- 1College of Science and Engineering, James Cook University, Townsville, QLD, Australia
- 2Australian Institute of Marine Science, Townsville, QLD, Australia
- 3AIMS@JCU, Division of Research and Innovation, James Cook University, Townsville, QLD, Australia
Microplastics are ubiquitous contaminants in marine environments and organisms. Concerns about potential impacts on marine organisms are usually associated with uptake of microplastics, especially via ingestion. This study used environmentally relevant exposure conditions to investigate microplastic ingestion and depuration kinetics of the planktivorous damselfish, Pomacentrus amboinensis. Irregular shaped blue polypropylene (PP) particles (longest length 125–250 μm), and regular shaped blue polyester (PET) fibers (length 600–700 μm) were selected based on physical and chemical characteristics of microplastics commonly reported in the marine environment, including in coral reef ecosystems. Individual adult damselfish were exposed to a single dose of PP particles and PET fibers at concentrations reported for waters of the Great Barrier Reef (i.e., environmentally relevant concentrations, ERC), or future projected higher concentrations (10x ERC, 100x ERC). Measured microplastic concentrations were similar to their nominal values, confirming that PP particles and PET fibers were present at the desired concentrations and available for ingestion by individual damselfish. Throughout the 128-h depuration period, the 88 experimental fish were sampled 2, 4, 8, 16, 32, 64, and 128-h post microplastic exposure and their gastrointestinal tracts (GIT) analyzed for ingested microplastics. While damselfish ingested both experimental microplastics at all concentrations, body burden, and depuration rates of PET fibers were significantly larger and longer, respectively, compared to PP particles. For both microplastic types, exposure to higher concentrations led to an increase in body burden and lower depuration rates. These findings confirm ingestion of PP particles and PET fibers by P. amboinensis and demonstrate for the first time the influence of microplastic characteristics and concentrations on body burden and depuration rates. Finally, despite measures put in place to prevent contamination, extraneous microplastics were recovered from experimental fish, highlighting the challenge to completely eliminate contamination in microplastic exposure studies. These results are critical to inform and continuously improve protocols for future microplastics research, and to elucidate patterns of microplastic contamination and associated risks in marine organisms.
Introduction
Contamination of the marine environment with microplastics (plastic items 1 μm–5 mm in length) is prevalent, with up to 51 trillion floating microplastics estimated to occur in this environment globally (van Sebille et al., 2015). This staggering amount is also predicted to significantly increase if the global community does not address plastic production, use, reuse, and disposal management (Jambeck et al., 2015; Everaert et al., 2020). Under a “business as usual scenario,” concentrations of marine microplastic contamination are estimated to increase up to 10 times by 2100 (Everaert et al., 2020).
Microplastics are frequently found contaminating marine organisms (Halstead et al., 2018; Kroon F. J. et al., 2018; Qu et al., 2018), and may disrupt physiological processes resulting in, for example, cellular stress (Jeong et al., 2016; Espinosa et al., 2017), and energy (Welden and Cowie, 2016; Lo and Chan, 2018) and hormonal (Zhao et al., 2020) imbalances. As a consequence, microplastics and their associated impacts could ultimately affect marine organisms by changing growth, reproduction, and/or mortality of individuals (Liu et al., 2020). Reports of microplastic-related risks for marine organisms have, for the most part, been associated with their uptake, and specifically direct ingestion of microplastic items (GESAMP, 2019). Other pathways, however, such as passive uptake through the gills (Bour et al., 2020a) or via trophic transfer from prey items (Santana et al., 2017; Miller et al., 2020) have been demonstrated in controlled laboratory experiments. Thus, similar to other contaminants (Blanco et al., 2018; Amoroso et al., 2020; Hassell et al., 2020), depuration is a major factor influencing the potential effects of microplastics following ingestion. Microplastic depuration alters the amount of contamination present within the gastrointestinal tract (GIT) of organisms over time, thereby influencing toxicity, vectorization of additives and sorbed chemicals, and the likelihood of trophic transfer (Dawson et al., 2018; Bour et al., 2020b). Hence, a better understanding of microplastic ingestion and depuration kinetics of marine organisms may help elucidate risks posed by this contaminant.
Microplastic ingestion and depuration kinetics have not been rigorously evaluated in marine organisms under controlled exposures, and rarely reflect environmentally relevant exposure characteristics, such as microplastic polymer composition, shape, size, color, and concentration (Lu et al., 2016; Cong et al., 2019; Table 1). For example, polystyrene (PS) beads represent the most common microplastic evaluated in controlled ingestion and depuration studies, yet microfibers and irregular microparticles comprising of other polymers, such as polyester (PET), polyethylene (PE), and polypropylene (PP) are more abundant in marine environments (Coyle et al., 2020). Furthermore, experimental microplastic concentrations are generally much higher than reported environmental concentrations (Xu S. et al., 2020). In terms of suitable species, controlled exposure studies specifically examining ingestion and depuration kinetics rarely consider organisms likely exposed to and contaminated with microplastics in the marine environment. For example, ingestion and depuration studies have mainly focused on aquatic invertebrates (Wang X. et al., 2019; Chae and An, 2020; Ehlers et al., 2020), and freshwater fish (Grigorakis et al., 2017; Xiong et al., 2019; Hoang and Felix-Kim, 2020). In contrast, only four ingestion and depuration studies have been conducted on brackish/marine fish (Manabe et al., 2011; Cong et al., 2019; Bour et al., 2020b) despite these being some of the most frequently reported organisms contaminated with microplastics (Lusher, 2015; Kroon F. J. et al., 2018; Jensen et al., 2019). Hence, there is a major shortcoming in the current literature limiting our understanding of microplastic ingestion and depuration kinetics in marine organisms. A more comprehensive exploration of environmentally relevant exposure conditions on a broader range of organisms in the marine environment is warranted (Bour et al., 2020b).
In this controlled exposure study, we investigated microplastic ingestion, body burden and depuration kinetics of the planktivorous damselfish Pomacentrus amboinensis. Adults of this species, common to shallow Indo-Pacific coral reefs, actively feed on food particles carried on water currents (McCormick and Weaver, 2012), playing a key role in transferring energy from plankton up the food web (Emslie et al., 2019). Microplastic contamination in adult P. amboinensis was common among individuals collected on reefs in the central Great Barrier Reef, Australia (Jensen et al., 2019), making this a relevant species for studies on microplastic impacts resulting from ingestion. Here, adult ambon damselfish were exposed once to environmentally relevant types (irregular shaped blue PP particles and regular shaped blue PET fibers) and concentrations (ERC) of microplastics, based on characteristics or estimations of microplastics found in sea surface waters (Cole et al., 2014; Cozar et al., 2014; Abayomi et al., 2017; Kanhai et al., 2017; Syakti et al., 2017), including at the Great Barrier Reef (Jensen et al., 2019; Everaert et al., 2020), and Lizard Island (Santana et al., unpublished data). Dose response was also assessed by exposing the damselfish to a range of microplastic concentrations (Critchell and Hoogenboom, 2018), including future scenarios of marine microplastic contamination, i.e., 10X ERC and 100X ERC – extending concentrations beyond 2100 predictions (Everaert et al., 2020). To elucidate ingestion and depuration kinetics, ambon damselfish were sampled incrementally at 2, 4, 8, 16, 32, 64, and 128-h post microplastic exposure and GITs analyzed for ingested microplastic body burden. Finally, unintended sample contamination with extraneous microplastics, generally not monitored or reported in the current literature, was determined during the controlled exposure and/or sample processing. Our findings on the residence time of these items within a marine fish and under different exposure scenarios of exposure contribute to improved understanding of the potential ecological risks posed by microplastic contamination in marine environments.
Materials and Methods
Study Area
Fish collection and the controlled exposure experiment were conducted at the Australian Museum’s Lizard Island Research Station (LIRS). Lizard Island (14°40′08″S 145°27′34″E) is a mid-shelf reef located in the northern area of the Great Barrier Reef World Heritage Area (GBR WHA). The reef system is situated approximately 30 km northeast from the Australian continent and 250 km north from the largest city in the region (Cairns; population ∼151,000). Despite its relatively remote location, there is potential for microplastic contamination coral reefs and in reef fish of Lizard Island based on recent studies reporting microplastic contamination from surface waters nearby (Reisser et al., 2013; Hall et al., 2015; Jensen et al., 2019) and from reef fish collected at Lizard Island (Kroon F. J. et al., 2018; Jensen et al., 2019).
Fish Collection and Husbandry
The fish collection and experiment were performed in accordance with relevant institutional and national guidelines and regulations (Great Barrier Reef Marine Park Authority permit G12/35236.1 and James Cook University Animal Ethics Committee Approval Number A2635). In total, 92 adult P. amboinensis were captured on SCUBA using fence and dip nets, and temporarily immobilized using a diluted solution containing clove oil (Kroon, 2015). Immediately following collection, four of the 92 fish were individually placed in resealable zip lock plastic bags and euthanized with an overdose of clove oil to establish the background level of microplastics in the study species. These four fish were processed as per laboratory fish (refer to “Quantification of Ingested Microplastics”) and GITs analyzed for putative microplastics (refer to “Preventing and Monitoring Contamination”). A sample of these plastic bags was included in the customized contaminant library to monitor unintended sample contamination with extraneous microplastics (refer to “Preventing and Monitoring Contamination”). The remaining 88 fish were transported to LIRS, placed in individual 12 L transparent polystyrene (PS) tanks (34 cm × 20 cm × 21 cm) with PP lids, and given 3–6 days to recover and acclimate prior to microplastic exposure. The use of plastic tanks and lids was due to logistical, financial, and safety risks of shipping glass aquaria via road and sea for experimental use.
The 88 experimental tanks were located in two enclosed and inter-connected laboratory rooms at LIRS, with restricted access throughout the duration of the study. Both rooms and all tanks were thoroughly cleaned with fresh bore water prior the experiment. After cleaning, tanks were air dried overnight, subsequently filled with filtered (50 μm; Puretec®, PP Series Pleated Sediment Cartridge) natural seawater from the Lizard Island lagoon and left in flow-through mode for 48 h prior to introducing the fish. Once individual fish were introduced, tanks were operated in flow-through mode with a complete tank turnover of 1.5 L h–1 to ensure good water quality and adequate aeration. The room temperature was maintained at 24°C and fish were subjected to a 12:12 h artificial light:dark cycle. Basic seawater physical (temperature, T; pH, dissolved oxygen, DO) and chemical (ammonia, NH3; nitrate, NO3) parameters were monitored with a HACH 40 portable multi-parameter meter (T, 0.1°C; DO, 0.01 mg L–1), FisherbrandTM strips (pH, 0.1) and Aquasonic test kits (NH3, 0.1 ppm; NO3, 5 ppm), respectively. Measurements were taken either directly from the tank (T and DO) or from a subsample taken with a syringe (pH and chemical parameters) every second day during the acclimation, exposure, and depuration periods in at least 30% of tanks using a random number generator each time. During the 128 h depuration period, the random number generator was only applied to those tanks that still contained fish.
Throughout the study, fish were fed with an equivalent of 1.25% of the average adult P. amboinensis biomass, adapted from Critchell and Hoogenboom (2018). Food comprised of 125–250 μm irregular shaped commercial food pellets (INVE Aquaculture; proteins min. 55%, lipids min. 9%, and natural fibrous materials max. 1.9%), and post-hatched artemia (500–800 μm) reared from a frozen artemia culture at LIRS. Food items were of similar size dimensions to experimental microplastics (Figure 1). Individual fish were considered acclimated when observed consuming the food pellets and artemia.
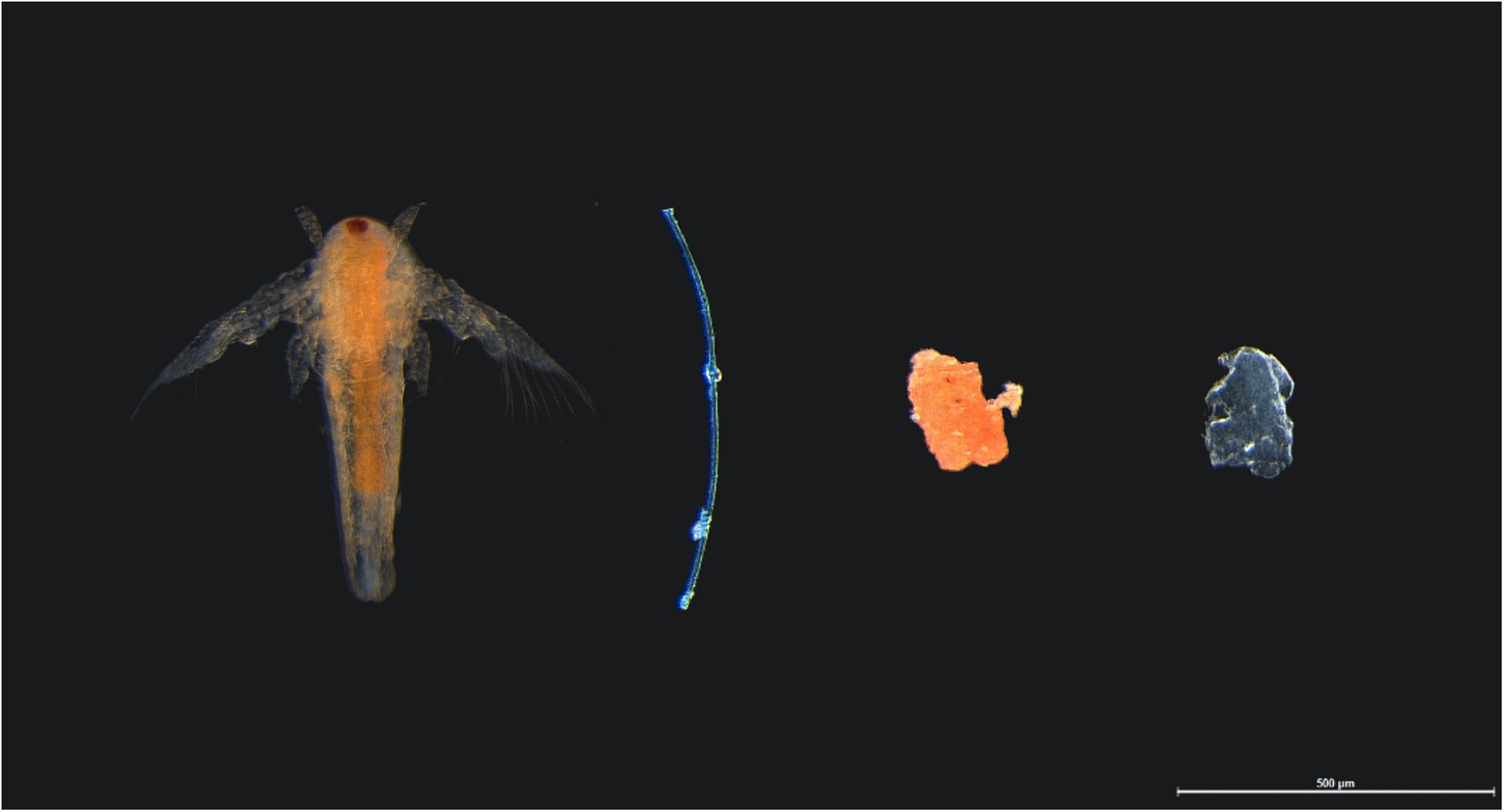
Figure 1. Comparable shapes and sizes of food and experimental microplastics given to adult damselfish Pomacentrus amboinensis in a controlled laboratory experiment. Food included orange post-hatched artemia (far left) and orange food pellet (second right). Experimental microplastics included blue polyester fiber (2nd left) and blue polypropylene particle (far right), with photo taken before biofouling of microplastics.
Experimental Microplastics
The following two experimental microplastics were used: irregular shaped blue secondary PP particles (longest length 125–250 μm), and regular shaped blue secondary PET fibers (length 600–700 μm) (Figure 1). Both microplastics were artificially produced at the Australian Institute of Marine Science (AIMS) laboratories in Townsville, QLD, Australia. The PP particles were sourced from 15 mL falcon tube lids (Greiner), milled with a commercial blender (up to 10,000 RPM), and dry sieved through two stainless steel laboratory test sieves (Endecotts) of 125 and 250 μm aperture sizes. The PET fibers were sourced from sewing thread (Gütermann, CA 02776), cut with sterile surgical blades (Paramount, BS EN 27740), and sized using calipers (Kincrome, 1/1000 in). The chemical composition of both PP particles and PET fibers were confirmed by Fourier transform infrared spectroscopy (FTIR) (Supplementary Information, SI).
To simulate microplastics found in the marine environment, PP particles, and PET fibers were biofouled at AIMS using a method modified from Kulcsár (2019). Briefly, both experimental microplastics were loosely packed into 85 mL opaque cartridges (Telos-Kinesis) (one polymer type per cartridge) each capped with a 263 μm stainless steel mesh on the outflow. Both cartridges were then connected to an overflow from a 2500 L flow-through seawater system inhabited by coral reef organisms including invertebrates (e.g., corals, sea urchins, and sea stars) and fish. To facilitate biofouling of experimental microplastics, cartridges were exposed to natural day:night conditions and ambient temperature for 7 days. Ambient seawater temperature in the cartridges was maintained by immersing both cartridges in a 13 L seawater tank connected to the same flow-through seawater system used to supply seawater for the cartridges. After 7 days, biofouled PP particles and PET fibers were hand picked and individually transferred into 20 mL scintillation vials containing 15 mL filtered (0.45 μm) natural seawater and individual doses of the three concentrations (i.e., ERC, 10x ERC, and 100x ERC) were prepared. ERC was based on monitored (0.04–0.48 m–3) (Jensen et al., 2019) and modeled (0.01–0.02 microplastic/m2) (Everaert et al., 2020) microplastic concentrations in sea surface of the Great Barrier Reef Region. Nominal microplastic concentrations were manually prepared at 1 PP particle and 1 PET fiber per 12 L [37 (h) × 22 (d) cm] tank (ERC), 10x ERCs at 10 PP particles and 10 PET fibers per 12 L tank, and 100x ERCs at 100 PP particles and 100 PET fibers per 12 L tank (Table 2). Prepared microplastic doses were kept at room temperature and exposed to an artificial 12:12 light:dark cycle for a maximum of 15 days prior to use. On the day of the exposure, standard concentrations of food pellets and cultured artemia (see section “Fish Collection and Husbandry”) were added to each vial, and to four control vials containing 15 mL filtered (0.45 μm) natural seawater. Each vial was shaken and their entire contents transferred to the designated tank. The vials were rinsed liberally with filtered natural seawater to ensure all contents were transferred.
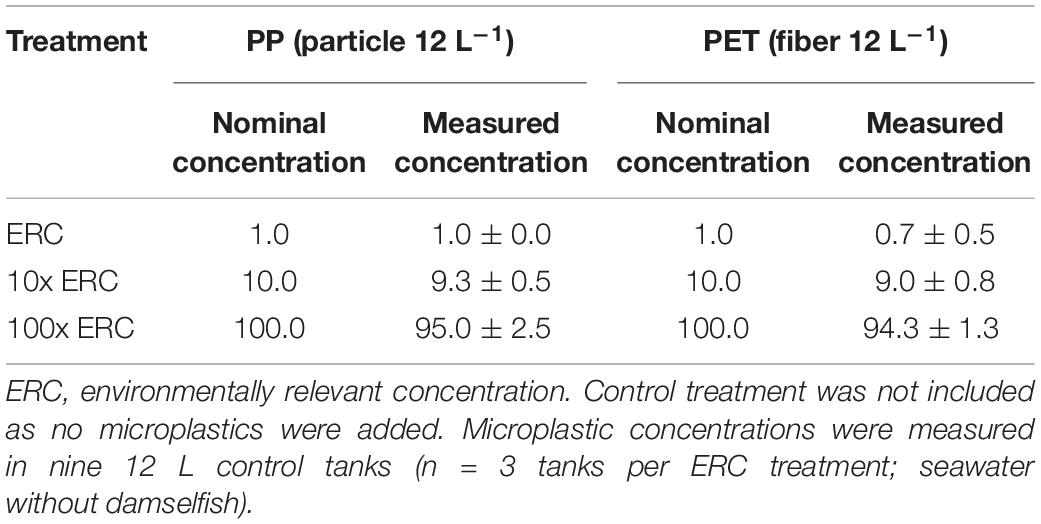
Table 2. Nominal (1, 10, or 100 particles or fibers 12 L–1) and measured (mean ± standard deviation) concentrations of microplastics in three treatment groups during single exposure of adult damselfish Pomacentrus amboinensis to blue polypropylene (PP) particles and polyester (PET) fibers in our controlled laboratory experiment.
Experimental Design and Conduct
Individual fish were acclimated for 3–6 days and fed twice daily as described above. Following acclimation, each of the 88 tanks were assigned randomly to one of four treatment groups and seven sampling periods (customized random generator script, RStudio version 1.1.463). Pre-prepared microplastic doses were added to the three exposure treatments: ERC (n = 28 tanks), 10x ERC (n = 28), and 100x ERC (n = 28) (Table 2). No experimental microplastics were added to the control group (n = 4). Space restrictions within the laboratory influenced the design of the experiment and the number of controls, precluding an equivalent number of fish for the control replicates, and the use of tanks without fish to monitor for airborne and seawater contaminants.
Prior to exposure with the experimental microplastics, all fish were starved for 24 h. Starvation was imposed to (1) ensure clear GITs prior to experimental procedure, thereby reducing any uncontrolled variability within and between treatments, and (2) increase the likelihood of microplastic ingestion by increasing the desire/need to feed (Grigorakis et al., 2017; Song et al., 2019). On the day of exposure, individual fish received their normal food as well as their randomly assigned and pre-prepared doses of PP particles and PET fibers simultaneously, as described above. During the first 30 s of microplastic exposure, tanks continued to operate in flow-through mode to abet microplastic and food dispersion in the water column. After 30 s, all tanks were switched from flow-through to circulation mode to increase the likelihood of the fish encountering the microplastics. After 2 h exposure, flow-through mode was resumed, and fish were left to depurate for up to a total of 128 h. During depuration, fish were fed as usual – without additional microplastics – twice daily. Excess food and excretions were removed by siphon daily to avoid reingestion of depurated microplastics. Filters were applied to the seawater outflow to prevent the discharge of microplastics (experimental and extraneous) into the Lizard Island coral reef environment.
Quantification of Ingested Microplastics
At 2, 4, 8, 16, 32, 64, and 128-h post exposure, four individual fish randomly chosen from each treatment were removed from their tanks and humanely euthanized using ice slurry. Fish were measured (standard and fork length, 0.1 cm, Kincrome, 1/1000 in), weighed (0.01 g, AND EK-410i), and preserved in 70% ethanol (EtOH) for transport and storage. All control fish (n = 4) were sampled at the first sampling time (2 h) to accurately demonstrate if, during the exposure period, fish were exposed to microplastics other than those intentionally offered (i.e., other than the experimental microplastics).
Individual fish were dissected to remove the entire GIT from the top of the esophagus to the rectum. Individual GITs were weighed (wet weight, w.w., Sartorius TE31025, max 3100 g, d 0.01 g) and subjected to alkaline digestion using a method adapted from Karami et al. (2017) to recover PP particles and PET fibers. Briefly, GIT digestion was conducted with 10% potassium hydroxide (KOH; AR, Fisher, CAS No. 1310-58) at 40°C for 48 h in a ratio of 1:20 of GIT wet weight (g) to volume of KOH (mL). Digested GIT solutions were filtered through 77 and 26 μm stainless steel mesh filters (19 mm diameter) (Schlawinsky, 2020), and rinsed with 70% EtOH to remove fat vestiges (Dawson et al., 2020). Microplastics retained on mesh filters were visually identified, counted and photographed using stereomicroscopy (Leica MZ16A, Leica DFC 500, Leica Application Suite LAS 4.4.0). PP particles and PET fibers were readily distinguishable from other particulates based on the combinations of shape, size and color.
Microplastic Exposure Validation and Spike-Recovery Tests
To validate the nominal concentrations of experimental microplastics, nine individual 12 L tanks without fish were dosed with biofouled and pre-prepared experimental PP and PET doses (ERC n = 3, 10x ERC n = 3, and 100x ERC n = 3) and left for 30 s in flow-through mode. Each tank was then emptied via a drain over a 40 μm nylon mesh to capture microplastics. Experimental microplastics (PP and PET) retained on mesh filters were visually identified and counted using stereomicroscopy (Zeiss SteREO Discovery.V8).
A spike-recovery test was conducted, to (1) account for impacts of the adapted KOH method on the experimental microplastics, and (2) establish recovery rates for spiked PP particles and PET fibers after the KOH digestion. Specifically, biofouled PP particles and PET fibers at the three concentrations of exposure (ERC, 10x ERC, and 100x ERC) were exposed to 1.5 mL of 10% KOH and processed as above. Three replicates were spiked for each treatment (ERC n = 3, 10X ERC n = 3, 100X ERC n = 3). Following digestion at 40°C for 48 h, samples were filtered, rinsed and the 77 and 26 μm stainless steel mesh filters visually assessed using stereomicroscopy. Recovered spiked microplastics were counted to estimate recovery rates for experimental PP particles and PET fibers after the KOH digestion.
Preventing and Monitoring Contamination
A range of measures were implemented to minimize potential microplastic contamination during room preparation, experimental procedure, and sample processing including fish dissection, and GIT digestion and filtration. As previously stated, all experimental tanks were placed in a closed room and isolated from potential air and waterborne microplastic contaminants (as much as possible) using lids and filters. Throughout the study, and whenever used, equipment and tools were sequentially cleaned with reverse osmosis H2O, Milli-Q H2O, 70% EtOH, or a combination of these. Cellulose-based cloths were used to wipe surfaces with 70% EtOH. Prior to use, 10% KOH, and 70% EtOH solutions were filtered to 0.45 μm (Millipore® HA filters). Clothing and lab coats worn during microplastic preparation, dosing and sample processing were made from naturally derived materials (e.g., cotton) to eliminate introducing synthetic fibers and specifically non-experimental PETs. All clothing was also delinted using a lint roller (Scotch-Brite®, 3M) prior to sample handling. Nevertheless, the use of plastic material was unavoidable as described above, including consumables such as zip lock bags for fish collection, and plastic gloves and parafilm during sample processing. To control for these sources of plastic items that could be unintentionally introduced into the experiment, we developed a customized contaminant library following Kroon F. et al. (2018) to enable detection of such extraneous microplastic contamination from either the environment, the experimental procedure, or during sample processing. Briefly, samples of plastic gear used during fish collection (e.g., fish net and zip lock bag), experimental procedures (e.g., exposure tanks and lids, seawater pipeline) and sample processing (e.g., spray bottle and gloves) were included in a customized contaminant library (Supplementary Table 1), along with any airborne putative microplastic identified from filtered blank processing controls (i.e., Petri dishes with 20 mL of Milli-Q H2O). Materials from other equipment, such as probes from the HACH 40 portable multi-parameter meter and the cartridge from the Puretec® filter, were not included to avoid damage to expensive and delicate instruments. All items in the contaminant library were photographed for shape and color characterization and analyzed by FTIR for chemical composition.
To determine the occurrence and potential sources of microplastic contamination throughout the study, all putative microplastics (i.e., non-test PP and PET microplastics) identified in fish GITs (including field, control, and exposed fish) and blank processing controls were physically and chemically characterized following Kroon F. et al. (2018) and Kroon F. J. et al. (2018). Items were tentatively identified as microplastics based on key physical parameters (i.e., size, shape, color) commonly used in the literature (Norén, 2007; Hidalgo-Ruz et al., 2012). All putative microplastics were then analyzed by FTIR to confirm polymer composition using either PerkinElmer Spectrum 100 FTIR [1 mm ATR window, pressure gauge = 150, eight scans at 4 cm–1 resolution, wavenumber range between 4000 and 600 cm–1, atmospheric (CO2/H2O) suppression, atmospheric vapor compensation, and background scans acquired every 10 acquired spectra] or PerkinElmer Spotlight 200i FT-IR microscope [100 μm ATR aperture, pressure gauge = 5%, 32 scans at 4 cm–1 resolution, wavenumber range between 4000 and 600 cm–1, atmospheric (CO2/H2O) suppression, atmospheric vapor compensation, and background scans acquired for every acquired spectrum]. FTIR spectra were searched against the NICDOCOM IR spectral libraries (Polymers and Additives, Coatings, Fibers, Dyes and Pigments, Petrochemicals; NICODOM Ltd., Czechia) and the matching polymer type assigned. FTIR spectra of microplastics retrieved from fish GITs were then compared with those in the custom-built contaminant library to identify potential microplastic contamination throughout the study, and infer possible sources of contamination (i.e., due to being inadvertently introduced from the environment, the experimental procedure, or during sample processing).
Data Analyses
Based on the size range of the two experimental microplastics and the limited evidence of microplastic translocation into fish tissue after uptake (Lu et al., 2016; Cong et al., 2019), we define microplastic body burden as the amount of microplastics present in the fish GIT. Microplastics body burden was quantified for PP particles and PET fibers separately and per individual fish GIT analyzed.
To determine whether microplastic body burden over time was affected by microplastic type or concentration, we used a general linear model (GLM, p < 0.05) following the equation:
where,
Type = PP particles or PET fibers,
Concentration = ERC, 10x ERC, or 100x ERC,
Time = time of collection (or depuration time),
Weight = fish weight (in g).
Fish weight was included as a covariant in the GLM because body weight is a common variable to be considered in toxicokinetic studies (Hendriks and Heikens, 2001; Lebrun et al., 2014; Miller et al., 2016). Based on the GLM model, effects of microplastic type, concentration of exposure, and depuration period were calculated as:
Plastic type:
Concentration of exposure:
Depuration period:
where, t = depuration period, ranging from 4 to 128-h.
Although fish had been randomly assigned to the four different treatments and seven sampling periods, fish weight was included in the model to account for potential variation in the amount of microplastics ingested and depurated due to fish size. To accommodate overdispersion resulting from high numbers of zero’s in the data set, the model followed a negative binomial distribution linked with log function. This data analysis was conducted with RStudio, version 1.1.463. The four control fish were not included in the GLM as these fish were never exposed to the experimental microplastics (see section “Results”).
The microplastics body burden as a function of time was used to estimate the depuration rates and elimination half-life of PP particles and PET fibers at the different exposure concentrations. Depuration rate constants were calculated based on the following first-order kinetics model, assuming that the ratio of microplastic elimination is directly proportional to microplastics concentration in the fish (Newman, 2012):
where,
Ct = amount of microplastics in the fish GIT at a particular time,
C0 = initial amount of microplastics in the fish GIT,
ke = elimination rate constant as number of microplastics per h,
t = time of measured concentration (Ct).
From this model, microplastic elimination half-life was calculated following:
where,
t1/2 = microplastic elimination half-life,
ke = elimination rate constant as number of microplastics per h.
Microplastic depuration rates and elimination half-life were calculated using GraphPad, version 8.4.1. Significant differences among depuration rates were compared using one-way ANOVA and Tukey’s multiple comparisons tests. Interaction between experimental microplastics and concentration was considered a factor (with six levels; p < 0.05).
Results
Basic Water Quality and Fish Condition
Water quality was measured on acclimation days 1, 2, 3, 4, and 5; on the day prior microplastic exposure (starvation day) and on two depuration days (2 and 4), totaling 132 times in randomly assigned tanks. Variability in seawater T (24.6°C ± 0.7), pH (8.0), and DO (8.19 mg L–1 ± 0.1) was negligible. Further, concentrations of NH3 (0 ppm) and NO3 (≤5 ppm) were consistently below limits considered harmful to marine fish.
Throughout the study, none of the fish presented any signs of stress and no mortality was recorded. Following establishment in their individual tanks, all 88 fish commenced feeding on the first day of the acclimation period and continued to do so throughout the experiment. Following microplastic exposure, no changes in fish behavior were observed across any of the four treatments.
Microplastic Exposure Validation and Spike-Recovery Tests
Measured concentrations of experimental microplastics in seawater across the three experimental treatments were similar to their nominal values (Table 2). This confirmed that irregular shaped blue PP particles and regular shaped blue PET fibers were present in the water at the desired concentrations and available for ingestion by individual damselfish.
No discernible changes in the blue color of spiked particles and fibers were observed by stereomicroscopy. Results from the spike-recovery test showed high efficiency for recovering both experimental microplastics after exposure to 10% KOH at 40°C for 48 h (Table 3). Specifically, the mean recovery rates for both PP particles and PET fibers were >85% (SD < 10) in all treatments, except for PP particles in the ERC treatment (67% ± 47).
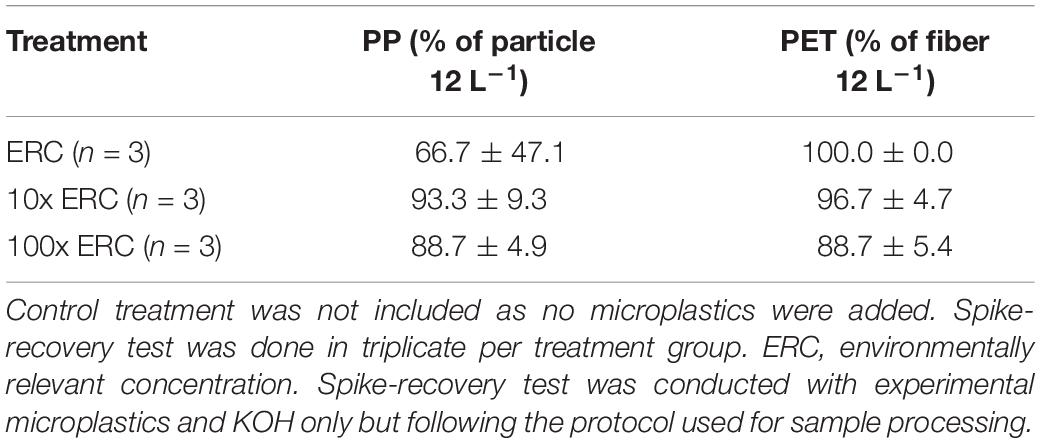
Table 3. Percentage (mean ± standard deviation) of microplastic recovery rates of from spike-recovery test in three treatment groups during single exposure of adult damselfish Pomacentrus amboinensis to blue polypropylene (PP) particles and polyethylene (PET) fibers in our controlled laboratory experiment.
Microplastic Body Burden
Both PP particles and PET fibers were observed in the GITs of exposed fish, confirming ingestion of experimental microplastics by P. amboinensis (Table 4 and Supplementary Table 2). PET fibers from the 100x ERC treatment were occasionally found entangled, sometimes with other organic materials (Figure 2). At the 2-h depuration period, all fish exposed to the future projected concentrations of 10x ERC and 100x ERC contained both experimental microplastic types in their GIT (Figure 3). The microplastic body burden for these fish represented 25–98% of the total microplastics offered in these treatments. Of fish exposed to the ERC treatment, two contained both microplastic types, one contained a single microplastic type, and one did not contain either of the microplastics offered. Similar trends were observed at the 4-h depuration time point. Both microplastic types offered were present in all fish exposed to the 10x ERC and 100x ERC concentrations, while fish from the ERC treatment contained only one microplastic type each (one a PET fiber; the other a PP particle). While microplastic body burden was similar at the 2 and 4 h depuration periods, it decreased dramatically following 8 h of depuration. At this time, the experimental microplastics were only observed in individuals exposed to the 100x ERC treatment, at a maximum of 5% of the PP particle dose and 20% of the PET fiber dose. In addition, PP were only isolated from one fish, while PET fibers were still present in all four fish sampled at 8 h depuration. After 16 h, PP particles were completely depurated from all fish across all treatments. In contrast, PET fibers were still detected in two individual fish from the 100x ERC treatment up to and including 128 h, albeit at low abundances (<2% of the body burden at 2 h depuration). Based on the fitted GLM (R2 = 0.86), mean microplastic body burden was influenced by the type of microplastic offered, the exposure concentration and the depuration period (p < 0.001), but not by fish weight (p = 0.49) (Table 5). Overall, mean body burden for PET fibers was 2.2 times higher than for PP particles (Figure 3). Within individual treatments, body burden increased as concentration of exposure increased. Fish exposed to the 10x and 100x ERC treatments contained 8.5 and 91.8 times more experimental microplastics than ERC, respectively. Finally, the most significant change in body burden over time occurred within 8 h, when, considering all concentrations of exposure, the number of experimental microplastics in the GIT dropped 83% from the first sampling time (2 h) (Table 4).
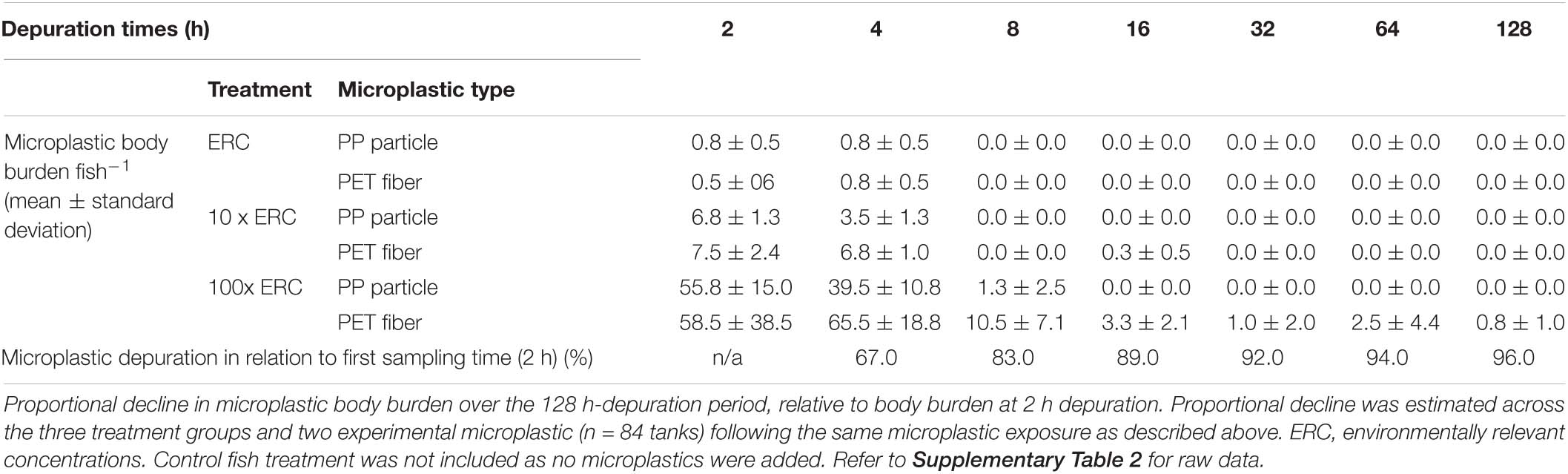
Table 4. Mean microplastic body burden fish–1 (absolute number ± standard deviation; n = 4 fish per treatment and depuration time) in three treatment groups (n = 84 tanks) during a single exposure of adult damselfish Pomacentrus amboinensis to blue polypropylene (PP) particles and polyester (PET) fibers in a controlled laboratory experiment.
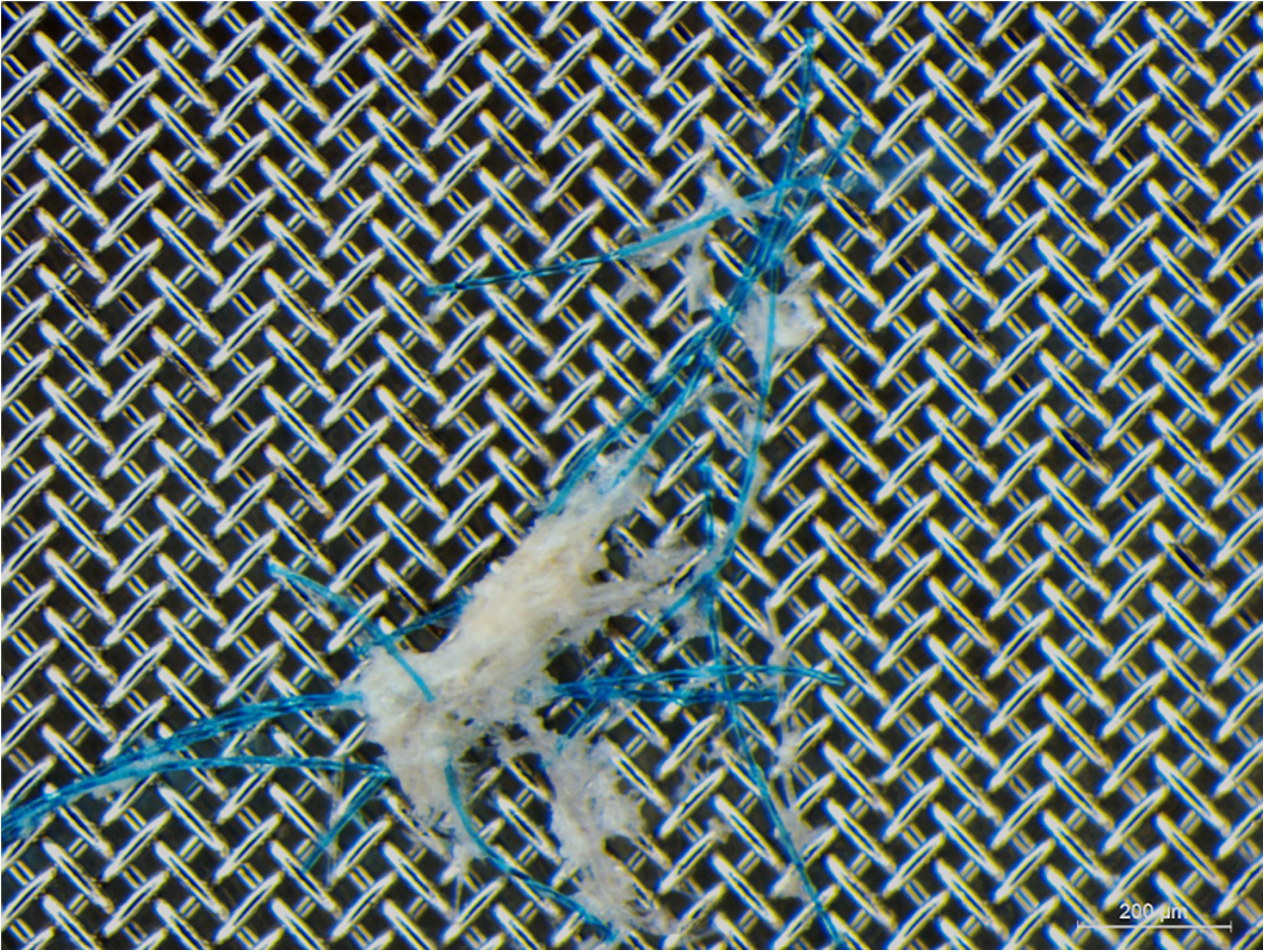
Figure 2. Polyester (PET) fibers, entangled with other ingested materials, recovered from an adult damselfish Pomacentrus amboinensis. Gut contents presented were recovered after 2 h of depuration following a single exposure to polypropylene particles and PET fibers at 100x environmentally relevant concentration.
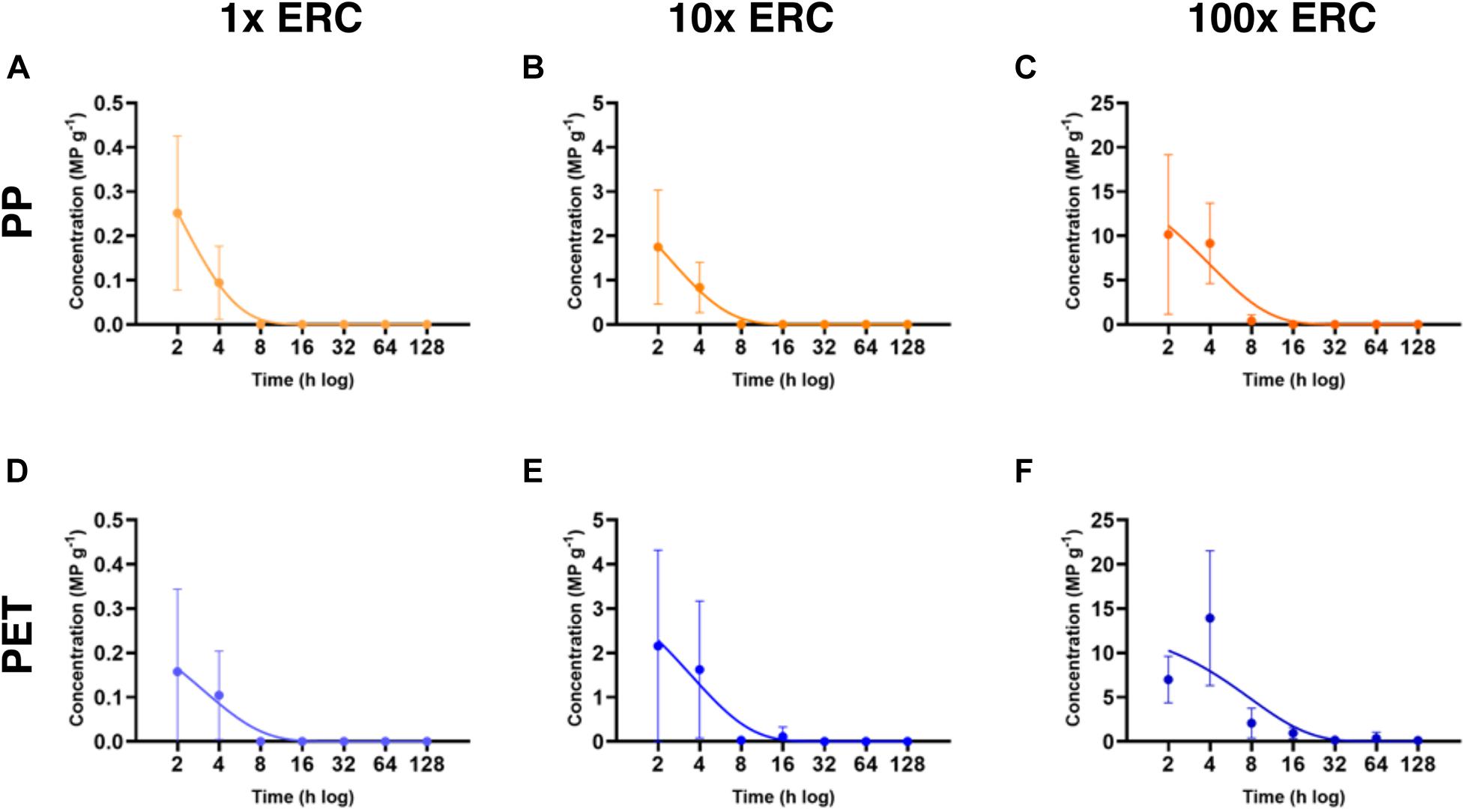
Figure 3. Mean microplastic body burden fish–1 (± standard deviation) in three treatment groups (n = 84 tanks) during a single exposure of adult damselfish Pomacentrus amboinensis to polypropylene (PP) particles and polyethylene (PET) fibers in a controlled laboratory experiment. Mean body burden is presented for four damselfish collected at each of the seven depuration times (2, 4, 8, 16, 32, 64, and 128 h). ERC, environmentally relevant concentrations. Each graph corresponds to a combination of experimental microplastic and concentration of exposure. (A) PP-ERC, (B) PP-10x ERC, (C) PP-100x ERC, (D) PET-ERC, (E) PET-10x ERC, and (F) PET-100x ERC.
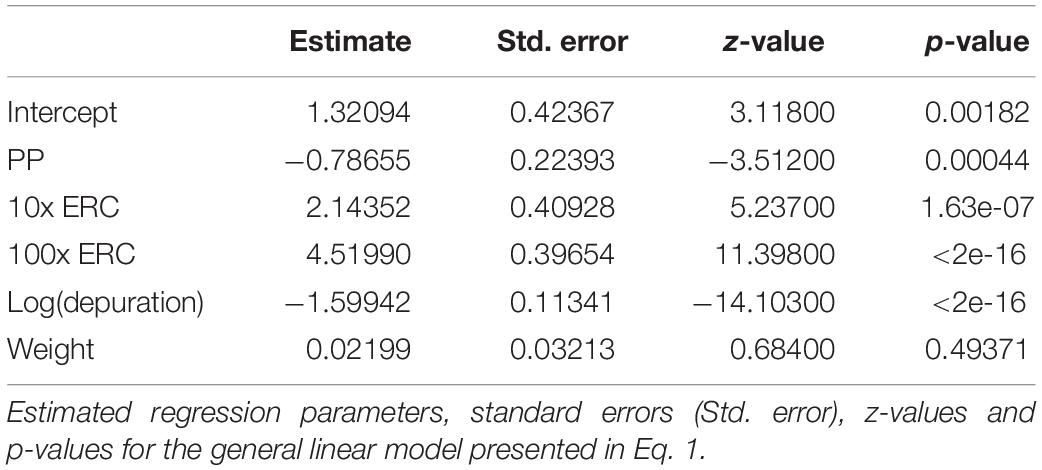
Table 5. Differences in body burden over time due to microplastic type, microplastic concentration or fish weight, following a single exposure of adult damselfish Pomacentrus amboinensis to blue polypropylene (PP) particles, and polyester (PET) fibers in a controlled laboratory experiment.
Microplastic Depuration Rates and Elimination Half-Life
Depuration rates varied from 0.13 microplastics h–1 (PET 100x ERC) to 0.52 microplastics h–1 (PP ERC) (Table 6), and were significantly influenced by microplastic type and concentration offered [Welch’s ANOVA W (5, 72.49) = 41.48, p < 0.0001] (Figure 3). PP depuration rates were significantly faster than those of PET at all concentrations tested (p < 0.05) (Supplementary Table 3). However, regardless of microplastic type encountered, depuration rates decreased with increasing concentration. Fish from the 100x ERC treatment had significantly slower depuration rates than fish from the two lower ERC treatments (p < 0.0001). The shortest elimination half-life was observed for PP particles at the lowest ERC (1.34 h); the longest was for PET fibers at 100x ERC (5.41 h) (Table 6).
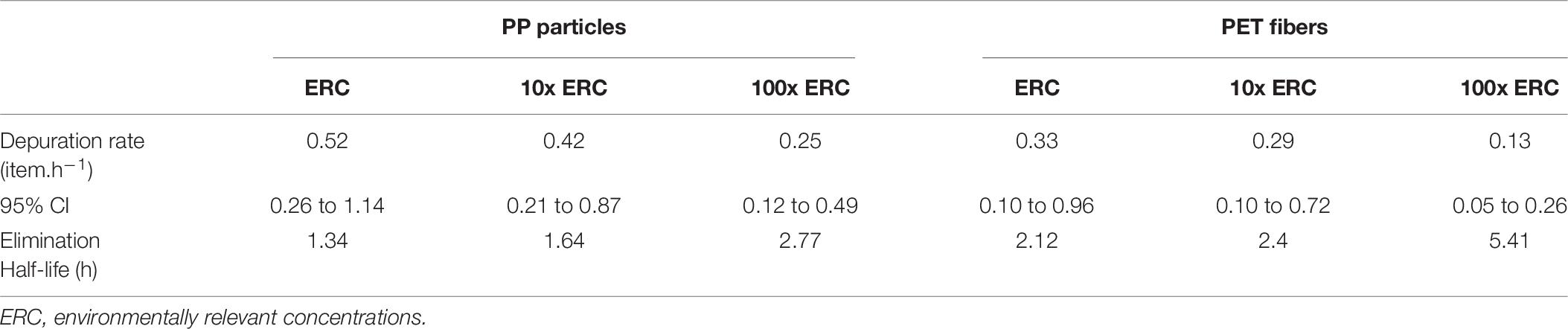
Table 6. Microplastics depuration rate (in items h–1) with 95% confidence interval (CI), and elimination half-life (h) of polypropylene (PP) particles, and polyester (PET) fibers in three treatment groups (n = 84 tanks) following a single exposure of adult damselfish Pomacentrus amboinensis in a controlled laboratory experiment.
Monitoring Contamination
No experimental microplastics (PP particles and PET fibers) were found in the GITs of the four control fish, nor in blank processing controls collected during fish dissection, GIT digestion and filtration, confirming that (cross-)contamination over the course of the study was not evident. On the other hand, extraneous putative microplastics were visually identified in the GITs of all field control, experimental control, and experimental fish (n = 92) and likewise in all blank processing controls (n = 7, one per sampling time) despite best efforts to minimize such contamination. In total, 374 putative microplastics were visually identified across all 92 fish. Forty-four putative microplastics were excluded from further analysis as polymer composition could not be determined due to the poor quality of acquired FTIR spectra. Of the remaining 330 extraneous putative microplastics, 67 matched physical and chemical characteristics of items from the contaminant library (i.e., same shape, color and ≥90% spectral match) and were deemed to have originated from sample processing activities (Figure 4A). From these items, the majority was airborne contamination (n = 56), the rest originated from clothing, including the lab coat, and was comprised of cellulose-based items (n = 11). No microplastic contamination from the experimental set up was observed (e.g., tanks, pipelines, and filtration system). The other 263 items did not match physical and chemical characteristics of items from the contaminant library, including equipment such as fish tanks and tank lids, and were deemed to come from experimental procedures (i.e., experimental air, water, or fish food). While it is possible that some of these items came with the fish from the environment, the pre-depuration time of 3–6 days should preclude this.
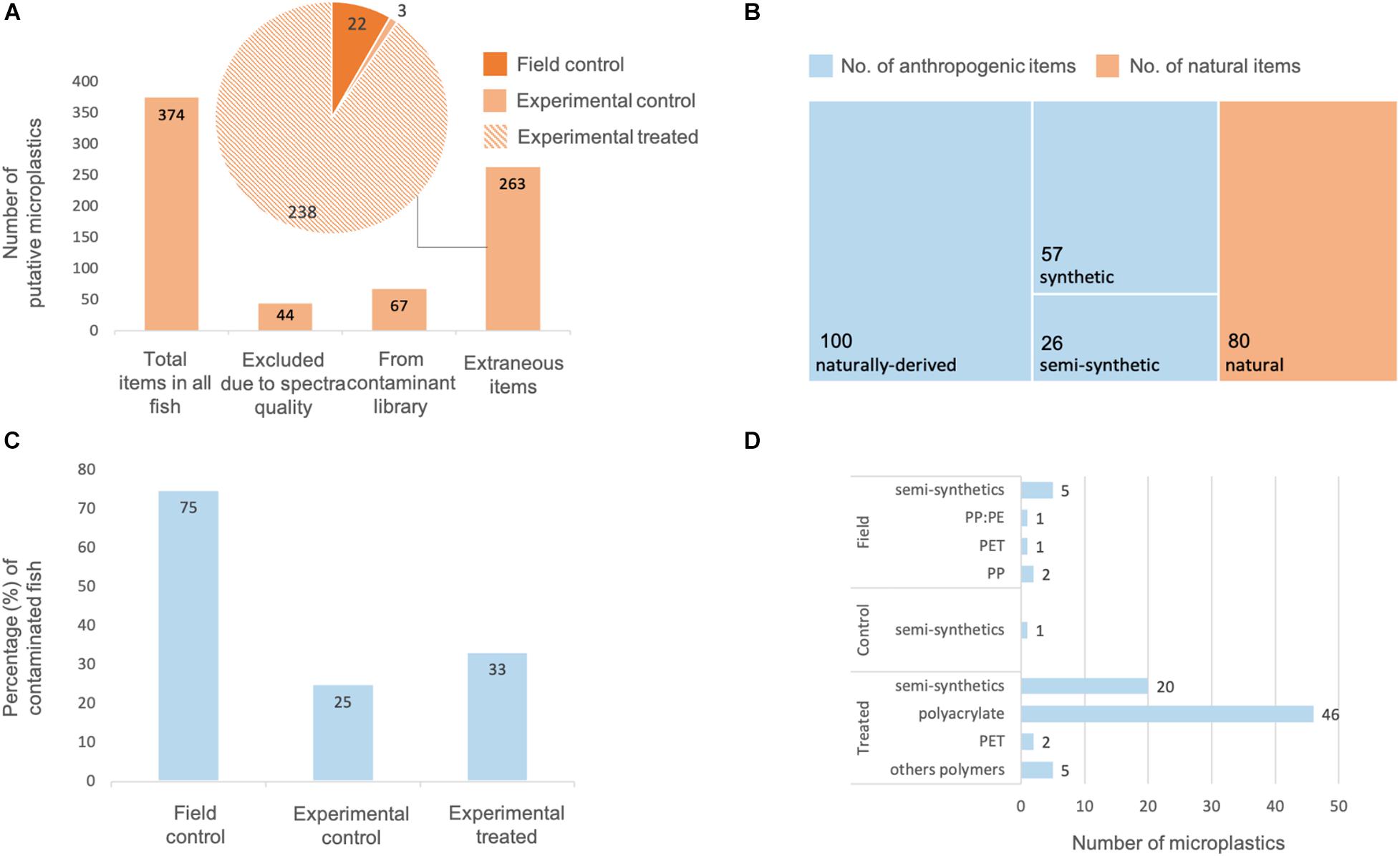
Figure 4. Quantification and potential sources of extraneous microplastic contamination throughout a single exposure study of adult damselfish Pomacentrus amboinensis to polypropylene (PP) particles and polyethylene (PET) fibers in a controlled laboratory experiment. (A) Total number of putative microplastics visually identified in the fishes’ gastrointestinal tracts (GIT), with 67 items likely originating from sample processing procedures, and 263 items deemed to come from experimental system and procedures. (B) Assignment of 263 putative microplastics to natural, and anthropogenic – naturally derived, semi-synthetic, or synthetic items. (C) Percentage of field control, experimental control, and experimental treated fish contaminated with extraneous microplastics. (D) Polymer type and number of extraneous microplastics found in field control, experimental control, and experimental treated fish.
Physical and chemical characterization of these 263 extraneous putative microplastics revealed that 57 were synthetic plastics, 26 were semi-synthetic plastics (Figure 4B), and 100 were naturally derived anthropogenic polymers. For example, many fibers were identified as being cellulose-based but were highly colored with uniform shape and/or were intertwined (i.e., anthropogenic but naturally derived). The remaining 80 items were determined to be naturally derived and a natural origin could not be discounted. Three of the four field control fish contained microplastics in their GITs including both synthetic (e.g., 2 PP, 1 PE, and 1 with both PE and PP) and semi-synthetic (e.g., 2 rayon:PET) items (Figures 4C,D). In contrast, none of the four laboratory acclimated control fish contained any synthetic items and only one contained a semi-synthetic item (cotton:rayon fiber). Similarly, the majority of laboratory exposed fish were not contaminated with microplastics (n = 56 of 84; 67%). Most of those that were contaminated with extraneous microplastics contained either one (n = 14 fish) or two (n = 9 fish) items, which were predominantly polyacrylate, polyester, and nylon-based synthetic fibers (e.g., cotton:PET, rayon:PET, and rayon:nylon). Nine synthetic items were isolated from a single treated fish, and semi-synthetic items were found in 16 treated fish. Overall, contamination of field, control and treated fish with synthetic and semi-synthetic extraneous items comprised predominantly of fibers (n = 64) rather than particles (n = 19).
Discussion
Ours is one of the first studies to investigate and confirm microplastic ingestion using environmentally relevant exposure conditions in a controlled laboratory experiment (Rochman et al., 2019), and provide strong support for microplastic contamination trends observed in marine organisms collected in the field. Specifically, microplastic ingestion by adult P. amboinensis, a planktivorous coral reef fish, occurred regardless of the microplastic polymer type (PP, PET), shape (irregular fragments, regular fibers), size (125–250 μm, 600–700 μm), or exposure concentration (ERC, 10x ERC, 100x ERC). Importantly, our measured microplastic concentrations were similar to their nominal values, confirming that PP particles and PET fibers were present at the desired concentrations and available for ingestion by individual damselfish. Both body burdens and depuration rates differed between the two experimental microplastics, with body burden of PET fibers being 2.2 times greater, and depuration rates of PET fibers being significantly lower than that for PP particles. For both microplastic types, exposure to higher concentrations led to an increase in microplastic body burden and lower depuration rates. These findings confirm ingestion of environmentally relevant PP particles and PET fibers by P. amboinensis and demonstrate for the first time the influence of microplastic characteristics and concentration on depuration rates of coral reef fish.
Ingestion of microplastic fragments and fibers has been reported for a variety of marine planktivorous fish species, both in the field (Tanaka and Takada, 2016; Compa et al., 2018; Jensen et al., 2019) and in the laboratory (Critchell and Hoogenboom, 2018; Cong et al., 2019; Xiong et al., 2019). Based on the literature, two mechanisms could explain the pattern of microplastic ingestion observed in our study: (1) fish selectively or non-randomly ingesting microplastics (Mizraji et al., 2017; Ory et al., 2018; Jensen et al., 2019; Bour et al., 2020b), or (2) fish passively or inadvertently ingesting microplastics while feeding (Roch et al., 2020). In our study, we cannot rule out selective feeding as the experimental fish were pre-starved and the biofouled experimental microplastics, likely emitting dimethyl sulfide, may have acted as an attractant as has been reported for seabirds (Savoca et al., 2016), fish (Savoca et al., 2017), and copepods (Procter et al., 2019). Conversely, passive or inadvertent ingestion may have occurred given that exposures were conducted concurrently with normal feeding, and microplastic body burden appeared proportional to the exposure concentration. Passive or inadvertent microplastic ingestion could also explain the observed variabilities in body burden within the same exposure treatments, such as those ranging from 0 to 100% in the ERC treatment. Highly variable microdebris ingestion, including microplastic ingestion, is commonly reported for fish, both in field (Compa et al., 2018; Kroon F. J. et al., 2018; Garnier et al., 2019; Jensen et al., 2019) and in experimental (Lu et al., 2016; Xiong et al., 2019; Hoang and Felix-Kim, 2020) studies. For example, adult P. amboinensis collected in the central GBR WHA contained an average of four microdebris items per individual fish, with a range from zero to 131 items (Jensen et al., 2019). In the laboratory, larvae of the same species also showed high variability in microplastic intake (McCormick et al., 2020). Together, our results and those of previous studies indicate likely but inconsistent ingestion of microplastics by fish, particularly at environmentally relevant levels of exposure. With increasing exposure concentrations, however, variability in microplastic ingestion decreased suggesting that it may become more difficult for fish like P. amboinensis to avoid ingesting microplastics inadvertently while feeding.
The depuration rate of experimental microplastics ingested by P. amboinensis was relatively short, with most being eliminated within 8 h. At this time point, PP particles, and PET fibers were only observed in the GITs of fish exposed to the 100x ERC treatment. After 16 h, PP particles were no longer observed in any of the fish, and after 128 h only two fish contained a small number of PET fibers. The observed residence time of microplastics in fish GITs suggests temporary microplastic contamination and a low likelihood of accumulation after ingestion. Although microplastics are commonly reported in coastal and marine fish collected in the field, when these studies are more closely evaluated, as in Kroon F. J. et al. (2018), the majority of individual fish examined (i.e., >80%) do not appear to be contaminated. This may indicate that microplastics are transitory in wild fish and may not accumulate at current or future ERCs (Santana et al., 2017). Nonetheless, compared with natural food, which is depurated by P. amboinensis typically within 3–5 h and at the most <10 h after ingestion (Marnane and Bellwood, 1997), PP and PET microplastics were substantially slower to traverse the GIT. Whether these differences in depuration rates between natural food and microplastics affect energy acquisition for this species is unknown, but possible in theory. For example, the longer residency time of microplastics compared to natural food items could support the hypothesis that microplastic ingestion can impact organism fitness (Besseling et al., 2013; Cole et al., 2015; Lo and Chan, 2018).
Similar or even shorter microplastic depuration periods have been reported for various aquatic organisms, including fish (Mazurais et al., 2015; Dawson et al., 2018; Xiong et al., 2019). The subtle differences in depuration periods among studies could be related to variations in experimental design, including characteristics of microplastics used, such as polymer type, shape, size, color, and concentration (Xiong et al., 2019). Furthermore, characteristics of the species being investigated (such as feeding habits, trophic levels, morphology, and life stage) may also influence ingestion and depuration rates (Bour et al., 2018; Xu X. et al., 2020). For example, P. amboinensis larvae were reported to take up to 14 h to depurate transparent PS microspheres (200–300 μm, 167 microsphere.L–1) ingested during a 1 h exposure experiment (McCormick et al., 2020). In turn, microplastic exposure (i.e., 50 PET fibers of 50–500 μm in length, and 50 PE irregular fragments >63 μm per food pellet, with no color specification) of planktivorous adult goldfish Carassius auratus resulted in similar microplastic retention as our fish species (up to 3 of 50 beads and fibers ingested after 6 days), but in slower depuration rate (Grigorakis et al., 2017). To better elucidate patterns of microplastic contamination and associated risks in marine organisms, future depuration studies should compare and investigate the role of physical and chemical properties of different microplastics, as well as biological and ecological characteristics of the species under investigation.
Our findings show that fish body burden and depuration rates were significantly affected by the experimental microplastic they were exposed to. At all doses, body burdens were lower and depuration rates faster for PP particles compared to PET fibers. Considering that our test microplastics differed in (i) polymer composition, (ii) size, and (ii) shape, any or all of these factors could potentially influence the observed results. We are not aware of any studies comparing microplastic uptake and depuration rates across different polymer compositions (Grigorakis et al., 2017). In contrast, larger microplastics have been observed to be depurated more quickly (Xiong et al., 2019), suggesting that the shape and size of our experimental microplastics may be likely explanations for the observed patterns. The slower depuration of PET fibers observed here corroborates studies that report the prevalence of fibers in wild organisms, including fish, from coastal and marine environments (Kroon F. J. et al., 2018; Jensen et al., 2019; Filgueiras et al., 2020; Xu S. et al., 2020). Moreover, it supports the hypothesis that microplastic fibers may pose a greater risk to marine organisms than microplastic particles (Au et al., 2015; Song et al., 2019), although few studies to date have examined potential biological effects of microplastic fibers. Accumulation and entanglement of fibers in the GIT has been observed in fish (Jensen et al., 2019) and may impact the gut passage time of microplastics in fish and other marine organisms (Welden and Cowie, 2016; Xiong et al., 2019). Xiong et al. (2019) also suggested that the presence of food along with microplastics in the GIT can increase microplastic retention. In the present study, clumps of PET fibers entangled with gut contents were occasionally found in the GIT of exposed fish (Figure 2), however, they were not observed consistently enough to confidently state that they contributed to the differences in depuration rates. Another hypothesis is that the GIT morphology may contribute to retention of fibers, potentially influencing fiber gut passage time (Welden and Cowie, 2016); whether this is true for P. amboinensis remains to be determined.
For both experimental microplastics, body burdens and depuration rates increased with increasing exposure concentrations. This is opposite to patterns for natural food which moves faster through fish GITs with increasing concentration (German, 2011). This suggests that microplastic ingestion, and potential effects on planktivorous fish like P. amboinensis, are strongly influenced by the amount of microplastics present in their environment (Ding et al., 2018; Roch et al., 2020). Thus, higher risk of biological contamination and subsequent effects are likely associated with environments that are more contaminated (Everaert et al., 2020), such as accumulation zones [e.g., gyres (Eriksen et al., 2013) and benthic habitats (Ogata et al., 2009; Barrett et al., 2020)], as well as areas adjacent to highly populated regions or areas of industrial (Li et al., 2020) and commercial fishery (Xue et al., 2020) interest. Similarly, our findings are consistent with predictions that microplastic body burdens will increase in marine fish along with projected increases in marine microplastic contamination (Jambeck et al., 2015; Geyer et al., 2017; Everaert et al., 2020), driven by the estimated 400% rise in annual plastic production by 2100 (Everaert et al., 2020).
Complete elimination of contamination with extraneous microplastics is challenging (Prata et al., 2021) and efforts to do so are rarely quantified and reported on in microplastic exposure studies (e.g., Nanninga et al., 2020; Zhao et al., 2020). Ours is the first to quantify extraneous microplastics in the GITs of individual fish and link them to potential sources (i.e., field, experimental system or sample processing procedures) to discuss potential elimination strategies. Control fish collected and euthanized in the field contained both synthetic and semi-synthetic extraneous items different to those found in experimental control and exposed fish. This corroborates that experimental fish had depurated any microplastics brought in from the field during acclimation. Despite measures put in place to prevent contamination, 67 extraneous putative microplastics were retrieved from experimental control and exposed fish, with 20% of these putative microplastics linked with items used and recovered during sample processing procedures, specifically airborne items and clothing. All clothing, including lab coats, worn during this study were cellulose-based, thus were not considered further. Nevertheless, monitoring it highlighted how important is to avoid synthetic and semi-synthetic clothing while conducting microplastic studies, including experimental procedures. The remaining 80%, primarily polyacrylate and semi-synthetics fibers, could not be linked to specific items and is assumed to have originated from the experimental air, water or fish food. Whether these extraneous microplastics influenced the ingestion and depuration rates of experimental microplastics remains unknown. Our exposure study was conducted in a general use laboratory at a remote field research station, and access to sophisticated filtration systems to clarify incoming air and seawater, was not available. Similar limitations could be faced by many experimental researchers, however, these are not frequently considered or discussed in the microplastic literature (Prata et al., 2021). Our results highlight the need for elimination of contamination sources where possible, and importantly for enhanced data quality control through robust study design with relevant experimental controls and stringent monitoring of background contamination. This is particularly important for microplastic studies using exposure conditions that reflect ERCs.
In summary, this study confirmed that the planktivorous damselfish P. amboinensis ingests environmentally relevant types (specifically irregular shaped blue PP particles and PET fibers) and concentrations of microplastics. After a single exposure to experimental microplastics, the majority of PP particles and PET fibers were eliminated from the GIT within 8 h, with most items completely purged by 128 h. Damselfish ingested both experimental microplastics at all concentrations, with body burden of PET fibers being 2.2 times greater, and depuration rates of PET fibers significantly lower than that for PP particles. For both microplastic types, exposure to higher concentrations led to an increase in body burden and lower depuration rates. These results corroborate the higher abundance of microplastic fibers versus particles reported in many wild-caught marine organisms, and highlight the need for more research on the impacts of microplastic fibers on marine organisms. Our findings also support the hypothesis that environments with higher levels of microplastic contamination (e.g., current “hotspots”) pose a greater risk to marine organisms due to increased microplastic ingestion and prolonged depuration rates, and that sporadic exposure to microplastics might have lower microplastic-associated risks. Finally, despite measures put in place to prevent contamination extraneous microplastics were recovered from experimental fish, highlighting the challenge to completely eliminate contamination in microplastic exposure studies. This is of particular concern for experiments examining the impacts of environmentally relevant microplastic exposure. We strongly recommend that controlled exposure studies quantify and report on contamination with extraneous microplastics to inform and continuously improve protocols for future microplastics research.
Data Availability Statement
The original contributions presented in the study are included in the article/Supplementary Material, further inquiries can be directed to the corresponding author.
Ethics Statement
The animal study was reviewed and approved by the James Cook University Animal Ethics Committee (Approval Number A2635).
Author Contributions
MS obtained funding, conceptualized the study, conducted field and laboratory work, analyzed the data, wrote the original draft, and reviewed and edited further versions of the manuscript. AD contributed to study design, analyzed the data, and contributed to reviewing and editing the manuscript. CM contributed to study design and reviewing and editing the manuscript. LH contributed to developing field relevant experimental research at the outset and to reviewing and editing the final manuscript. CL conducted field and laboratory work. FK obtained funding, contributed to study design, conducted field work, and contributed to reviewing and editing the manuscript. All authors contributed to the article and approved the submitted version.
Funding
This work was supported by the Australian Institute of Marine Science and James Cook University (AIMS@JCU Ph.D. Scholarship to MS); Australian Museum and Lizard Island Research Station (2018 Rossi Foundation Grant to MS); National Environmental Science Program Tropical Water Quality Hub (TWQ Hub, Ph.D. funding to MS); the Key Program for International S&T Cooperation Projects: Sino-Australian Centre for Healthy Coasts (No. 2016YFE0101500), as well as the Australia China Science Research Fund Grant ACSRF 48162, Australian Government Department of Industry, Innovation and Science, and the Queensland Government Department of Environment and Science (AD and FK); and the Australian Institute of Marine Science (AD, CM, CL, and FK).
Conflict of Interest
The authors declare that the research was conducted in the absence of any commercial or financial relationships that could be construed as a potential conflict of interest.
Acknowledgments
The authors would like to thank all funding sources listed under Funding for the support given to conduct this study, including field trip and sample collection activities, experimental work, and sample processing. The authors acknowledge the Dingaal people, the Traditional Owners of Lizard Island, the Wulgurukaba and Bindal people, and the Traditional Owners of Townsville, where this study was conducted. Thank you also to the constructive input from two reviewers of this work, which improved the final submission for publication.
Supplementary Material
The Supplementary Material for this article can be found online at: https://www.frontiersin.org/articles/10.3389/fenvs.2021.641135/full#supplementary-material
References
Abayomi, O. A., Range, P., Al-Ghouti, M. A., Obbard, J. P., Almeer, S. H., and Ben-Hamadou, R. (2017). Microplastics in coastal environments of the Arabian Gulf. Mar. Pollut. Bull. 124, 181–188. doi: 10.1016/j.marpolbul.2017.07.011
Amoroso, M. G., Langellotti, A. L., Russo, V., Martello, A., Monini, M., Di Bartolo, I., et al. (2020). Accumulation and depuration kinetics of rotavirus in mussels experimentally contaminated. Food Environ. Virol. 12, 48–57. doi: 10.1007/s12560-019-09413-0
Au, S. Y., Bruce, T. F., Bridges, W. C., and Klaine, S. J. (2015). Responses of Hyalella azteca to acute and chronic microplastic exposures. Environ. Toxicol. Chem. 34, 2564–2572. doi: 10.1002/etc.3093
Barrett, J., Chase, Z., Zhang, J., Holl, M. M. B., Willis, K., Williams, A., et al. (2020). Microplastic pollution in deep-sea sediments from the great australian bight. Front. Mar. Sci. 7:576170. doi: 10.3389/fmars.2020.576170
Besseling, E., Wegner, A., Foekema, E. M., Van Den Heuvel-Greve, M. J., and Koelmans, A. A. (2013). Effects of microplastic on fitness and PCB bioaccumulation by the lugworm Arenicola marina (L.). Environ. Sci. Technol. 47, 593–600. doi: 10.1021/es302763x
Blanco, J., Alvarez, G., Rengel, J., Diaz, R., Marino, C., Martin, H., et al. (2018). Accumulation and biotransformation of dinophysis toxins by the surf clam Mesodesma donacium. Toxins (Basel) 10:314. doi: 10.3390/toxins10080314
Bour, A., Avio, C. G., Gorbi, S., Regoli, F., and Hylland, K. (2018). Presence of microplastics in benthic and epibenthic organisms: Influence of habitat, feeding mode and trophic level. Environ. Pollut. 243, 1217–1225. doi: 10.1016/j.envpol.2018.09.115
Bour, A., Hossain, S., Taylor, M., Sumner, M., and Almroth, B. C. (2020a). Synthetic microfiber and microbead exposure and retention time in model aquatic species under different exposure scenarios. Front. Environ. Sci. 8:83. doi: 10.3389/fenvs.2020.00083
Bour, A., Sturve, J., Höjesjö, J., and Carney Almroth, B. (2020b). Microplastic vector effects: are fish at risk when exposed via the trophic chain? Front. Environ. Sci. 8:90. doi: 10.3389/fenvs.2020.00090
Chae, Y., and An, Y. J. (2020). Effects of food presence on microplastic ingestion and egestion in Mytilus galloprovincialis. Chemosphere 240:124855. doi: 10.1016/j.chemosphere.2019.124855
Cole, M., Lindeque, P., Fileman, E., Halsband, C., and Galloway, T. S. (2015). The impact of polystyrene microplastics on feeding, function and fecundity in the marine copepod Calanus helgolandicus. Environ. Sci. Technol. 49, 1130–1137. doi: 10.1021/es504525u
Cole, M., Webb, H., Lindeque, P. K., Fileman, E. S., Halsband, C., and Galloway, T. S. (2014). Isolation of microplastics in biota-rich seawater samples and marine organisms. Sci. Rep. 4:4528.
Compa, M., Ventero, A., Iglesias, M., and Deudero, S. (2018). Ingestion of microplastics and natural fibres in Sardina pilchardus (Walbaum, 1792) and Engraulis encrasicolus (Linnaeus, 1758) along the Spanish Mediterranean coast. Mar. Pollut. Bull. 128, 89–96. doi: 10.1016/j.marpolbul.2018.01.009
Cong, Y., Jin, F., Tian, M., Wang, J., Shi, H., Wang, Y., et al. (2019). Ingestion, egestion and post-exposure effects of polystyrene microspheres on marine medaka (Oryzias melastigma). Chemosphere 228, 93–100. doi: 10.1016/j.chemosphere.2019.04.098
Coyle, R., Hardiman, G., and Driscoll, K. O. (2020). Microplastics in the marine environment: A review of their sources, distribution processes and uptake into ecosystems. Case Stud. Chem. Environ. Eng. 2:100010. doi: 10.1016/j.cscee.2020.100010
Cozar, A., Echevarria, F., Gonzalez-Gordillo, J. I., Irigoien, X., Ubeda, B., Hernandez-Leon, S., et al. (2014). Plastic debris in the open ocean. Proc. Natl. Acad. Sci. U.S.A. 111, 10239–10244.
Critchell, K., and Hoogenboom, M. O. (2018). Effects of microplastic exposure on the body condition and behaviour of planktivorous reef fish (Acanthochromis polyacanthus). PLoS One 13:e0193308. doi: 10.1371/journal.pone.0193308
Dawson, A., Huston, W., Kawaguchi, S., King, C., Cropp, R., Wild, S., et al. (2018). Uptake and Depuration Kinetics Influence Microplastic Bioaccumulation and Toxicity in Antarctic Krill (Euphausia superba). Environ. Sci. Technol. 52, 3195–3201. doi: 10.1021/acs.est.7b05759
Dawson, A. L., Motti, C. A., and Kroon, F. J. (2020). Solving a sticky situation: microplastic analysis of lipid-rich tissue. Front. Environ. Sci. 8:563565. doi: 10.3389/fenvs.2020.563565
Ding, J., Zhang, S., Razanajatovo, R. M., Zou, H., and Zhu, W. (2018). Accumulation, tissue distribution, and biochemical effects of polystyrene microplastics in the freshwater fish red tilapia (Oreochromis niloticus). Environ. Pollut. 238, 1–9. doi: 10.1016/j.envpol.2018.03.001
Ehlers, S. M., Maxein, J., and Koop, J. H. E. (2020). Low-cost microplastic visualization in feeding experiments using an ultraviolet light-emitting flashlight. Ecol. Res. 35, 265–273. doi: 10.1111/1440-1703.12080
Elizalde-Velazquez, A., Carcano, A. M., Crago, J., Green, M. J., Shah, S. A., and Canas-Carrell, J. E. (2020). Translocation, trophic transfer, accumulation and depuration of polystyrene microplastics in Daphnia magna and Pimephales promelas. Environ. Pollut. 259, 113937. doi: 10.1016/j.envpol.2020.113937
Emslie, M. J., Logan, M., and Cheal, A. J. (2019). The distribution of Planktivorous damselfishes (Pomacentridae) on the great barrier reef and the relative influences of habitat and predation. Diversity 11:33. doi: 10.3390/d11030033
Eriksen, M., Maximenko, N., Thiel, M., Cummins, A., Lattin, G., Wilson, S., et al. (2013). Plastic pollution in the South Pacific subtropical gyre. Mar. Pollut. Bull. 68, 71–76. doi: 10.1016/j.marpolbul.2012.12.021
Espinosa, C., Cuesta, A., and Esteban, M. A. (2017). Effects of dietary polyvinylchloride microparticles on general health, immune status and expression of several genes related to stress in gilthead seabream (Sparus aurata L.). Fish Shellfish Immunol. 68, 251–259. doi: 10.1016/j.fsi.2017.07.006
Everaert, G., De Rijcke, M., Lonneville, B., Janssen, C. R., Backhaus, T., Mees, J., et al. (2020). Risks of floating microplastic in the global ocean. Environ. Pollut. 267, 115499. doi: 10.1016/j.envpol.2020.115499
Fernandez, B., and Albentosa, M. (2019a). Dynamic of small polyethylene microplastics (</=10mum) in mussel’s tissues. Mar. Pollut. Bull. 146, 493–501. doi: 10.1016/j.marpolbul.2019.06.021
Fernandez, B., and Albentosa, M. (2019b). Insights into the uptake, elimination and accumulation of microplastics in mussel. Environ. Pollut. 249, 321–329. doi: 10.1016/j.envpol.2019.03.037
Filgueiras, A. V., Preciado, I., Carton, A., and Gago, J. (2020). Microplastic ingestion by pelagic and benthic fish and diet composition: a case study in the NW Iberian shelf. Mar. Pollut. Bull. 160:111623. doi: 10.1016/j.marpolbul.2020.111623
Frydkjaer, C. K., Iversen, N., and Roslev, P. (2017). Ingestion and egestion of microplastics by the cladoceran Daphnia magna: effects of regular and irregular shaped plastic and Sorbed Phenanthrene. Bull. Environ. Contam. Toxicol. 99, 655–661. doi: 10.1007/s00128-017-2186-3
Garnier, Y., Jacob, H., Guerra, A. S., Bertucci, F., and Lecchini, D. (2019). Evaluation of microplastic ingestion by tropical fish from Moorea Island, French Polynesia. Mar. Pollut. Bull. 140, 165–170. doi: 10.1016/j.marpolbul.2019.01.038
German, D. P. (2011). “Digestive efficiency,” in Encyclopedia of Fish Physiology: From Genome to Environment, ed. A. P. Farrell (Amsterdam: Elsevier), 1596–1607. doi: 10.1016/b978-0-12-374553-8.00142-8
GESAMP (2019). in Guidelines for the Monitoring and Assessment of Plastic Litter in the Ocean, eds P. Kershaw, A. Turra, and F. Galgani (Nairobi: United Nations Environmental Program).
Geyer, R., Jambeck, J. R., and Law, K. L. (2017). Production, use, and fate of all plastics ever made. Sci. Adv. 3:e1700782. doi: 10.1126/sciadv.1700782
Goncalves, C., Martins, M., Sobral, P., Costa, P. M., and Costa, M. H. (2019). An assessment of the ability to ingest and excrete microplastics by filter-feeders: a case study with the Mediterranean mussel. Environ. Pollut. 245, 600–606. doi: 10.1016/j.envpol.2018.11.038
Graham, P., Palazzo, L., Andrea De Lucia, G., Telfer, T. C., Baroli, M., and Carboni, S. (2019). Microplastics uptake and egestion dynamics in Pacific oysters, Magallana gigas (Thunberg, 1793), under controlled conditions. Environ. Pollut. 252, 742–748. doi: 10.1016/j.envpol.2019.06.002
Grigorakis, S., Mason, S. A., and Drouillard, K. G. (2017). Determination of the gut retention of plastic microbeads and microfibers in goldfish (Carassius auratus). Chemosphere 169, 233–238. doi: 10.1016/j.chemosphere.2016.11.055
Hall, N. M., Berry, K. L. E., Rintoul, L., and Hoogenboom, M. O. (2015). Microplastic ingestion by scleractinian corals. Mar. Biol. 162, 725–732. doi: 10.1007/s00227-015-2619-7
Halstead, J. E., Smith, J. A., Carter, E. A., Lay, P. A., and Johnston, E. L. (2018). Assessment tools for microplastics and natural fibres ingested by fish in an urbanised estuary. Environ. Pollut. 234, 552–561. doi: 10.1016/j.envpol.2017.11.085
Hassell, K. L., Coggan, T. L., Cresswell, T., Kolobaric, A., Berry, K., Crosbie, N. D., et al. (2020). Dietary uptake and depuration kinetics of perfluorooctane sulfonate, perfluorooctanoic acid, and hexafluoropropylene oxide dimer acid (GenX) in a benthic fish. Environ. Toxicol. Chem. 39, 595–603. doi: 10.1002/etc.4640
Hendriks, A. J., and Heikens, A. (2001). The power of size. 2. Rate constants and equilibrium ratios for accumulation of inorganic substances related to species weight. Environ. Toxicol. Chem. 20, 1421–1437. doi: 10.1002/etc.5620200704
Hidalgo-Ruz, V., Gutow, L., Thompson, R. C., and Thiel, M. (2012). Microplastics in the marine environment: a review of the methods used for identification and quantification. Environ. Sci. Technol. 46, 3060–3075. doi: 10.1021/es2031505
Hoang, T. C., and Felix-Kim, M. (2020). Microplastic consumption and excretion by fathead minnows (Pimephales promelas): influence of particles size and body shape of fish. Sci. Total Environ. 704, 135433. doi: 10.1016/j.scitotenv.2019.135433
Hu, L., Su, L., Xue, Y., Mu, J., Zhu, J., Xu, J., et al. (2016). Uptake, accumulation and elimination of polystyrene microspheres in tadpoles of Xenopus tropicalis. Chemosphere 164, 611–617. doi: 10.1016/j.chemosphere.2016.09.002
Jambeck, J. R., Geyer, R., Wilcox, C., Siegler, T. R., Perryman, M., Andrady, A., et al. (2015). Plastic waste inputs from land into the ocean. Science 347, 768–771. doi: 10.1126/science.1260352
Jensen, L. H., Motti, C. A., Garm, A. L., Tonin, H., and Kroon, F. J. (2019). Sources, distribution and fate of microfibres on the Great Barrier Reef, Australia. Sci. Rep. 9:9021.
Jeong, C. B., Won, E. J., Kang, H. M., Lee, M. C., Hwang, D. S., Hwang, U. K., et al. (2016). Microplastic size-dependent toxicity, oxidative stress induction, and p-JNK and p-p38 activation in the monogonont rotifer (Brachionus koreanus). Environ. Sci. Technol. 50, 8849–8857. doi: 10.1021/acs.est.6b01441
Kanhai, D. K., Officer, R., Lyashevska, O., Thompson, R. C., and O’connor, I. (2017). Microplastic abundance, distribution and composition along a latitudinal gradient in the Atlantic Ocean. Mar. Pollut. Bull. 115, 307–314. doi: 10.1016/j.marpolbul.2016.12.025
Kaposi, K. L., Mos, B., Kelaher, B. P., and Dworjanyn, S. A. (2014). Ingestion of microplastic has limited impact on a marine larva. Environ. Sci. Technol. 48, 1638–1645. doi: 10.1021/es404295e
Karami, A., Golieskardi, A., Ho, Y. B., Larat, V., and Salamatinia, B. (2017). Microplastics in eviscerated flesh and excised organs of dried fish. Sci. Rep. 7:5473.
Kroon, F., Motti, C., Talbot, S., Sobral, P., and Puotinen, M. (2018). A workflow for improving estimates of microplastic contamination in marine waters: a case study from North-Western Australia. Environ. Pollut. 238, 26–38. doi: 10.1016/j.envpol.2018.03.010
Kroon, F. J. (2015). The efficacy of clove oil for anaesthesia of eight species of Australian tropical freshwater teleosts. Limnol. Oceanogr. Methods 13, 463–475. doi: 10.1002/lom3.10040
Kroon, F. J., Motti, C. E., Jensen, L. H., and Berry, K. L. E. (2018). Classification of marine microdebris: a review and case study on fish from the Great Barrier Reef, Australia. Sci. Rep. 8:16422.
Kulcsár, D. (2019). Microplastic Ingestion and Selectivity in Anemonefish (Amphiprion melanopus) from Field Observations (Great Barrier Reef) and Controlled Laboratory Exposures. Ph. D. Thesis, University of Copenhagen, Copenhagen.
Lebrun, J. D., Leroy, D., Giusti, A., Gourlay-France, C., and Thome, J. P. (2014). Bioaccumulation of polybrominated diphenyl ethers (PBDEs) in Gammarus pulex: relative importance of different exposure routes and multipathway modeling. Aquat. Toxicol. 154, 107–113. doi: 10.1016/j.aquatox.2014.05.015
Li, B., Su, L., Zhang, H., Deng, H., Chen, Q., and Shi, H. (2020). Microplastics in fishes and their living environments surrounding a plastic production area. Sci. Total Environ. 727:138662. doi: 10.1016/j.scitotenv.2020.138662
Liu, W., Zhao, Y., Shi, Z., Li, Z., and Liang, X. (2020). Ecotoxicoproteomic assessment of microplastics and plastic additives in aquatic organisms: a review. Comp. Biochem. Physiol. Part D Genomics Proteom. 36:100713. doi: 10.1016/j.cbd.2020.100713
Lo, H. K. A., and Chan, K. Y. K. (2018). Negative effects of microplastic exposure on growth and development of Crepidula onyx. Environ. Pollut. 233, 588–595. doi: 10.1016/j.envpol.2017.10.095
Lu, Y., Zhang, Y., Deng, Y., Jiang, W., Zhao, Y., Geng, J., et al. (2016). Uptake and accumulation of polystyrene microplastics in zebrafish (Danio rerio) and toxic effects in liver. Environ. Sci. Technol. 50, 4054–4060. doi: 10.1021/acs.est.6b00183
Lusher, A. (2015). “Microplastics in the marine environment: distribution, interactions and effects,” in Marine Anthropogenic Litter, eds M. Bergmann, L. Gutow, and M. Klages (Cham: Springer), 245–307. doi: 10.1007/978-3-319-16510-3_10
Manabe, M., Tatarazako, N., and Kinoshita, M. (2011). Uptake, excretion and toxicity of nano-sized latex particles on medaka (Oryzias latipes) embryos and larvae. Aquat. Toxicol. 105, 576–581. doi: 10.1016/j.aquatox.2011.08.020
Marnane, M. J., and Bellwood, D. R. (1997). Marker technique for investigating gut throughput rates in coral reef®shes. Mar. Biol. 129, 15–22. doi: 10.1007/s002270050141
Mazurais, D., Ernande, B., Quazuguel, P., Severe, A., Huelvan, C., Madec, L., et al. (2015). Evaluation of the impact of polyethylene microbeads ingestion in European sea bass (Dicentrarchus labrax) larvae. Mar. Environ. Res. 112, 78–85. doi: 10.1016/j.marenvres.2015.09.009
McCormick, M. I., Chivers, D. P., Ferrari, M. C. O., Blandford, M. I., Nanninga, G. B., Richardson, C., et al. (2020). Microplastic exposure interacts with habitat degradation to affect behaviour and survival of juvenile fish in the field. Proc. Biol. Sci. 287:20201947. doi: 10.1098/rspb.2020.1947
McCormick, M. I., and Weaver, C. J. (2012). It pays to be pushy: intracohort interference competition between two reef fishes. PLoS One 7:e42590. doi: 10.1371/journal.pone.0042590
Miller, M. E., Hamann, M., and Kroon, F. J. (2020). Bioaccumulation and biomagnification of microplastics in marine organisms: a review and meta-analysis of current data. PLoS One 15:e0240792. doi: 10.1371/journal.pone.0240792
Miller, T. H., Mceneff, G. L., Stott, L. C., Owen, S. F., Bury, N. R., and Barron, L. P. (2016). Assessing the reliability of uptake and elimination kinetics modelling approaches for estimating bioconcentration factors in the freshwater invertebrate, Gammarus pulex. Sci. Total Environ. 547, 396–404. doi: 10.1016/j.scitotenv.2015.12.145
Mizraji, R., Ahrendt, C., Perez-Venegas, D., Vargas, J., Pulgar, J., Aldana, M., et al. (2017). Is the feeding type related with the content of microplastics in intertidal fish gut? Mar. Pollut. Bull. 116, 498–500. doi: 10.1016/j.marpolbul.2017.01.008
Nanninga, G. B., Scott, A., and Manica, A. (2020). Microplastic ingestion rates are phenotype-dependent in juvenile anemonefish. Environ. Pollut. 259:113855. doi: 10.1016/j.envpol.2019.113855
Newman, M. C. (2012). “Bioaccumulation,” in Quantitative Ecotoxicology, ed. M. C. Newman (Boca Raton, FL: CRC Press), 77–138.
Ogata, Y., Takada, H., Mizukawa, K., Hirai, H., Iwasa, S., Endo, S., et al. (2009). International Pellet Watch: global monitoring of persistent organic pollutants (POPs) in coastal waters. 1. Initial phase data on PCBs, DDTs, and HCHs. Mar. Pollut. Bull. 58, 1437–1446. doi: 10.1016/j.marpolbul.2009.06.014
Ory, N., Chagnon, C., Felix, F., Fernandez, C., Ferreira, J. L., Gallardo, C., et al. (2018). Low prevalence of microplastic contamination in planktivorous fish species from the southeast Pacific Ocean. Mar. Pollut. Bull. 127, 211–216. doi: 10.1016/j.marpolbul.2017.12.016
Prata, J. C., Reis, V., Da Costa, J. P., Mouneyrac, C., Duarte, A. C., and Rocha-Santos, T. (2021). Contamination issues as a challenge in quality control and quality assurance in microplastics analytics. J. Hazard. Mater. 403:123660. doi: 10.1016/j.jhazmat.2020.123660
Procter, J., Hopkins, F. E., Fileman, E. S., and Lindeque, P. K. (2019). Smells good enough to eat: Dimethyl sulfide (DMS) enhances copepod ingestion of microplastics. Mar. Pollut. Bull. 138, 1–6. doi: 10.1016/j.marpolbul.2018.11.014
Qu, X., Su, L., Li, H., Liang, M., and Shi, H. (2018). Assessing the relationship between the abundance and properties of microplastics in water and in mussels. Sci. Total Environ. 621, 679–686. doi: 10.1016/j.scitotenv.2017.11.284
Reisser, J., Shaw, J., Wilcox, C., Hardesty, B. D., Proietti, M., Thums, M., et al. (2013). Marine plastic pollution in waters around Australia: characteristics, concentrations, and pathways. PLoS One 8:e80466. doi: 10.1371/journal.pone.0080466
Rist, S., Baun, A., and Hartmann, N. B. (2017). Ingestion of micro- and nanoplastics in Daphnia magna – Quantification of body burdens and assessment of feeding rates and reproduction. Environ. Pollut. 228, 398–407. doi: 10.1016/j.envpol.2017.05.048
Rist, S., Steensgaard, I. M., Guven, O., Nielsen, T. G., Jensen, L. H., Moller, L. F., et al. (2019). The fate of microplastics during uptake and depuration phases in a blue mussel exposure system. Environ. Toxicol. Chem. 38, 99–105. doi: 10.1002/etc.4285
Roch, S., Friedrich, C., and Brinker, A. (2020). Uptake routes of microplastics in fishes: practical and theoretical approaches to test existing theories. Sci. Rep. 10:3896.
Rochman, C. M., Brookson, C., Bikker, J., Djuric, N., Earn, A., Bucci, K., et al. (2019). Rethinking microplastics as a diverse contaminant suite. Environ. Toxicol. Chem. 38, 703–711. doi: 10.1002/etc.4371
Santana, M. F. M., Moreira, F. T., and Turra, A. (2017). Trophic transference of microplastics under a low exposure scenario: insights on the likelihood of particle cascading along marine food-webs. Mar. Pollut. Bull. 121, 154–159. doi: 10.1016/j.marpolbul.2017.05.061
Savoca, M. S., Tyson, C. W., Mcgill, M., and Slager, C. J. (2017). Odours from marine plastic debris induce food search behaviours in a forage fish. Proc. R. Soc. B Biol. Sci. 284:20171000.
Savoca, M. S., Wohlfeil, M. E., Ebeler, S. E., and Nevitt, G. A. (2016). Marine plastic debris emits a keystone infochemical for olfactory foraging seabirds. Sci. Adv. 2:e1600395. doi: 10.1126/sciadv.1600395
Schlawinsky, M. (2020). The Intake of Microplastic debris by Marine Organisms on the Great Barrier Reef. Ph. D. Thesis, Oldenburg University, Oldenburg.
Song, Y., Cao, C., Qiu, R., Hu, J., Liu, M., Lu, S., et al. (2019). Uptake and adverse effects of polyethylene terephthalate microplastics fibers on terrestrial snails (Achatina fulica) after soil exposure. Environ. Pollut. 250, 447–455. doi: 10.1016/j.envpol.2019.04.066
Syakti, A. D., Bouhroum, R., Hidayati, N. V., Koenawan, C. J., Boulkamh, A., Sulistyo, I., et al. (2017). Beach macro-litter monitoring and floating microplastic in a coastal area of Indonesia. Mar. Pollut. Bull. 122, 217–225. doi: 10.1016/j.marpolbul.2017.06.046
Tanaka, K., and Takada, H. (2016). Microplastic fragments and microbeads in digestive tracts of planktivorous fish from urban coastal waters. Sci. Rep. 6:34351.
van Sebille, E., Wilcox, C., Lebreton, L., Maximenko, N., Hardesty, B. D., Van Franeker, J. A., et al. (2015). A global inventory of small floating plastic debris. Environ. Res. Lett. 10:124006. doi: 10.1088/1748-9326/10/12/124006
Wang, X., Huang, W., Wei, S., Shang, Y., Gu, H., Wu, F., et al. (2019). Microplastics impair digestive performance but show little effects on antioxidant activity in mussels under low pH conditions. Environ. Pollut. 258:113691. doi: 10.1016/j.envpol.2019.113691
Wang, Y., Mao, Z., Zhang, M., Ding, G., Sun, J., Du, M., et al. (2019). The uptake and elimination of polystyrene microplastics by the brine shrimp, Artemia parthenogenetica, and its impact on its feeding behavior and intestinal histology. Chemosphere 234, 123–131. doi: 10.1016/j.chemosphere.2019.05.267
Watts, A. J., Lewis, C., Goodhead, R. M., Beckett, S. J., Moger, J., Tyler, C. R., et al. (2014). Uptake and retention of microplastics by the shore crab Carcinus maenas. Environ. Sci. Technol. 48, 8823–8830.
Welden, N. A. C., and Cowie, P. R. (2016). Environment and gut morphology influence microplastic retention in langoustine, Nephrops norvegicus. Environ. Pollut. 214, 859–865. doi: 10.1016/j.envpol.2016.03.067
Woods, M. N., Stack, M. E., Fields, D. M., Shaw, S. D., and Matrai, P. A. (2018). Microplastic fiber uptake, ingestion, and egestion rates in the blue mussel (Mytilus edulis). Mar. Pollut. Bull. 137, 638–645. doi: 10.1016/j.marpolbul.2018.10.061
Xiong, X., Tu, Y., Chen, X., Jiang, X., Shi, H., Wu, C., et al. (2019). Ingestion and egestion of polyethylene microplastics by goldfish (Carassius auratus): influence of color and morphological features. Heliyon 5:e03063. doi: 10.1016/j.heliyon.2019.e03063
Xu, S., Ma, J., Ji, R., Pan, K., and Miao, A. J. (2020). Microplastics in aquatic environments: occurrence, accumulation, and biological effects. Sci. Total Environ. 703:134699. doi: 10.1016/j.scitotenv.2019.134699
Xu, X., Wong, C. Y., Tam, N. F. Y., Lo, H. S., and Cheung, S. G. (2020). Microplastics in invertebrates on soft shores in Hong Kong: Influence of habitat, taxa and feeding mode. Sci. Total Environ. 715:136999. doi: 10.1016/j.scitotenv.2020.136999
Xue, B., Zhang, L., Li, R., Wang, Y., Guo, J., Yu, K., et al. (2020). Underestimated microplastic pollution derived from fishery activities and “Hidden” in deep sediment. Environ. Sci. Technol. 54, 2210–2217. doi: 10.1021/acs.est.9b04850
Keywords: polypropylene, polyester, particle, fiber, marine environment, uptake, impact, damselfish
Citation: Santana MFM, Dawson AL, Motti CA, van Herwerden L, Lefevre C and Kroon FJ (2021) Ingestion and Depuration of Microplastics by a Planktivorous Coral Reef Fish, Pomacentrus amboinensis. Front. Environ. Sci. 9:641135. doi: 10.3389/fenvs.2021.641135
Received: 13 December 2020; Accepted: 01 March 2021;
Published: 01 April 2021.
Edited by:
Cristina Panti, University of Siena, ItalyReviewed by:
Rachel Louise Coppock, Plymouth Marine Laboratory, United KingdomJoao Pinto Da Costa, University of Aveiro, Portugal
Copyright © 2021 Santana, Dawson, Motti, van Herwerden, Lefevre and Kroon. This is an open-access article distributed under the terms of the Creative Commons Attribution License (CC BY). The use, distribution or reproduction in other forums is permitted, provided the original author(s) and the copyright owner(s) are credited and that the original publication in this journal is cited, in accordance with accepted academic practice. No use, distribution or reproduction is permitted which does not comply with these terms.
*Correspondence: Marina F. M. Santana, bWFyaW5hLnNhbnRhbmFAbXkuamN1LmVkdS5hdQ==