- 1Laboratory of Environmental Genomics, Limnological Institute, University of Konstanz, Konstanz, Germany
- 2Laboratory of Conservation Genetics, Wildlife Ecology and Management, University of Freiburg, Freiburg im Breisgau, Germany
Effective management of both endangered native and invasive alien crayfishes requires knowledge about distribution, monitoring of existing and early detection of newly established populations. Complementary to traditional survey methods, eDNA sampling has recently emerged as a highly sensitive non-invasive detection method to monitor crayfish populations. To advance the use of eDNA as detection tool for crayfish we used a twofold approach: 1) we designed a novel set of specific eDNA-assays for all native (Austropotamobius torrentium, Austropotamobius pallipes, Astacus astacus) and the most relevant invasive crayfish species (Pacifastacus leniusculus, Faxonius limosus, Faxonius immunis) in Central Europe. To ensure specificity each primer pair was tested in silico, in vitro, and in situ; 2) we assessed the influence of spatio-temporal variables (distance to upstream population, season, stream size) on eDNA detection in seven streams using two different detection methods (qualitative endpoint PCR and quantitative droplet digital PCR, ddPCR). The newly developed eDNA assays successfully detected all crayfish species across different lotic and lentic habitats. eDNA detection rate (endpoint PCR) and eDNA-concentration (ddPCR) were significantly influenced by distance and season. eDNA detection was successful up to 7 km downstream of the source population and across all seasons, although detectability was lowest in winter. eDNA detection rate further decreased with increasing stream size. Finally, eDNA-concentration correlated positively with estimated upstream population size. Overall, we provide near operational eDNA assays for six crayfish species, enabling year-round detection, which represents a clear benefit over conventional methods. Due to its high sensitivity, eDNA detection is also suitable for the targeted search of as-yet unrecorded or newly emerging populations. Using quantitative ddPCR might further allow for a rough estimation of population size, provided that the identified spatio-temporal factors are accounted for. We therefore recommend implementing eDNA-detection as a complementary survey tool, particularly for a large-scale screening of data-deficient catchments or a year-round monitoring.
Introduction
Freshwater crayfish (Crustacea, Decapoda, Astacidae) are among the most threatened animal taxa in Central European fresh waters (Souty-Grosset et al., 2006; Chucholl and Schrimpf, 2016). All of the three indigenous crayfish species (ICS; Astacus astacus, Austropotamobius pallipes, Austropotamobius torrentium) are listed under the Habitats Directive and their conservation status is “unfavourable-inadequate” to “unfavourable-bad” in all biogeographical regions of the European Union with deteriorating population trends (Habitats Directive 92/43/EEC). The alarming population declines of native crayfish are driven by several threats, of which the most imminent is the ongoing spread of invasive non-indigenous crayfish species (NICS) of North American origin (Holdich et al., 2009). These act as a reservoir host for the causative agent of crayfish plague (Aphanomyces astaci), a lethal disease for native crayfish (Almeida et al., 2014; Chucholl and Schrimpf, 2016). In addition to displacement by NICS and crayfish plague, ICS suffer from pollution and degradation of habitats, and the increasing frequency of summer droughts due to climate change (Chucholl and Schrimpf, 2016).
Effective management of both ICS and NICS requires knowledge about distribution, early detection of newly emerging and monitoring of existing populations (Chucholl and Dehus, 2011; Kouba et al., 2014; Agersnap et al., 2017). However, conventional detection methods, such as manual search and trapping, are often hampered by the nocturnal behavior and elusive nature of crayfish (Peay, 2004). Specifically, crayfish populations are very hard to detect at low population densities, which are typical for early invasion stages of NICS and deteriorating populations of ICS (Cowart et al., 2018; Rice et al., 2018). What is more, conventional methods are mostly successful during summer when animals are active, and are normally associated with a disturbance of the habitat (Peay, 2004; Olarte et al., 2019).
In recent years, environmental DNA (eDNA) has emerged as new monitoring tool to survey aquatic environments (Ficetola et al., 2008; Goldberg et al., 2015; Yates et al., 2019), including crayfish (Dougherty et al., 2016; Mauvisseau et al., 2017; Rusch et al., 2020). The advantages of eDNA as monitoring tool are the high sensitivity of the method, the possibility to screen large sections of running waters with only a few sampling sites and the non-invasive nature of the sampling (Larson et al., 2017; Geerts et al., 2018). Detection by means of eDNA therefore shows considerable promise for both the early detection of new or spreading biological invasions (Jerde et al., 2011; Cowart et al., 2018), as well as the monitoring of rare or endangered species that also often occur at low abundances (Atkinson et al., 2019; Ikeda et al., 2019). Moreover, the simultaneous detection of both ICS and NICS is possible (Robinson et al., 2018). Studies on eDNA detection of crayfish show general applicability of this novel method and confirm its high sensitivity (Agersnap et al., 2017; Mauvisseau et al., 2017; Rusch et al., 2020). For instance, in small headwater streams in Japan, crayfish detection by means of eDNA was more sensitive than conventional methods, i.e. eDNA was found in sampling sites where a manual capture failed to detect crayfish (Ikeda et al., 2016).
However, it is still largely unknown how environmental factors and population properties affect detectability of crayfish populations. For instance, eDNA transport distances in lotic systems, that are often taxon-specific (Deiner and Altermatt, 2014; Wacker et al., 2019), are unknown for freshwater crayfish, although persistence of eDNA was assessed in laboratory and mesocosm experiments (Dunn et al., 2017; Harper et al., 2018). Moreover, unlike in other taxa (Buxton et al., 2018; Wacker et al., 2019; Curtis et al., 2020), seasonal variation of crayfish eDNA detectability has never been consistently assessed; existing studies either sampled only during one season or included preliminary field experiments (Ikeda et al., 2016; Harper et al., 2018; Rusch et al., 2020). Finally, water volume, e.g. during flood conditions, has been found to influence eDNA detectability (Curtis et al., 2020). This might imply an influence of stream size on crayfish eDNA detectability, which, however, has not been investigated yet. Overall, this lack of information is unfortunate as the routine application of eDNA detection, for instance in applied conservation projects, generally requires knowledge about these relationships.
To advance eDNA as detection tool for Central European crayfish we used a twofold approach: 1) we designed a novel set of specific endpoint eDNA-assays for all native (Austropotamobius torrentium, Austropotamobius pallipes, Astacus astacus) and the most relevant invasive crayfish species (Pacifastacus leniusculus, Faxonius limosus, Faxonius immunis) using a consistent multiple step approach, including an in silico, in vitro and in situ evaluation; 2) we assessed the influence of spatio-temporal variables (distance to upstream population, season, stream size) on eDNA detection. For this, we took water samples from 40 sampling sites in seven streams using two different detection methods (qualitative endpoint PCR and quantitative droplet digital PCR, ddPCR). All samples were subjected to endpoint PCR, whereas only a subset of samples (30 sampling sites in four streams) was analyzed with ddPCR. Finally, ddPCR was used to assess the relationship between estimated population size and eDNA concentration in the water.
We expected that eDNA detection probability is highest within or directly downstream of the population (Rice et al., 2018) and during the main activity time from spring to autumn (Harper et al., 2018). For small streams we expected a higher eDNA detection probability than for large rivers because the eDNA signal is probably diluted in a larger volume of water. Furthermore, we hypothesized a correlation between eDNA concentration in the water and estimated upstream population size, as the eDNA signal probably integrates with the populated stretch through downstream flow (Rice et al., 2018).
Materials and Methods
Primer Design and Evaluation
For each crayfish species a specific eDNA-assay with species-specific primers was developed. Primers were initially designed by visually screening alignments using the software Geneious Prime 2020.0.5 and evaluated for specificity using the program ecoPCR (Ficetola et al., 2010). Species-specific primers, amplifying fragments of 58–295 bp, were designed using alignments of the standard animal barcoding marker Cytochrome c Oxidase subunit I (COI). Alignments were built using published sequences of the respective target species and sequences of a range of non-target species. Accession numbers of the sequences used are listed in Supplementary Table S1.
For Astacus astacus, Faxonius limosus and Faxonius immunis all European sequences were used as target sequences. For Austropotamobius pallipes only sequences from the West European haplotype were included in the alignment, as A. pallipes represents a species complex with several proposed (sub)species, of which only the western form occurs in Central and Northern Europe (Grandjean et al., 2000; Gouin et al., 2006). For Austropotamobius torrentium, only haplotypes from the North of the Alps were included as target sequences, as haplotypes from the South of the Alpes belong to genetically different lineages (Trontelj et al., 2005) For non-native Pacifastacus leniusculus only sequences that belong to the subspecies P. l. leniusculus were included, because the other subspecies have never been imported from North America into Europe (Larson et al., 2012). As outgroups, sequences of all native and non-native crayfish species occurring in Europe, aquatic species that are likely to occur in the same habitats (e.g., Cottus gobio, Salmo trutta, and Gammarus fossarum) and species that represent common contaminants (e.g., humans and chicken) were included in the alignment.
The alignment was visually inspected for suitable primer pairs, which were subsequently tested in an in silico PCR for their specificity against the public NCBI database, covering approximately 160,000 taxa, using the program ecoPCR (Ficetola et al., 2010). The specified conditions for the in silico amplification allowed for a maximum of three mismatches for each primer, but demanded a perfect match on the last two nucleotides of the 3’ end of each primer. The minimum and maximum amplified sequence lengths (excluding primers) were 5 and 1,000 bp, respectively. Output of the ecoPCR was subsequently screened to ensure that amplified non-target species do not occur in European freshwater habitats (i.e. they were marine species, terrestrial species or species with a distribution range outside Europe).
After the in silico evaluation, the most suitable primer pairs were tested in vitro for their efficiency and specificity against tissue and environmental samples (see below) in an endpoint PCR. Each primer pair was tested with DNA extractions from tissue samples of both the target species and of non-target crayfish species, using DNA of the following: A. torrentium, A. pallipes, A. astacus, P. leniusculus, F. limosus, F. immunis, Procambarus virginalis. Finally, the best performing primer pair for each crayfish species was selected (Table 1).
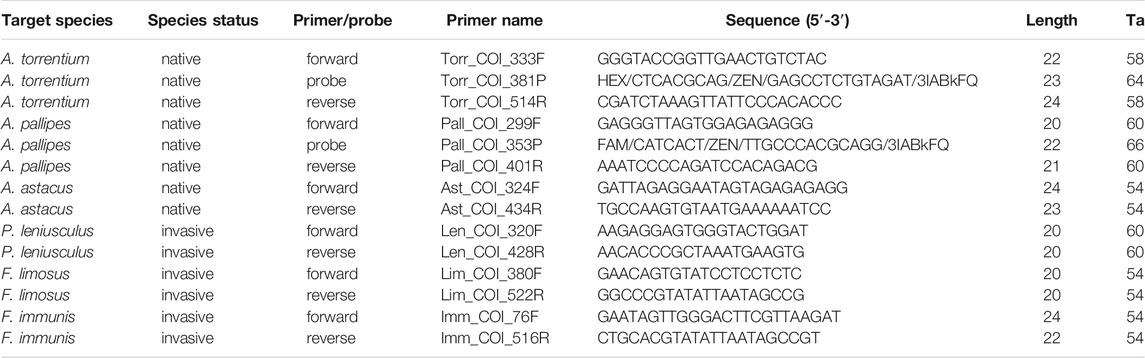
TABLE 1. Specific primer pairs for all crayfish species investigated in this study and ddPCR probes for A. torrentium and A. pallipes (species name and status, primer/probe name, primer sequence, length in basepairs, annealing temperature (Ta) used in the PCR reaction).
To ensure that the selected primers correctly amplified the target amplicon, also in DNA extractions of environmental samples, PCR-products of at least one environmental sample for each primer were purified with ExoSAP-ITTM PCR Product Cleanup (ThermoFisher Scientific) and DNA sequences were obtained through Sanger-Sequencing (performed by Microsynth Seqlab, Göttingen).
Study Populations
To test the efficiency and specificity of the novel set of eDNA-assays in situ, a total of 13 water bodies with known populations of the six target species were sampled (Figure 1). Detailed information on water body characteristics and eDNA sampling is summarized in Table 2. To prevent accidental transmission of the crayfish plague agent between water bodies, the used sampling equipment was cleaned and thoroughly dried for a minimum of 7 days upon each sampling occasion (OIE, 2019). In streams with several sampling locations, sites were visited in direction of stream flow to avoid upstream transmission of A. astaci spores. Furthermore, in streams with both manual capture and eDNA sampling (see Population Abundance and Size), the eDNA sampling was always done before the manual capture, to prevent contamination of eDNA sampling equipment with crayfish DNA.
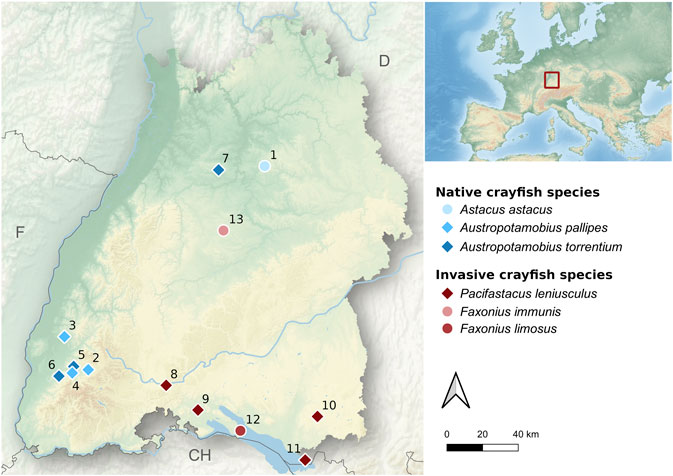
FIGURE 1. Map of the study area (federal state of Baden-Württemberg, southwestern Germany) showing all investigated water bodies. Native and invasive crayfish populations are presented by blue- and red-colored symbols, respectively. Sampling sites with diamonds were used for spatio-temporal eDNA sampling, whereas sampling sites with circles were only used for primer evaluation. SeeTable 2 for habitat characteristics.
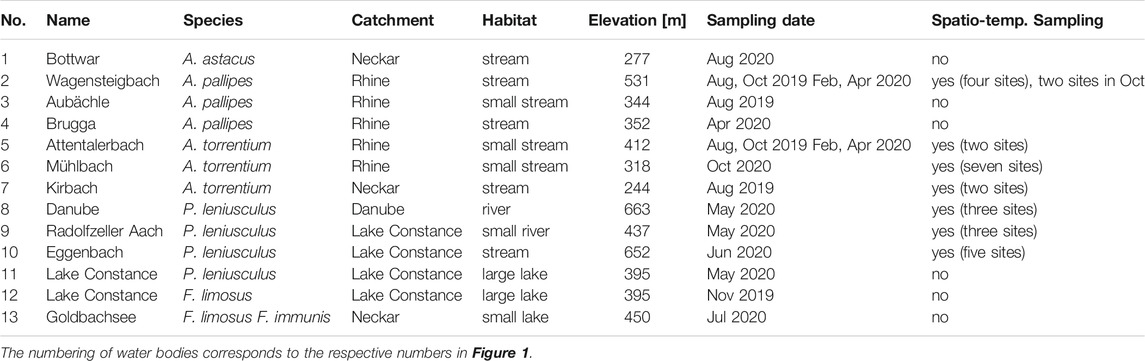
TABLE 2. Overview of the investigated water bodies with information on the target species, name and catchment of water body, habitat characteristics, sampling date and sampling method.
eDNA Sample Collection and Extraction
At each sampling site and occasion, four replicates of water samples were taken using sterile standup Whirl-Pak® sampling bags (V = 2041 ml, Nasco). Upon sampling, water samples were immediately put on ice in an opaque box and transported to the laboratory. Per sample 2 L of water were filtered, but in some cases, where the filter was clogged, the filtered volume was reduced to 1.5 L. All water samples were filtered on the same day using a membrane vacuum pump (VWR vacuum pump/compressor VCP 130) connected to a filter flask attached to a sterile analytical filter funnel with a Cellulose-Nitrate filter inside (analytical filter funnels, CN, Nalgene®, pore size 0.45 µm). For each sample, a new sterile filter funnel was used. Filters were removed from the funnel with sterile forceps and the filter was loosely rolled and put into a sterile 8 ml tube (Sarstedt). Subsequently, samples were frozen at −20°C until DNA-extraction. Extractions of eDNA samples were performed in a clean laboratory that had never been exposed to crayfish DNA. All extractions were carried out under an eDNA-extraction hood in a pre-PCR lab that is physically separated from the PCR and post-PCR lab to avoid back-contamination from PCR products. Before and after every DNA-extraction the bench of the extraction hood was cleaned with DNA-exitus, wiped with ddH2O and sterilized with UV-light for 15 min. Extractions were carried out using the DNeasy Power Water kit from Qiagen, following the protocol of the manufacturer. For every 18 samples, one extraction blank was included.
Spatio-Temporal Sampling Design
To test for spatio-temporal effects (distance to upstream population, season, and stream size) on eDNA detection a total of 40 sampling sites in seven streams with known populations of either A. torrentium (N = 3), A. pallipes (N = 1) or P. leniusculus (N = 3) were sampled (Table 2). Contemporary population extent was either known from monitoring surveys within the last 6 months or was surveyed during this study in May and August 2020 using manual capture following Chucholl and Schrimpf (2016). To account for spatial effects on eDNA detection, each of these streams was sampled with two to seven sampling sites. The most upstream sampling site was always located within the core population and the remaining sampling sites were distributed downstream of the population in an equally spaced distance from each other. The distance to the upstream crayfish population was measured as in-stream distance between the lower distribution limit of the population and the respective sampling site and ranged between 800 and 7,000 m. The number of sampling sites downstream of the core population was determined by the distance between the lower distribution limit and the confluence of the stream with the main water course. To make sure that eDNA-concentration in the flow was not significantly diluted by other waters, only streams without major tributaries were selected. To investigate seasonal effects on eDNA detection, a year-round sampling in spring, summer, autumn and winter was done in two streams (c.f. Table 2). To assess the effect of stream size on eDNA detection all investigated streams were categorized into four size classes, according to their width (0–5 m = small stream, >5–10 m = stream, >10–20 m = small river, >20 m = river).
PCR Procedures
Endpoint PCR
Qualitative endpoint PCR was performed for all tissue and environmental samples using a Flex Cycler (Analytik Jena). All PCR assays were prepared in a prePCR lab either on a bench (tissue samples) or under a UV hood reserved for PCR setup of eDNA extractions (environmental water samples) that was cleaned as outlined above for the UV hood used for DNA extraction.
For runs that contained only tissue samples, each 21.5 µl reaction contained 10 µl myTaq mix (MyTaqTM Mix, Bioline), 8 µl DEPC treated H2O, 0.5 µl BSA (4 mg/ml), 1 µl of each primer (10 µM) and 1 µl template DNA. For amplification the following cycling protocol was used: 95°C for 1 min (initial denaturation), 35 cycles of 95°C for 20 s (denaturation), 55 °C for 30 s (annealing), 72°C for 20 s (elongation), and a final elongation step at 72°C for 2 min.
For environmental water samples, all reactions were run in triplicates including a positive control (tissue), an extraction blank and a negative template control (NTC) for every run of N = 22 samples. Prior to use, the PCR buffer, MgSO4 and BSA solution were subjected to 5 min of UV irradiation at short distance from the light bulbs in a UVP crosslinker CL-1000 (see recommendations by Champlot et al. 2010). Each subsequent 25 µl reaction contained 15.05 µl water, 2.5 µl buffer (10x), 0.25 µl dNTPs (25 mM), 1 µl BSA (4 mg/ml), 1 µl MgSO4 (50 mM), 1 µl of each Primer (10 µM), 0.2 µl polymerase (PlatinumTMTaq DNA-Polymerase High Fidelity, InvitrogenTM, ThermoFisher Scientific, 5 U/ul) and 3 µl template DNA. For amplification the following cycling protocol was used: Initial denaturation at 94°C for 4 min, 55 cycles of denaturation at 94°C for 30 s, primer-specific annealing at 52°C (F. limosus), 56°C (F. immunis, A. astacus), 58°C (A. torrentium), 60°C (A. pallipes, P. leniusculus) for 30 s, elongation at 72°C for 20 s, and a final elongation step at 72°C for 2 min. After amplification PCR products were visually checked for bands of the correct amplicon size using a 2% agarose gel electrophoresis stained with GelRed (Biotium, Hayward, CA). If only one technical PCR replicate was positive, at least one PCR product from this site was purified with ExoSAP-ITTM PCR Product Cleanup (ThermoFisher Scientific) and subjected to Sanger-Sequencing (performed by Microsynth Seqlab, Göttingen) to exclude the possibility of false positives. Finally, the detection rate was calculated as proportion of positive PCR replicates per sampling site by dividing the number of positive PCR replicates per site by the total number of PCR replicates per site. The lower and a upper 95% confidence interval (CI) of the detection rate was calculated using the R package epiR (R Core Team, 2019; Nunes et al., 2020).
Droplet Digital PCR
In addition to the endpoint PCR, environmental samples from the A. pallipes and A. torrentium streams (cf. Table 2) were subjected to a quantitative droplet digital PCR (ddPCR) using a BioRad QX200 system. To quantify target DNA of A. pallipes and A. torrentium a double-quenched TaqMan probe was developed according to the assay design guidelines for ddPCR (Bio-Rad). The A. pallipes probe had a length of 22 base pairs: Pallipes_COI_353P (/56-FAM/CAT CAG CTA/ZEN/TTG CCC ACG CAG G/3IABkFQ/) and the A. torrentium probe had a length of 23 base pairs: Torrentium_COI_381P (/56-HEX/CTC ACG CAG/ZEN/GAG CCT CTG TAG AT/3IABkFQ/). In the ddPCR every sample is partitioned in 20,000 droplets and target and non-target DNA is distributed randomly. In every single droplet a PCR reaction takes place. The target DNA is marked by the fluorescence probe and the number of positive droplets is measured by a droplet reader. All ddPCR reactions were run in triplicates with a positive control (tissue), an extraction blank and an NTC for each 96 well PCR plate. Each 22 µl reaction contained 11 µl of ddPCR Supermix for Probes (Bio Rad), 1 µl of the probe (5.5 µM), 1 µl of each primer (19.8 µM), 6 µl of DEPC treated H2O and 3 µl of template DNA. Of each 22 µl reaction 20 µl were transferred to a BioRad QX200 droplet generator, which partitioned each reaction mixture into nanodroplets by combining 20 µl of the reaction mixture with 70 µl of BioRad droplet oil for Probes. After processing, this resulted in a total nanodroplet volume of 40 μl, which was transferred to a 96 well PCR plate for amplification on Bio Rad C1000 Touch™ thermal cycler using the following cycling protocol: hold at 95°C for 10 min, 40 cycles of 94°C for 30 s (denaturation), 58°C (A. torrentium) or 60°C (A. pallipes) for 30 s (annealing), 60°C for 30 s (elongation), and a final enzyme deactivation step at 98°C for 10 min. The plate was then analyzed on a QX200 droplet reader. The DNA copies in each reaction were calculated (absolute quantification) using the manufactures software (QuantaSoftTM Analysis Pro 1.0.596), which separates negative from positive droplets. A ddPCR replicate was scored as positive, when two or more droplets were positive. The QuantaSoftTM software automatically calculates the number of copies per µl of the final PCR Mix, which was subsequently converted to copies per µl of the starting sample using the following formula: number of copies per μl × 25 μl (the initial volume of the PCR Mix used for each reaction)/3 µl (the volume of template DNA used for each reaction). As for the endpoint PCR, a water sample was considered as positive, when one or more of the three technical ddPCR replicates were positive. Finally, the mean number of DNA copies per µl was calculated for each water sample by averaging the three technical ddPCR replicates.
Population Abundance and Size
To assess whether eDNA-concentration obtained by ddPCR was related to upstream crayfish population size, the size of six A. pallipes and A. torrentium populations was estimated from point abundance data and the population extent upstream of the sampling site. Abundance (indiv./m2) of crayfish was estimated with the “removal capture”-method (two-pass depletion approach) in August 2020 (Gouin et al., 2011). For this, a defined stretch of the stream was searched twice for crayfish by carefully inspecting potential shelters (e.g. stones, submerged roots) with a standardized effort. After the first pass, captured animals were temporarily removed from the stream until the second pass was undertaken. From the number of crayfish caught in the first and the second pass and the sampled area an estimate for the abundance (±confidence interval, CI) was calculated using the R package FSA (Ogle et al., 2020). Upstream population extent was assessed as in-stream distance between the respective eDNA sampling site and the upper distribution limit of the population. The distribution limit was taken from recent crayfish surveys in the course of the Habitats Directive (survey years: 2017–2019, fish and crayfish database of the federal state of Baden-Württemberg, FiAKa). Upstream population size was then calculated from the point abundance estimate and the upstream population extent, with confidence intervals propagated from the CI of the removal capture estimate. All crayfish samplings were done in agreement with the federal fisheries and nature conservation laws.
Statistical Analyses
All statistical analyses were performed using the software R 4.0.3 (R Core Team, 2019). Prior to statistical analyses, all variables were checked for normality and nonparametric tests were used when appropriate. Multiple linear mixed-effect models (LMM) were used to assess the effects of the spatio-temporal candidate predictors (distance to upstream population, season, and stream size) on eDNA detection rate (endpoint PCR) and eDNA-concentration (ddPCR), respectively. For the endpoint PCR, the proportion of positive PCRs per sampling site (detection rate) and for the ddPCR, the mean eDNA concentration per sampling site was used as dependent response variable. The stream ID was included as a random factor, as samplings sites were not independent from each other. Distance to upstream population (Dist. [m]), season (factor with four levels) and stream size (ordered factor with four levels) were included as independent explanatory variables. To identify the optimal model, four candidate models containing different combinations of the predictor variables were compared based on the Akaike information criterion, corrected for small sample size (AICc). The AICc is a model selection tool that measures model fit based on likelihood, with a penalty for model complexity (Chambers and Hastie, 1992; Zuur et al., 2009). Then the model with the highest Akaike weight (wAICc) and the highest relative likelihood (rel. LL) was selected and its performance was tested against a null model that only included a constant predictor as a fixed effect. For all model comparisons, individual models were fitted with maximum likelihood (ML) (Zuur et al., 2009). To examine the optimal model in detail, it was fitted with restricted maximum likelihood (REML) and predictor effects were assessed by analysis of deviance (Type II Test) (Zuur et al., 2009). As a measure of goodness of fit of the optimal model, marginal R2 were calculated following Nakagawa et al., 2017. To test for a correlation between estimated population size and eDNA concentration a Pearson’s product-moment correlation was undertaken. Then a linear model (LM) was used to assess the effect of estimated population size on eDNA concentration.
Results
Primer Evaluation
In silico evaluation of the primers for the six target crayfish species showed high specificity (no amplification of non-target species at zero nucleotide mismatches for all primers). When allowing for 1–3 mismatches amplification of N = 0 (A. torrentium primers), N = 7 (A. pallipes primers), N = 6 (A. astacus primers), N = 19 (F. limosus primers), N = 4 (F. immunis primers), and N = 285 (P. leniusculus primers) non-target species occurred, none of which are known to inhabit European freshwaters. In vitro validation with tissue samples confirmed that all six primer pairs amplified their target species successfully, with no cross-amplification of tissue from other crayfish species (A. torrentium, A. pallipes, A. astacus, P. leniusculus, F. limosus, F. immunis, P. virginalis). In environmental water samples, taken in situ, detection of target-DNA was also successful for all tested crayfish species, but detectability varied across species and habitats (Table 3). None of the negative controls (extraction blank or NTC) was positive. Moreover, sanger sequencing of a subset of PCR products from water samples confirmed the correct amplicon for each species.
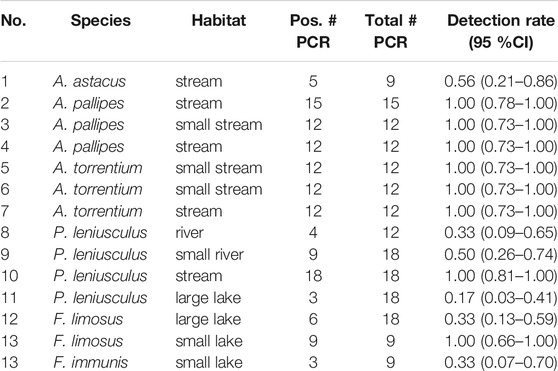
TABLE 3. Results of species-specific eDNA detection from sampling sites used for primer evaluation (see Figure 1 and Table 2 for location and characteristics of water bodies). Detection rate is given as proportion of positive endpoint PCRs (including 95% confidence interval).
Spatio-Temporal Effects on eDNA Detectability
Multiple mixed regression analysis revealed a significant effect of distance, season and stream size on eDNA detectability (Figure 2). A ranking of the best performing candidate models is shown in Table 4. LMM 1 and LMM A were considered optimal models based on the applied model selection criteria (δAICc, ωAICc, and rel. LL). Both models were highly significant and performed better than the respective null models with a constant predictor as a fixed effect (chi-square test: χ2= 61.14, p < 0.001 and χ2 = 38.17, p < 0.001 for LMM 1 and LMM A, respectively). Goodness of fit of the optimal models, as assessed by marginal R2, ranged between 0.69 (LMM A) and 0.72 (LMM 1).
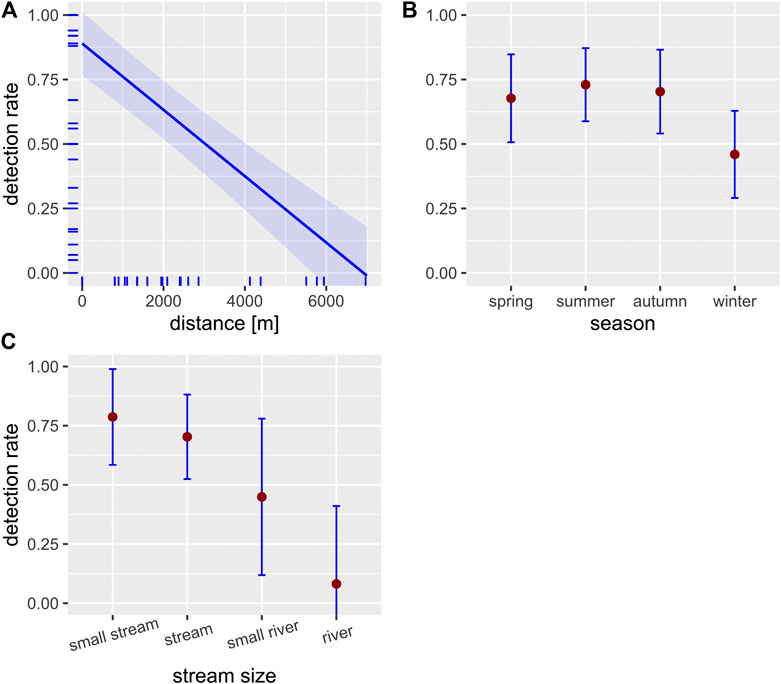
FIGURE 2. Effect plots of the LMM used to predict the effect of the three independent variables distance (A), season (B) and stream size (C) on the detection rate (proportion of positive PCRs; blue-shaded areas and error bars represent the 95% confidence intervals; N = 40, seeTable 5 for model statistics). Small ticks on the axes indicate the marginal distribution of the data.
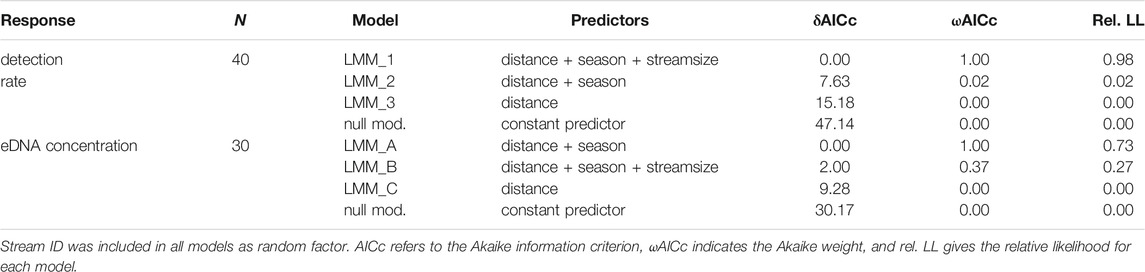
TABLE 4. Ranking of the three best performing candidate models to explain the detection rate (proportion of positive PCRs) and eDNA concentration in relation to the respective null model (with a constant predictor as fixed effect).
The fixed effects of the spatio-temporal predictors on detection rate and eDNA concentration, as contained in the optimal model, are summarized in Table 5. Distance had a strong negative effect on detection rate and eDNA concentration (Figures 2,3, respectively). Season showed a clear effect on both response variables, with a lower detection rate and eDNA concentration in winter compared to the other seasons (Figures 2,3, respectively). Stream size was negatively associated with detection rate (Figure 2). The detection rate was independent of the PCR method (endpoint PCR vs. ddPCR) (Wilcoxon Signed Rank Test, N = 30, p > 0.3).
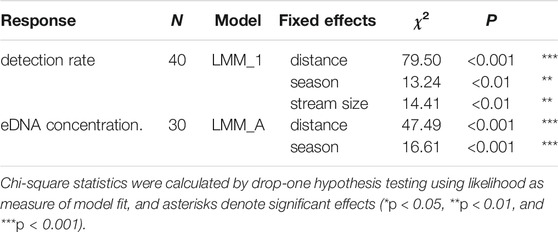
TABLE 5. Fixed effects of spatio-temporal variables on the detection rate (proportion of positive PCRs) and eDNA concentration as assessed by mixed-model analysis with stream ID as a random factor (seeTable 4 for model statistics).
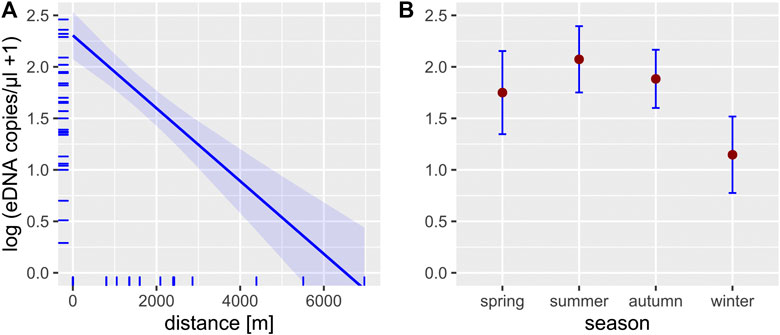
FIGURE 3. Effect plots of the LMM used to predict the effect of the two independent variables distance (A) and season (B) on the eDNA concentration (blue-shaded area and error bars represent the 95% confidence intervals; N = 30, seeTable 5 for model statistics). Small ticks on the axes indicate the marginal distribution of the data.
Relationship Between eDNA Concentration and Estimated Population Size
There was a significant correlation between estimated upstream population size and eDNA concentration in the water from sampling sites within populations (Pearson’s product-moment correlation, N = 6, R = 0.93, p < 0.01). Linear regression analysis revealed a positive effect of estimated population size on eDNA concentration (Figure 4, adj. R2 = 0.83, p < 0.01).
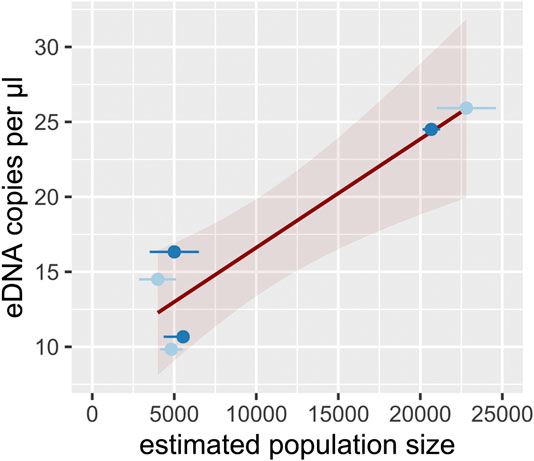
FIGURE 4. eDNA concentration within populations (median per sampling site) in relation to estimated upstream population size. Trend line is given by linear regression (R2 = 0.83, p < 0.01, red-shaded area represents the 95% confidence interval). Light-blue and dark-blue dots represent populations of A. pallipes and A. torrentium, respectively. Horizontal error bars indicate the lower and upper 95% CI for the population estimate.
Discussion
Primer Evaluation and eDNA Detection
We designed a novel set of specific assays for all native (A. torrentium, A. pallipes, A. astacus) and the most relevant invasive crayfish species (P. leniusculus, F. limosus, F. immunis) in Europe. To ensure specificity and sensitivity of our assays we used a consistent multiple step approach, consisting of in silico, in vitro and in situ evaluation as well as Sanger sequencing to confirm correct amplification of each amplicon.
Our approach is largely consistent to the recently proposed validation scale for targeted eDNA assays (Thalinger et al., 2020). According to this scale there are five levels ranging from “incomplete” to “operational.” Within this scale our assays can be classified from level 4 “substantial” to level 5 “operational.” Except for establishing a limit of detection (LOD), which is not possible when using endpoint PCR, our assays met all criteria propagated by the validation scale (Thalinger et al., 2020).
Our study shows successful eDNA detection of all investigated native and invasive crayfishes in a variety of habitats. Detection efficiency was 100%, i.e. crayfish were successfully detected at all sites where they have been known to occur (c.f. Table 3). This is in line with results of previous studies that investigated the suitability of eDNA as monitoring tool for freshwater crayfish (Tréguier et al., 2014; Dougherty et al., 2016; Ikeda et al., 2016; Agersnap et al., 2017; Mauvisseau et al., 2017; Ikeda et al., 2019; Troth et al., 2019; Rusch et al., 2020). However, detection efficiency varied among studies. For example, Treguier et al. (2014) detected the invasive crayfish Procambarus clarkii in 59% of ponds where it was trapped. Mauvisseau et al. (2017) investigated ponds in the same region but with a different assay and detected P. clarkii in 70% and F. limosus in 66% of the ponds, where presence was confirmed. Dougherty et al. (2016) investigated eDNA detection of Faxonius rusticus at low abundances in inland lakes of North America and showed a 100% accordance with conventional methods. A recent study of Rusch et al. (2020) investigated eDNA detection of native (A. astacus) and invasive (P. leniusculus, F. limosus and P. virginalis) crayfishes in a wide range of habitats in Central Europe and found crayfish in 95% of the habitats, where presence was confirmed.
Detection rate, i.e. the number of positive PCRs, in our study ranged between 17 and 100% for the respective sampling sites (Table 3). Ikeda et al. (2016), by comparison, showed a lower eDNA detection rate (range 12–50%) for their sampling sites in small headwater streams. This difference might be explained by the lower filter volume (0.25 L vs 2 L) used by Ikeda et al. (2016), the differences in extraction methods (DNeasy Blood & Tissue kit vs. DNeasy Power Water Kit) and the polymerase used (Taq Man Environmental Master Mix 2.0 vs. PlatinumTMTaq DNA-Polymerase High Fidelity).
Spatio-Temporal Effects on eDNA Detectability
Our study is among the first to coherently assess eDNA detectability of crayfish using a spatial and temporal sampling design. Previous field studies on eDNA detection of crayfish have primarily focused on either determining presence/absence (Tréguier et al., 2014; Harper et al., 2018; Mauvisseau, 2019) or on correlations between abundance/biomass and eDNA concentrations (Dougherty et al., 2016; Larson et al., 2017; Rice et al., 2018).
Our results show successful eDNA detection of crayfish species up to 7 km downstream of the source population (Figures 2, 3), whereby detection rate was independent of the PCR method. As expected, both detection rate and eDNA concentration were highest within or directly downstream of the source population and decreased with increasing in-stream distance. This represents a known effect, as eDNA in lotic waters is transported downstream through advection, until settlement and decay processes lead to a complete vanishing of eDNA from the water column (Sansom and Sassoubre, 2017). Over which distances detectable eDNA persists, is variable and depends on hydraulic properties of the water body (e.g., flow rate) as well as species-specific eDNA shedding rates (Nukazawa et al., 2018) and upstream population size (this study, Rice et al., 2018). Observed eDNA distances in previous studies vary therefore between less than 1 km in mesocosm and field experiments (Pilliod et al., 2014; Jane et al., 2015; Wilcox et al., 2016) to more than 100 km for a large river, where eDNA of a lake-dwelling fish species was detected (Pont et al., 2018). For freshwater crayfish, specific eDNA transport distance have not been assessed until now but are presumably more comparable with distances reported for other benthic invertebrates rather than fish and amphibians. Indeed, eDNA detection distances observed for Unio tumidus, a lake-dwelling benthic freshwater mussel, were with a maximum distance of 9 km relatively similar to detection distances in our study (Deiner and Altermatt, 2014). Generally, it seems that each species or taxon has its own spatial eDNA footprint, depending on individual eDNA shedding rates that are determined by the biology of the investigated species (Deiner and Altermatt, 2014; Wacker et al., 2019). Compared to fish and amphibians, freshwater crayfish are expected to have low eDNA shedding rates, because crayfish lack mucous producing structures present in fish and amphibians. Additionally, crayfish exhibit a hard exoskeleton that limits the release of extracellular eDNA into the water (Tréguier et al., 2014; Cai et al., 2017).
Detection of crayfish was successful year-round. However, LMM analysis indicated that there is a difference in eDNA detectability between winter and summer samples both with regard to detection rate and eDNA concentration (Figures 2, 3). This result is in line with previous studies, showing that eDNA detectability can be affected by season, depending on the biology of the target species (e.g., reproduction period, activity) (Buxton et al., 2018; Curtis et al., 2020). For crayfish, reduced detection rates in winter were an anticipated effect, since all European crayfish species show reduced activity and metabolism during winter (Bubb et al., 2002), which likely results in reduced eDNA shedding rates. Nonetheless, detection rate in winter never dropped below a threshold of 50% (range 50–100%), even at the most downstream sites, suggesting a reliable eDNA detection. Previous studies that analyzed winter samples for eDNA detection of crayfish showed contrasting results (Ikeda et al., 2016; Harper et al., 2018; Rusch et al., 2020). A Scottish study from Harper et al. (2018) completely failed to detect eDNA of P. leniusculus in three streams in winter. Another study from Japan was able to detect Cambaroides japonicus at one site with a low detection rate (12.5%), but failed at two other sites, where it was presumed to occur (Ikeda et al., 2016). A recent study from Rusch et al. (2020) investigated two lakes and two streams in winter, three of them with syntopic occurrences of F. limosus and P. virginalis and one of them with an abundant F. limosus population. eDNA detection was successful in all habitats, where species presence was currently confirmed, but failed in one habitat, where historic presence was documented. Differences in detectability among studies might be in part related to varying filter volumes and/or differences in extraction methods. For example, Harper et al. (2018) used a filter volume of 15 ml combined with precipitation as extraction method, whereas Rusch et al. (2020) filtered a total volume of 10 L and used the CTAB method for extraction (Strand et al., 2019).
Finally, stream size also had a negative effect on eDNA detection rate (Figure 2). In addition, the lakes examined in this study also tended to show a lower detection rate with increasing water body size (cf.Table 3). This was an expected outcome as the eDNA signal is probably diluted in a larger volume of water. Rusch et al. (2020) made a similar observation in a large river in Hungary, where the detection of an abundant F. limosus population failed. In contrast to that, Pont et al. (2018) were able to successfully detect a fish species in a large river habitat. This difference is probably due to the fact that crayfish are benthic littoral species that usually only colonize the area near the shore due to the increased flow rate in the middle of streams (Bohl, 1999). Population size of crayfish is therefore not linearly related to water body size and the eDNA signal is presumably disproportionally more diluted in large water bodies. This is a marked difference to pelagic taxa, whose population size can be expected to be more closely related to water body size, suggesting similar eDNA concentrations across different sized habitats.
Relationship Between eDNA Concentration and Estimated Population Size
eDNA concentration correlated positively with estimated upstream population size (Figure 4). This result met our expectations as eDNA concentrations in lotic environments probably depend on both abundance and length of the populated stretch. According to Rice et al. (2018) it is very likely that the eDNA signal integrates with the populated stretch through downstream transport of water and accumulates with increasing stream distance. For that reason, it might be difficult to infer abundance from eDNA concentration in lotic environments, in particular when population extent is unknown and not accounted for. In line with this reasoning, Rice et al. (2018) found that the likelihood to detect Faxonius eupunctus in a large lotic system was independent from local crayfish abundance but increased with the upstream length of the populated stretch. In lentic situations, previous studies mostly reported a relationship between eDNA copy number and relative abundance of crayfish, estimated by trapping (Dougherty et al., 2016; Larson et al., 2017) or visual counts (Cai et al., 2017), although a recent study found no correlation between trapping data and eDNA concentration (Johnsen et al., 2020). In our study, manual capture was used as reference method, which is known to have a very high capture probability in streams of the study region (seeChucholl and Schrimpf, 2016), and, which is also known as the least biased conventional sampling method (Peay, 2004; Hilber et al., 2020). In general, rough quantification of crayfish abundance using eDNA copy numbers seems therefore possible (cf. Yates et al., 2019), provided that population extent and habitat type (lotic vs lentic) as well as sampling season are accounted for.
Conclusion
Our results highlight that eDNA is a suitable tool for year-round detection of native and invasive crayfish species in a wide variety of habitats. Opposed to most conventional methods, eDNA based monitoring allows detection of crayfish independent of their activity pattern, even in natural habitats with abundant or inaccessible shelters, where detection with conventional methods is difficult and labor intensive (Peay, 2004). Typical applications could include non-invasive monitoring of native populations, for instance following reintroductions or population bottlenecks, and control of functionality of invasive species barriers (Cowart et al., 2018). Moreover, eDNA detection allows to scan large sections of running waters with only few sampling sites, which can be used for the targeted search of as-yet unrecorded or newly emerging populations. This feature makes eDNA an excellent tool for initial large-scale surveys, whereby sampling effort should be higher in large water bodies and winter to maximise the detection rate and, thus, detection probability. Subsequent validation and fine-scale localization of populations should include conventional monitoring methods, though (c.f. Johnsen et al., 2020). Finally, eDNA might allow for a rough quantification of upstream population size. However, further research across different habitats and natural settings is needed to refine our ability to predict population size or abundance from eDNA surveys.
Data Availability Statement
The datasets presented in this study can be found in the Supplementary Material (Supplementary Tables S1-S3).
Author Contributions
FC designed the study with input from LE and GS, conducted the data analysis, visualized analysed data and wrote the manuscript. FC and FF collected samples and carried out laboratory work. LE and GS supervised the study and contributed to the article.
Funding
This study was funded by the scholarship program of the German Federal Environmental Foundation (DBU) to FC. Lab work was enabled through funding by the Young Scholar Fund of the University of Konstanz to LE.
Conflict of Interest
The authors declare that the research was conducted in the absence of any commercial or financial relationships that could be construed as a potential conflict of interest.
Acknowledgments
We thank L. Gutbrod (University of Constance) for laboratory assistance and C. Chucholl (Fisheries Research Station BW) for crayfish distribution data, field work assistance and helpful comments on the manuscript.
Supplementary Material
The Supplementary Material for this article can be found online at: https://www.frontiersin.org/articles/10.3389/fenvs.2021.639380/full#supplementary-material.
References
Agersnap, S., Larsen, W. B., Knudsen, S. W., Strand, D., Thomsen, P. F., Hesselsøe, M., et al. (2017). Monitoring of noble, signal and narrow-clawed crayfish using environmental DNA from freshwater samples. PLoS One 12, e0179261. doi:10.1371/journal.pone.0179261 |
Almeida, D., Ellis, A., England, J., and Copp, G. H. (2014). Time-series analysis of native and non-native crayfish dynamics in the Thames River Basin (south-eastern England): dynamics of native and non-native crayfish. Aquat. Conserv. Mar. Freshw. Ecosyst. 24, 192–202. doi:10.1002/aqc.2366
Atkinson, S., Carlsson, J. E. L., Ball, B., Kelly-Quinn, M., and Carlsson, J. (2019). Field application of an eDNA assay for the threatened white-clawed crayfish Austropotamobius pallipes. Freshw. Sci., 35, 503–509. doi:10.1086/704712
Bohl, E. (1999). Motion of individual noble crayfish Astacus astacus in different biological situations: in-situ studies using radio telemetry. Freshw. Crayfish 12, 677–687.
Bubb, D. H., Lucas, M. C., and Thom, T. J. (2002). “Winter movements and activity of signal crayfish Pacifastacus leniusculus in an upland river, determined by radio telemetry,” in Aquatic telemetry, Editors E. B. Thorstad, I. A. Fleming, and T. F. Næsje, (Dordrecht, Netherlands: Springer), 111–119. doi:10.1007/978-94-017-0771-8_13
Buxton, A. S., Groombridge, J. J., and Griffiths, R. A. (2018). Seasonal variation in environmental DNA detection in sediment and water samples. PLoS One 13, e0191737. doi:10.1371/journal.pone.0191737 |
Cai, W., Ma, Z., Yang, C., Wang, L., Wang, W., Zhao, G., et al. (2017). Using eDNA to detect the distribution and density of invasive crayfish in the Honghe-Hani rice terrace World Heritage site. PLoS One 12, e0177724. doi:10.1371/journal.pone.0177724 |
Chambers, J. M., and Hastie, T. J. (1992). Statistical models in S. Pacific Grove, CA: Wadsworth & Brooks. Cole advanced books & software.
Champlot, S., Berthelot, C., Pruvost, M., Bennett, E. A., Grange, T., and Geigl, E.-M. (2010). An efficient multistrategy DNA decontamination procedure of PCR reagents for hypersensitive PCR applications. PLoS One 5, e13042. doi:10.1371/journal.pone.0013042 |
Chucholl, C., and Dehus, P. (2011). Flusskrebse in Baden-Württemberg. Fischereiforschungsstelle Baden-Württemberg (LAZBW), Baden-Württemberg, Langenargen: Ministerium für Ländlichen Raum und Verbraucherschutz.
Chucholl, C., and Schrimpf, A. (2016). The decline of endangered stone crayfish (Austropotamobius torrentium) in southern Germany is related to the spread of invasive alien species and land-use change. Aquat. Conserv. Mar. Freshw. Ecosyst. 26, 44–56. doi:10.1002/aqc.2568
Cowart, D. A., Breedveld, K. G. H., Ellis, M. J., Hull, J. M., and Larson, E. R. (2018). Environmental DNA (eDNA) applications for the conservation of imperiled crayfish (Decapoda: Astacidea) through monitoring of invasive species barriers and relocated populations. J. Crustac. Biol. 38, 257–266. doi:10.1093/jcbiol/ruy007
Curtis, A. N., Tiemann, J. S., Douglass, S. A., Davis, M. A., and Larson, E. R. (2020). High stream flows dilute environmental DNA (eDNA) concentrations and reduce detectability. Divers. Distrib. 00, 1–14. doi:10.1111/ddi.13196
Deiner, K., and Altermatt, F. (2014). Transport distance of invertebrate environmental DNA in a natural river. PLoS One 9, e88786. doi:10.1371/journal.pone.0088786 |
Dougherty, M. M., Larson, E. R., Renshaw, M. A., Gantz, C. A., Egan, S. P., Erickson, D. M., et al. (2016). Environmental DNA (eDNA) detects the invasive rusty crayfish Orconectes rusticus at low abundances. J. Appl. Ecol., 53, 722–732. doi:10.1111/1365-2664.12621 |
Dunn, N., Priestley, V., Herraiz, A., Arnold, R., and Savolainen, V. (2017). Behavior and season affect crayfish detection and density inference using environmental DNA. Ecol. Evol. 7, 7777–7785. doi:10.1002/ece3.3316 |
Ficetola, G., Coissac, E., Zundel, S., Riaz, T., Shehzad, W., Bessière, J., et al. (2010). An in silico approach for the evaluation of DNA barcodes. BMC Genomics 11, 434. doi:10.1186/1471-2164-11-434 |
Ficetola, G. F., Miaud, C., Pompanon, F., and Taberlet, P. (2008). Species detection using environmental DNA from water samples. Biol. Lett. 4, 423–425. doi:10.1098/rsbl.2008.0118 |
Geerts, A. N., Boets, P., Van den Heede, S., Goethals, P., and Van der heyden, C. (2018). A search for standardized protocols to detect alien invasive crayfish based on environmental DNA (eDNA): a lab and field evaluation. Ecol. Indic. 84, 564–572. doi:10.1016/j.ecolind.2017.08.068
Goldberg, C. S., Strickler, K. M., and Pilliod, D. S. (2015). Moving environmental DNA methods from concept to practice for monitoring aquatic macroorganisms. Biol. Conserv. 183, 1–3. doi:10.1016/j.biocon.2014.11.040
Gouin, N., Grandjean, F., and Souty-Grosset, C. (2006). Population genetic structure of the endangered crayfish Austropotamobius pallipes in France based on microsatellite variation: biogeographical inferences and conservation implications. Freshw. Biol. 51, 1369–1387. doi:10.1111/j.1365-2427.2006.01570.x
Gouin, N., Souty-Grosset, C., Bórquez, J., Bertin, A., and Grandjean, F. (2011). Disentangling the impact of demographic factors on population differentiation of an endangered freshwater crayfish (Austropotamobius pallipes) using population density and microsatellite data: genetic drift in A. pallipes populations. Freshw. Biol. 56, 2105–2118. doi:10.1111/j.1365-2427.2011.02629.x
Grandjean, F., Harris, D. J., Souty-Grosset, C., and Crandall, K. A. (2000). Systematics of the european endangered crayfish species Austropotamobius pallipes (Decapoda: Astacidae). J. Crustac. Biol. 20, 522–529. doi:10.1651/0278-0372(2000)020[0522:SOTEEC]2.0.CO;2
Harper, K., Anucha, P., Turnbull, J., Bean, C., and Leaver, M. (2018). Searching for a signal: environmental DNA (eDNA) for the detection of invasive signal crayfish, Pacifastacus leniusculus (Dana, 1852). Manag. Biol. Invasions 9, 137–148. doi:10.3391/mbi.2018.9.2.07
Hilber, T., Oehm, J., Effenberger, M., and Maier, G. (2020). Evaluating the efficiency of three methods for monitoring of native crayfish in Germany. Limno. 85, 125821. doi:10.1016/j.limno.2020.125821
Holdich, D. M., Reynolds, J. D., Souty-Grosset, C., and Sibley, P. J. (2009). A review of the ever increasing threat to European crayfish from non-indigenous crayfish species. Knowl. Manag. Aquat. Ecosyst. 11, 394–395. doi:10.1051/kmae/2009025
Ikeda, K., Doi, H., Tanaka, K., Kawai, T., and Negishi, J. N. (2016). Using environmental DNA to detect an endangered crayfish Cambaroides japonicus in streams. Conserv. Genet. Resour. 8, 231–234. doi:10.1007/s12686-016-0541-z
Ikeda, K., Doi, H., Terui, S., Kato, A., Mitsuzuka, T., Kawai, T., et al. (2019). Estimating native and invasive crayfish distributions in relation to culvert barriers with environmental DNA. Freshw. Sci., doi:10.1086/704998
Jane, S. F., Wilcox, T. M., McKelvey, K. S., Young, M. K., Schwartz, M. K., Lowe, W. H., et al. (2015). Distance, flow and PCR inhibition: eDNA dynamics in two headwater streams. Mol. Ecol. Resour. 15, 216–227. doi:10.1111/1755-0998.12285 |
Jerde, C. L., Mahon, A. R., Chadderton, W. L., and Lodge, D. M. (2011). “Sight-unseen” detection of rare aquatic species using environmental DNA: eDNA surveillance of rare aquatic species. Conserv. Lett. 4, 150–157. doi:10.1111/j.1755-263X.2010.00158.x
Johnsen, S. I., Strand, D. A., Rusch, J. C., and Vrålstad, T. (2020). Environmental DNA (eDNA) monitoring of noble crayfish Astacus astacus in lentic environments offers reliable presence-absence surveillance - but fails to predict population density. Front. Environ. Sci. 8, 612253. doi:10.3389/fenvs.2020.612253
Kouba, A., Petrusek, A., and Kozák, P. (2014). Continental-wide distribution of crayfish species in Europe: update and maps. Knowl. Manag. Aquat. Ecosyst. 413, 05. doi:10.1051/kmae/2014007
Larson, E. R., Abbott, C. L., Usio, N., Azuma, N., Wood, K. A., Herborg, L.-M., et al. (2012). The signal crayfish is not a single species: cryptic diversity and invasions in the Pacific Northwest range of Pacifastacus leniusculus: the signal crayfish is not a single species. Freshw. Biol. 57, 1823–1838. doi:10.1111/j.1365-2427.2012.02841.x
Larson, E. R., Renshaw, M. A., Gantz, C. A., Umek, J., Chandra, S., Lodge, D. M., et al. (2017). Environmental DNA (eDNA) detects the invasive crayfishes Orconectes rusticus and Pacifastacus leniusculus in large lakes of North America. Hydrobiologia 800, 173–185. doi:10.1007/s10750-017-3210-7
Mauvisseau, Q., Coignet, A., Delaunay, C., Pinet, F., Bouchon, D., and Souty-Grosset, C. (2017). Environmental DNA as an efficient tool for detecting invasive crayfishes in freshwater ponds. Hydrobiologia. 805, 163–175. doi:10.1007/s10750-017-3288-y
Mauvisseau, Q. (2019). Early detection of an emerging invasive species: eDNA monitoring of a parthenogenetic crayfish in freshwater systems. Manag. Biol. Invasions 10, 461–472. doi:10.3391/mbi.2019.10.3.04
Nakagawa, S., Johnson, P. C., and Schielzeth, H. (2017). The coefficient of determination R2 and intra-class correlation coefficient from generalized linear mixed-effects models revisited and expanded. J. R. Soc. Interf. 14, 20170213. doi:10.1098/rsif.2017.0213
Nukazawa, K., Hamasuna, Y., and Suzuki, Y. (2018). Simulating the advection and degradation of the environmental DNA of common carp along a river. Environ. Sci. Technol. 52, 10562–10570. doi:10.1021/acs.est.8b02293 |
Nunes, M. S. With contributions from T., Heuer, C., Marshall, J., Sanchez, J., Thornton, R., Reiczigel, J., et al. (2020). epiR: tools for the analysis of epidemiological data. Available at: https://CRAN.R-project.org/package=epiR.
Ogle, D. H., Wheeler, P., and Dinno, A. (2020). FSA: fisheries stock analysis. Available at: https://github.com/droglenc/FSA.
OIE (2019). Infection with Aphanomyces astaci (crayfish plague): manual of diagnostic tests for aquatic animals. Available at: https://www.oie.int/index.php?id=2439&L=0&htmfile=chapitre_aphanomyces_astaci.htm. (Accessed December 31, 2020).
Olarte, N., García-Arberas, L., and Antón, A. (2019). Evaluation of different kinds of disturbance on the efficiency of sampling methods employed for an endangered freshwater crayfish, Austropotamobius pallipes Lereboullet, 1858 (Decapoda: Astacidea: Astacidae), in the northern Iberian Peninsula. J. Crustac. Biol. 39, 342–348. doi:10.1093/jcbiol/ruz037
Peay, S. (2004). A cost-led evaluation of survey methods and monitoring for white-clawed crayfish- lessons from the UK. Bull. Fr. Pêche Piscic. 335–352. doi:10.1051/kmae:2004008
Pilliod, D. S., Goldberg, C. S., Arkle, R. S., and Waits, L. P. (2014). Factors influencing detection of eDNA from a stream-dwelling amphibian. Mol. Ecol. Resour. 14, 109–116. doi:10.1111/1755-0998.12159 |
Pont, D., Rocle, M., Valentini, A., Civade, R., Jean, P., Maire, A., et al. (2018). Environmental DNA reveals quantitative patterns of fish biodiversity in large rivers despite its downstream transportation. Sci. Rep. 8, 10361. doi:10.1038/s41598-018-28424-8 |
R Core Team (2019). R: a language and environment for statistical computing. Vienna, Austria: R Foundation for Statistical Computing. Available at: https://www.R-project.org/.
Rice, C. J., Larson, E. R., and Taylor, C. A. (2018). Environmental DNA detects a rare large river crayfish but with little relation to local abundance. Freshw. Biol. 63, 443–455. doi:10.1111/fwb.13081
Robinson, C. V., Uren Webster, T. M., Cable, J., James, J., and Consuegra, S. (2018). Simultaneous detection of invasive signal crayfish, endangered white-clawed crayfish and the crayfish plague pathogen using environmental DNA. Biol. Conservation 222, 241–252. doi:10.1016/j.biocon.2018.04.009
Rusch, J. C., Mojžišová, M., Strand, D. A., Svobodová, J., Vrålstad, T., and Petrusek, A. (2020). Simultaneous detection of native and invasive crayfish and Aphanomyces astaci from environmental DNA samples in a wide range of habitats in Central Europe. NeoBiota 58, 1–32. doi:10.3897/neobiota.58.49358
Sansom, B. J., and Sassoubre, L. M. (2017). Environmental DNA (eDNA) shedding and decay rates to model freshwater mussel eDNA transport in a river. Environ. Sci. Technol. 51, 14244–14253. doi:10.1021/acs.est.7b05199 |
Souty-Grosset, C., Holdich, D. M., and Noël, P. Y. (2006). Atlas of crayfish in Europe. Paris, France: Publ. Scientifiques du Muséum National d’Histoire Naturelle
Strand, D. A., Johnsen, S. I., Rusch, J. C., Agersnap, S., Larsen, W. B., Knudsen, S. W., et al. (2019). Monitoring a Norwegian freshwater crayfish tragedy: eDNA snapshots of invasion, infection and extinction. J. Appl. Ecol. 56, 1661–1673. doi:10.1111/1365-2664.13404
Thalinger, B., Deiner, K., Harper, L. R., Rees, H. C., Blackman, R. C., Sint, D., et al. (2020). A validation scale to determine the readiness of environmental DNA assays for routine species monitoring. bioRxiv preprint. doi:10.1101/2020.04.27.063990 (Accessed November 27, 2020)
Tréguier, A., Paillisson, J.-M., Dejean, T., Valentini, A., Schlaepfer, M. A., and Roussel, J.-M. (2014). Environmental DNA surveillance for invertebrate species: advantages and technical limitations to detect invasive crayfish Procambarus clarkii in freshwater ponds. J. Appl. Ecol. 51, 871–879. doi:10.1111/1365-2664.12262
Trontelj, P., Machino, Y., and Sket, B. (2005). Phylogenetic and phylogeographic relationships in the crayfish genus Austropotamobius inferred from mitochondrial COI gene sequences. Mol. Phylogenet. Evol. 34, 212–226. doi:10.1016/j.ympev.2004.09.010 |
Troth, C. R., Burian, A., Mauvisseau, Q., Bulling, M., Nightingale, J., Mauvisseau, C., et al. (2019). Development and application of eDNA-based tools for the conservation of white-clawed crayfish. Ecology doi:10.1101/732941
Wacker, S., Fossøy, F., Larsen, B. M., Brandsegg, H., Sivertsgård, R., and Karlsson, S. (2019). Downstream transport and seasonal variation in freshwater pearl mussel (Margaritifera margaritifera) eDNA concentration. Environ. DNA 1, 64–73. doi:10.1002/edn3.10
Wilcox, T. M., McKelvey, K. S., Young, M. K., Sepulveda, A. J., Shepard, B. B., Jane, S. F., et al. (2016). Understanding environmental DNA detection probabilities: a case study using a stream-dwelling char Salvelinus fontinalis. Biol. Conserv. 194, 209–216. doi:10.1016/j.biocon.2015.12.023
Yates, M. C., Fraser, D. J., and Derry, A. M. (2019). Meta‐analysis supports further refinement of eDNA for monitoring aquatic species‐specific abundance in nature. Environ. DNA 1, 5–13. doi:10.1002/edn3.7
Keywords: environmental DNA, species detection, crayfish, freshwater systems, monitoring
Citation: Chucholl F, Fiolka F, Segelbacher G and Epp LS (2021) eDNA Detection of Native and Invasive Crayfish Species Allows for Year-Round Monitoring and Large-Scale Screening of Lotic Systems. Front. Environ. Sci. 9:639380. doi: 10.3389/fenvs.2021.639380
Received: 08 December 2020; Accepted: 20 January 2021;
Published: 11 March 2021.
Edited by:
Ivana Maguire, University of Zagreb, CroatiaReviewed by:
Chloe Victoria Robinson, University of Guelph, CanadaDavid A. Strand, Norwegian Veterinary Institute, Norway
Copyright © 2021 Chucholl, Fiolka, Segelbacher and Epp. This is an open-access article distributed under the terms of the Creative Commons Attribution License (CC BY). The use, distribution or reproduction in other forums is permitted, provided the original author(s) and the copyright owner(s) are credited and that the original publication in this journal is cited, in accordance with accepted academic practice. No use, distribution or reproduction is permitted which does not comply with these terms.
*Correspondence: Franziska Chucholl, ZnJhbnppc2thLmNodWNob2xsQHVuaS1rb25zdGFuei5kZQ==; Laura Saskia Epp, bGF1cmEuc2Fza2lhLmVwcEBnbWFpbC5jb20=