Z., Abdullah, V. M., and Ghole, S. (2006). Effect of dimethoate residues on soil micro-arthropods population in the valley of Zendan, Yemen. J. Appl. Sci. Environ. Manag. 10, 37–41. doi: 10.4314/jasem.v10i2.43657
Al-Assiuty, A. I. M., and Khalil, M. A. (1996). Effects of the herbicide atrazine on Entomobrya musatica (Collembola) in field and laboratory experiments. Appl. Soil Ecol. 4, 139–146. doi: 10.1016/0929-1393(96)00107-2
Al-Assiuty, A.-N. I. M., Khalil, M. A., Ismail, A.-W. A., van Straalen, N. M., and Ageba, M. F. (2014). Effects of fungicides and biofungicides on population density and community structure of soil oribatid mites. Sci. Total Environ. 466–467, 412–420. doi: 10.1016/j.scitotenv.2013.07.063
Alford, A., and Krupke, C. H. (2017). Translocation of the neonicotinoid seed treatment clothianidin in maize. PLoS One 12:e0173836. doi: 10.1371/journal.pone.0173836
Alix, A., Cortesero, A. M., Nénon, J. P., and Anger, J. P. (2001). Selectivity assessment of chlorfenvinphos reevaluated by including physiological and behavioral effects on an important beneficial insect. Environ. Toxicol. Chem. 20, 2530–2536. doi: 10.1002/etc.5620201119
Amorim, M. J. B., Novais, S., Römbke, J., and Soares, A. M. V. M. (2008). Avoidance test with Enchytraeus albidus (Enchytraeidae): effects of different exposure time and soil properties. Environ. Pollut. 155, 112–116. doi: 10.1016/j.envpol.2007.10.028
Amorim, M. J. B., Römbke, J., Scheffczyk, A., and Soares, A. M. V. M. (2005a). Effect of different soil types on the enchytraeids Enchytraeus albidus and Enchytraeus luxuriosus using the herbicide Phenmedipham. Chemosphere 61, 1102–1114. doi: 10.1016/j.chemosphere.2005.03.048
Amorim, M. J. B., Römbke, J., and Soares, A. M. V. M. (2005b). Avoidance behaviour of Enchytraeus albidus: effects of Benomyl, Carbendazim, phenmedipham and different soil types. Chemosphere 59, 501–510. doi: 10.1016/j.chemosphere.2005.01.057
Anderson, N. L., and Harmon-Threatt, A. N. (2019). Chronic contact with realistic soil concentrations of imidacloprid affects the mass, immature development speed, and adult longevity of solitary bees. Sci. Rep. 9:3724. doi: 10.1038/s41598-019-40031-9
Armenta, R., Martinez, A. M., Chapman, J. W., Magallanes, R., Goulson, D., Caballero, P., et al. (2003). Impact of a Nucleopolyhedrovirus bioinsecticide and selected synthetic insecticides on the abundance of insect natural enemies on Maize in Southern Mexico. J. Econ. Entomol. 96, 649–661.
Atwood, L. W., Mortensen, D. A., Koide, R. T., and Smith, R. G. (2018). Evidence for multi-trophic effects of pesticide seed treatments on non-targeted soil fauna. Soil Biol. Biochem. 125, 144–155. doi: 10.1016/j.soilbio.2018.07.007
Balvanera, P., Pfisterer, A. B., Buchmann, N., He, J.-S., Nakashizuka, T., Raffaelli, D., et al. (2006). Quantifying the evidence for biodiversity effects on ecosystem functioning and services: biodiversity and ecosystem functioning/services. Ecol. Lett. 9, 1146–1156. doi: 10.1111/j.1461-0248.2006.00963.x
Bandow, C., Coors, A., Karau, N., and Römbke, J. (2014). Interactive effects of lambda-cyhalothrin, soil moisture, and temperature on Folsomia candida and Sinella curviseta (Collembola): interaction of chemical and climatic factors on Collembola. Environ. Toxicol. Chem. 33, 654–661. doi: 10.1002/etc.2479
Bandow, C., Ng, E. L., Schmelz, R. M., Sousa, J. P., and Römbke, J. (2016). A TME study with the fungicide pyrimethanil combined with different moisture regimes: effects on enchytraeids. Ecotoxicology 25, 213–224. doi: 10.1007/s10646-015-1581-y
Banks, J. E., Stark, J. D., Vargas, R. I., and Ackleh, A. S. (2014). Deconstructing the surrogate species concept: a life history approach to the protection of ecosystem services. Ecol. Appl. 24, 770–778. doi: 10.1890/13-0937.1
Bardgett, R. D., and van der Putten, W. H. (2014). Belowground biodiversity and ecosystem functioning. Nature 515, 505–511. doi: 10.1038/nature13855
Bart, S., Amossé, J., Lowe, C. N., Mougin, C., Péry, A. R. R., and Pelosi, C. (2018). Aporrectodea caliginosa, a relevant earthworm species for a posteriori pesticide risk assessment: current knowledge and recommendations for culture and experimental design. Environ. Sci. Pollut. Res. Int. 25, 33867–33881. doi: 10.1007/s11356-018-2579-9
Bart, S., Laurent, C., Péry, A. R. R., Mougin, C., and Pelosi, C. (2017). Differences in sensitivity between earthworms and enchytraeids exposed to two commercial fungicides. Ecotoxicol. Environ. Saf. 140, 177–184. doi: 10.1016/j.ecoenv.2017.02.052
Biondi, A., Mommaerts, V., Smagghe, G., Viñuela, E., Zappalà, L., and Desneux, N. (2012). The non-target impact of spinosyns on beneficial arthropods. Pest Manag. Sci. 68, 1523–1536. doi: 10.1002/ps.3396
Blouin, M., Hodson, M. E., Delgado, E. A., Baker, G., Brussaard, L., Butt, K. R., et al. (2013). A review of earthworm impact on soil function and ecosystem services: earthworm impact on ecosystem services. Eur. J. Soil Sci. 64, 161–182. doi: 10.1111/ejss.12025
Burrows, L. A., and Edwards, C. A. (2004). The use of integrated soil microcosms to assess the impact of carbendazim on soil ecosystems. Ecotoxicology 13, 143–161. doi: 10.1023/B:ECTX.0000012411.14680.21
Carrascosa, M., Sánchez-Moreno, S., and Alonso-Prados, J. L. (2014). Relationships between nematode diversity, plant biomass, nutrient cycling and soil suppressiveness in fumigated soils. Eur. J. Soil Biol. 62, 49–59. doi: 10.1016/j.ejsobi.2014.02.009
Chagnon, M., Kreutzweiser, D., Mitchell, E. A. D., Morrissey, C. A., Noome, D. A., and Van der Sluijs, J. P. (2015). Risks of large-scale use of systemic insecticides to ecosystem functioning and services. Environ. Sci. Pollut. Res. 22, 119–134. doi: 10.1007/s11356-014-3277-x
Colgan, T. J., Fletcher, I. K., Arce, A. N., Gill, R. J., Ramos Rodrigues, A., Stolle, E., et al. (2019). Caste- and pesticide-specific effects of neonicotinoid pesticide exposure on gene expression in bumblebees. Mol. Ecol. 28, 1964–1974. doi: 10.1111/mec.15047
Cortet, J., Vauflery, A. G.-D., Poinsot-Balaguer, N., Gomot, L., Texier, C., and Cluzeau, D. (1999). The use of invertebrate soil fauna in monitoring pollutant effects. Eur. J. Soil Biol. 35, 115–134. doi: 10.1016/S1164-5563(00)00116-3
da Silva Souza, T., Christofoletti, C. A., Bozzatto, V., and Fontanetti, C. S. (2014). The use of diplopods in soil ecotoxicology – A review. Ecotoxicol. Environ. Saf. 103, 68–73. doi: 10.1016/j.ecoenv.2013.10.025
Daam, M. A., Leitão, S., Cerejeira, M. J., and Paulo Sousa, J. (2011). Comparing the sensitivity of soil invertebrates to pesticides with that of Eisenia fetida. Chemosphere 85, 1040–1047. doi: 10.1016/j.chemosphere.2011.07.032
Dalby, P. R., Baker, G. H., and Smith’, S. E. (1995). Glyphosate, 2,4-DB and dimethoate: effects on earthworm survival and growth. Soil Biol. Biochem. 27, 1661–1662.
De Silva, P. M. C. S., Pathiratne, A., van Straalen, N. M., and van Gestel, C. A. M. (2010). Chlorpyrifos causes decreased organic matter decomposition by suppressing earthworm and termite communities in tropical soil. Environ. Pollut. 158, 3041–3047. doi: 10.1016/j.envpol.2010.06.032
Díaz, S., Fargione, J., Chapin, F. S. III., and Tilman, D. (2006). Biodiversity loss threatens human well-being. PLoS Biol. 4:e277. doi: 10.1371/journal.pbio.0040277
DiBartolomeis, M., Kegley, S., Mineau, P., Radford, R., and Klein, K. (2019). An assessment of acute insecticide toxicity loading (AITL) of chemical pesticides used on agricultural land in the United States. PLoS One 14:e0220029. doi: 10.1371/journal.pone.0220029
Dirzo, R., Young, H. S., Galetti, M., Ceballos, G., Isaac, N. J. B., and Collen, B. (2014). Defaunation in the Anthropocene. Science 345, 401–406. doi: 10.1126/science.1251817
Donley, N. (2019). The USA lags behind other agricultural nations in banning harmful pesticides. Environ. Health 18:44. doi: 10.1186/s12940-019-0488-0
Doublet, J., Mamy, L., and Barriuso, E. (2009). Delayed degradation in soil of foliar herbicides glyphosate and sulcotrione previously absorbed by plants: consequences on herbicide fate and risk assessment. Chemosphere 77, 582–589. doi: 10.1016/j.chemosphere.2009.06.044
Douglas, M. R., Rohr, J. R., and Tooker, J. F. (2015). EDITOR’S CHOICE: neonicotinoid insecticide travels through a soil food chain, disrupting biological control of non-target pests and decreasing soya bean yield. J. Appl. Ecol. 52, 250–260. doi: 10.1111/1365-2664.12372
Douglas, M. R., and Tooker, J. F. (2015). Large-scale deployment of seed treatments has driven rapid increase in use of neonicotinoid insecticides and preemptive pest management in U.S. Field Crops. Environ. Sci. Technol. 49, 5088–5097. doi: 10.1021/es506141g
Douglas, M. R., and Tooker, J. F. (2016). Meta-analysis reveals that seed-applied neonicotinoids and pyrethroids have similar negative effects on abundance of arthropod natural enemies. PeerJ 4:e2776. doi: 10.7717/peerj.2776
Drobne, D., Blažič, M., Van Gestel, C. A. M., Lešer, V., Zidar, P., Jemec, A., et al. (2008). Toxicity of imidacloprid to the terrestrial isopod Porcellio scaber (Isopoda. Crustacea). Chemosphere 71, 1326–1334. doi: 10.1016/j.chemosphere.2007.11.042
Dubey, A., Lewis, M. T., Dively, G. P., and Hamby, K. A. (2020). Ecological impacts of pesticide seed treatments on arthropod communities in a grain crop rotation. J. Appl. Ecol. 57, 936–951. doi: 10.1111/1365-2664.13595
Easton, A., Guven, K., and de Pomerai, D. I. (2001). Toxicity of the dithiocarbamate fungicide Mancozeb to the nontarget soil nematode. Caenorhabditis elegans. J. Biochem. Mol. Toxicol. 15, 15–25.
Eijsackers, H., Beneke, P., Maboeta, M., Louw, J. P. E., and Reinecke, A. J. (2005). The implications of copper fungicide usage in vineyards for earthworm activity and resulting sustainable soil quality. Ecotoxicol. Environ. Saf. 62, 99–111. doi: 10.1016/j.ecoenv.2005.02.017
Endlweber, K., Schädler, M., and Scheu, S. (2006). Effects of foliar and soil insecticide applications on the collembolan community of an early set-aside arable field. Appl. Soil Ecol. 31, 136–146. doi: 10.1016/j.apsoil.2005.03.004
Engenheiro, E. L., Hankard, P. K., Sousa, J. P., Lemos, M. F., Weeks, J. M., and Soares, A. M. V. M. (2005). Influence of dimethoate on acetylcholinesterase activity and locomotor function in terrestrial Isopods. Environ. Toxicol. Chem. 24, 603–609. doi: 10.1897/04-131R.1
Ewald, J. A., Wheatley, C. J., Aebischer, N. J., Moreby, S. J., Duffield, S. J., Crick, H. Q. P., et al. (2015). Influences of extreme weather, climate and pesticide use on invertebrates in cereal fields over 42 years. Glob. Change Biol. 21, 3931–3950. doi: 10.1111/gcb.13026
FAO (2020). State of Knowledge of Soil Biodiversity - Status, Challenges and Potentialities: Report 2020. Rome: FAO, doi: 10.4060/cb1928en
Ferreira, N. G. C., Morgado, R., Santos, M. J. G., Soares, A. M. V. M., and Loureiro, S. (2015). Biomarkers and energy reserves in the isopod Porcellionides pruinosus: the effects of long-term exposure to dimethoate. Sci. Total Environ. 502, 91–102. doi: 10.1016/j.scitotenv.2014.08.062
Fischer, E., Farkas, S., Hornung, E., and Past, T. (1997). Sublethal effects of an organophosphorous insecticide, dimethoate, on the isopod Porcellio scaber Latr. Comp. Biochem. Physiol. C Pharmacol. Toxicol. Endocrinol. 116, 161–166. doi: 10.1016/S0742-8413(96)00164-8
Folker-Hansen, P., Krogh, P. H., and Holmstrup, M. (1996). Effect of dimethoate on body growth of representatives of the soil living mesofauna. Ecotoxicol. Environ. Saf. 33, 207–216. doi: 10.1006/eesa.1996.0027
Forister, M. L., Pelton, E. M., and Black, S. H. (2019). Declines in insect abundance and diversity: we know enough to act now. Conserv. Sci. Pract. 1:e80. doi: 10.1111/csp2.80
Förster, B., Garcia, M., Francimari, O., and Römbke, J. (2006). Effects of carbendazim and lambda-cyhalothrin on soil invertebrates and leaf litter decomposition in semi-field and field tests under tropical conditions (Amazônia, Brazil). Eur. J. Soil Biol. 42, S171–S179. doi: 10.1016/j.ejsobi.2006.07.011
Fountain, M. T., Brown, V. K., Gange, A. C., Symondson, W. O. C., and Murray, P. J. (2007). The effects of the insecticide chlorpyrifos on spider and Collembola communities. Pedobiologia 51, 147–158. doi: 10.1016/j.pedobi.2007.03.001
Fox, C. J. S. (1964). The effects of five herbicides on the number of certain invertebrate animals in grassland soil. Can. J. Plant Sci. 44, 405–409.
Frampton, G. K., Jänsch, S., Scott-Fordsmand, J. J., Römbke, J., and Van den Brink, P. J. (2006). Effects of pesticides on soil invertebrates in laboratory studies: a review and analysis using species sensitivity distributions. Environ. Toxicol. Chem. 25, 2480. doi: 10.1897/05-438R.1
Francisco, A., Nunes, P. H., Nocelli, R. C. F., and Fontanetti, C. S. (2016). Changes in synapsin levels in the millipede gymnostreptus olivaceus schubart, 1944 exposed to different concentrations of deltamethrin. Microsc. Microanal. 22, 48–54. doi: 10.1017/S1431927615015627
Gao, F., Cui, S., Li, P., Wang, X., Li, M., Song, J., et al. (2018). Ecological toxicological effect of antibiotics in soil. IOP Conf. Ser. Earth Environ. Sci. 186:012082. doi: 10.1088/1755-1315/186/3/012082
Gaupp-Berghausen, M., Hofer, M., Rewald, B., and Zaller, J. G. (2015). Glyphosate-based herbicides reduce the activity and reproduction of earthworms and lead to increased soil nutrient concentrations. Sci. Rep. 5:12886. doi: 10.1038/srep12886
Gels, J. A., Held, D. W., and Potter, D. A. (2002). Hazards of insecticides to the bumble bees bombus impatiens (Hymenoptera: Apidae) foraging on flowering white clover in turf. J. Econ. Entomol. 95, 722–728. doi: 10.1603/0022-0493-95.4.722
Gibbons, D., Morrissey, C., and Mineau, P. (2015). A review of the direct and indirect effects of neonicotinoids and fipronil on vertebrate wildlife. Environ. Sci. Pollut. Res. 22, 103–118. doi: 10.1007/s11356-014-3180-5
Gill, R. J., Baldock, K. C. R., Brown, M. J. F., Cresswell, J. E., Dicks, L. V., Fountain, M. T., et al. (2016). “Protecting an ecosystem service,” in Advances in Ecological Research eds G. Woodward, and D. A. Bohan (Amsterdam: Elsevier), 135–206. doi: 10.1016/bs.aecr.2015.10.007
Gomez-Eyles, J. L., Svendsen, C., Lister, L., Martin, H., Hodson, M. E., and Spurgeon, D. J. (2009). Measuring and modelling mixture toxicity of imidacloprid and thiacloprid on Caenorhabditis elegans and Eisenia fetida. Ecotoxicol. Environ. Saf. 72, 71–79. doi: 10.1016/j.ecoenv.2008.07.006
Goulson, D. (2015). Neonicotinoids impact bumblebee colony fitness in the field; a reanalysis of the UK’s Food & Environment Research Agency 2012 experiment. PeerJ 3:e854. doi: 10.7717/peerj.854
Goulson, D., Thompson, J., and Croombs, A. (2018). Rapid rise in toxic load for bees revealed by analysis of pesticide use in Great Britain. PeerJ 6:e5255. doi: 10.7717/peerj.5255
Gradish, A. E., van der Steen, J., Scott-Dupree, C. D., Cabrera, A. R., Cutler, G. C., Goulson, D., et al. (2019). Comparison of pesticide exposure in honey bees (Hymenoptera: Apidae) and Bumble Bees (Hymenoptera: Apidae): implications for risk assessments. Environ. Entomol. 48, 12–21. doi: 10.1093/ee/nvy168
Gyldenkñrne, S., Ravn, H. P., and Halling-Sùrensen, B. (2000). The effect of dimethoate and cypermethrin on soil-dwelling beetles under semi-field conditions. Chemosphere 41, 1045–1057.
Haegerbaeumer, A., Raschke, R., Reiff, N., Traunspurger, W., and Höss, S. (2019). Comparing the effects of fludioxonil on non-target soil invertebrates using ecotoxicological methods from single-species bioassays to model ecosystems. Ecotoxicol. Environ. Saf. 183:109596. doi: 10.1016/j.ecoenv.2019.109596
Hagner, M., Mikola, J., Saloniemi, I., Saikkonen, K., and Helander, M. (2019). Effects of a glyphosate-based herbicide on soil animal trophic groups and associated ecosystem functioning in a northern agricultural field. Sci. Rep. 9, 1–13. doi: 10.1038/s41598-019-44988-5
Hallmann, C. A., Foppen, R. P., Van Turnhout, C. A., De Kroon, H., and Jongejans, E. (2014). Declines in insectivorous birds are associated with high neonicotinoid concentrations. Nature 511, 341–343. doi: 10.1038/nature13531
Hallmann, C. A., Sorg, M., Jongejans, E., Siepel, H., Hofland, N., Schwan, H., et al. (2017). More than 75 percent decline over 27 years in total flying insect biomass in protected areas. PLoS One 12:e0185809. doi: 10.1371/journal.pone.0185809
Hardstone, M. C., and Scott, J. G. (2010). Is Apis mellifera more sensitive to insecticides than other insects? Pest Manag. Sci. 66, 1171–1180. doi: 10.1002/ps.2001
Helson, B. V., Barber, K. N., and Kingsbury, P. D. (1994). Laboratory toxicology of six forestry insecticides to four species of bee (Hymenoptera: Apoidea). Arch. Environ. Contam. Toxicol. 27, 107–114. doi: 10.1007/BF00203895
Hitaj, C., Smith, D. J., Code, A., Wechsler, S., Esker, P. D., and Douglas, M. R. (2020). Sowing Uncertainty: what we do and don’t know about the planting of pesticide-treated seed. BioScience 70, 390–403. doi: 10.1093/biosci/biaa019
Honek, A., Martinkova, Z., and Jarosik, V. (2003). Ground beetles (Carabidae) as seed predators. Eur. J. Entomol. 100, 531–544. doi: 10.14411/eje.2003.081
House, G., Worsham, A., Sheets, T., and Stinner, R. (1987). Herbicide effects on soil arthropod dynamics and wheat straw decomposition in a North Carolina no-tillage agroecosystem. Biol. Fertil. Soils 4. doi: 10.1007/bf00256982
Ishibashi, N., Kondo, E., and Ito, S. (1983). Effects of application of certain herbicides on soil nematodes and aquatic invertebrates in rice paddy fields in Japan. Crop Prot. 2, 289–304. doi: 10.1016/0261-2194(83)90003-0
Jablonowski, N. D., Schäffer, A., and Burauel, P. (2011). Still present after all these years: persistence plus potential toxicity raise questions about the use of atrazine. Environ. Sci. Pollut. Res. Int. 18, 328–331. doi: 10.1007/s11356-010-0431-y
Jänsch, S., Frampton, G. K., Römbke, J., Van den Brink, P. J., and Scott-Fordsmand, J. J. (2006). Effects of pesticides on soil invertebrates in model ecosystem and field studies: a review and comparison with laboratory toxicity data. Environ. Toxicol. Chem. 25, 2490–2501. doi: 10.1897/05-439R.1
Jänsch, S., Garcia, M., and Römbke, J. (2005). Acute and chronic isopod testing using tropical Porcellionides pruinosus and three model pesticides. Eur. J. Soil Biol. 41, 143–152. doi: 10.1016/j.ejsobi.2005.09.010
Jeschke, P., Nauen, R., Schindler, M., and Elbert, A. (2011). Overview of the status and global strategy for neonicotinoids. J. Agric. Food Chem. 59, 2897–2908. doi: 10.1021/jf101303g
Joy, V. C., and Chakravorty, P. P. (1991). Impact of insecticides on nontarget microarthropod fauna in agricultural soil. Ecotoxicol. Environ. Saf. 22, 8–16. doi: 10.1016/0147-6513(91)90041-M
Kibblewhite, M. G., Ritz, K., and Swift, M. J. (2008). Soil health in agricultural systems. Philos. Trans. R. Soc. B Biol. Sci. 363, 685–701. doi: 10.1098/rstb.2007.2178
Kortenkamp, A. (2007). Ten years of mixing cocktails: a review of combination effects of endocrine-disrupting chemicals. Environ. Health Perspect. 115(Suppl 1), 98–105. doi: 10.1289/ehp.9357
Kreutzweiser, D. P., Good, K. P., Chartrand, D. T., Scarr, T. A., and Thompson, D. G. (2008). Are leaves that fall from imidacloprid-treated maple trees to control asian longhorned beetles toxic to non-target decomposer organisms? J. Environ. Qual. 37, 639–646. doi: 10.2134/jeq2007.0278
Kronberg, M. F., Clavijo, A., Moya, A., Rossen, A., Calvo, D., Pagano, E., et al. (2018). Glyphosate-based herbicides modulate oxidative stress response in the nematode Caenorhabditis elegans. Comp. Biochem. Physiol. Part C Toxicol. Pharmacol. 214, 1–8. doi: 10.1016/j.cbpc.2018.08.002
Kula, H., and Larink, O. (1997). Development and standardization of test methods for the prediction of sublethal effects of chemicals on earthworms. Soil Biol. Biochem. 29, 635–639. doi: 10.1016/S0038-0717(96)00030-2
Lal, R. (2004a). Soil carbon sequestration impacts on global climate change and food Security. Science 304, 1623–1627. doi: 10.1126/science.1097396
Lal, R. (2004b). Soil carbon sequestration to mitigate climate change. Geoderma 123, 1–22. doi: 10.1016/j.geoderma.2004.01.032
Lamichhane, J. R., You, M. P., Laudinot, V., Barbetti, M. J., and Aubertot, J.-N. (2019). Revisiting sustainability of fungicide seed treatments for field crops. Plant Dis. 104, 610–623. doi: 10.1094/PDIS-06-19-1157-FE
Larink, O., and Sommer, R. (2002). Influence of coated seeds on soil organisms tested with bait lamina. Eur. J. Soil Biol. 38, 287–290. doi: 10.1016/S1164-5563(02)01161-5
Larson, J. L., Redmond, C. T., and Potter, D. A. (2012). Comparative impact of an anthranilic diamide and other insecticidal chemistries on beneficial invertebrates and ecosystem services in turfgrass. Pest Manag. Sci. 68, 740–748. doi: 10.1002/ps.2321
Lavelle, P., Decaëns, T., Aubert, M., Barot, S., Blouin, M., Bureau, F., et al. (2006). Soil invertebrates and ecosystem services. Eur. J. Soil Biol. 42, S3–S15. doi: 10.1016/j.ejsobi.2006.10.002
Legal Information Institute (2020). 40 C.F.R. §158.630-Terrestrial and Aquatic Nontarget Organisms Data Requirements Table. Available online at: https://www.law.cornell.edu/cfr/text/40/158.630 (accessed June 14, 2020).
Leitão, S., Cerejeira, M. J., Van den Brink, P. J., and Sousa, J. P. (2014). Effects of azoxystrobin, chlorothalonil, and ethoprophos on the reproduction of three terrestrial invertebrates using a natural Mediterranean soil. Appl. Soil Ecol. 76, 124–131. doi: 10.1016/j.apsoil.2013.12.013
Loureiro, S., Soares, A. M. V. M., and Nogueira, A. J. A. (2005). Terrestrial avoidance behaviour tests as screening tool to assess soil contamination. Environ. Pollut. 138, 121–131. doi: 10.1016/j.envpol.2005.02.013
Lundgren, J. G., Hesler, L. S., Clay, S. A., and Fausti, S. F. (2013). Insect communities in soybeans of eastern South Dakota: the effects of vegetation management and pesticides on soybean aphids, bean leaf beetles, and their natural enemies. Crop Prot. 43, 104–118. doi: 10.1016/j.cropro.2012.08.005
Luo, Y., Zang, Y., Zhong, Y., and Kong, Z. (1999). Toxicological study of two novel pesticides on earthworm Eisenia foetida. Chemosphere 39, 2347–2356. doi: 10.1016/S0045-6535(99)00142-3
Main, A. R., Webb, E. B., Goyne, K. W., and Mengel, D. (2020). Reduced species richness of native bees in field margins associated with neonicotinoid concentrations in non-target soils. Agric. Ecosyst. Environ. 287:106693. doi: 10.1016/j.agee.2019.106693
Mallinger, R. E., Werts, P., and Gratton, C. (2015). Pesticide use within a pollinator-dependent crop has negative effects on the abundance and species richness of sweat bees, Lasioglossum spp., and on bumble bee colony growth. J. Insect Conserv. 19, 999–1010. doi: 10.1007/s10841-015-9816-z
Marques, C., Pereira, R., and Gonçalves, F. (2009). Using earthworm avoidance behaviour to assess the toxicity of formulated herbicides and their active ingredients on natural soils. J. Soils Sediments 9, 137–147. doi: 10.1007/s11368-009-0058-0
Martin, H. L., Svendsen, C., Lister, L. J., Gomez-Eyles, J. L., and Spurgeon, D. J. (2009). Measurement and modeling of the toxicity of binary mixtures in the nematode Caenorhabditis elegans—a test of independent action. Environ. Toxicol. Chem. 28, 97–104. doi: 10.1897/07-215.1
Mayer, D. F., Kovács, G., Brett, B. L., and Bisabri, B. L. (2001). The effects of spinosad insecticide to adults of Apis mellifera, Megachile rotundata and Nomia melanderi (Hymenoptera: Apidae). Int. J. Hortic. Sci. 7, 93–97.
Menta, C. (2012). “Soil fauna diversity - function, soil degradation, biological indices, soil restoration,” in Biodiversity Conservation and Utilization in a Diverse World, ed. G. A. Lameed (Norderstedt: BoD – Books on Demand), doi: 10.5772/51091
Menta, C., and Remelli, S. (2020). Soil health and arthropods: from complex system to worthwhile investigation. Insects 11:54. doi: 10.3390/insects11010054
Miličić, M., Popov, S., Branco, V. V., and Cardoso, P. (2020). Insect threats and conservation through the lens of global experts. bioRxiv [Preprint]. doi: 10.1101/2020.08.28.271494
Moffat, C., Pacheco, J. G., Sharp, S., Samson, A. J., Bollan, K. A., Huang, J., et al. (2015). Chronic exposure to neonicotinoids increases neuronal vulnerability to mitochondrial dysfunction in the bumblebee (Bombus terrestris). FASEB J. 29, 2112–2119. doi: 10.1096/fj.14-267179
Morgado, R. G., Gomes, P. A. D., Ferreira, N. G. C., Cardoso, D. N., Santos, M. J. G., Soares, A. M. V. M., et al. (2016). Toxicity interaction between chlorpyrifos, mancozeb and soil moisture to the terrestrial isopod Porcellionides pruinosus. Chemosphere 144, 1845–1853. doi: 10.1016/j.chemosphere.2015.10.034
Mourtzinis, S., Krupke, C. H., Esker, P. D., Varenhorst, A., Arneson, N. J., Bradley, C. A., et al. (2019). Neonicotinoid seed treatments of soybean provide negligible benefits to US farmers. Sci. Rep. 9, 1–7. doi: 10.1038/s41598-019-47442-8
Nash, M. A., Hoffmann, A. A., and Thomson, L. J. (2010). Identifying signature of chemical applications on indigenous and invasive nontarget arthropod communities in vineyards. Ecol. Appl. 20, 1693–1703. doi: 10.1890/09-1065.1
Natal-da-Luz, T., Amorim, M. J. B., Römbke, J., and Paulo Sousa, J. (2008). Avoidance tests with earthworms and springtails: defining the minimum exposure time to observe a significant response. Ecotoxicol. Environ. Saf. 71, 545–551. doi: 10.1016/j.ecoenv.2007.09.005
Ng, E. L., Bandow, C., Proença, D. N., Santos, S., Guilherme, R., Morais, P. V., et al. (2014). Does altered rainfall regime change pesticide effects in soil? A terrestrial model ecosystem study from Mediterranean Portugal on the effects of pyrimethanil to soil microbial communities under extremes in rainfall. Appl. Soil Ecol. 84, 245–253. doi: 10.1016/j.apsoil.2014.08.006
Niemeyer, J. C., de Santo, F. B., Guerra, N., Ricardo Filho, A. M., and Pech, T. M. (2018). Do recommended doses of glyphosate-based herbicides affect soil invertebrates? Field and laboratory screening tests to risk assessment. Chemosphere 198, 154–160. doi: 10.1016/j.chemosphere.2018.01.127
Novais, S. C., Soares, A. M. V. M., and Amorim, M. J. B. (2010). Can avoidance in Enchytraeus albidus be used as a screening parameter for pesticides testing? Chemosphere 79, 233–237. doi: 10.1016/j.chemosphere.2010.01.011
Ockleford, C., Adriaanse, P., Berny, P., Brock, T., Duquesne, S., Grilli, S., et al. (2017). Scientific Opinion addressing the state of the science on risk assessment of plant protection products for in-soil organisms. EFSA J. 15:e04690. doi: 10.2903/j.efsa.2017.4690
Oliver, J. B., Reding, M. E., Moyseenko, J. J., Klein, M. G., Mannion, C. M., and Bishop, B. (2006). Survival of Adult Tiphia vernalis (Hymenoptera: Tiphiidae) After Insecticide, fungicide, and herbicide exposure in laboratory bioassays. J. Econ. Entomol. 99, 288–294.
Owojori, O. J., Waszak, K., and Roembke, J. (2014). Avoidance and reproduction tests with the predatory mite Hypoaspis aculeifer: effects of different chemical substances: avoidance and reproduction tests with Hypoaspis aculeifer. Environ. Toxicol. Chem. 33, 230–237. doi: 10.1002/etc.2421
Paoletti, M. G., and Purrington, F. F. (1991). Invertebrates as bioindicators of soil use. Agric. Ecosyst. Environ. 34, 341–362.
Peck, D. C. (2009). Long-term effects of imidacloprid on the abundance of surface- and soil-active nontarget fauna in turf. Agric. For. Entomol. 11, 405–419. doi: 10.1111/j.1461-9563.2009.00454.x
Pelosi, C., Barot, S., Capowiez, Y., Hedde, M., and Vandenbulcke, F. (2014). Pesticides and earthworms. A review. Agron. Sustain. Dev. 34, 199–228. doi: 10.1007/s13593-013-0151-z
Pelosi, C., Bertrand, M., Thénard, J., and Mougin, C. (2015). Earthworms in a 15 years agricultural trial. Appl. Soil Ecol. 88, 1–8. doi: 10.1016/j.apsoil.2014.12.004
Pelosi, C., Joimel, S., and Makowski, D. (2013). Searching for a more sensitive earthworm species to be used in pesticide homologation tests - a meta-analysis. Chemosphere 90, 895–900. doi: 10.1016/j.chemosphere.2012.09.034
Pereira, C. M. S., Novais, S. C., Soares, A. M. V. M., and Amorim, M. J. B. (2013). Dimethoate affects cholinesterases in Folsomia candida and their locomotion — False negative results of an avoidance behaviour test. Sci. Total Environ. 443, 821–827. doi: 10.1016/j.scitotenv.2012.11.044
Perrings, C., Jackson, L., Bawa, K., Brussaard, L., Brush, S., Gavin, T., et al. (2006). Biodiversity in agricultural landscapes: saving natural capital without losing interest. Conserv. Biol. 20, 263–264. doi: 10.1111/j.1523-1739.2006.00390.x
Peveling, R., McWilliam, A. N., Nagel, P., Rasolomanana, H., Rakotomianina, L. R., Ravoninjatovo, A., et al. (2003). Impact of locust control on harvester termites and endemic vertebrate predators in Madagascar. J. Appl. Ecol. 40, 729–741. doi: 10.1046/j.1365-2664.2003.00833.x
Pisa, L. W., Amaral-Rogers, V., Belzunces, L. P., Bonmatin, J. M., Downs, C. A., Goulson, D., et al. (2015). Effects of neonicotinoids and fipronil on non-target invertebrates. Environ. Sci. Pollut. Res. 22, 68–102. doi: 10.1007/s11356-014-3471-x
Potter, D. A., Buxton, M. C., Redmond, C. T., Patterson, C. G., and Powell, A. J. (1990). Toxicity of pesticides to earthworms (Oligochaeta: Lumbricidae) and effect on thatch degradation in kentucky bluegrass turf. J. Econ. Entomol. 83, 2362–2369. doi: 10.1093/jee/83.6.2362
Puglisi, E. (2012). Response of microbial organisms (aquatic and terrestrial) to pesticides. EFSA Support. Publ. 9:359E. doi: 10.2903/sp.efsa.2012.EN-359
Rahman, T., Spafford, H., and Broughton, S. (2011). Compatibility of spinosad with predaceous mites (Acari) used to control Frankliniella occidentalis (Pergande) (Thysanoptera: Thripidae). Pest Manag. Sci. 67, 993–1003. doi: 10.1002/ps.2144
Ramirez, K. S., Döring, M., Eisenhauer, N., Gardi, C., Ladau, J., Leff, J. W., et al. (2015). Toward a global platform for linking soil biodiversity data. Front. Ecol. Evol. 3:91. doi: 10.3389/fevo.2015.00091
Reilly, J. R., Artz, D. R., Biddinger, D., Bobiwash, K., Boyle, N. K., Brittain, C., et al. (2020). Crop production in the USA is frequently limited by a lack of pollinators. Proc. Royal Soc. B P Roy Soc B-Biol Sci 287:20200922. doi: 10.1098/rspb.2020.0922
Reinecke, S. A., and Reinecke, A. J. (2007). The impact of organophosphate pesticides in orchards on earthworms in the Western Cape. South Africa. Ecotoxicol. Environ. Saf. 66, 244–251. doi: 10.1016/j.ecoenv.2005.10.006
Rodrìguez, E., Peña, A., Sánchez Raya, A. J., and Campos, M. (2003). Evaluation of the effect on arthropod populations by using deltamethrin to control Phloeotribus scarabaeoides Bern. (Coleoptera: Scolytidae) in olive orchards. Chemosphere 52, 127–134. doi: 10.1016/S0045-6535(03)00184-X
Rogers, M. E., and Potter, D. A. (2003). Effects of Spring Imidacloprid Application for White Grub Control on Parasitism of Japanese beetle (Coleoptera: Scarabaeidae) by Tiphia vernalis (Hymenoptera: Tiphiidae). J. Econ. Entomol. 96, 1412–1419.
Römbke, J., Schmelz, R. M., and Pélosi, C. (2017). Effects of organic pesticides on enchytraeids (Oligochaeta) in agroecosystems: laboratory and higher-tier tests. Front. Environ. Sci. 5:20. doi: 10.3389/fenvs.2017.00020
Salminen, J., Eriksson, I., and Haimi, J. (1996). Effects of terbuthylazine on soil fauna and decomposition processes. Ecotoxicol. Environ. Saf. 34, 184–189. doi: 10.1006/eesa.1996.0062
Sánchez-Bayo, F. (2011). “Impacts of agricultural pesticides on terrestrial ecosystems,” in. Chapter 4, 63–87.
Sánchez-Bayo, F., and Wyckhuys, K. A. G. (2019). Worldwide decline of the entomofauna: a review of its drivers. Biol. Conserv. 232, 8–27. doi: 10.1016/j.biocon.2019.01.020
Sappington, J. D. (2018). Imidacloprid alters ant sociobehavioral traits at environmentally relevant concentrations. Ecotoxicology 27, 1179–1187. doi: 10.1007/s10646-018-1976-7
Saxena, P. N., Gupta, S. K., and Murthy, R. C. (2014). Comparative toxicity of carbaryl, carbofuran, cypermethrin and fenvalerate in Metaphire posthuma and Eisenia fetida —A possible mechanism. Ecotoxicol. Environ. Saf. 100, 218–225. doi: 10.1016/j.ecoenv.2013.11.006
Seibold, S., Gossner, M. M., Simons, N. K., Blüthgen, N., Müller, J., Ambarlı, D., et al. (2019). Arthropod decline in grasslands and forests is associated with landscape-level drivers. Nature 574, 671–674. doi: 10.1038/s41586-019-1684-3
Silva, V., Mol, H. G., Zomer, P., Tienstra, M., Ritsema, C. J., and Geissen, V. (2019). Pesticide residues in European agricultural soils – a hidden reality unfolded. Sci. Total Environ. 653, 1532–1545. doi: 10.1016/j.scitotenv.2018.10.441
Simões, T., Novais, S. C., Natal-da-Luz, T., Renaud, M., Leston, S., Ramos, F., et al. (2019). From laboratory to the field: validating molecular markers of effect in Folsomia candida exposed to a fungicide-based formulation. Environ. Int. 127, 522–530. doi: 10.1016/j.envint.2019.03.073
Singh, J., Schädler, M., Demetrio, W., Brown, G. G., and Eisenhauer, N. (2019). Climate change effects on earthworms - a review. SOIL Org. 91, 114–138. doi: 10.25674/so91iss3pp114
Sgolastra, F., Medrzycki, P., Bortolotti, L., Renzi, M. T., Tosi, S., Bogo, G., et al. (2016). Synergistic mortality between a neonicotinoid insecticide and an ergosterol-biosynthesis-inhibiting fungicide in three bee species. Pest Manag. Sci. 73, 1236–1243. doi: 10.1002/ps.4449
Stark, J. D. (1992). Comparison of the impact of a neem seed-kernel extract formulation, “Margosan-O” and chlorpyrifos on non-target invertebrates inhabiting turf grass. Pestic. Sci. 36, 293–299. doi: 10.1002/ps.2780360317
Steinbauer, M. J., and Peveling, R. (2011). The impact of the locust control insecticide fipronil on termites and ants in two contrasting habitats in northern Australia. Crop Prot. 30, 814–825. doi: 10.1016/j.cropro.2011.02.001
Stork, N. E., and Eggleton, P. (1992). Invertebrates as determinants and indicators of soil quality. Am. J. Altern. Agric. 7, 38–47. doi: 10.1017/S0889189300004446
Sullivan, G. T., and Ozman-Sullivan, S. K. (2021). Alarming evidence of widespread mite extinctions in the shadows of plant, insect and vertebrate extinctions. Austr. Ecol. 46, 163–176. doi: 10.1111/aec.12932
Sur, R., and Stork, A. (2003). Uptake, translocation and metabolism of imidacloprid in plants. Bull. Insectol. 56, 35–40.
Thiel, S., and Köhler, H.-R. (2016). A sublethal imidacloprid concentration alters foraging and competition behaviour of ants. Ecotoxicology 25, 814–823. doi: 10.1007/s10646-016-1638-6
Tuell, J. K., and Isaacs, R. (2010). Community and species-specific responses of wild bees to insect pest control programs applied to a pollinator-dependent crop. J. Econ. Entomol. 103, 668–675. doi: 10.1603/EC09314
U.S. EPA (2016a). Improving Labels to Reduce Pesticide Drift. Available online at: https://www.epa.gov/reducing-pesticide-drift/improving-labels-reduce-pesticide-drift (accessed December 6, 2020).
U.S. EPA (2016b). Preliminary Pollinator Assessment to Support the Registration Review of Imidacloprid. Available online at: https://www.regulations.gov/document?D=EPA-HQ-OPP-2008-0844-0140 (accessed March 31, 2016).
U.S. EPA (2018). Draft Risk Assessment (DRA) and Drinking Water Assessment (DWA) in Support of the Registration Review for Conventional Uses of Dimethyl Disulfide (DMDS) and Conventional and Antimicrobial Uses of Chloropicrin, Dazomet, Methyl Isothiocyanate (MITC), Metam Potassium (metam-K), and Metam Sodium (metam-Na). Available online at: https://www.regulations.gov/document?D=EPA-HQ-OPP-2013-0153-0032 (accessed December 15, 2020).
U.S. EPA (2019a). Decision Memorandum Supporting the Registration Decision for New Uses of the Active Ingredient Sulfoxaflor on Alfalfa, Cacao, Citrus, Corn, Cotton, Cucurbits, Grains, Pineapple, Sorghum, Soybeans, Strawberries and Tree Plantations and Amendments to the Labels. Available online at: https://www.regulations.gov/document?D=EPA-HQ-OPP-2010-0889-0570 (accessed July 13, 2019).
U.S. EPA (2019b). Sulfoxaflor: Ecological Risk Assessment for Section 3 Registration for Various Proposed New Uses. Available online at: https://www.regulations.gov/document?D=EPA-HQ-OPP-2010-0889-0566 (accessed July 13, 2019).
U.S. EPA (2020a). Final Registration Decision for the New Active Ingredient Inpyrfluxam. Available online at: https://www.regulations.gov/document?D=EPA-HQ-OPP-2018-0038-0040 (accessed September 15, 2020).
U.S. EPA (2020b). Permethrin: Proposed Interim Registration Review Decision Case Number 2510. Available online at: https://www.regulations.gov/document?D=EPA-HQ-OPP-2011-0039-0129 (accessed June 5, 2020).
U.S. EPA (2020c). Proposed Registration Decision for the New Active Ingredient Broflanilide. Available online at: https://www.regulations.gov/document?D=EPA-HQ-OPP-2018-0053-0027 (accessed November 2, 2020).
U.S. EPA (2020d). Proposed Registration Decision for the New Active Ingredient Tetraniliprole. Available online at: https://www.regulations.gov/document?D=EPA-HQ-OPP-2017-0233-0024 (accessed October 24, 2020).
USDA (2016). USDA Public Comments on the Proposed Decision to Register the Insecticide Sulfoxaflor with Reduced Uses, a Proposed Registration Published in the Federal Register on May 17, 2016; EPA Docket Identification (ID) Number EPA-HQ-OPP-2010-0889. Available online at: https://www.regulations.gov/document?D=EPA-HQ-OPP-2010-0889-0515 (accessed July 2, 2016).
Vaj, C., Van Gestel, C. A. M., and Vighi, M. (2014). Year-round behaviour of soil microarthropod communities under plant protection product application. Ecotoxicology 23, 898–913. doi: 10.1007/s10646-014-1232-8
van Klink, R., Bowler, D. E., Gongalsky, K. B., Swengel, A. B., Gentile, A., and Chase, J. M. (2020). Meta-analysis reveals declines in terrestrial but increases in freshwater insect abundances. Science 368, 417–420. doi: 10.1126/science.aax9931
Velki, M., and Ečimović, S. (2015). Changes in exposure temperature lead to changes in pesticide toxicity to earthworms: a preliminary study. Environ. Toxicol. Pharmacol. 40, 774–784. doi: 10.1016/j.etap.2015.09.009
Veresoglou, S. D., Halley, J. M., and Rillig, M. C. (2015). Extinction risk of soil biota. Nat. Commun. 6:8862. doi: 10.1038/ncomms9862
Walker, P. W., Story, P. G., and Hose, G. C. (2016). Comparative effects of pesticides, fenitrothion and fipronil, applied as ultra-low volume formulations for locust control, on non-target invertebrate assemblages in Mitchell grass plains of south-west Queensland. Australia. Crop Prot. 89, 38–46. doi: 10.1016/j.cropro.2016.06.020
Wiles, J. A., and Frampton, G. K. (1996). A field bioassay approach to assess the toxicity of insecticide residues on soil to Collembola. Pestic. Sci. 47, 273–285.
Willis Chan, D. S., Prosser, R. S., Rodríguez-Gil, J. L., and Raine, N. E. (2019). Assessment of risk to hoary squash bees (Peponapis pruinosa) and other ground-nesting bees from systemic insecticides in agricultural soil. Sci. Rep. 9:11870. doi: 10.1038/s41598-019-47805-1
Wood, T. J., and Goulson, D. (2017). The environmental risks of neonicotinoid pesticides: a review of the evidence post 2013. Environ. Sci. Pollut. Res. 24, 17285–17325. doi: 10.1007/s11356-017-9240-x
Zaller, J. G., König, N., Tiefenbacher, A., Muraoka, Y., Querner, P., Ratzenböck, A., et al. (2016). Pesticide seed dressings can affect the activity of various soil organisms and reduce decomposition of plant material. BMC Ecol. 16:37. doi: 10.1186/s12898-016-0092-x
Zhang, J., Jiang, S., Zhang, M., and Wu, X. (2011). “Monitoring the acute and subacute toxicity effects of herbicide acetochlor by bacterivorous nematode,” in 2011 International Conference on New Technology of Agricultural, Zibo: IEEE, 669–672. doi: 10.1109/ICAE.2011.5943887
Zhang, J., Liang, W., Wu, X., Jiang, S., and Li, Q. (2013). Toxic effects of acetochlor on mortality, reproduction and growth of Caenorhabditis elegans and Pristionchus pacificus. Bull. Environ. Contam. Toxicol. 90, 364–368. doi: 10.1007/s00128-012-0915-1
Keywords: pesticide, soil, regulation, EPA, invertebrate, terrestrial
Citation: Gunstone T, Cornelisse T, Klein K, Dubey A and Donley N (2021) Pesticides and Soil Invertebrates: A Hazard Assessment. Front. Environ. Sci. 9:643847. doi: 10.3389/fenvs.2021.643847
Received: 18 December 2020; Accepted: 07 April 2021;
Published: 04 May 2021.
Edited by:
Christophe Darnault, Clemson University, United StatesReviewed by:
Roberta Fulthorpe, University of Toronto Scarborough, CanadaCopyright © 2021 Gunstone, Cornelisse, Klein, Dubey and Donley. This is an open-access article distributed under the terms of the Creative Commons Attribution License (CC BY). The use, distribution or reproduction in other forums is permitted, provided the original author(s) and the copyright owner(s) are credited and that the original publication in this journal is cited, in accordance with accepted academic practice. No use, distribution or reproduction is permitted which does not comply with these terms.
*Correspondence: Nathan Donley, bmRvbmxleUBiaW9sb2dpY2FsZGl2ZXJzaXR5Lm9yZw==
Disclaimer: All claims expressed in this article are solely those of the authors and do not necessarily represent those of their affiliated organizations, or those of the publisher, the editors and the reviewers. Any product that may be evaluated in this article or claim that may be made by its manufacturer is not guaranteed or endorsed by the publisher.
Research integrity at Frontiers
Learn more about the work of our research integrity team to safeguard the quality of each article we publish.