- 1Institute of Soil and Water Resources and Environmental Science, College of Environmental and Resource Sciences, Zhejiang Provincial Key Laboratory of Agricultural Resources and Environment, Zhejiang University, Hangzhou, China
- 2Soil and Fertilizer Institute of Hunan Province, Hunan Academy of Agricultural Sciences, Changsha, China
- 3Institute of Soil and Environmental Sciences, University of Agriculture, Faisalabad, Pakistan
- 4School of Chemistry, Environmental and Life Sciences, University of The Bahamas, Nassau, The Bahamas
Mining activity is a growing environmental concern as it contributes to heavy metals (HMs) pollution in agricultural soils. Microbial communities play an important role in the biogeochemical cycling of HMs and have the potential to be used as bioindicators. Arsenic (As) and lead (Pb) are the most hazardous HMs and are mainly originated from mining activities. However, spatial variation in microbial community in response to As and Pb contamination in paddy soils remains overlooked. In this study, the biological and chemical properties of sixteen soil samples from four sites (N01, N02, N03, and N04) near a Pb-Zn mining site at different As and Pb levels were examined. The results showed that soil pH, total As and Pb, bioavailable As and Pb, nitrate-nitrogen (NO3−-N) and ammonia-nitrogen (NH4+-N) were the most important factors in shaping the bacterial community structure. In addition, significant correlations between various bacterial genera and As and Pb concentrations were observed, indicating their potential roles in As and Pb biogeochemical cycling. These findings provide insights into the variation of paddy soil bacterial community in soils co-contaminated with different levels of As and Pb.
Introduction
Mining mineral ore has both significant positive and negative impacts on mining areas. Mining resources have been endorsed as a mean to stimulate socioeconomic development and reduce poverty (Li et al., 2014). However, mining activities generate waste, such as mine tailings, acid mine drainages and fly ashes that contain harmful trace heavy metals (HMs) (Chen et al., 2018; Liu et al., 2019). HMs can be transported from mining sites to neighboring agricultural soils through surface runoff and atmospheric dust deposition (Mu et al., 2020). Consequently, most neighboring agricultural soils are contaminated with HMs, and the soil thereafter serves as a long-term sink for HMs (Hahn et al., 2019; Cheng et al., 2020).
In China, mining activities are prevalent and this has led to serious soil HMs contamination nationwide (Zhong et al., 2020). According to the Chinese National Soil Contamination Survey Report, approximately 33.4% of soil around mining sites were contaminated with HMs (Mee and Mnr, 2014). Among them, arsenic (As) and lead (Pd) commonly co-exist in high concentrations due to mining and smelting of mineral ores (Wang et al., 2020a; Fernandez-Macias et al., 2020), and threaten soil, plant and human health. In paddy soil, excess As and Pb can reduce soil quality, stunt plant growth, and ultimately affect human health through the food chain (Du et al., 2018; Rai et al., 2019). For example, Wang et al. (2018) reported that the concentrations of As and Pb (up to 2749 and 624 μg kg−1, respectively) in rice grains grown in fields neighboring mining sites were significantly higher than the threshold value of 200 μg kg−1 (GB2762–2017). Therefore, the investigation of the HMs pollution in paddy soils surrounding mining areas can assess the risk of HMs to human health and provide the scientific basis for the remediation of HMs contaminated soils.
HMs accumulation in soils affects both the soil physiochemical properties and the microbial community structure over short- and long-term periods (Zhu et al., 2019). Zhang et al. (2015) reported that HMs in paddy soil may have adverse effects on the microbial activities and nutrients bioavailability. Pb contaminations have been shown to inhibit soil respiration and reduce microbial biomass (Liu et al., 2017). Similarly, dynamic changes were observed in the soil microbial community as a result of As contamination (Wang et al., 2016). Furthermore, in the soil-rice system, As contamination can markedly influence the iron (Fe) plaque-associated microbial community structure and metabolic potential (Hu et al., 2019). Although many attempts were tried to remediate As and Pb pollution in soils neighboring mining sites, few have reported the combined effect of As and Pb contamination on the soil microbial community (Tian et al., 2020; She et al., 2021).
Molecular techniques such as high-throughput sequencing techniques have been used to evaluate the changes in microbial community due to HMs pollution (Ros et al., 2009; Gołębiewski et al., 2014). Changes in soil microbial community structure and diversity could serve as biomarkers to study the effect of HMs on soil health (Vinhal-Freitas et al., 2017; Li et al., 2020b; Di Cesare et al., 2020). However, it should be noted that some studies have reported that HMs contamination had limited effect on the microbial community structure (Lorenz et al., 2006). Other soil factors such as pH, organic matter (OM), moisture, clay content and nutrient content can have a profound influence on the microbial activities, which might play more important role in reshaping microbial communities than the HMs concentrations (Jiang et al., 2019). However, studies on the difference between microbial communities in paddy soils with different distances from the mining site and the responses of microorganisms to HMs and soil properties are limited. HMs polluted paddy soil with various distances from the mining site may present different soil characteristics and HMs concentration gradients, then the most important factor in shaping the microbial communities remains unknown.
For this purpose, this present study was conducted with soil samples from four sites along a Pb-Zn mining site to 1) explore the influence of the different distance from the mining site on the physiochemical properties of As and Pb polluted paddy soils; 2) evaluate the combined effects of As and Pb pollution on soil bacterial community structure; 3) examine the correlativity between soil physiochemical properties and bacterial communities. Chemical analysis coupled with Miseq sequencing techniques were applied to achieve the goals. The observations and findings in this study could be used to develop more effective bioremediation strategies for the polluted paddy soil in mining area.
Materials and Methods
Study Area and Sampling
The study area is located near a Pb-Zn mining site (29°59′35.00″ N, 120°45′55.20″ E) in Shangyu city, Zhejiang province, China (Figure 1). The study area has a typical subtropical climate, densely surrounded by paddy field. A total of 16 top-soil samples (0–20 cm) were collected from the four sites referred to as N01, N02, N03, and N04. The four sites are all paddy fields and one growth cycle is performed over one year. Soil samples (four in total, approximately 300 g each) were collected from random points at each site and were mixed uniformly to form a composite sample. Four replicate composite samples were collected from each site (denoted as samples N01–1, N01–2, N01–3, N01–4, and so on). Soil samples were properly sealed and transported to the laboratory on ice. The collected soil samples were divided into two parts; one part was stored at −80°C for molecular analyses, and the other part was used for the physicochemical analysis.
Chemical Analysis
Soil samples from N01, N02, N03, and N04 site were analyzed for different physicochemical parameters including soil pH, OM, moisture content, dissolved organic carbon (DOC), available nitrate-nitrogen (NO3−-N) and ammonia-nitrogen (NH4+-N) concentrations, total As, Pb and other HMs concentrations, total sulfur (S) and SO42-. Soil pH and OM were measured following methods described in our pervious study (Zou et al., 2018). Moisture content was measured by drying fresh soil samples overnight in an oven at 105°C (O’Kelly, 2004). DOC was extracted with ultrapure water from fresh soil at a ratio of 1:2 (soil: water) and the concentration was analyzed with an automated N/C analyzer (Multi N/C 2100, Analytik Jena, Germany) (Xu et al., 2015). Available NH4+-N and NO3−-N concentrations were determined in 1 M KCl extracts (1:5, w/v) using the colorimetric methods (Stock, 1983). Soil SO42- was extracted by shaking freeze-dried soils with ultrapure water at a ratio 1:25 (soil:water) and analyzed by ion chromatography (Dionex, United States) (Xu et al., 2015). In order to determine the total Fe, S and other HMs, 0.2 g soil was digested using a concentrated acid mixture (5 ml of HNO3, 3 ml of HClO4, and 5 ml of HF) and was analyzed by inductively coupled plasma-optical emission spectrometry (ICP-OES, iCAP 6300, Thermo, United States). For total As analysis, 0.2 g air-dried soil was digested with 10 ml diluted aqua regia (aqua regia: water = 1:1, v/v) at 100°C for 2 h, and As concentration in digested samples were determined by atomic fluorescence spectrometry (AFS-9130, Beijing Jitian Instrument Company, China) (Zou et al., 2018). In order to determine the accuracy of the total metal analysis, a reference soil (GBW 07429, the National Research Center for standards in China) was used. The extract efficiencies ranged from 97.8 to 104.6% of the target values.
Bioavailable As and Pb Fraction in Soil
As and Pb were fractionated according to a 4-step modified European Community Bureau of Reference (BCR) sequential extraction procedure including F1: extracted with 0.11 M acetic acid (exchangeable fraction); F2: extracted with 0.5 M hydroxylammonium chloride (reducible fraction); F3: extracted with 8.8 M hydrogen peroxide followed by 1.0 M ammonium acetate (oxidizable fraction); F4: extracted with hydrofluoric acid followed by Aqua Regia (nitric acid + hydrochloric acid; 1:3 v/v) (residual fraction) (Rauret et al., 1999). The sum of exchangeable and reducible fractions was defined as bioavailable fractions according to a previous study (Roosa et al., 2014). The extracted solutions were analyzed by ICP-OES for different As and Pb fractions. The difference between the sum of each phase concentration and the total concentrations of As and Pb were compared to verify the accuracy of the sequential extraction procedure.
Microbial Biomass Carbon
Microbial biomass carbon (MBC) in soils was estimated by chloroform fumigation-extraction method (Vance et al., 1987; Brookes, 1995). Briefly, 6 subsamples of 5 g moist soil were weighed into different glass centrifuge tubes and three of them were fumigated with ethanol-free chloroform. Both fumigated and non-fumigated samples were incubated for 24 h at 25°C in the dark. After incubation, all the samples were extracted with 25 ml of 0.5 M K2SO4 and filtered. Then, the fumigated samples were heated in a 100°C water bath for 60 min and lost moisture was replenished. Total dissolved carbon in the extracts was determined by an automated N/C analyzer (Multi N/C 2100, Analytik Jena, Germany). MBC was calculated according to Eq. 1 (Jaenicke et al., 2011):
where Cf = total dissolved carbon extracted from fumigated soil, Cnf = total dissolved carbon extracted from nonfumigated soil and kEC = 0.45.
Soil DNA Extraction, PCR Amplification and Sequencing Analysis
Total DNA was extracted (four replicate samples) for each site using Fast DNA® Spin Kit for Soil (MP Biomedicals, Santa Ana, United States) according to the manufacturer’s protocol. Total genomic DNA was amplified using primers 926 F (5’ - AAA CTY AAA KGA ATT GAC GG - 3′) and 1392 R (5’ -ACG GGC GGTGTG TRC- 3′) that amplifies the V6-V8 region of the 16 S rRNA gene (Luo et al., 2016). The 16 S rRNA tag-encoded high-throughput sequencing was carried out on Illumina Miseq platform at the Tianke Technology Co., Ltd (Hangzhou, China). Sequencing reads were assigned to each sample according to the individual unique barcode and filtered to remove ambiguous reads. Operational taxonomic units (OTUs) were clustered with 97% similarity cutoff using UPARSE (V8.1.1861) (Wang et al., 2007). For each OTU, a representative sequence was selected and used to assign taxonomic composition by using the RDP classifier (V11.4) against the SILVA database (www.arb-silva.de). The 16S rRNA gene sequences reported in this paper have been deposited in the NCBI SRA under the accession No. PRJNA678872.
Statistical Analysis
Statistical analyses for the experimental data were performed using the SPSS® 19.0 (SPSS, United States) software. The significance of the treatment effects was assessed by analysis of variance (ANOVA), followed by comparisons between treatment means using the least significant difference (LSD) at p < 0.05. Alpha diversity index including Chao1, Shannon, Simpson, ACE and Coverage were calculated using QIIME software (V1.9.1) (Caporaso et al., 2010). The similarity among microbial communities in different soil samples was determined using UniFrac analysis by QIIME software. Principal coordinate analysis (PCoA) plots of the OTU-based weighed UniFrac distances between all samples and heatmap of Spearman’s rank correlations coefficients between geochemical parameters and the top 35 bacterial genera were generated by R software (V3.5.1) (http://www.r-project.org/). Redundancy analysis (RDA) was performed based on abundant bacteria genera (relative abundance top 35) and selected physicochemical parameters by Canoco 5 (Biometrics Wageningen, The Netherlands) (Aazami et al., 2015).
Results and Discussion
Physicochemical Characterization of Soil Samples
The long term and continuous use of As and Pb contaminated paddy soil poses a serious health risks, since rice have a high tendency to accumulate As and Pb in its grains (Biswas et al., 2020). Paddy soil contaminated by As and Pb in mining areas has been widely documented (Kashyap et al., 2019; Zheng et al., 2020). However, the impact of mining activities on paddy soil physicochemical and microbial properties at various distances away from the mine is not well understood. HMs contamination and physicochemical properties of paddy soil play an important role in microbial metabolism (Zhao et al., 2013). Physicochemical characteristics of collected soils from the four sites were presented in Table 1. For all four sites, the soil pH was close to neutral (6.56–7.33), and the maximum pH was observed in site N01. The NO3−-N and NH4+-N concentrations were significantly higher in site N01 as compared to the other studied sites (p < 0.05).
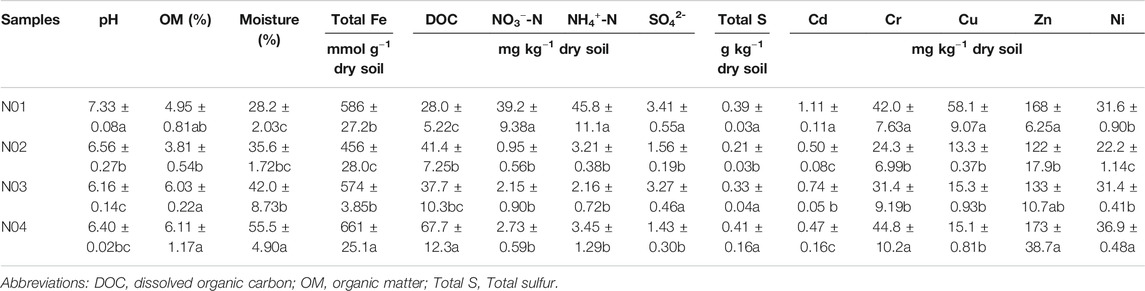
TABLE 1. Physicochemical characteristics of the paddy soil samples from each site. Different letters indicate significant differences among the different sites (p < 0.05).
As and Pb were found to be the dominant HMs in the selected sites (Table 1 and Table 2). The high As and Pb concentrations can be ascribed to intensive mining activities in the area. Previous studies have shown that As and Pb can easily be translocated from the mining site to neighboring farmlands via surface runoff and/or dust particles deposition (Wang et al., 2011; Guo et al., 2017; Li et al., 2020c). The total As and Pb concentrations in the studied area ranged between 44.4–388 mg kg−1 and 0.20–2.12 g kg−1, respectively. The results also showed that the As and Pb concentrations in all the sites exceeded the soil environmental quality risk control standard for soil contamination of agricultural land in China (GB15618–2018) (6.5 < pH ≤ 7.5, As 25 mg kg−1, Pb 120 mg kg−1) (Mee, 2018). For example, the As and Pb concentrations in N01 were 15 and 17 times of the soil environmental quality risk control standard (GB15618–2018), respectively. These results were consistent with the previous studies that suggested agricultural soils near mining sites were seriously polluted (Leung et al., 2017; Zhen et al., 2019).

TABLE 2. Total and bioavailability concentrations of As and Pb in soil samples collected from four sites. Different letters indicate significant differences among the different sites (p < 0.05).
A clear As and Pb gradient was observed at all four sites. The As and Pb concentrations decreased with increasing distance from the mining site and vice versa (Table 2). Previous studies have reported that the HMs concentrations in the topsoil usually decrease with the increase in the distance away from the mine (Li et al., 2015; Shen et al., 2017; Li et al., 2020c). A significant correlation was observed between total As and total Pb (r = 0.970, p < 0.001) (Supplementary Table S1), which indicated that these metal (loid)s had similar pollution sources (Sun et al., 2010). Furthermore, HMs bioavailable concentrations in soils have been reported to have better correlation with their accumulation in plants, which would directly threaten human health via food chain (Zhang et al., 2018). The bioavailable As concentration in N01 was significantly higher than other sites (p < 0.05). However, bioavailable As concentrations in N02, N03, and N04 were much lower compared to their total As concentrations. On the contrary, the bioavailable Pb concentrations were about half of the total concentrations for all four sites. Bioavailable As (r = 0.715, p < 0.01) and Pb (r = 0.667, p < 0.01) were positively corrected with soil pH, while bioavailable As (r = −0.656, p < 0.01) and Pb (r = −0.585, p < 0.05) were negatively corrected with soil OM (Supplementary Table S1). This indicates that pH and OM might be the main factors influencing As and Pb bioavailability in our study. pH may affect the bioavailability of As and Pb by influencing the adsorption of As and Pb on soil minerals. For example, the decreasing positive surface charge of soil minerals with increasing pH facilitated the desorption of As (Masscheleyn et al., 1991). With the increase of OM, the toxicity of HMs could be reduced through chelation and sequestration of metal cations onto OM (Macoustra et al., 2019).
Changes in Soil Microbial Biomass Carbon
Soil microbial biomass carbon (MBC) has been widely used as a bioindicator to evaluate soil quality since the soil microbial community is more sensitive to changes in soil composition compared to soil physiochemical properties (Feyzi et al., 2020). The MBC concentrations in N03 (1.39 mg g−1) and N04 (1.15 mg g−1) were significantly (p < 0.05) higher compared to those in N01 (0.52 mg g−1) and N02 (0.47 mg g−1) (Figure 2). The MBC concentrations increased with decreasing As and Pb concentrations. Moreover, significant negative correlations between MBC and As and Pb concentrations were observed (Supplementary Table S1). Our results are consistent with those of a previous study (Zhen et al., 2019) reporting that MBC was significantly reduced in soils collected near a mining site in Shaoguan city, China, particularly due to the presence of high HMs concentration. Other studies also noticed that MBC decreased significantly with the increasing metal concentrations (Khan et al., 2010; Xu et al., 2019). This indicated that due to the presence of excess metals in the contaminated soils, the MBC was suppressed and the microbial population was impeded to multiply (Xu et al., 2019). This may have occurred because soil MBC is sensitive to HMs, since microorganisms require additional energy to deal with stress caused by HMs (Yang et al., 2006). This additional energy requirement may result in a reduction in the amount of substrate used for microbial growth (Xiao et al., 2017).
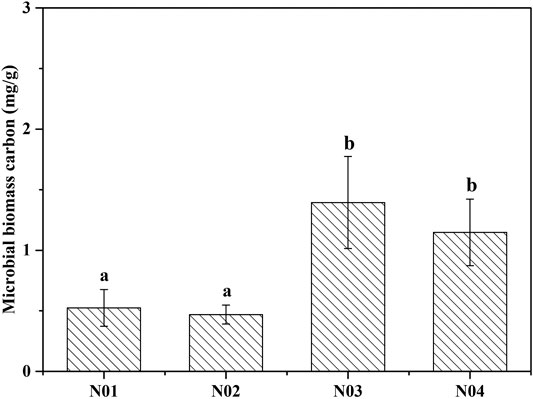
FIGURE 2. Microbial biomass carbon (MBC) collected from four different sites near a Pb-Zn mining site. Different letters indicate significant differences among the different sites (p < 0.05).
Changes in Microbial Community Structure
Soil microbial community structure and diversity have also been used as an index for soil HMs pollution due to their sensitivity to soil physiochemical changes (Song et al., 2018). A total of 882,322 valid reads were obtained from the sixteen paddy soil samples through Illumina MiSeq platform and the average sequence length was 463 bp. A total of 642,574 operational taxonomic units (OTUs) was revealed. The number of OTUs from N01 was significantly lower than those from N02, N03 and N04 (p < 0.05) (Supplementary Table S2). The alpha diversity indexes including ACE and Chao1 from N03 and N04 were significantly higher than the samples from N01 and N02 (p < 0.05) (Supplementary Table S2). Soil microbial diversity in moderately and heavily contaminated soil were observed to be reduced compared to those of healthy soil (Golebiewski et al., 2014). Similar to our study, Shen et al. (2019) also observed that As and Pb contamination could decrease microbial diversity. Furthermore, the PCoA [PC1 vs PC2 (explaining 86.74%)] analysis suggested an obvious separation of the bacterial communities in N01, N02 and N03 while the bacterial communities in N04 was close to N02 and N03 (Supplementary Figure S1).
The microbial community structures differed among the four sampled sites. The relative abundance of the sequences obtained from the different sites at the phylum level are shown in Figure 3. The top 10 dominant phyla in all samples were, Proteobacteria (28.9–39.8%), Acidobacteria (9.3–16.6%), Chloroflexi (6.2–18.7%), Nitrospirae (2.8–12.7%), Bacteroidetes (2.0–8.9%), Gemmatimonadetes (1.9–4.4%), Planctomycetes (2.5–4.0%), Verrucomicrobia (1.9–3.9%), Chlorobi (1.9–3.9%) and Actinobacteria (1.2–2.2%). These phyla have also been reported in previous studies as dominant bacterial groups in paddy soil (Li et al., 2017; Zhen et al., 2019; Li et al., 2020a). Pollution-related changes in the relative abundance of the phyla were observed. The relative abundances of Proteobacteria, Bacteroidetes, Planctomycetes, and Verrucomicrobia were significantly higher in N01 than those in N02, N03 and N04 (p < 0.05), while the relative abundances of Chloroflexi, Nitrospirae, and Chlorobi were significantly lower in N01 (p < 0.05). These results indicate that soil HMs pollution may suppress some microbial phyla while promote the growth of HMs resistant microbes (Xu et al., 2018). Thus, we can deduce from our results that the phyla Proteobacteria and Bacteroidetes are able to endure high As and Pb stresses. Previous studies have reported that the members of the phylum Proteobacteria are able to tolerate high HMs stress (Schneider et al., 2017; Li et al., 2020c), which was consistent with the results of our study. Moreover, other studies have shown that Proteobacteria dominate in the metal-tolerant cultures, because many species belonging to this phyla can reduce Fe and facilitate soil-adsorbed metals release which inadvertently increase metal stress (Francis and Dodge, 1990; Jiang et al., 2017). Proteobacteria and Bacteroidetes have also been reported to harbor the majority of HMs resistance genes base on metagenome sequencing (Yan et al., 2020). Furthermore, an increase in Bacteroidetes relative abundance have been observed in Pb spiked soils compared to the unspiked soils (Hou et al., 2020).
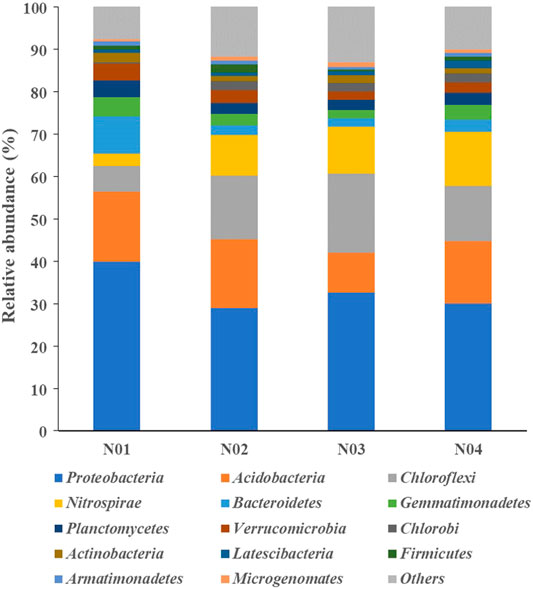
FIGURE 3. Relative abundances (>1%) of dominant microbial taxa across different samples at the phylum levels.
In order to further understand the microbial community response to geochemical conditions and HMs, the OTUs were further classified to the genus level (Figure 4). The four sampled sites were clustered into two distinct groups (one group included N01 and the other group included N02, N03, and N04) which was consistent with the results shown in the PCoA plot (Figure 4 and Supplementary Figure S1). Flavobacterium (2.0%), Haliangium (1.8%), Nitrospira (1.5%), Sphingomonas (1.4%) and Pseudomonas (1.3%) were the most abundant genera in N01, while Geobacter (1.4–1.9%), Thiobacillus (0.8–3.0%), and Bryobacter (0.6–1.5%) were the most abundant genera in N02, N03 and N04. The relative abundance of Haliangium, Nitrospira, Flavobacterium, Opitutus, Sphingomonas, Pseudomonas, Aquicella, Acidibacter, Blastocatella, and Sorangium were significantly higher in N01 than those in N02, N03, and N04 (p < 0.05). Previous studied have shown that the most of the bacterial strains from these genera are commonly involved in As resistance (Guo et al., 2017; Titah et al., 2018; Li et al., 2020c). Haliangium was observed to flourish in HMs stressed environments (Marti et al., 2017). Similarly, the abundances of Flavobacterium were observed to increase under high Pb stress (Wang et al., 2020b). Flavobacterium was also isolated from As contaminated soil as an As-resistant genus and it was able to reduce arsenate (As(V)) under erobic conditions (Wang and Mulligan, 2006). Besides, Flavobacterium related groups are able to methylate As as a detoxification strategy (Honschopp et al., 1996). Nitrospira are nitrite-oxidizing bacteria that can provide nitrate which takes part in the oxidation of arsenite (As(III)) (Yu et al., 2020). Studies have found that in HMs polluted soil, Sphingomonas was the most abundant genus and had a potential to remove Pb from seawater (Chen, 2012; Guo et al., 2017). Pseudomonas has been reported to be involved in As redox processes (Cavalca et al., 2013). Moreover, the As(III) efflux pump protein gene arsB and As transporter protein gene acr3P was also detected within the genus of Pseudomonas (Sanyal et al., 2016). Acidibacter and Blastocatella increased in HMs polluted soil and their significant positive correlation with Pb or As was also observed by Guo et al. (2017). The relative abundance of Geobacter, Thiobacillus, Sideroxydans, Anaerolinea, Anaeromyxobacter, Leptolinea, Spirochaeta_2, Desulfobacca, Syntrophorhabdus, and Ignavibacterium were significantly higher in N02, N03, N04 than those in N01 (p < 0.05). These results indicated that these microorganisms were relatively sensitive to As and Pb toxicity and the abundances were abruptly declined with the increase of HMs concentrations (Giller et al., 1998). Geobacter populations were reported as Fe and sulfate reducing bacteria, while Anaeromyxobacter were Fe reducing bacteria and Desulfobacca were sulfate reducing bacteria (Sun et al., 2015a). Besides, Thiobacillus were found to be involved in Fe and S oxidation and Thiobacillus can oxidize elemental sulfur (Wang et al., 2021). Sideroxydans were involved in Fe oxidation (Blothe and Roden, 2009). Most of these sensitive microorganisms were Fe- and S-related bacteria indicating their important roles in lightly contaminated sites. We speculated that As and Pb contamination in paddy soil might have an inhibition effect on Fe and S cycling in paddy soil.
Correlation Between Environmental Parameters and Microbial Community
In microbial ecology, understanding the relationship between environmental variables and microbial community structure is a key goal (Liang et al., 2011). Spearman’s rank correlations coefficients between geochemical parameters and bacterial abundances were determined (Figure 5). The relative abundance of Acidibacter positively correlated with total As, total Pb, bioavailable Pb (p < 0.05) and bioavailable As (p < 0.001) concentrations in soil. Similar results have also been reported by Guo et al. (2017). Nitrospira, Aquicella and Sorangium were also positively correlated with total As, bioavailable As, total Pb and bioavailable Pb concentrations. Other genera like Sphingomonas, Pseudomonas and Massilia also had positive correlations with total or bioavailable As or Pb. These results suggested that As and Pb contamination may facilitate the growth of these bacterial groups. Thioalkalispira (p < 0.001), Desulfobacca (p < 0.01) and Spirochaeta_2 (p < 0.01) were negatively correlated with total As, bioavailable As, total Pb and bioavailable Pb concentrations. Among them, Nitrospira, Aquicella and Sorangium were positively correlated with different forms of As and Pb, indicating their high HMs tolerance while Thioalkalispira, Desulfobacca and Spirochaeta_2 were sensitive to As and Pb pollution. pH, moisture and NH4+-N had significant correlations with most of the genera. Redundancy analysis (RDA) was used to reveal the possible linkages between the microbial communities and geochemical parameters (Figure 6). Although the pollution source of the soil was from a Pb-Zn mining site, no significant correlation between the microbial communities and the Zn concentration could be found. It might be due to the low Zn concentrations in soil. Besides, it has been reported that high levels of Zn had no effect on the species diversity compared to unpolluted soil in mining areas (Xu et al., 2017). Total As, bioavailable As, total Pb and bioavailable Pb concentrations along with pH, NH4+-N, NO3−-N, moisture, SO42- and MBC were the main factors influencing bacterial community compositions (Figure 6). Total As, bioavailable As, total Pb and bioavailable Pb concentrations along with pH, NH4+-N and NO3−-N positively correlated with microbial communities from N01 while negatively correlated with microbial communities from N02, N03, and N04. Soil moisture positively correlated with microbial communities from N02, N03, and N04. It is widely accepted that pH has a significant effect on the overall diversity and composition of microbial communities (Gu et al., 2017). Any significant deviation in pH could impose stress on single-celled organisms because the intracellular pH of most microorganisms are usually within 1 pH unit of neutral (Fierer and Jackson, 2006). Soil pH could also influence other environmental parameters (e.g. nutrient availability or cationic metal solubility), that plays an important role in microbial survival (Sun et al., 2015b). Previous studies also reported that pH had a greater impact on bacterial community composition in HMs contaminated agricultural soils when compared with HMs in soil (Cavani et al., 2016; Jiang et al., 2016). Two types of nitrogen (NH4+-N and NO3−-N) were significantly correlated with soil genera in this study, suggesting their important role in shaping the soil microbial community (Deng et al., 2018). Anderson et al. (2009) reported that NH4+-N and NO3−-N showed positive correlations with the shifts in microbial community structure, which was consistent with our results. Paddy soils could provide good habitats for nitrogen cycling, which in turn have the potential to shape the microbial communities (Sun et al., 2015a). Additionally, moisture is a major environmental factor that determines the redox conditions of soils and the metabolism of microbes (Honma et al., 2016). For example, wet-dry transition in soil would result in a microbial community shift of anaerobic to erobic microbes (Pett-Ridge and Firestone., 2005). This is especially true for paddy soil which are intermittently flooded during rice production.
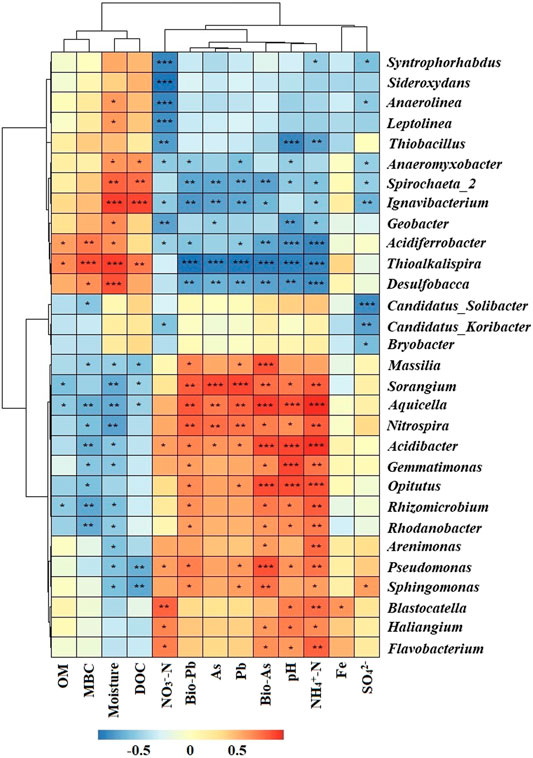
FIGURE 5. Heatmap of Spearman’s rank correlations coefficients between geochemical parameters and the relative abundance of top 35 bacterial genera. The corresponding value of heatmap is Spearman correlation coefficient where “r” value is between −1 and 1, r < 0 is negative correlation, r > 0 is positive correlation. The asterisk indicates significant differences. *p < 0.05, **p < 0.01, ***p < 0.001. Abbreviations: As, Pb, Fe (total As, Pb, Fe in soil); Bio-As, Pb (bioavailability As, Pb); MBC (microbial biomass carbon); OM (organic matter); DOC (dissolved organic carbon).
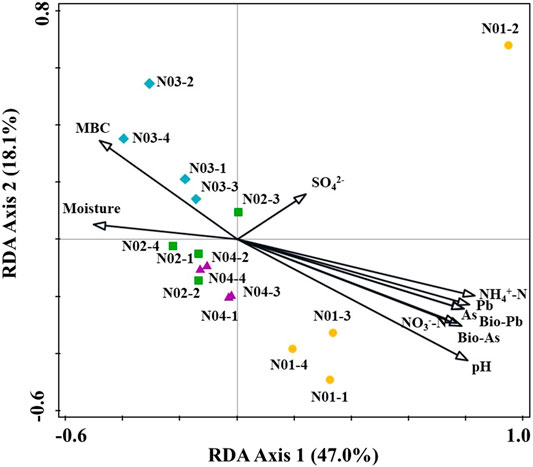
FIGURE 6. Redundancy analysis (RDA) diagram of the geochemical parameters and the top 35 bacterial genera. Abbreviations: As, Pb, Fe (total As, Pb, Fe in soil); Bio-As, Pb (bioavailability As, Pb); MBC (microbial biomass carbon).
Conclusion
In this study, we used the high throughput sequencing to examine the effects of physicochemical parameters on the microbial community variation in HMs contaminated soils along an As and Pb gradient. Our results showed that high amounts of HMs were presented near the mining site, and their concentrations decreased with increasing distance from the mining site. As and Pb contamination have a profound impact on the microbial community structure of paddy soils. Different forms of As and Pb (total and bioavailable) and soil physicochemical components (pH, NH4+-N, NO3−-N, moisture, and MBC) had significant influences on the bacterial community compositions. Some genera including Haliangium, Nitrospira, and Flavobacterium were positively correlated with different forms of As and Pb, indicating their high tolerance and their important role in As and Pb cycling while other genera including Geobacter, Thiobacillus, and Sideroxydans were sensitive to HMs contamination. Our finding provided important information that should be taken into consideration when developing bioremediation strategies for the polluted paddy soil in mining area.
Data Availability Statement
The datasets presented in this study can be found in online repositories. The names of the repository/repositories and accession number(s) can be found in the article/Supplementary Material.
Author Contributions
XT and JN performed the study conceptualization and supervision. LZ performed the literature search and wrote the original draft. YL, YD, MK, WG, and LY carried out the writing, reviewing and JS and JX performed the study supervision and validation editing. All authors read and approved the final manuscript.
Conflict of Interest
The authors declare that the research was conducted in the absence of any commercial or financial relationships that could be construed as a potential conflict of interest.
Acknowledgments
The National Key Technology R&D Program (2018YFD0800202) supported this study.
Supplementary Material
The Supplementary Material for this article can be found online at: https://www.frontiersin.org/articles/10.3389/fenvs.2021.630668/full#supplementary-material.
References
Aazami, J., Esmaili-Sari, A., AbdoliSohrabi, A. H., and Van den Brink, P. J. (2015). Monitoring and Assessment of Water Health Quality in the Tajan River, Iran Using Physicochemical, Fish and Macroinvertebrates Indices. J. Environ. Health Sci. Engineer. 13, 29. doi:10.1186/s40201-015-0186-y
Anderson, J. A. H., Hooper, M. J., Zak, J. C., and Cox, S. B. (2009). Characterization of the Structural and Functional Diversity of Indigenous Soil Microbial Communities in Smelter-Impacted and Nonimpacted Soils. Environ. Toxicol. Chem. 28, 534–541. doi:10.1897/08-281.1
Biswas, J. K., Warke, M., Datta, R., and Sarkar, D. (2020). Is Arsenic in Rice a Major Human Health Concern? Curr. Pollut. Rep. 6, 37–42. doi:10.1007/s40726-020-00148-2
Blothe, M., and Roden, E. E. (2009). Composition and Activity of an Autotrophic Fe(II)-oxidizing, Nitrate-Reducing Enrichment Culture. Appl. Environ. Microbiol. 75, 6937–6940. doi:10.1128/AEM.01742-09
Brookes, P. C. (1995). The Use of Microbial Parameters in Monitoring Soil Pollution by Heavy Metals. Biol. Fertil. Soils. 19, 269–279. doi:10.1007/bf00336094
Caporaso, J. G., Kuczynski, J., Stombaugh, J., Bittinger, K., Bushman, F. D., Costello, E. K., et al. (2010). QIIME Allows Analysis of High-Throughput Community Sequencing Data. Nat. Methods 7, 335–336. doi:10.1038/nmeth.f.303
Cavalca, L., Corsini, A., Zaccheo, P., Andreoni, V., and Muyzer, G. (2013). Microbial Transformations of Arsenic: Perspectives for Biological Removal of Arsenic from Water. Future Microbiol. 8, 753–768. doi:10.2217/fmb.13.38
Cavani, L., Manici, L. M., Caputo, F., Peruzzi, E., and Ciavatta, C. (2016). Ecological Restoration of a Copper Polluted Vineyard: Long-Term Impact of Farmland Abandonment on Soil Bio-Chemical Properties and Microbial Communities. J. Environ. Manage. 182, 37–47. doi:10.1016/j.jenvman.2016.07.050
Cesare, A. D., Pjevac, P., Eckert, E., Curkow, N., Šparica, M. M., Corno, G., et al. (2020). The Role of Metal Contamination in Shaping Microbial Communities in Heavily Polluted Marine Sediments. Environ. Pollut. 265, 114823. doi:10.1016/j.envpol.2020.114823
Chen, M., Lu, G., Wu, J., Yang, C., Niu, X., Tao, X., et al. (2018). Migration and Fate of Metallic Elements in a Waste Mud Impoundment and Affected River Downstream: A Case Study in Dabaoshan Mine, South China. Ecotoxicology Environ. Saf. 164, 474–483. doi:10.1016/j.ecoenv.2018.08.063
Cheng, W., Lei, S., Bian, Z., Zhao, Y., Li, Y., and Gan, Y. (2020). Geographic Distribution of Heavy Metals and Identification of Their Sources in Soils Near Large, Open-Pit Coal Mines Using Positive Matrix Factorization. J. Hazard. Mater. 387, 121666. doi:10.1016/j.jhazmat.2019.121666
Deng, S., Ke, T., Li, L., Cai, S., Zhou, Y., Liu, Y., et al. (2018). Impacts of Environmental Factors on the Whole Microbial Communities in the Rhizosphere of a Metal-Tolerant Plant: Elsholtzia Haichowensis Sun. Environ. Pollut. 237, 1088–1097. doi:10.1016/j.envpol.2017.11.037
Du, F., Yang, Z., Liu, P., and Wang, L. (2018). Accumulation, Translocation, and Assessment of Heavy Metals in the Soil-Rice Systems Near a Mine-Impacted Region. Environ. Sci. Pollut. Res. 25, 32221–32230. doi:10.1007/s11356-018-3184-7
Fernandez-Macias, J. C., Gonzalez-Mille, D. J., Garcia-Arreola, M. E., Cruz-Santiago, O., Rivero-Perez, N. E., Perez-Vazquez, F., et al. (2020). Integrated Probabilistic Risk Assessment in Sites Contaminated with Arsenic and Lead by Long-Term Mining Liabilities in San Luis Potosi, Mexico. Ecotox. Environ. Safe. 197, 8. doi:10.1016/j.ecoenv.2020.110568
Feyzi, H., Chorom, M., and Bagheri, G. (2020). Urease Activity and Microbial Biomass of Carbon in Hydrocarbon Contaminated Soils. A Case Study of Cheshmeh-Khosh Oil Field. Iran. Ecotox. Environ. Safe. 199, 11. doi:10.1016/j.ecoenv.2020.110664
Fierer, N., and Jackson, R. B. (2006). The Diversity and Biogeography of Soil Bacterial Communities. Proc. Natl. Acad. Sci. 103, 626–631. doi:10.1073/pnas.0507535103
Francis, A. J., and Dodge, C. J. (1990). Anaerobic Microbial Remobilization of Toxic Metals Coprecipitated with Iron Oxide. Environ. Sci. Technol. 24, 373–378. doi:10.1021/es00073a013
Giller, K. E., Witter, E., and Mcgrath, S. P. (1998). Toxicity of Heavy Metals to Microorganisms and Microbial Processes in Agricultural Soils: A Review. Soil Biol. Biochem. 30, 1389–1414. doi:10.1016/s0038-0717(97)00270-8
Gołębiewski, M., Deja-Sikora, E., Cichosz, M., Tretyn, A., and Wróbel, B. (2014). 16S rDNA Pyrosequencing Analysis of Bacterial Community in Heavy Metals Polluted Soils. Microb. Ecol. 67, 635–647. doi:10.1007/s00248-013-0344-7
Gu, Y., Van Nostrand, J. D., Wu, L., He, Z., Qin, Y., Zhao, F. J., et al. (2017). Bacterial Community and Arsenic Functional Genes Diversity in Arsenic Contaminated Soils from Different Geographic Locations. Plos. One. 12. e0189656. doi:10.1371/journal.pone.0189656
Guo, H., Nasir, M., Lv, J., Dai, Y., and Gao, J. (2017). Understanding the Variation of Microbial Community in Heavy Metals Contaminated Soil Using High Throughput Sequencing. Ecotoxicology Environ. Saf. 144, 300–306. doi:10.1016/j.ecoenv.2017.06.048
Hahn, J., Mann, B., Bange, U., and Kimmel, M. (2019). Horizon-specific Effects of Heavy Metal Mobility on Nitrogen Binding Forms in Forest Soils Near a Historic Smelter (Germany). Geoderma 355, 8. doi:10.1016/j.geoderma.2019.113895
Honma, T., Ohba, H., Kaneko-Kadokura, A., Makino, T., Nakamura, K., and Katou, H. (2016). Optimal Soil Eh, pH, and Water Management for Simultaneously Minimizing Arsenic and Cadmium Concentrations in Rice Grains. Environ. Sci. Technol. 50, 4178–4185. doi:10.1021/acs.est.5b05424
Honschopp, S., Brunken, N., Nehrkorn, A., and Breunig, H. J. (1996). Isolation and Characterization of a New Arsenic Methylating Bacterium from Soil. Microbiol. Res. 151, 37–41. doi:10.1016/s0944-5013(96)80053-x
Hou, X. L., Han, H., Tigabu, M., Li, Q. Y., Li, Z. X., Zhu, C. L., et al. (2020). Lead Contamination Alters Enzyme Activities and Microbial Composition in the Rhizosphere Soil of the Hyperaccumulator Pogonatherum Crinitum. Ecotoxicol Environ. Saf. 207, 111308. doi:10.1016/j.ecoenv.2020.111308
Hu, M., Sun, W., Krumins, V., and Li, F. (2019). Arsenic Contamination Influences Microbial Community Structure and Putative Arsenic Metabolism Gene Abundance in Iron Plaque on Paddy Rice Root. Sci. Total Environ. 649, 405–412. doi:10.1016/j.scitotenv.2018.08.388
Jaenicke, S., Ander, C., Bekel, T., Bisdorf, R., Dröge, M., Gartemann, K. H., et al. (2011). Comparative and Joint Analysis of Two Metagenomic Datasets from a Biogas Fermenter Obtained by 454-pyrosequencing. Plos. One. 6. e14519. doi:10.1371/journal.pone.0014519
Jiang, B., Adebayo, A., Jia, J., Xing, Y., Deng, S., Guo, L., et al. (2019). Impacts of Heavy Metals and Soil Properties at a Nigerian E-Waste Site on Soil Microbial Community. J. Hazard. Mater. 362, 187–195. doi:10.1016/j.jhazmat.2018.08.060
Jiang, J., Pan, C. H., Xiao, A. P., Yang, X. A., and Zhang, G. M. (2017). Isolation, Identification, and Environmental Adaptability of Heavy-Metal-Resistant Bacteria from Ramie Rhizosphere Soil Around Mine Refinery. 3 Biotech. 7, 6. doi:10.1007/s13205-017-0603-2
Jiang, L. F., Song, M. K., Yang, L., Zhang, D. Y., Sun, Y. T., Shen, Z. G., et al. (2016). Exploring the Influence of Environmental Factors on Bacterial Communities within the Rhizosphere of the Cu-Tolerant Plant. Elsholtzia Splendens. Sci. Rep. 6, 10. doi:10.1038/srep36302
Kashyap, R., Ahmad, M., Uniyal, S. K., and Verma, K. S. (2019). Dietary Consumption of Metal(loid)s-Contaminated Rice Grown in Croplands Around Industrial Sectors: a Human Health Risk Perspective. Int. J. Environ. Sci. Technol. 16, 8505–8516. doi:10.1007/s13762-019-02258-x
Khan, S., El-Latif Hesham, A., Qiao, M., Rehman, S., and He, J.-Z. (2010). Effects of Cd and Pb on Soil Microbial Community Structure and Activities. Environ. Sci. Pollut. Res. 17, 288–296. doi:10.1007/s11356-009-0134-4
Leung, H. M., Duzgoren-Aydin, N. S., Au, C. K., Krupanidhi, S., Fung, K. Y., Cheung, K. C., et al. (2017). Monitoring and Assessment of Heavy Metal Contamination in a Constructed Wetland in Shaoguan (Guangdong Province, China): Bioaccumulation of Pb, Zn, Cu and Cd in Aquatic and Terrestrial Components. Environ. Sci. Pollut. Res. 24, 9079–9088. doi:10.1007/s11356-016-6756-4
Li, P. F., Liu, M., Ma, X. Y., Wu, M., Jiang, C. Y., Liu, K., et al. (2020a). Responses of Microbial Communities to a Gradient of Pig Manure Amendment in Red Paddy Soils. Sci. Total. Environ. 705, 8. doi:10.1016/j.scitotenv.2019.135884
Li, P., Lin, C., Cheng, H., Duan, X., and Lei, K. (2015). Contamination and Health Risks of Soil Heavy Metals Around a Lead/zinc Smelter in Southwestern China. Ecotoxicology Environ. Saf. 113, 391–399. doi:10.1016/j.ecoenv.2014.12.025
Li, S., Wu, J., Huo, Y., Zhao, X., and Xue, L. (2020b). Profiling Multiple Heavy Metal Contamination and Bacterial Communities Surrounding an Iron Tailing Pond in Northwest China. Sci. Total. Environ. 752, 141827. doi:10.1016/j.scitotenv.2020.141827
Li, S., Zhao, B., Jin, M., Hu, L., Zhong, H., and He, Z. (2020c). A Comprehensive Survey on the Horizontal and Vertical Distribution of Heavy Metals and Microorganisms in Soils of a Pb/Zn Smelter. J. Hazard. Mater. 400. 123255. doi:10.1016/j.jhazmat.2020.123255
Li, X., Meng, D., Li, J., Yin, H., Liu, H., Liu, X., et al. (2017). Response of Soil Microbial Communities and Microbial Interactions to Long-Term Heavy Metal Contamination. Environ. Pollut. 231, 908–917. doi:10.1016/j.envpol.2017.08.057
Li, Z., Ma, Z., Van Der Kuijp, T. J., Yuan, Z., and Huang, L. (2014). A Review of Soil Heavy Metal Pollution from Mines in China: Pollution and Health Risk Assessment. Sci. Total Environ. 468-469, 843–853. doi:10.1016/j.scitotenv.2013.08.090
Liang, Y., Van Nostrand, J. D., Deng, Y., He, Z., Wu, L., Zhang, X., et al. (2011). Functional Gene Diversity of Soil Microbial Communities from Five Oil-Contaminated Fields in China. Isme. J. 5, 403–413. doi:10.1038/ismej.2010.142
Liu, G., Ling, S., Zhan, X., Lin, Z., Zhang, W., and Lin, K. (2017). Interaction Effects and Mechanism of Pb Pollution and Soil Microorganism in the Presence of Earthworm. Chemosphere 173, 227–234. doi:10.1016/j.chemosphere.2017.01.022
Liu, J., Yin, M., Zhang, W., Tsang, D. C. W., Wei, X., Zhou, Y., et al. (2019). Response of Microbial Communities and Interactions to Thallium in Contaminated Sediments Near a Pyrite Mining Area. Environ. Pollut. 248, 916–928. doi:10.1016/j.envpol.2019.02.089
Lorenz, N., Hintemann, T., Kramarewa, T., Katayama, A., Yasuta, T., Marschner, P., et al. (2006). Response of Microbial Activity and Microbial Community Composition in Soils to Long-Term Arsenic and Cadmium Exposure. Soil Biol. Biochem. 38, 1430–1437. doi:10.1016/j.soilbio.2005.10.020
Luo, F., Devine, C. E., and Edwards, E. A. (2016). Cultivating Microbial Dark Matter in Benzene-Degrading Methanogenic Consortia. Environ. Microbiol. 18, 2923–2936. doi:10.1111/1462-2920.13121
Macoustra, G., Holland, A., Stauber, J., and Jolley, D. F. (2019). Effect of Various Natural Dissolved Organic Carbon on Copper Lability and Toxicity to the Tropical Freshwater Microalga Chlorella Sp. Environ. Sci. Technol. 53, 2768–2777. doi:10.1021/acs.est.8b04737
Marti, R., Becouze-Lareure, C., Ribun, S., Marjolet, L., Souibgui, C. B., Aubin, J. B., et al. (2017). Bacteriome Genetic Structures of Urban Deposits Are Indicative of Their Origin and Impacted by Chemical Pollutants. Sci. Rep. 7, 14. doi:10.1038/s41598-017-13594-8
Masscheleyn, P. H., Delaune, R. D., and Patrick, W. H. (1991). Effect of Redox Potential and pH on Arsenic Speciation and Solubility in a Contaminated Soil. Environ. Sci. Technol. 25, 1414–1419. doi:10.1021/es00020a008
Mee, C. (2018). Soil Environmental Quality Risk Control Standard for Soil Contamination of Agricultural Land.
Mu, X., Wang, Z., Liu, L., Guo, X., Gu, C., Xu, H., et al. (2020). Multiple Exposure Pathways of First-Year University Students to Heavy Metals in China: Serum Sampling and Atmospheric Modeling. Sci. Total Environ. 746, 141405. doi:10.1016/j.scitotenv.2020.141405
O’Kelly, B. C. (2004). Accurate Determination of Moisture Content of Organic Soils Using the Oven Drying Method. Dry. Technol. 22, 1767–1776. doi:10.1081/DRT-200025642
Pett-Ridge, J., and Firestone, M. K. (2005). Redox Fluctuation Structures Microbial Communities in a Wet Tropical Soil. Aem 71, 6998–7007. doi:10.1128/aem.71.11.6998-7007.2005
Rai, P. K., Lee, S. S., Zhang, M., Tsang, Y. F., and Kim, K.-H. (2019). Heavy Metals in Food Crops: Health Risks, Fate, Mechanisms, and Management. Environ. Int. 125, 365–385. doi:10.1016/j.envint.2019.01.067
Rauret, G., López-Sánchez, J. F., Sahuquillo, A., Rubio, R., Davidson, C., Ure, A., et al. (1999). Improvement of the BCR Three Step Sequential Extraction Procedure Prior to the Certification of New Sediment and Soil Reference Materials. J. Environ. Monitor. 1, 57–61. doi:10.1039/a807854h
Roosa, S., Wattiez, R., Prygiel, E., Lesven, L., Billon, G., and Gillan, D. C. (2014). Bacterial Metal Resistance Genes and Metal Bioavailability in Contaminated Sediments. Environ. Pollut. 189, 143–151. doi:10.1016/j.envpol.2014.02.031
Ros, M., Pascual, J. A., Moreno, J. L., Hernandez, M. T., and Garcia, C. (2009). Evaluation of Microbial Community Activity, Abundance and Structure in a Semiarid Soil under Cadmium Pollution at Laboratory Level. Water Air Soil Pollut. 203, 229–242. doi:10.1007/s11270-009-0006-z
Sanyal, S. K., Mou, T. J., Chakrabarty, R. P., Hoque, S., Hossain, M. A., and Sultana, M. (2016). Diversity of Arsenite Oxidase Gene and Arsenotrophic Bacteria in Arsenic Affected Bangladesh Soils. Amb. Express. 6, 11. doi:10.1186/s13568-016-0193-0
Schneider, A. R., Gommeaux, M., Duclercq, J., Fanin, N., Conreux, A., Alahmad, A., et al. (2017). Response of Bacterial Communities to Pb Smelter Pollution in Contrasting Soils. Sci. Total Environ. 605-606, 436–444. doi:10.1016/j.scitotenv.2017.06.159
She, J. Y., Wang, J., Wei, X. D., Zhang, Q., Xie, Z. Y., Beiyuan, J. Z., et al. (2021). Survival Strategies and Dominant Phylotypes of Maize-Rhizosphere Microorganisms under Metal(loid)s Contamination. Sci. Total. Environ. 774, 145143. doi:10.1016/j.scitotenv.2021.145143
Shen, F., Liao, R., Ali, A., Mahar, A., Guo, D., Li, R., et al. (2017). Spatial Distribution and Risk Assessment of Heavy Metals in Soil Near a Pb/Zn Smelter in Feng County, China. Ecotoxicology Environ. Saf. 139, 254–262. doi:10.1016/j.ecoenv.2017.01.044
Shen, T., Liu, L., Li, Y., Wang, Q., Dai, J., and Wang, R. (2019). Long-term Effects of Untreated Wastewater on Soil Bacterial Communities. Sci. Total Environ. 646, 940–950. doi:10.1016/j.scitotenv.2018.07.223
Song, J., Shen, Q., Wang, L., Qiu, G., Shi, J., Xu, J., et al. (2018). Effects of Cd, Cu, Zn and Their Combined Action on Microbial Biomass and Bacterial Community Structure. Environ. Pollut. 243, 510–518. doi:10.1016/j.envpol.2018.09.011
Stock, W. D. (1983). An Evaluation of Some Manual Colorimetric Methods for the Determination of Inorganic Nitrogen in Soil Extracts. Commun. Soil Sci. Plant Anal. 14, 925–936. doi:10.1080/00103628309367420
Sun, M., Xiao, T., Ning, Z., Xiao, E., and Sun, W. (2015a). Microbial Community Analysis in Rice Paddy Soils Irrigated by Acid Mine Drainage Contaminated Water. Appl. Microbiol. Biotechnol. 99, 2911–2922. doi:10.1007/s00253-014-6194-5
Sun, W., Xiao, T., Sun, M., Dong, Y., Ning, Z., Xiao, E., et al. (2015b). Diversity of the Sediment Microbial Community in the Aha Watershed (Southwest China) in Response to Acid Mine Drainage Pollution Gradients. Appl. Environ. Microbiol. 81, 4874–4884. doi:10.1128/aem.00935-15
Sun, Y., Zhou, Q., Xie, X., and Liu, R. (2010). Spatial, Sources and Risk Assessment of Heavy Metal Contamination of Urban Soils in Typical Regions of Shenyang, China. J. Hazard. Mater. 174, 455–462. doi:10.1016/j.jhazmat.2009.09.074
Tian, H. J., Feng, J., Zhang, L. M., He, J. Z., and Liu, R. Y. (2020). Ecological Drivers of Methanotrophic Communities in Paddy Soils Around Mercury Mining Areas. Sci. Total. Environ. 721. 137760. doi:10.1016/j.scitotenv.2020.137760
Titah, H. S., Abdullah, S. R. S., Idris, M., Anuar, N., Basri, H., Mukhlisin, M., et al. (2018). Arsenic Resistance and Biosorption by Isolated Rhizobacteria from the Roots of Ludwigia Octovalvis. Int. J. Microbiol. 2018, 1–10. doi:10.1155/2018/3101498
Vance, E. D., Brookes, P. C., and Jenkinson, D. S. (1987). An Extraction Method for Measuring Soil Microbial Biomass C. Soil Biol. Biochem. 19, 703–707. doi:10.1016/0038-0717(87)90052-6
Vinhal-Freitas, I. C., Corrêa, G. F., Wendling, B., Bobuľská, L., and Ferreira, A. S. (2017). Soil Textural Class Plays a Major Role in Evaluating the Effects of Land Use on Soil Quality Indicators. Ecol. Indicators. 74, 182–190. doi:10.1016/j.ecolind.2016.11.020
Wang, L., Chen, L., Guo, B., Tsang, D. C. W., Huang, L., Ok, Y. S., et al. (2020a). Red Mud-Enhanced Magnesium Phosphate Cement for Remediation of Pb and as Contaminated Soil. J. Hazard. Mater. 400. doi:10.1016/j.jhazmat.2020.123317
Wang, L., Ma, L., and Yang, Z. (2018). Spatial Variation and Risk Assessment of Heavy Metals in Paddy Rice from Hunan Province, Southern China. Int. J. Environ. Sci. Technol. 15, 1561–1572. doi:10.1007/s13762-017-1504-y
Wang, Q., Garrity, G. M., Tiedje, J. M., and Cole, J. R. (2007). Naïve Bayesian Classifier for Rapid Assignment of rRNA Sequences into the New Bacterial Taxonomy. AEM 73, 5261–5267. doi:10.1128/aem.00062-07
Wang, R., Hou, D., Chen, J., Li, J., Fu, Y., Wang, S., et al. (2020b). Distinct Rhizobacterial Functional Assemblies Assist Two Sedum Alfredii Ecotypes to Adopt Different Survival Strategies under Lead Stress. Environ. Int. 143, 105912. doi:10.1016/j.envint.2020.105912
Wang, S., and Mulligan, C. N. (2006). Natural Attenuation Processes for Remediation of Arsenic Contaminated Soils and Groundwater. J. Hazard. Mater. 138, 459–470. doi:10.1016/j.jhazmat.2006.09.048
Wang, Y.-J., Chen, Z., Liu, P.-P., Sun, G.-X., Ding, L.-J., and Zhu, Y.-G. (2016). Arsenic Modulates the Composition of Anode-Respiring Bacterial Community during Dry-Wet Cycles in Paddy Soils. J. Soils Sediments. 16, 1745–1753. doi:10.1007/s11368-016-1369-6
Wang, Y., Zhao, Q. L., Hu, Y., Du, X., Ge, Wei., and Liu, W. J. (2011). Survey and Contamination Assessment of Heavy Metals in Soil and Plants Around the Pb/Zn Mine in Shangyu, Zhejiang Province (In Chinese). Environ. Chem. 30, 1354–1360.
Wang, Z., Zhang, B., He, C., Shi, J., Wu, M., and Guo, J. (2021). Sulfur-based Mixotrophic Vanadium (V) Bio-Reduction towards Lower Organic Requirement and Sulfate Accumulation. Water Res. 189, 116655. doi:10.1016/j.watres.2020.116655
Xiao, X.-Y., Wang, M.-W., Zhu, H.-W., Guo, Z.-H., Han, X.-Q., and Zeng, P. (2017). Response of Soil Microbial Activities and Microbial Community Structure to Vanadium Stress. Ecotoxicology Environ. Saf. 142, 200–206. doi:10.1016/j.ecoenv.2017.03.047
Xu, X., Zhang, Z., Hu, S., Ruan, Z., Jiang, J., Chen, C., et al. (2017). Response of Soil Bacterial Communities to Lead and Zinc Pollution Revealed by Illumina MiSeq Sequencing Investigation. Environ. Sci. Pollut. Res. 24, 666–675. doi:10.1007/s11356-016-7826-3
Xu, Y., He, Y., Zhang, Q., Xu, J., and Crowley, D. (2015). Coupling between Pentachlorophenol Dechlorination and Soil Redox as Revealed by Stable Carbon Isotope, Microbial Community Structure, and Biogeochemical Data. Environ. Sci. Technol. 49, 5425–5433. doi:10.1021/es505040c
Xu, Y., Seshadri, B., Bolan, N., Sarkar, B., Ok, Y. S., Zhang, W., et al. (2019). Microbial Functional Diversity and Carbon Use Feedback in Soils as Affected by Heavy Metals. Environ. Int. 125, 478–488. doi:10.1016/j.envint.2019.01.071
Xu, Y., Seshadri, B., Sarkar, B., Wang, H., Rumpel, C., Sparks, D., et al. (2018). Biochar Modulates Heavy Metal Toxicity and Improves Microbial Carbon Use Efficiency in Soil. Sci. Total Environ. 621, 148–159. doi:10.1016/j.scitotenv.2017.11.214
Yan, C. C., Wang, F., Geng, H. H., Liu, H. J., Pu, S. Y., Tian, Z. J., et al. (2020). Integrating High-Throughput Sequencing and Metagenome Analysis to Reveal the Characteristic and Resistance Mechanism of Microbial Community in Metal Contaminated Sediments. Sci. Total. Environ. 707, 11. doi:10.1016/j.scitotenv.2019.136116
Yang, Y., Campbell, C. D., Clark, L., Cameron, C. M., and Paterson, E. (2006). Microbial Indicators of Heavy Metal Contamination in Urban and Rural Soils. Chemosphere 63, 1942–1952. doi:10.1016/j.chemosphere.2005.10.009
Yu, Z. J., Liu, X. D., Zeng, X. B., Yin, H. Q., and Zeng, W. M. (2020). Effect of Arsenic Pollution Extent on Microbial Community in Shimen Long-Term Arsenic-Contaminated Soil. Water Air Soil Poll. 231 (7), 340. doi:10.1007/s11270-020-04716-6
Zhang, J., Li, H., Zhou, Y., Dou, L., Cai, L., Mo, L., et al. (2018). Bioavailability and Soil-To-Crop Transfer of Heavy Metals in Farmland Soils: A Case Study in the Pearl River Delta, South China. Environ. Pollut. 235, 710–719. doi:10.1016/j.envpol.2017.12.106
Zhang, X. Y., Zhong, T. Y., Liu, L., and Ouyang, X. Y. (2015). Impact of Soil Heavy Metal Pollution on Food Safety in China. Plos. One. 10, 14. doi:10.1371/journal.pone.0135182
Zhao, F.-J., Harris, E., Yan, J., Ma, J., Wu, L., Liu, W., et al. (2013). Arsenic Methylation in Soils and its Relationship with MicrobialarsMAbundance and Diversity, and as Speciation in Rice. Environ. Sci. Technol. 47, 7147–7154. doi:10.1021/es304977m
Zhen, Z., Wang, S. B., Luo, S. W., Ren, L., Liang, Y. Q., Yang, R. C., et al. (2019). Significant Impacts of Both Total Amount and Availability of Heavy Metals on the Functions and Assembly of Soil Microbial Communities in Different Land Use Patterns. Front. Microbiol. 10, 14. doi:10.3389/fmicb.2019.02293
Zheng, S. N., Wang, Q., Yu, H. Y., Huang, X. Z., and Li, F. B. (2020). Interactive Effects of Multiple Heavy Metal(loid)s on Their Bioavailability in Cocontaminated Paddy Soils in a Large Region. Sci. Total. Environ. 708, 8. doi:10.1016/j.scitotenv.2019.135126
Zhong, X., Chen, Z. W., Li, Y. Y., Ding, K. B., Liu, W. S., Liu, Y., et al. (2020). Factors Influencing Heavy Metal Availability and Risk Assessment of Soils at Typical Metal Mines in Eastern China. J. Hazard. Mater. 400. 123289. doi:10.1016/j.jhazmat.2020.123289
Zhu, N., Qiao, J., and Yan, T. (2019). Arsenic Immobilization through Regulated Ferrolysis in Paddy Field Amendment with Bismuth Impregnated Biochar. Sci. Total Environ. 648, 993–1001. doi:10.1016/j.scitotenv.2018.08.200
Keywords: Pb-Zn mining site, arsenic, lead, microbial community, paddy soil
Citation: Zou L, Lu Y, Dai Y, Khan MI, Gustave W, Nie J, Liao Y, Tang X, Shi J and Xu J (2021) Spatial Variation in Microbial Community in Response to As and Pb Contamination in Paddy Soils Near a Pb-Zn Mining Site. Front. Environ. Sci. 9:630668. doi: 10.3389/fenvs.2021.630668
Received: 18 November 2020; Accepted: 15 April 2021;
Published: 28 April 2021.
Edited by:
Jian Chen, Florida International University, United StatesReviewed by:
Xiaomin Li, South China Normal University, ChinaBaogang Zhang, China University of Geosciences, China
Copyright © 2021 Zou, Lu, Dai, Khan, Gustave, Nie, Liao, Tang, Shi and Xu. This is an open-access article distributed under the terms of the Creative Commons Attribution License (CC BY). The use, distribution or reproduction in other forums is permitted, provided the original author(s) and the copyright owner(s) are credited and that the original publication in this journal is cited, in accordance with accepted academic practice. No use, distribution or reproduction is permitted which does not comply with these terms.
*Correspondence: Jun Nie, bmllanVuMTk3QDE2My5jb20=; Xianjin Tang, eGlhbmppbkB6anUuZWR1LmNu