- 1Department of Agricultural Sciences, University of Naples Federico II, Naples, Italy
- 2Department of Biology, University of Naples Federico II, Naples, Italy
- 3Independent Researcher, Mercogliano, Italy
- 4Dipartimento Scienze Bio Agroalimentari DiSBA, Institute for Mediterranean Agricultural and Forest Systems, National Research Council of Italy, Naples, Italy
In southern Mediterranean areas, vineyards are facing the combination of increasing air temperature, drought and frequency of extreme events (e.g., heat waves) due to climate change. Since most of the berry growth and ripening phases occur during the aridity period, such environmental constraints are responsible for limitations in yield and berry quality. Within this scenario, to achieve vineyard sustainability, renewed approaches in vineyard management have been proposed and the use of plant biostimulants seems a prominent and environmental friendly practice. The aim of this study was to test four combinations of a tropical plant extract and conventional chemicals for disease control on morpho-anatomical, physiological, biochemical and berry quality in Vitis vinifera L. subsp. vinifera “Aglianico.” In particular, we aimed to evaluate the possibility to counteract the negative effects of the reductions in copper distribution, by applying the tropical plant extract enriched with: micronutrients, enzymes involved in the activation of natural defense, aminoacids, and vitamins. The halved dose of Cu in combination with the tropical plant extract allowed maintaining a reduced vegetative vigor. In the second year of treatment, the addition of the plant extract significantly improved leaf gas exchanges and photochemistry as well as the synthesis of photosynthetic pigments. At berry level, the plant extract induced an increase in phenolics accompanied by a decrease in soluble sugars. The overall results showed that the expected differences in growth performance and productivity in vines are linked to different eco-physiological and structural properties induced by the various treatments. The tropical plant extract also primed plant defenses at the leaf and fruit levels, mainly due to modifications of some structural and biochemical traits, respectively.
Introduction
The scientists and extension specialists are called to make local farming communities and crop production more resilient to climate change. There is an urgent need for developing mitigation and adapting solutions to cope with the scarcity of natural resources and to the increase yield stability under multiple stress conditions. Such objectives can be achieved through a sustainable agriculture targeted to improve the use efficiency of farm resources, simultaneously increasing crop yield and quality. Indeed, the climate conditions for world winegrowing regions are expected to change (Webb et al., 2013) with severe repercussions on viticulture which is an high-income agricultural sector (Jones and Webb, 2010; Goode, 2012).
In Europe, where viticulture is one of the most important agricultural sectors (average annual production of 168 million hectoliters, 54% of global consumption; FAOSTAT, 2020), a decrease in rainfall, associated with an increase in temperature, is expected especially in the Mediterranean region (IPCC, 2014). The combination of high air temperature and water deficit, coupled with marked inter-annual and intra-annual climate variability and scarce water resources (Costa et al., 2007; Rogiers and Clarke, 2013; Lopes et al., 2014; Valverde et al., 2015), leads to a severe depletion in soil water availability resulting in an important vulnerability of rainfed agriculture. Mediterranean viticulture is highly threatened by such projected environmental limitations because berry growth and ripening occur under conditions of high air temperature and soil water deficit which may limit yield and berry quality (Medrano et al., 2003; Chaves et al., 2007, 2010; Lereboullet et al., 2013, 2014). Moreover, this phenomenon is exacerbated by the competition for water resource with other sectors (e.g., industry) and by the prohibition of irrigation in most “Demarcation of controlled production areas” (DOC).
Other than climate change, European viticulture is facing also a legislative rearrangement which includes several restrictions in the use of chemical compounds suitable for pest and disease management. In particular, copper compounds, that have been used for more than one century mainly to control downy mildew in vineyards at relatively high rates depending on climatic conditions, caused a metallic copper accumulation in the topsoil of many vineyards (Rusjan et al., 2007), especially in organic cultivation. Therefore, the use of copper fungicides has been strongly restricted by the European Commission, firstly by the UE Reg. n. 889//2008, that allowed to use no more than 6 kg ha–1 of metallic copper per year, and recently even more by UE Reg. n. 1981/2018 that limits the amount of metallic copper to the maximum cumulated threshold of 28 kg ha–1 in seven consecutive years. However, despite its unfavorable ecotoxicological profile, copper is still tolerated due to its distinctiveness as wide spectrum fungicide, at least until an alternative product or control strategy will be identified (Dagostin et al., 2011). During the last two decades, significant efforts have been done by researchers and by European agricultural policy makers to comply with environmental safety and organic farming needs in the screening and evaluation of several alternatives to metallic copper compounds, including both inorganic substances at lower concentration of copper and plant extracts (Cohen et al., 2006; Chuang et al., 2007).
In this framework, securing yield stability and improving berry quality under multiple/combined stressful conditions (i.e., high temperature and drought stress) are two important goals of viticulture, winemaking industry and scientists, especially in a context of climate change (Poni et al., 2018). A promising, efficient and sustainable innovation for the achievement of such objectives could be the use of biostimulants (Rouphael and Colla, 2018, 2020). The definition of biostimulants has been intensively debated over the last decade, mainly for regulatory purposes (du Jardin, 2012, 2015; Yakhin et al., 2017; Caradonia et al., 2019). Recently under the EU fertilizer regulation 2019/1009: a plant biostimulant shall be “an EU fertilizing product able to modify plant physiological functions, with the objectives of enhancing one or more of the following agronomic claims: i) tolerance/resistance to abiotic stressors, ii) nutrient uptake and efficiency, iii) qualitative characteristics and iv) availability of nutrients confined in the rhizosphere or to the soil” (European Union [EU], 2019). The effectiveness of natural plant biostimulants (humic acids, seaweed and plant extracts, protein hydrolyzates, and silicon) in imparting tolerance for horticultural crops (fruit trees, grapevine, and vegetables) against sub-optimal conditions (high temperature and radiation, drought, and biotic pressure), has been attributed to several putative direct and indirect physiological and molecular mechanisms. Among these, there are: 1) enhanced macro and micronutrient uptake due to a modulation of the root system architecture in terms of biomass, soil exploitation, branching and density, 2) increased physiological status (higher leaf CO2 exchange rates, stomatal conductance, leaf water potential, and water use efficiency), 3) regulation of key genes involved in detoxification process and synthesis of osmolytes (proline, glycinebetaine and sorbitol), and 4) modulation of phytohormone signaling (Mancuso et al., 2006; Calvo et al., 2014; Yakhin et al., 2017; Rouphael and Colla, 2020). Researches on the beneficial use of biostimulants in the fruit production sector, grapevine in particular, are still limited compared to cereals and vegetables, and the findings are not always consistent in terms of repeatability and efficacy (Basile et al., 2020). This evidence is probably associated to the perennial nature of the woody tree species and the variability of the environmental conditions occurring year after year under field conditions.
Despite the fact that several studies regarding the application of biostimulants in the frame of modulating the nutritional, functional and aromatic profile of berries, musts and wines composition have been conducted (Parrado et al., 2007; Ferrara and Brunetti, 2010; Martínez-Gil et al., 2012, 2013; Sánchez-Gómez et al., 2016a, b; Frioni et al., 2018; Popescu and Popescu, 2018), the information on the effects of biostimulant application on grapevine morpho-anatomical, biochemical and physiological response mechanisms to counteract biotic/abiotic stresses is still missing.
The aim of this experiment was to evaluate, in a concurrent climate and regulation change scenario, the application of a plant extract to mitigate possible negative effects due to the reduced doses of synthetic chemicals, in particular metallic copper, in Vitis vinifera L. subsp. vinifera “Aglianico.” Therefore, four combinations of a tropical plant extract and conventional chemicals for disease control were applied in a vineyard in southern Italy, over two years, and vine response was evaluated in terms of growth performance, morpho-physiological traits and berry quality.
Materials and Methods
Plant Material, Cultural Practices and Experimental Design
The experimental trial was conducted in a commercial vineyard (Fonzone-Caccese winery, F-Cw) (40°57′50.0′′N 15°03′49.8′′E, 400–450 m asl), located in Paternopoli (Avellino, Campania region, Southern Italy). The study was conducted during two consecutive growing seasons 2017–2018 on Vitis vinifera L. “Aglianico” (clone VCR 23), grafted onto 420 A (V. riparia × V. berlandieri). Vines were planted in 2006 on a medium west-faced slope (about 10%), with W-E row direction. A meteorological wireless station (WatchDog, PCE Instruments) was installed at the beginning of 2017 to collect air temperature and rainfall data during the two years of experimental trials.
Along the slope, two different soils (in the upper and lower parts, Haplic Calcisols and Calcaric Cambisols, respectively; IUSS Working Group WRB, 2015) were identified by Brook et al. (2020) through a pedological survey supported by georadar investigations. Although both soils were classified as silt loam, they showed a different hydraulic behavior due to the different presence of sand and rock fragments in their horizons (Brook et al., 2020). Therefore, the experimental trial was realized only on the slope surface characterized by the presence of Calcisol.
Plantation spacing was 2.2 × 1 m, yielding a plant density of 4,500 vines ha–1; vines were trained to a vertical shoot positioned espalier system, spur pruned (horizontal spur cordon at 90 cm height from the ground), with a bud load of about 10 vine–1 on 5 spurs. During both years, canopy management operations were performed in terms of suckering (i.e., the mechanical removing of shoots arising from the trunk and from the cordon, except those from spurs) and vertical shoot positioning.
The experimental trials were based on different treatments for disease control management. More specifically, different spraying plant protection mixtures were obtained by the combination of two levels of a copper oxychloride commercial product (Coprantol WG, Syngenta) and only one level of wettable sulfur (Tiovit jet, Syngenta) with or without the addition of a tropical plant extract in liquid formulation (Trym®, Italpollina, Rivoli Veronese, VR, Italy). The trials were conducted on 12 adjacent vine rows (3 rows × 4 disease control treatments) of a vineyard homogeneous for exposition, slope and pedological characteristics. The experimental design compared four treatments: T1, F-Cw industry water mixture of copper oxychloride (thus considered the control treatment), obtained following ordinary dose prescriptions (400 g hL–1) for copper oxychloride and wettable sulfur (400 g hL–1); T2, F-Cw industry water mixture (copper oxychloride at 400 g hL–1 + sulfur at 400 g hL–1) plus Trym® (0.5 lt ha–1); T3, F-Cw industry water mixture with halved dose of copper oxychloride (200 g hL–1) and ordinary dose for wettable sulfur (400 g hL–1); T4, F-Cw industry water mixture with halved dose of copper oxychloride (200 g hL–1) and ordinary dose for wettable sulfur (400 g hL–1) plus Trym® (0.5 lt ha–1). The mixtures were sprayed directly on canopy with a shoulder sprayer pump (GeoTech SP 300 4T), equipped with three flat spray nozzles, operating at a pressure of about 15 bar, dispensing up to 10 hL of water solution according to the canopy growth. During both years, six spraying applications were performed, starting from the phenological phase of the third-fourth leaves unfolded (BBCH 13-14) to the berry touch complete (BBCH 79) as reported in Lorenz et al. (1995).
Biostimulant Characteristics
The tropical plant extract biostimulant Trym® was provided by the Italpollina Company (Rivoli Veronese, Italy). Trym® is a commercial plant biostimulant produced through water extraction and fermentation of tropical plant biomass from Aloe spp. and Hybiscus spp. The final product contains mostly micronutrients, enzymes involved in the activation of natural defense genes (proteases, N-acetyl-D-glucosamine kinase, B-glucuronidase, and B-galactosidase), aminoacids, and vitamins. Trym® has a density of 1.023 kg L–1, a pH of 4.1. It contains 0.85 g kg–1 of total proteins, 6.22 g kg–1 of sugars (3.97 g kg–1 of glucose, 2.10 g kg–1 of fructose, and 0.15 g kg–1 of sucrose) and 1.62 g kg–1 of starch.
It contains 221 g kg–1 of free amino acids, including 56 g kg–1 of essential aminoacids. The aminogram of the product (in g kg–1) is as follows: Ala (0.29), Arg (0.20), Asn (0.12), Asp (0.38), Glu (0.01), Gly (0.10), His (0.14), Ile (0.22), Leu (0.48), Lys (0.14), Orn (2.92) Phe (5.01), Pro (0.32), Ser (0.26), Thr (0.08), Trp (4), Tyr (0.05), and Val (0.23). The total phenolics, determined following the methods reported by Carillo et al. (2019), are 6.51 mg of gallic acid equivalent per gram of f.w. product. The Trym® mineral composition, determined by ion chromatography as described by Cirillo et al. (2019), is as follows (g kg–1 f.w): N–NH4 (0.92), N–NO3 (1.92), K (2.71), Na (1.64), Ca (2.86), Mg (2.02), Cl (5.12), and SO4 (13.30). No detectable phytohormones have been reported in Trym®.
Biometric Analyses and Yield
Biometric analyses were performed during four main phenological phases:pre-flowering, fruit set, veraison and harvest on 15 plants per treatment, selected on the central row of each treatment, in order to avoid drift interference. Two-year-old shoots (holding the production of the year) per plant were selected to monitor growth by recording shoot length, number of leaves and leaf area. Leaf area was estimated by measuring the lamina width and applying the equations calculated based on the measurement of width and area of 25 leaves per treatment by means of a electronic leaf area meter (LI-3100 model, LI-COR Inc., Lincoln, Nebraska, United States), according to Caccavello et al. (2017).
The bud break rate was determined on the selected shoots as the ratio between the number of shoots and buds.
The fruit set was also analyzed on the same shoots. More specifically, all the bunches on the shoots were photographed with a digital camera and images were subjected to digital image analysis through the software Image J (Rasband, NIH) to count the number of visible flowers per bunch. On the same date, from other plants, 12 bunches for treatment were photographed and sampled: for them, the number of flowers per bunch was counted both through digital image analysis and manually to achieve the real flower number. The relationships between the flowers counted through image analysis and the real number of flowers were extracted and the equations used to estimate the real number of flowers per bunch also in all the other bunches. In addition, at the harvest phase, the same procedure was repeated for the berries in order to calculate the fruit set rate as the ratio between the number of berries and of flowers. Finally, at the harvest phase, the number of bunches, their weight (total yield per vine) and the berry diameter (on 150 fruits per treatment) were also recorded. Vine fertility was then estimated as both real fertility (RF, the bunch number per number of buds) and potential fertility (PF, the bunch number per number of shoots.
Leaf Gas Exchange and Chlorophyll “a” Fluorescence Emission
Leaf gas-exchange and chlorophyll “a” fluorescence emission measurements were carried out on 2 well-exposed and fully expanded leaves per 15 plants during the veraison phase of the two growing seasons (2017–2018). Net CO2 assimilation rate (Pn) and stomatal conductance (gs) were performed by means of a portable infra-red gas-analyzer (LCA 4; ADC, BioScientific, Hoddesdon, United Kingdom) equipped with a broad-leaf PLC (cuvette area 6.25 cm2). Chlorophyll “a” fluorescence emission was measured using a portable FluorPen FP100 Max fluorometer with a light sensor (Photon System Instruments, Brno, Czech Republic). A blue LED internal light of 1–2 μmol photons m2 s–1 was used to induce the ground fluorescence F0 on 30′ dark adapted leaves. A saturating light pulse of 3.000 μmol photons m2 s–1 was applied to induce the maximal fluorescence level in the dark, Fm. The following parameters were considered: the maximum PSII photochemical efficiency (Fv/Fm) calculated as (Fm − F0)/Fm, the quantum yield of PSII linear electron transport (ΦPSII) and non-photochemical quenching (NPQ) (Genty et al., 1989; Bilger and Björkman, 1990). The measurements in the light were conducted from 12:00 to 14:00 pm under environmental Photosynthetic Photon Flux Density (PPFD) ranging between 1,800 and 2,300 photons m2 s–1.
Leaf Traits and Chlorophyll Quantification
During the growing season 2018, at the veraison phase, leaf functional traits, namely specific leaf area (SLA), leaf dry matter content (LDMC), and relative water content (RWC) were determined on 10 well-exposed and fully expanded leaves per treatment, following the methods reported in Cornelissen et al. (2003). The SLA was calculated as the ratio between leaf area and leaf dry mass (cm2 g–1). LDMC was measured as the leaf oven-dry mass (at 75°C for 48 h) divided by its water-saturated fresh mass and expressed as g g–1 wslm (water-saturated leaf mass). The RWC was expressed as percentage of (fresh weight − dry weight)/(saturated weight − dry weight). The saturated fresh weight was measured submerging the petiole of leaf blades in distilled water for 48 h in the dark.
From the same plants, five leaves per treatment were collected and used for the extraction of chlorophylls and carotenoids. Pigments were extracted in ice-cold 100% acetone with a mortar and pestle and centrifuged at 5,000 rpm for 5 min (Labofuge GL, Heraeus Sepatech, Hanau, Germany). The absorbance of supernatants was quantified by a spectrophotometer (UV-VIS Cary 100, Agilent Technologies, Santa Clara, CA, United States) at wavelengths of 470, 645, and 662 nm. The pigment content was calculated according to Lichtenthaler (1987) and expressed in μg cm–2.
Functional Anatomical Traits in Leaves and Fruits
During the growing season 2018, at the veraison phase, five fully expanded leaves were sampled from five plants per treatment. Each leaf was dissected to obtain two sub-samples from the median region of the lamina: one devoted to thin sectioning, the other to peeling for stomata characterization. The subsamples were immediately submerged in the FAA chemical fixative (5 mL 40% formaldehyde, 5 mL glacial acetic acid, and 90 mL 50% ethanol) for several days. During the harvest phase, five berries from five plants per each treatment were collected by dissecting them directly on the plant in order to analyze fruit samples having the same exposition to the light. Fruit samples were fixed in FAA as well. The sampled leaves and berries were further dissected under a reflected light microscope (SZX16, Olympus, Hamburg, Germany) to obtain subsamples of leaves (5 × 6 mm) and berries (8 × 8 mm, removing the seeds) which were dehydrated in an ethanol series (up to 95%), infiltrated and embedded in the JB4® acrylic resin (Polysciences, United States). Cross sections of the leaf lamina and longitudinal sections of the fruits were cut by means of a rotary microtome at 5 μm thickness. Leaf sections were stained with 0.5% toluidine blue in water (Feder and O’Brien, 1968), mounted with mineral oil for microscopy, and observed under a transmitted light microscope (BX60, Olympus, BX 60). Fruit sections were mounted, unstained, with mineral oil for fluorescence microscopy and observed under an epi-fluorescence microscope (BX60, Olympus) equipped with a mercury lamp, band-pass filter of 330–385 nm, dichromatic mirror of 400 nm and above, and a barrier filter of 420 nm and above, for the observation of simple phenolic compounds and suberized/lignified cell walls (Fukazawa, 1992; Ruzin, 1999; De Micco and Aronne, 2007). Images of leaf and fruit sections at different magnifications were captured though a camera (CAMEDIA C4040, Olympus) and analyzed through the image analysis software Olympus AnalySIS 3.2, in order to quantify the following morpho-anatomical traits: the thickness of the leaf lamina (total leaf thickness, TLT), of the palisade and spongy parenchyma (palisade tissue thickness, PT and spongy tissue thickness, ST); the ratio between the thickness of the palisade parenchyma and the thickness of the entire foliar lamina (PT/TLT); the ratio between the thickness of the spongy parenchyma and the thickness of the entire foliar lamina (ST/TLT); the percentage of intercellular spaces per surface area in the spongy parenchyma (intercellular spaces, IS); the thickness of the collenchyma located in the subepidermal portions of the upper (TCU) and lower (TCL) lamina surfaces at the vein level.
As regards the quantification of stomata traits, the abaxial epidermis was carefully peeled off with a tweezer, and the epidermis strips were flattened and mounted on a glass slide with distilled water. Three film strips from each sample were observed under a transmitted light microscope (BX60, Olympus, Hamburg, Germany). Digital images of the epidermis were collected and analyzed as reported above to measure: stomata frequency (SF), calculated by counting the number of stomata in five regions of the epidermis and expressed as the number of stomata per mm2; the guard cell length (GCL), quantified by measuring the length pole to pole, and the guard cell width (GCW) in the median position, in 20 stomata per sample.
Leaf Mineral Composition
During both growing seasons, at the veraison phase, six fully expanded leaves per treatment were sampled. Leaf dry tissues were finely ground with a mill (IKA, MF10.1, Staufen, Germany) with 0.5 mm-sieve. For the evaluation of mineral leaf composition in terms of cations (Na, NH4, K, Mg, and Ca), anions (NO3, SO4, PO4, and Cl) and organic acids (malate, tartrate, citrate, and isocitrate), 250 mg of dried material were suspended in 50 mL of ultrapure water (Milli-Q, Merk Millipore, Darmstadt, Germany), freezed and subjected to 10 min shaking in a water bath (ShakeTemp SW22, Julabo, Seelbach, Germany) at 80°C. Anions and cations were separated and quantified by ion chromatography equipped with a conductivity detection (ICP 3000 Dionex, Thermo fisher Scientific Inc., MA, United States), according to Zhifeng and Chengguang (1994).
Fruit Quality Traits
During both growing seasons, at the harvest phase, analytical determinations of standard chemical parameters, namely soluble solids content (SSC), juice pH and titratable acidity (TA), were carried out on a sample of 10 berries per each of 15 plants per treatment. To ensure a representative sample, berries were picked from the top to the bottom and from the internal to the external part of the bunch. The SSC was determined by refractometric analysis (HI96801 digital refractometer, HANNA Instruments Italia Srl, Padua) on unfiltered juice, obtained by squeezing the berries (European Union [EU], 1990), and expressed in Brix. The remaining juice was filtered and diluted 1:1 in distilled water and used to measure the juice pH and the TA. Juice pH values were recorded using a digital pH meter (CLB22, Crison Instruments, Alella, Barcelona, Spain); whereas for TA determination, diluted sample were titrated with a 0.1 N NaOH solution up to pH 8.2 and the titratable acidity was expressed as g L–1 of tartaric acid equivalent.
Statistical Analysis of Data
All experimental data were analyzed with the SPSS 13 statistical software (SPSS Inc., Chicago. IL, United States). The growth data were analyzed by three-way analysis of variance (ANOVA) considering the year (Y), the foliar treatment (T) and the phenological phase (PP) as main factors. A two-way ANOVA was performed on data collected at fruit set for shoot fertility and fruit set, and at harvest for yield components (total bunch weight and the number of bunches per vine) and for main qualitative parameters of berries (average berry diameter, SSC, pH, and TA), considering the year (Y) and the foliar treatment (T) as main factors. Whenever the interactions were significant, a one-way ANOVA was performed. Leaf traits and anatomical data were subjected to one-way ANOVA. To separate treatments per each measured parameter, the Duncan’s multiple range test was performed. The verification of normality was performed through the Shapiro–Wilk test; the percentage data were previously subjected to arcsine transformation.
Results
Meteorological Course
The montly average mean air temperature was similar during the two growing seasons, with an annual mean temperature of 14.2 and 14.5°C in 2017 and 2018, respectively (Figure 1A). The hottest month was August in 2017 (monthly average mean temperature of 26.2°C; maximum temperature of 34.9°C), while July in 2018 (monthly average mean temperature of 23.8°C; maximum temperature of 30.9°C). The cumulative annual precipitation was 568 mm in 2017, while 955 mm in 2018. The monthly precipitation of July and August was of 6.3 and 0.5 mm, respectively in 2017, while it was of 7.6 and 110.4 in 2018. Therefore, during the second year of trial the calculated difference between monthly rainfall and ET0 indicates a lower water availability during the grapevine growing season in 2017 (Figure 1B).
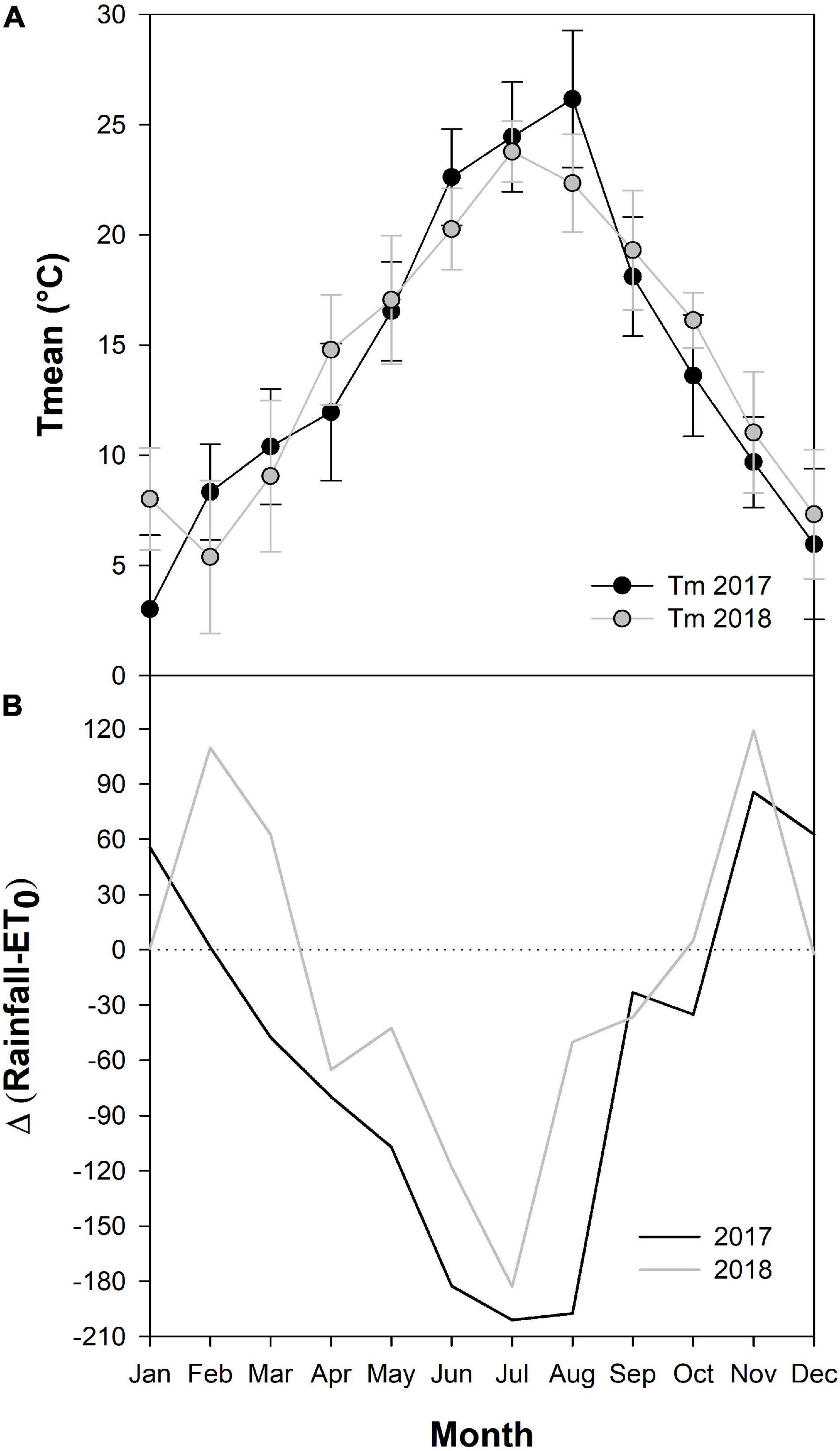
Figure 1. Diagrams showing the monthly average mean air temperature (A) and water deficit (calculated as difference between rainfall and ET0) (B) for 2017 (black line) and 2018 (gray line).
Growth Analysis and Production
Growth parameters (bud break percent, shoot length, number of leaves, and leaf area per shoot) of the four treatments applied to Aglianico vines, measured at the four phenological phases during the two growing seasons, are reported in Table 1. Considering the effects of year as main factor, results showed that in 2018, all growth parameters were significantly higher than 2017. Concerning the foliar treatment, the T4, containing Cu at 50% and Trym®, caused a significant reduction of all growth parameters but bud break percent. In particular, the average shoot length was reduced by 17% compared to T1 (Cu at 100%) and by about 9% compared to both T2 (Cu at 100% and Trym®) and T3 (Cu at 50%) (Table 1). Similarly, the total leaf area and leaf number per shoot were significantly decreased by T4 application compared to the other treatments (Table 1).
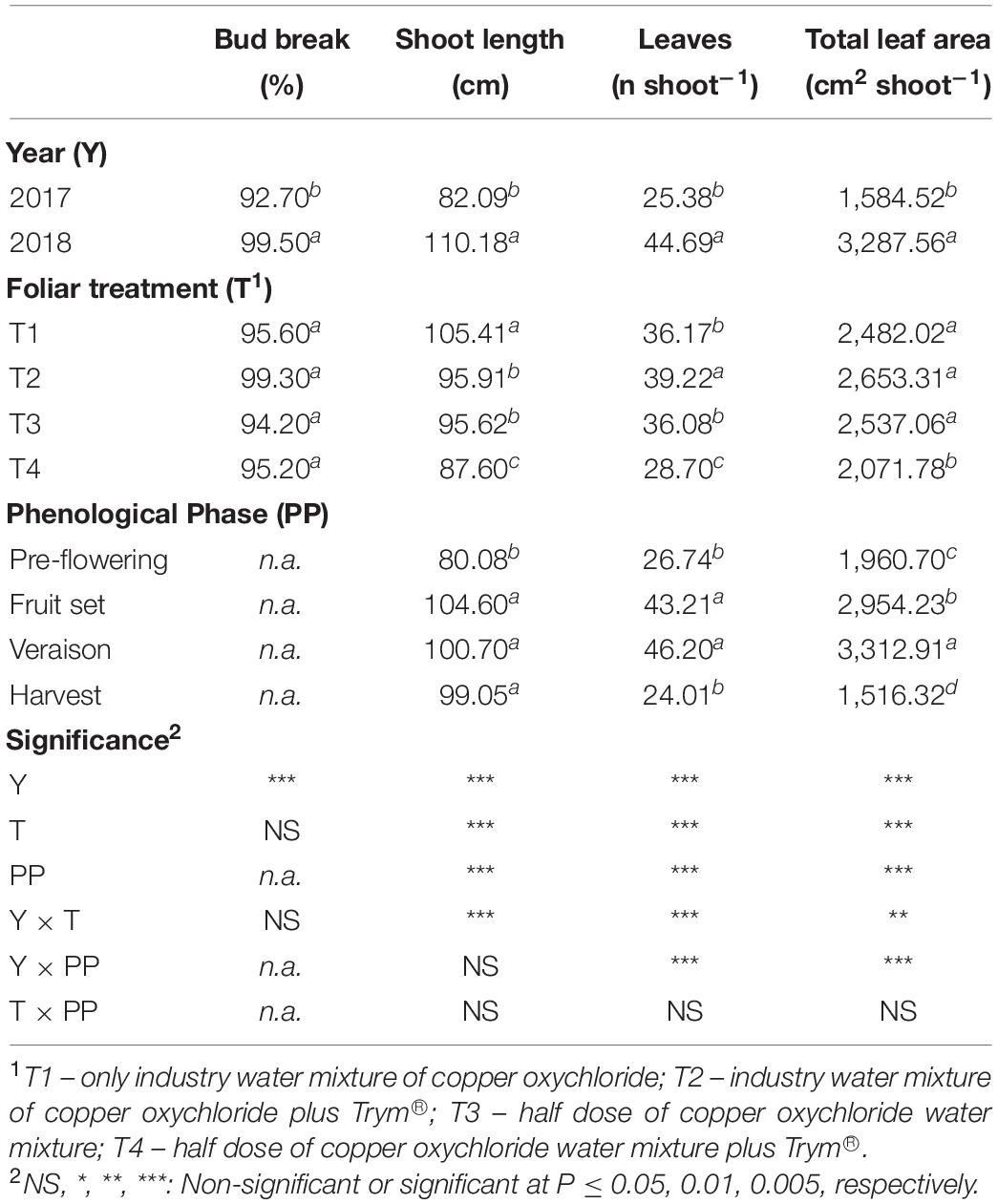
Table 1. Main effects of year, foliar treatment and phenological phase on vine bud break, shoot length, number of leaves per shoot, total leaf area per shoot of V. vinifera “Aglianico” vines. Mean values and significance of main factors’ interactions are shown. Different letters within column indicate significant differences according to Duncan’s multiple-range test (P ≤ 0.05).
Overall, among the vegetative phases, the major increment of growth parameters was recorded at veraison. The interaction between year and foliar treatment (Y × T) was significant for the average shoot length, number of leaves, and total leaf area (Table 1). In detail, the highest shoot length was observed in T1 during the year 2018; moreover, this parameter resulted significantly lower in all the other treatments both in 2017 and in 2018, with the lowest value in T4 in 2017 (Figure 2A). Similarly, the T4 treatment in 2017 induced the lowest leaf number and leaf area per shoot, whereas T2, the treatment containing the same dose of Cu but added with Trym®, showed the highest values (Figures 2B,C).
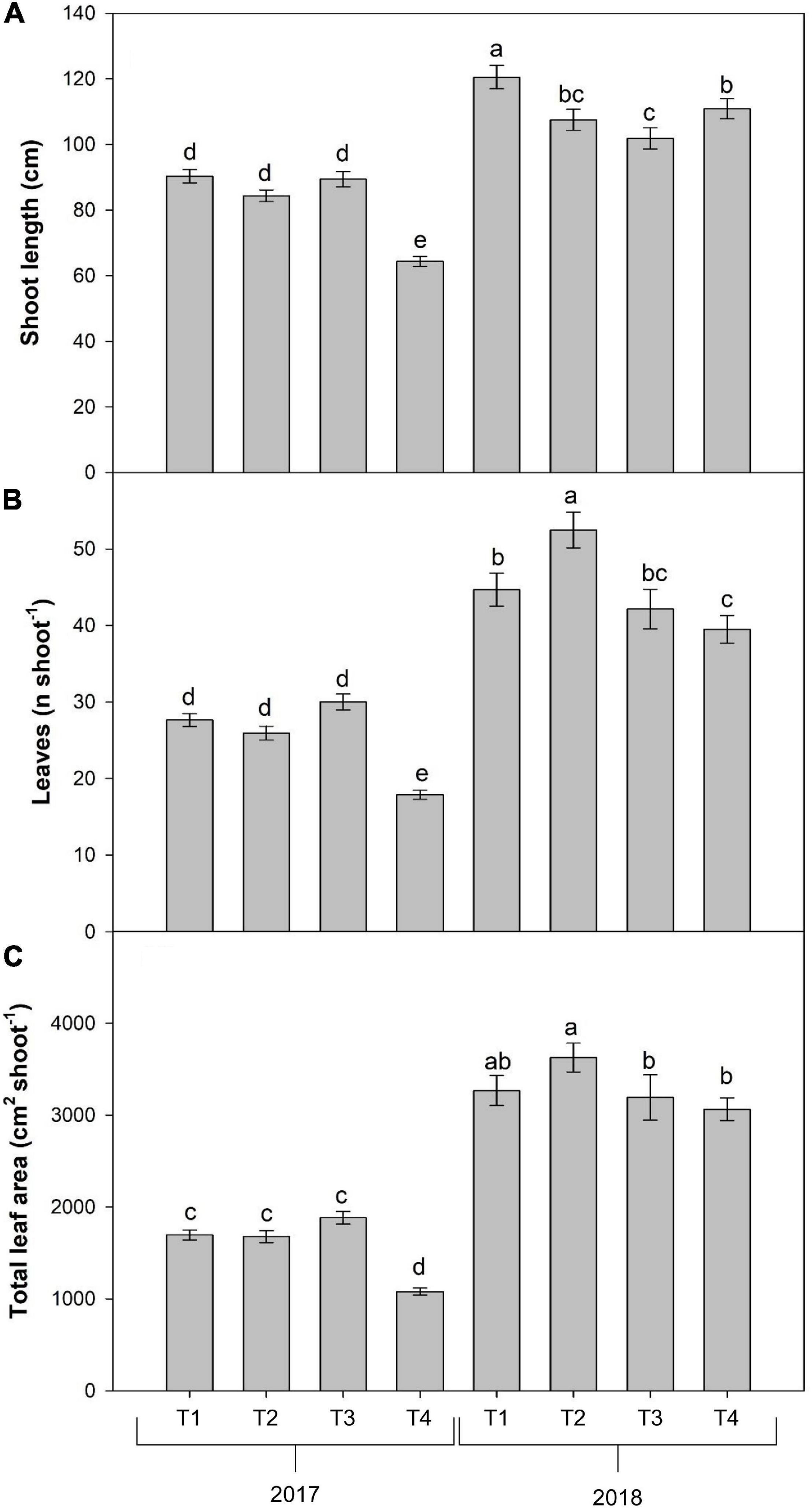
Figure 2. Combined effects of year and foliar treatments shoot length (A), leaf number (B) and total leaf area (C) per shoot of V. vinifera “Aglianico” vines (mean ± SE). Different letters indicate significant differences according to Duncan’s multiple-range test (P ≤ 0.05). [T1 – only industry water mixture of copper oxychloride; T2 – industry water mixture of copper oxychloride plus Trym®; T3 – half dose of copper oxychloride water mixture; T4 – half dose of copper oxychloride water mixture plus Trym®].
Significant differences were also found in grapevine production components (Table 2). Compared to 2017, in 2018 there was a decrease in potential fertility (−13.3%) and in the number of bunches per vine (−41%), and a contemporary increase in the fruit set (+19.4%) and in the average diameter of berries (+28.1%). The real fertility and weight of bunches per plant, however, were not significantly influenced (Table 2).
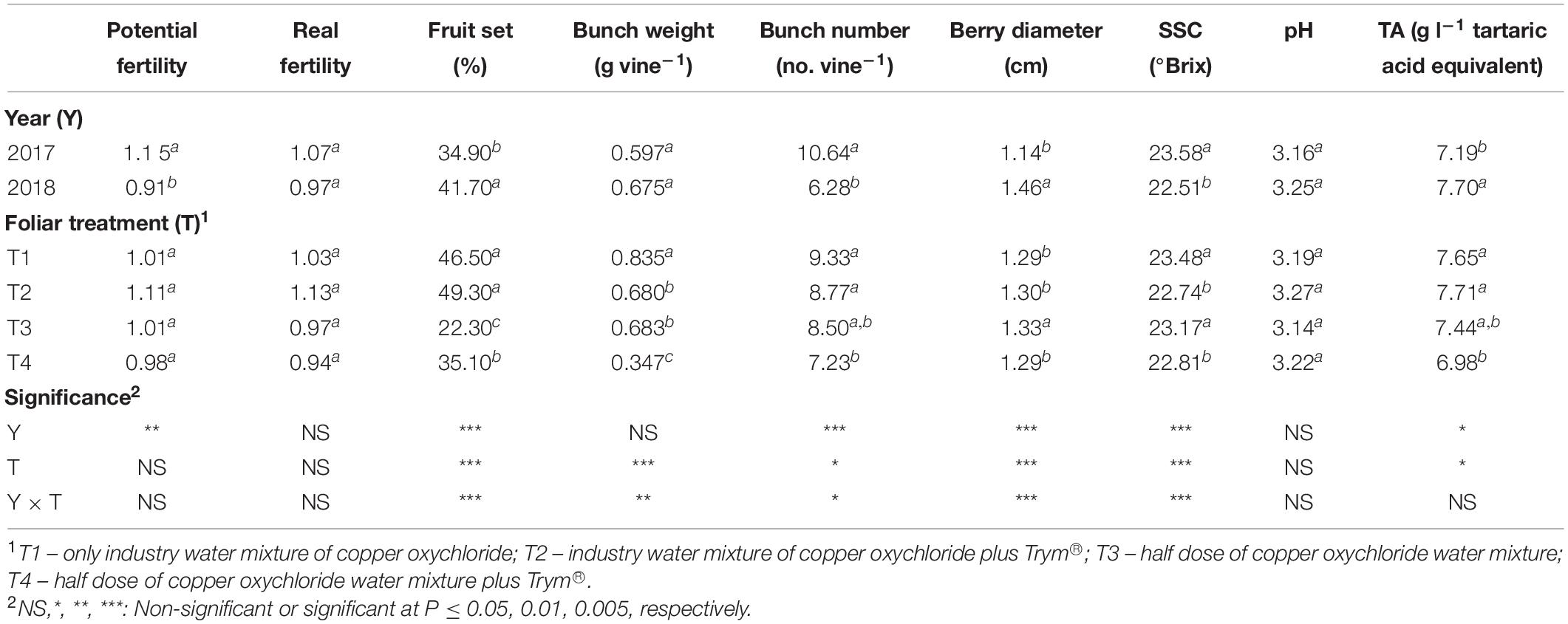
Table 2. Main effects of year and foliar treatment on potential and real fertility of shoots, fruit set, bunch weight and number, berry diameter, berry juice soluble solids content (SSC), pH, and titratable acidity (TA) of V. vinifera “Aglianico” vines. Mean values and significance of main factors’ interactions are shown. Different letters within column indicate significant differences according to Duncan’s multiple-range test (P ≤ 0.05).
Fruit set was affected by the Y × T interaction, with foliar treatment T1 and T2 in 2018 resulting in 54 and 53% of fruit set, followed by T2 (45%) and T1 (39%) in 2017 and T4 (44%) in 2018 as intermediate values, finally by T3 (28.5%) and T4 (26.5%) in 2017 and T3 (16%) in 2018.
The Y × T interaction resulted significant in all the main components of the yield, since the bunch number per vine was generally higher during 2017, with the highest number of bunches obtained in T1 treatment, whereas the lowest values were recorded in T2 and T4 treatments of 2018 (Figure 3A). On the contrary, the T1 treatment in 2018 induced the highest yield in terms of total bunch weight (nearly 1 kg per vine) and the T4 treatment in 2017 showed the lowest yield harvested (Figure 3B). Furthermore, even though in general the berry size resulted significantly increased in all the treatments in 2018, the T4 treatment in 2018 showed the highest value and the overall lowest one in 2017 (Figure 3C). Finally, SSC of berries was highest in T1 and T3 in 2017, intermediate in T2 and T4 in 2017 and T1 in 2018 and reached the lowest level in T2, T3, and T4 in 2018 (Figure 3D).
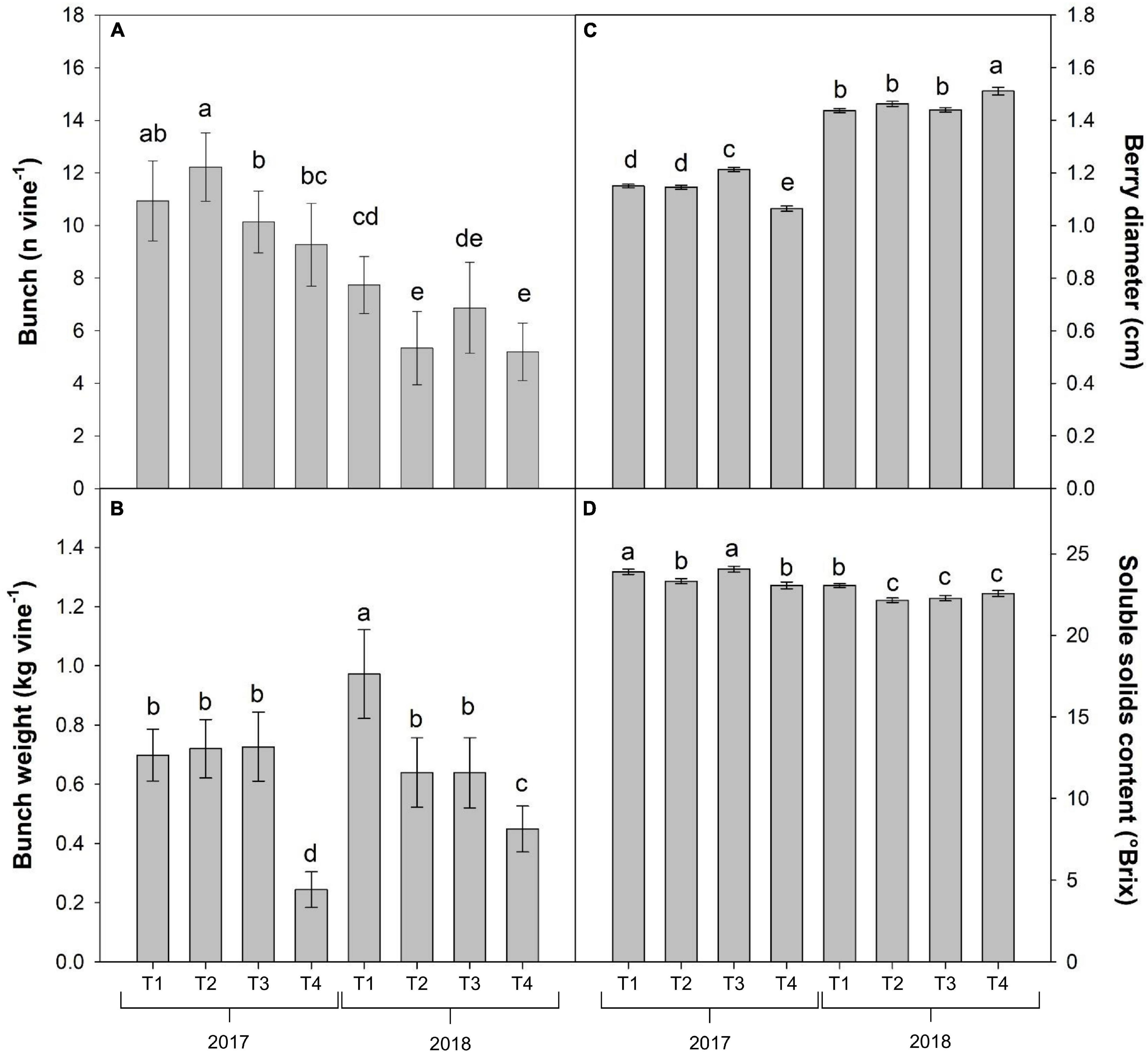
Figure 3. Combined effects of year and foliar treatments on bunches number (A) and weight (B) per vine and berry diameter (C) and soluble solids content (D) in V. vinifera “Aglianico” vines (mean ± SE). Different letters indicate significant differences according to Duncan’s multiple-range test (P ≤ 0.05). [T1 – only industry water mixture of copper oxychloride; T2 – industry water mixture of copper oxychloride plus Trym®; T3 – half dose of copper oxychloride water mixture; T4 – half dose of copper oxychloride water mixture plus Trym®].
Leaf Gas Exchange, Chlorophyll Fluorescence Emission and Photosynthetic Pigment Quantification
With the exception of stomatal conductance (gs), which was affected by the year only (with the highest values recorded in 2018), the physiological data at veraison, in particular the net photosynthetic rate (Pn) and the transpiration rate (E), were influenced by the interaction Y × T (Table 3). Indeed, Pn reached the highest level in T2 in 2018 and the lowest in T2 in 2017, whereas all the other treatments were intermediate (Figure 4A). Differently, the highest value of transpiration rate was found in T3 and T2 of year 2017 (3.63 and 3.28 mol H2O m–2s–1, respectively), whereas all the treatments in 2017 reached the lowest values (on average 0.93 mol H2O m–2s–1) (data not shown).
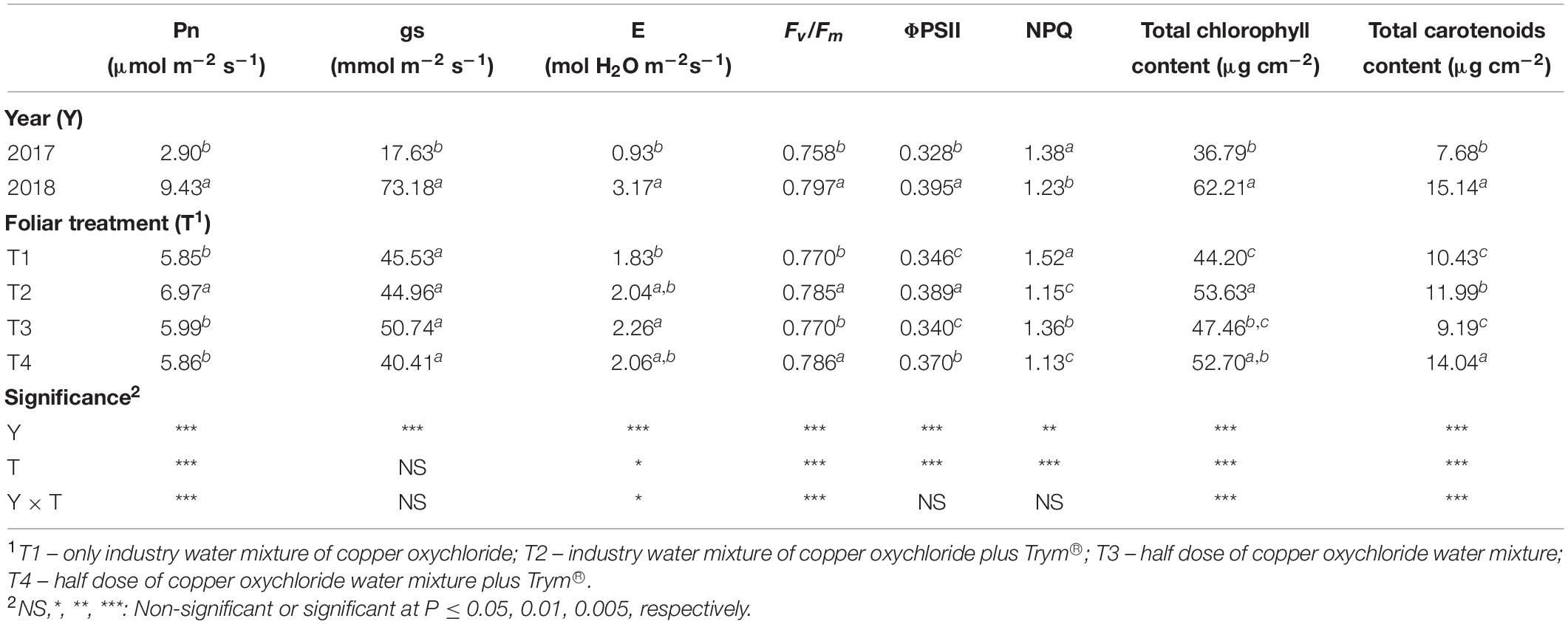
Table 3. Main effects of year and foliar treatment, on net photosynthetic rate (Pn), stomatal conductance (gs), leaf transpiration rate (E), maximum quantum efficiency of PSII photochemistry (Fv/Fm), quantum yield of PSII linear electron transport (ΦPSII), non-photochemical quenching (NPQ) and leaf pigment quantification of V. vinifera “Aglianico” vines at veraison. Mean values and significance of main factors’ interactions are shown. Different letters within column indicate significant differences according to Duncan’s multiple-range test (P ≤ 0.05).
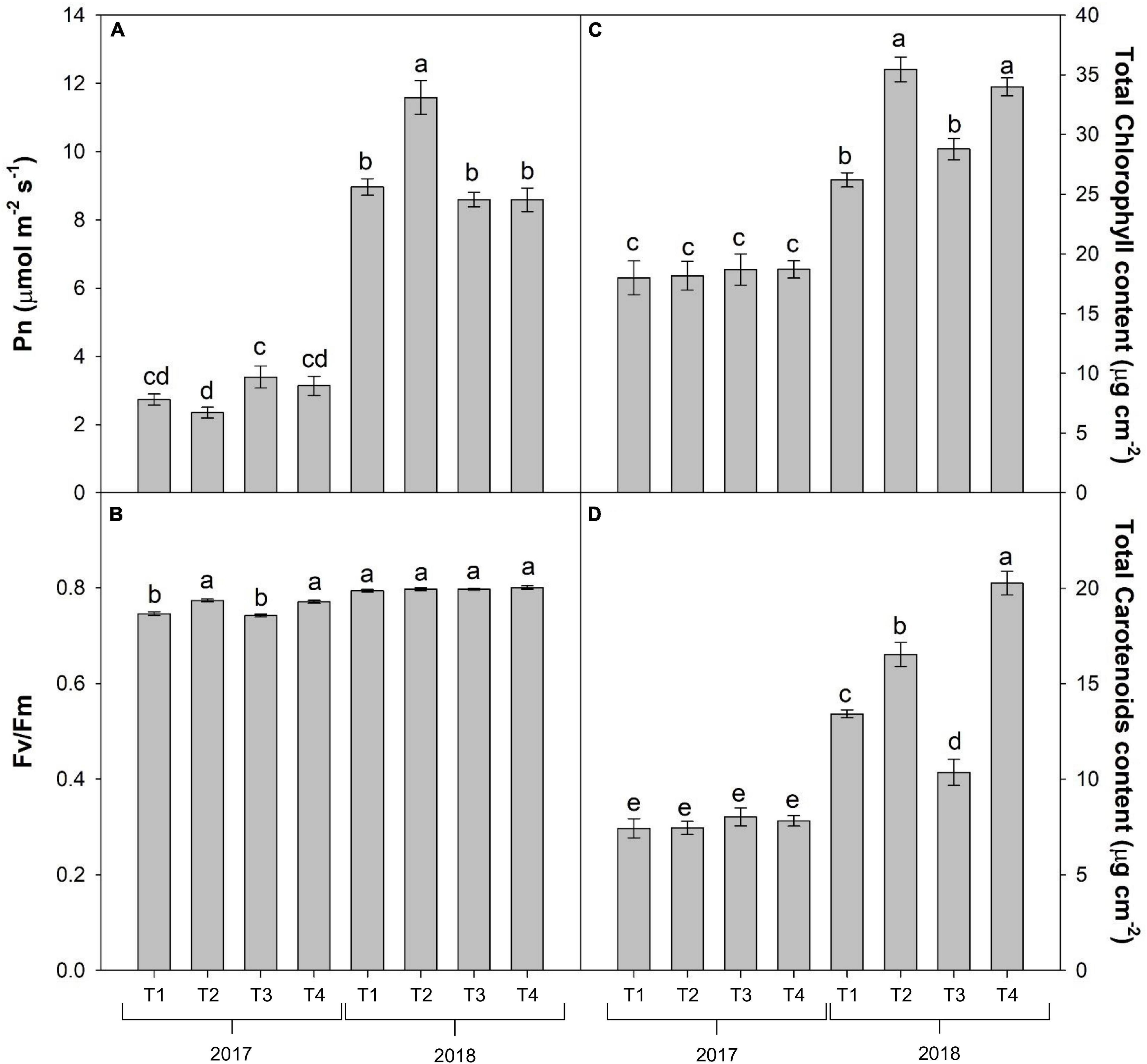
Figure 4. Combined effects of year and foliar treatments on net photosynthetic rate (Pn) (A), maximum quantum efficiency of PSII photochemistry (Fv/Fm) (B), total chlorophyll content (C) and total carotenoids content (D) of V. vinifera “Aglianico” vines (mean ± SE). Different letters indicate significant differences according to Duncan’s multiple-range test (P ≤ 0.05). [T1 – only industry water mixture of copper oxychloride; T2 – industry water mixture of copper oxychloride plus Trym®; T3 – half dose of copper oxychloride water mixture; T4 – half dose of copper oxychloride water mixture plus Trym®].
The maximum PSII photochemical efficiency (Fv/Fm) was significantly affected by the interaction Y × T, whereas the quantum yield of ΦPSII and NPQ were mainly influenced by the year, with all parameters in 2018 reaching higher values than in 2017, and by foliar treatment (Table 3). For instance, the lowest values of Fv/Fm were recorded in T1 and T3 treatments vines during the year 2017 (Figure 4B). T2 and T4 elicited significant increase (12.4 and 8.8%, respectively) in ΦPSII compared to the treatments without Trym® (T1 and T3), whereas the NPQ resulted significantly lowered in the foliar treatments containing Trym®, such as T2 and T4 (Table 3).
The total chlorophyll and carotenoid contents also increased in 2018 compared to 2017 and were affected by the Y × T interaction (Table 3 and Figures 4C,D).
Indeed, vines treated with sprays containing Cu and Trym® (T2 and T4) limitedly to 2018 also showed a significant rise in the photosynthetic pigment content compared to the other treatments.
Leaf Traits and Functional Anatomical Traits in Leaves and Fruits
As reported in Table 4, leaves subjected to the 50%Cu dose showed a significant reduction in the RWC compared to leaves treated with 100% Cu dose, whereas no significant differences were found in SLA and LDMC.
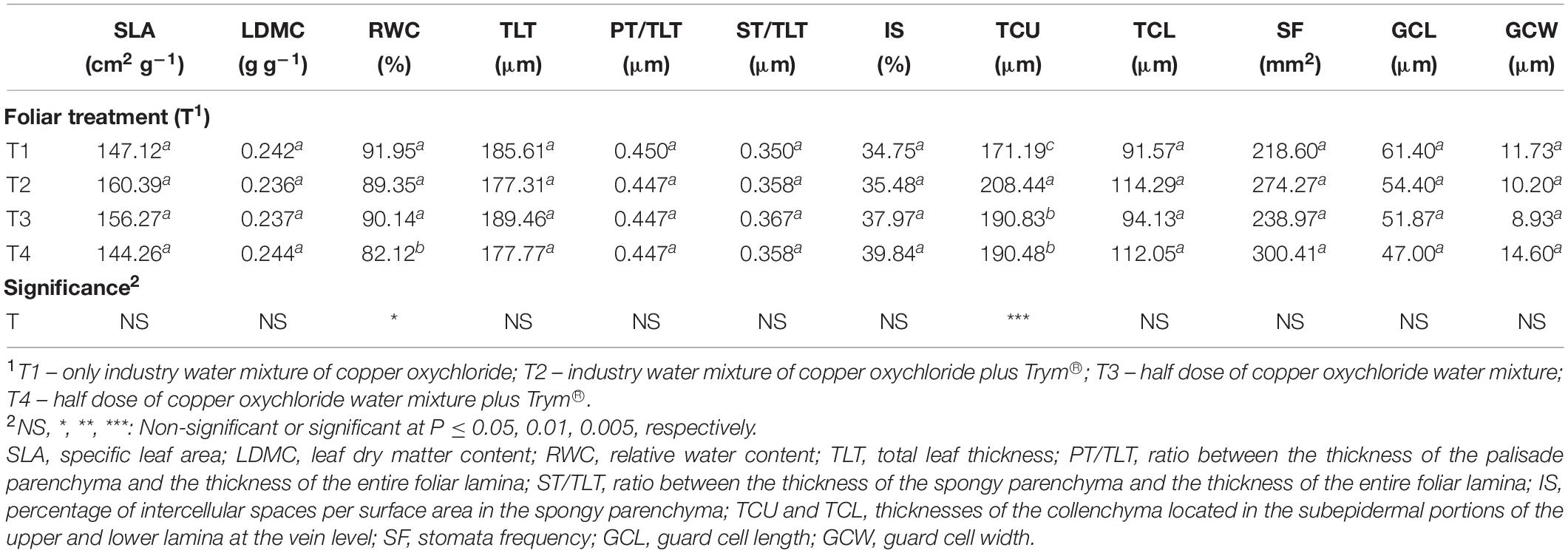
Table 4. Main effects of foliar treatments on functional anatomical traits in leaves of V. vinifera “Aglianico” vines. Different letters within column indicate significant differences according to Duncan’s multiple-range test (P ≤ 0.05).
Microscopy observations of leaves and fruits showed that there were no treatment-induced qualitative alterations in the tissue organization (Figure 4). The thickness of lamina, of palisade and spongy parenchyma tissues, as well as the spongy parenchyma density (i.e., percent of intercellular spaces) were not influenced by the foliar treatments (Table 4). Stomata traits, either frequency or size, were not significantly influenced as well (Table 4). The sole leaf anatomical parameter significantly influenced by the foliar treatments was the thickness of the collenchyma layers under the upper epidermis, in correspondence of the veins. This trait showed the lowest value in T1, significantly increased in T3 and T4 which in turn presented significantly lower values than T2 (Table 4).
Regarding the fruits, epi-fluorescence microscopy showed an intrinsic fluorescence, due to the presence of waxy substances on the surface of the exocarp, and of phenolic compounds both in vacuoles and along the membranes. The yellow-orange autofluorescence of these phenolic compounds was stronger at the subepidermal layers of cells compared to the inner parenchyma cell layers of the flesh. Indeed, the autofluorescence gradually faded moving toward the inner layers of the flesh. The intensity of autofluorescence was significantly affected by the foliar treatments. In particular, the reduction in the copper dose in T3 and T4 led to an increase in the intensity of phenolic autofluorescence especially in the cells of the sub-epidermal layers if compared to T1 and T2 (Figures 5E,F). T4 fruits showed not only the highest autofluorescence but also a thicker zone showing such an autofluorescence moving toward the inner part of the flesh (data not shown).
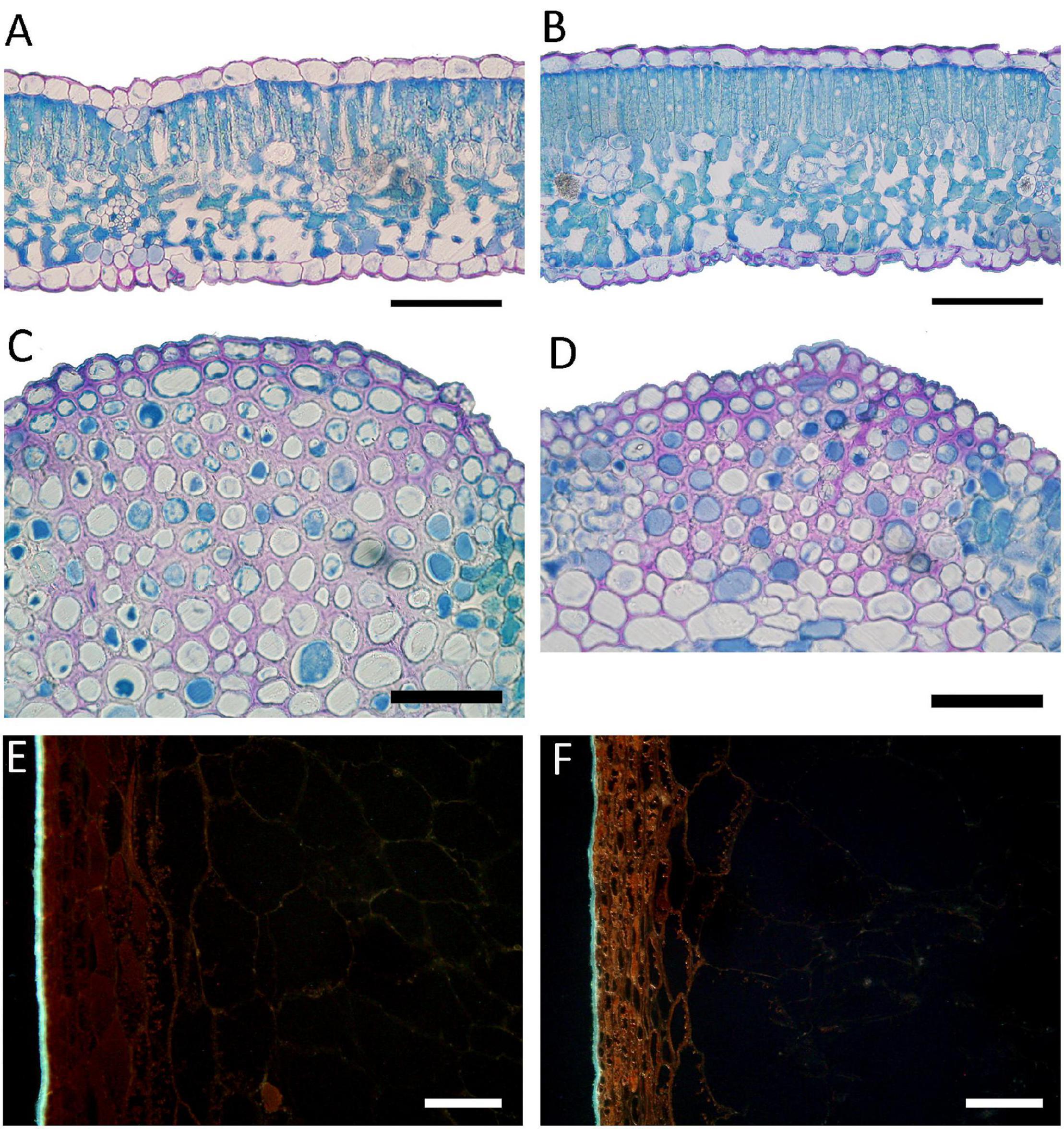
Figure 5. Light (A–D) and epi-fluorescence (E,F) microscopy views of leaf (A–D) and fruit (E,F) sections in V. vinifera “Aglianico” vines. (A,B) leaf cross section of T1 and T3; (C,D) detail of the collenchyma in T1 and T3; (E,F) fruit longitudinal section showing yellow-orange autofluorescence of phenolics in the sub-epidermal layers in T1 and T3, respectively. Bars = 100 μm in (A–D), 50 μm in (E,F). [T1 – only industry water mixture of copper oxychloride; T3 – half dose of copper oxychloride water mixture].
Leaf Mineral Composition
Leaf mineral composition in the first year of trial (2017) showed higher values of Na+, tartrate and citrate compared to 2018. However, in 2018 PO43– increased, while no differences were assessed for NH4+ between the two years (Table 5). The foliar spray treatment as main factor induced no significant differences for Na+, NH4+, PO43– and tartrate, whereas T2 and T4 affected positively the citrate concentration in the leaves compared to the two control treatments without Trym®, such as T1 and T3 (Table 5).
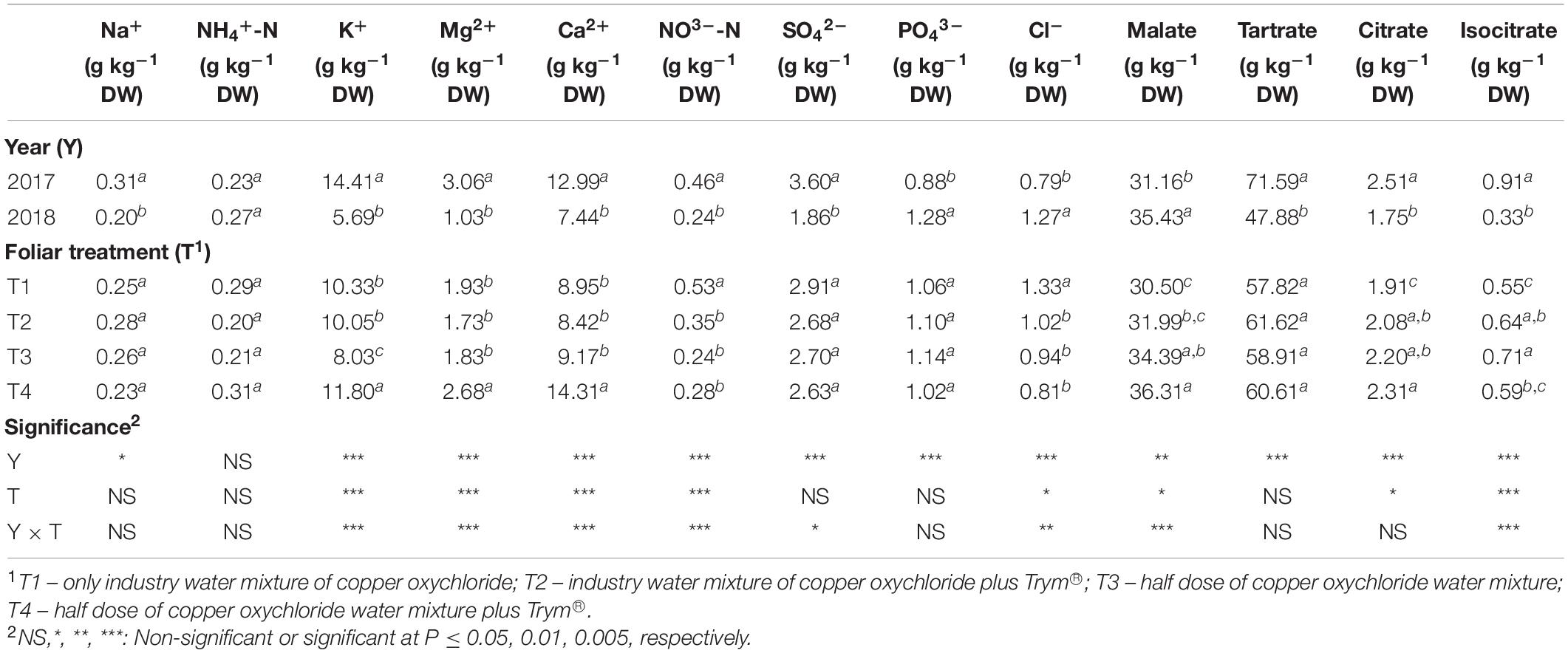
Table 5. Main effects of year and foliar treatments on leaf mineral composition of V. vinifera “Aglianico” vines. Mean values and significance of interactions are shown. Different letters within column indicate significant differences according to Duncan’s multiple-range test (P ≤ 0.05).
The Y × T interaction influenced significantly K+, Mg2+, and Ca2+ which increased in the leaves of vines treated with the foliar spray containing Cu at 50% and Trym® in the year 2018 (Figures 5A–C). On the other hand, the nitrate content resulted highest in T1 treatment in 2018 and reached the lowest values in all the other treatments in the same year, whereas it was increased in both the treatments containing the Trym® (T2 and T4) compared to related controls (T1 and T3) in the year 2017 (Figure 5D). The concentration of sulfate was generally decreased in all the treatments of 2018 compared to 2017, with the lowest level in T3 (Figure 5E). Finally, also the concentration of malate resulted significantly increased in the treatments T3 and T4 of 2018, while no differences were detected among all the other treatments (Figure 5F).
Fruit Quality Traits and Mineral Composition
Considering the year as main factor, 2018 showed a decrease in the SSC and an increase in titratable acidity (TA), while the pH was not influenced (Table 6). The TA was the only parameter significantly influenced by the dose of copper, showing a decrease in response to 50% Cu. Furthermore, TA in 2017 showed no significant differences among the four treatments. In 2018, however, it decreased in T4 (Figure 6). Trym® application, caused a reduction in the SSC compared to T1, while the other parameters were not affected.
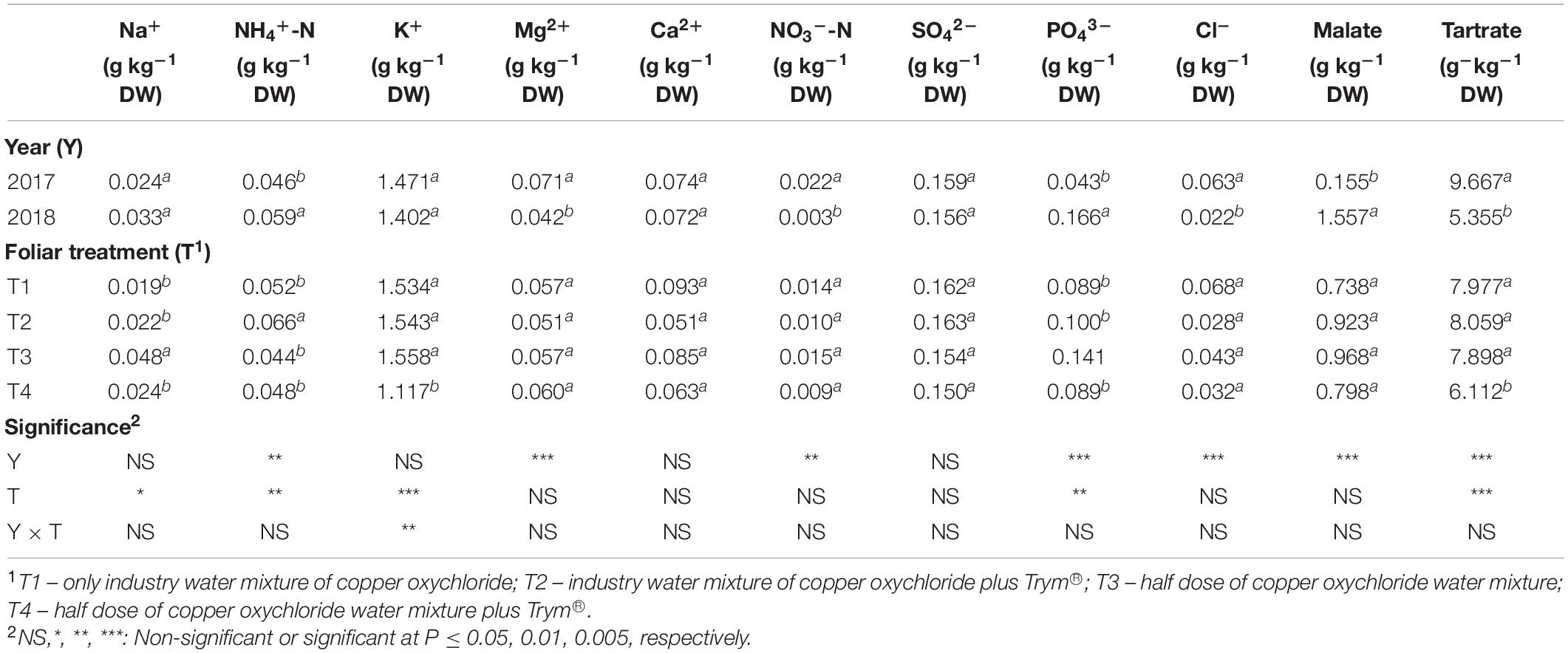
Table 6. Main effects of year and foliar treatments on fruit mineral composition of V. vinifera “Aglianico” vines. Mean values and significance of interactions are shown. Different letters within column indicate significant differences according to Duncan’s multiple-range test (P ≤ 0.05).
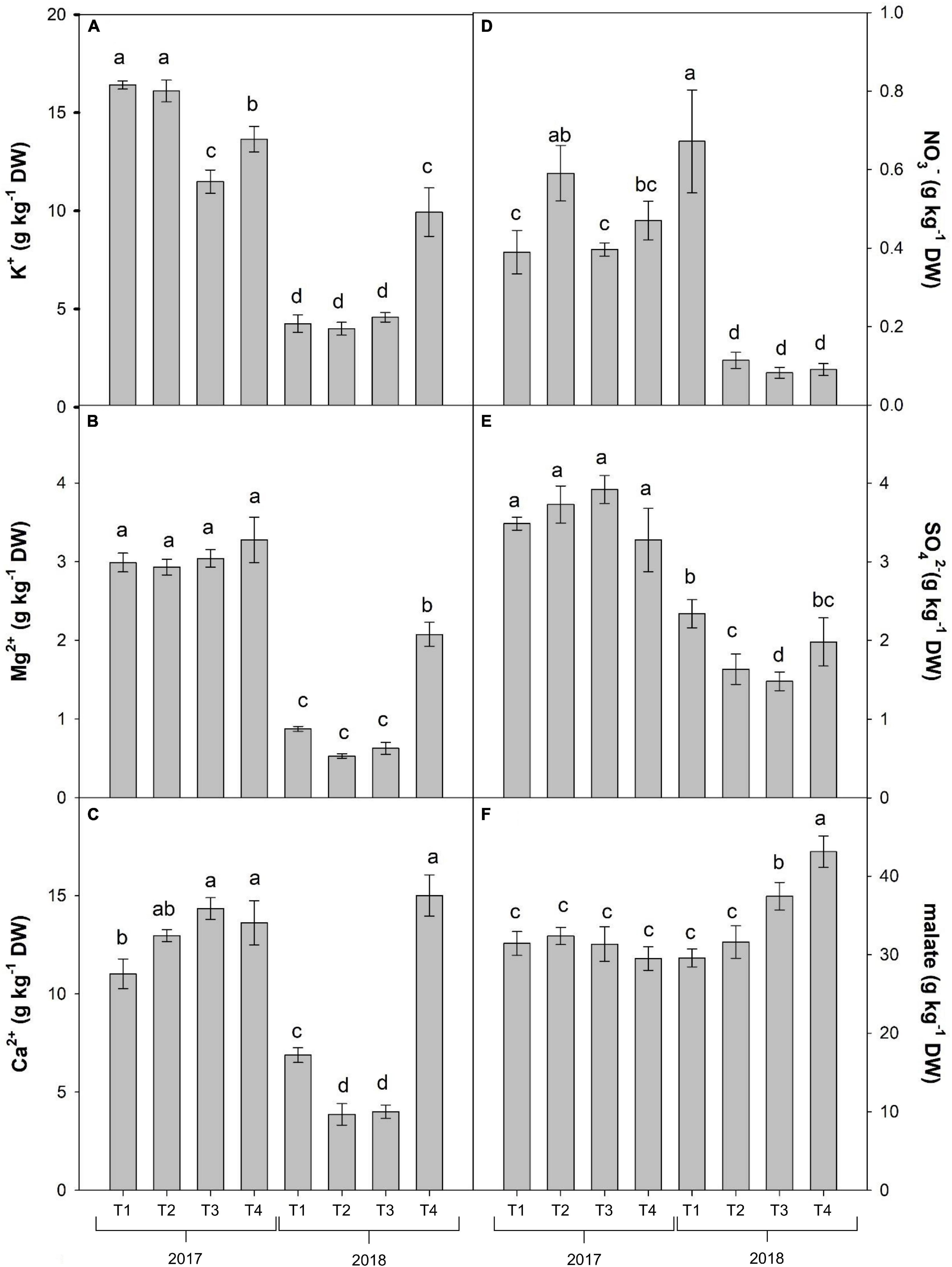
Figure 6. Combined effects of year and foliar treatments on leaf content of potassium (A), magnesium (B), calcium (C), nitrate (D), sulfate (E), and malate (F) of V. vinifera “Aglianico” vines (mean ± SE). Different letters indicate significant differences according to Duncan’s multiple-range test (P ≤ 0.05). [T1 – only industry water mixture of copper oxychloride; T2 – industry water mixture of copper oxychloride plus Trym®; T3 – half dose of copper oxychloride water mixture; T4 – half dose of copper oxychloride water mixture plus Trym®].
Except for the concentration of potassium, the mineral composition of berries was affected as main factors by year and by foliar treatment (Table 6). In particular, in the second year of measurements (2018) an increase in the content of NH4+, PO43–, malate, and a decrease in Mg2+, NO3–, Cl–, tartrate, was recorded compared to the first year (2017) (Table 6). Concerning the foliar treatment as the main factor, the reduction of the copper content to 50% led to an increase in Na+ and PO43– when applied alone (T3 vs T1) and to a decrease in NH4+ and tartrate content when applied with Trym® (T4 vs T2) (Table 6).
Discussion
In this study, we demonstrated that the reduction of Cu application coupled with the distribution of a tropical plant extract through foliar spraying in grapevine can be a promising strategy to support plant protection respectfully of chemical control restrictions. The application of plant-based biostimulants, in grapevine is increasing and has been proven to influence many plant processes, including nutrient absorption, photosynthesis, mechanisms of defense against biotic and abiotic stresses (Gutiérrez-Gamboa et al., 2019). Indeed, the effects are strictly dependent on chemical composition, dose, time of distribution, and cultivar, often leading to distinct plant responses such as increasing or decreasing vegetative growth and photosynthesis (Salvi et al., 2016; Gutiérrez-Gamboa et al., 2019). In the case of Aglianico, the application of the halved dose of Cu together with the tropical plant extract allowed maintaining a reduced vegetative vigor, only partly due to decreased photosynthetic levels, at least compared to the treatment with full Cu dose plus the Trym®. The reduction in vegetative growth, in agreement with other studies, is a desirable trait in Aglianico that is a cultivar characterized by high vigor thus being high-demanding in terms of canopy management (Bavaresco et al., 2005). Interestingly, the reduced dose of Cu determined a significant reduction in the fruit set that was partially recovered by the application of the Trym®. This suggests that the tropical plant extracts could have helped a different allocation of resources, still high due to high photosynthetic efficiency.
It is noteworthy that the application of the different treatments has induced a diverse regulation of plant photosynthetic capacity, determining an adaptation of grapevines to the new environmental conditions. The improvement in photosynthetic performance was evident only in the second year of the treatments. In the first year, the lower levels of gas exchanges (Pn and gs and photosynthetic pigments were not accompanied by any decrease in photochemistry when the tropical plant extract was applied. Conversely, in the second year, the addition of Trym® significantly improved leaf gas exchanges and photochemistry as well as the synthesis of photosynthetic pigments. The differential responses observed in the two years, may be ascribed to the different climatic conditions especially regarding the water deficit intensity and duration throughout the growing season. Indeed, the improvement in all the morphological and physiological traits recorded in the second year of the trial, especially with the application of Trym®, might be due to the higher cumulated rainfall compared to the previous year. Another explanation may be that many critical physiological processes, such as nutrient uptake by soil and photosynthetic carbon assimilation, need more time to be influenced, and that the plant extract exerts a long-lasting effect on the photosynthetic capacity. Even if plants did not show stress signals, as indicated by the comparable Fv/Fm ratio, the prolonged application of Trym® on leaves for two consecutive years might have promoted the PSII electron transport activity, allocating the reductive power of the electron transport chain in carbon fixation, rather than in non-radiative dissipation mechanisms. This assumption is confirmed by the higher Pn rates and lower NPQ index in plants treated with Trym® and both levels of copper oxychloride compared to plants without the Trym® application, during the second year of treatments. The positive effects exerted by the tropical plant extract on gas exchanges and photochemistry in the second year of treatment may also be ascribed to the enhancement of some leaf functional traits. More specifically, the increase in total leaf area, as well as the high content of chlorophylls and carotenoids found in plants treated with vegetal-based plant biostimulant, may have helped the light interception and conversion by the photosynthetic apparatus, thus favoring the photosynthesis. A direct presumed mechanism in the rise of photosynthesis could be attributed to the increase in N assimilation in crops, due to the positive effects of signaling molecules (amino acids and soluble peptides present in the product) on the production of C skeletons and energy supply, which are needed for amino acid biosynthesis (Colla et al., 2015, 2017). Moreover, another putative indirect mechanism behind the biostimulant activity (especially in T4) of tropical plant extract is the modulation of the root system architecture (e.g., length, number, density, and expansion of lateral roots) thus improving nutrient uptake (higher K, Ca, and Mg concentration in leaf tissue). Particularly, Mg is an essential element for plant growth and/or development and is involved in a wide range of biochemical and physiological activities, including pigment synthesis and photosynthetic carbon fixation (Gransee and Führs, 2013; Kumar et al., 2015). It is well known that the addition of copper-based foliar fertilizer increases photosynthetic pigment content compared to untreated samples by improving of soil chemical properties (Zhu et al., 2012). In our case, the treatment obtained mixing a half dose of copper oxychloride with Trym® did not produce a stimulatory effect on photosynthetic performance, despite the increased pigment content, probably because the chosen dose is not suitable for grapevine plants at veraison stage. However, the gas exchanges and photochemical behavior are also dependent on changes in plant structural traits induced by the different treatments. Hence, it cannot be excluded that the harmonization of structural and functional plant traits at the whole plant level has determined the overall grapevine physiological response.
The lack of any changes in the mesophyll and stomata traits related to the control of water conductivity and gas-exchange control, suggests that the treatments did not induce permanent structural changes in leaves, but adaptation relies more on short-term physiological adjustment.
Furthermore, the application of the tropical plant extracts, although slightly reducing yield compared to the normal farm practice, allowed reducing the SSC in grapes while maintaining a satisfying level of titratable acidity and also acting on berry size, content of some minerals and histological traits. In grapevine, it has been reported that foliar application of seaweed extracts induced a raise in uptake of cations, such as potassium and calcium that turned in an increased vegetative growth (Mancuso et al., 2006), In the present experiment, despite increased leaf concentrations of potassium, magnesium and calcium were observed when Trym® was added to the halved Cu dose, our data indicate a reduction in average shoot length whenever the tropical plant extract was applied independently of the copper dose. A higher level of magnesium in leaves has been suggested to counteract the incidence of bunch stem necrosis, that is a well-known physiological disorder in grapevine, related to magnesium deficiency (Bondada and Keller, 2012). As regards histological traits, the half dose of Cu together with the tropical plant extract lead to an increased autofluorescence of phenolics in the subepidermal layers and also an increase in the thickness of the flesh containing such phenolics, likely indicating an augmented content in phenolics in berries. This suggests a redirection of plant resources toward the production of secondary metabolites, whose accumulation is a typical strategy adopted by plants to improve the physiological defenses against abiotic and biotic stresses. Indeed, phenolic compounds are produced through the phenylpropanoid pathway which is soon activated to mediate plant interaction with abiotic and biotic factors and is considered the key of the robustness of gymnosperms and angiosperms to cope with stresses (Vogt, 2010). The biostimulatory action of the tropical plant extract on the synthesis and accumulation of antioxidant molecules (phenolic compounds) was likely related to the activation of secondary metabolism, in particular the increase in gene expression of the phenylalanine (tyrosine) ammonia-lyase enzyme, involved in the phenylpropanoid pathway (Schiavon et al., 2010; Ertani et al., 2011). Phenolics are generally accumulated in various plant tissues, especially in the subepidermal layers of organs, in order to protect them by predators and pathogens, being non-lethal feeding deterrents. Their localization at the periphery of plant organs enhances their role in chemical protection given that any injury at the tissue surface would cause the prompt release of the phenolics stored in the cells, thus likely activating inducible defenses (Franceschi et al., 1998; Graham et al., 2004). In the current experiment, the distribution of the tropical plant extract would likely have not only a role in berry defense, but is also a positive trait for the achievement of the phenolic maturity. Indeed, the optimal grape maturity is cultivar specific and defined by a specific combination of three main factors: i) technological maturity (i.e., sugar, acids or their ratio); ii) phenolic maturity (i.e., quantity and quality of all tannins and pigments); iii) aromatic ripeness (i.e., typical olfactory features reached without appearance of untypical aging or excessive veggie-green aromas). The decoupling between the above three factors is strongly aggravated under a global warming scenario (Palliotti et al., 2014). Higher temperatures increase the speed of sugar accumulation, hasten acid degradation, alter flavor compounds (Coombe and Iland, 2004; Lund and Bohlmann, 2006; Conde et al., 2007), and affect the synthesis/degradation of certain compounds as polyphenols and anthocyanins (Bergqvist et al., 2001; Spayd et al., 2002; Mori et al., 2007; Teixeira et al., 2013; Zarrouk et al., 2016). In the Campania region, a shifting of the suitable thermal areas for the Aglianico grapevine is expected (Bonfante et al., 2018), resulting in inadequate growing season temperatures, and then immature berries for winemaking. For this reason the potential beneficial effect of fitostimulants on nutritional use efficiency, yield and berry quality traits has recently gained raising interest in grapevine cultivation, despite the limitations related to the yearly different climatic conditions in open-field cultivation systems (Basile et al., 2020 and literature therein). In our study case, the plant extract-induced increase in phenolics’ content, coupled with the decrease in soluble sugars in berries, would help counteracting the decoupling between the technological and phenolic maturity.
This is in agreement with other studies reporting the distribution of biostimulants to delay the sugar accumulation (technological maturity), while favoring the achievement of phenolic maturity at harvest as a strategy to control sugar and phenolic accumulation during the ripening process (Salvi et al., 2016). Indeed, the use of aminoacid-based biostimulants is a widespread practice also in other crops as a way to improve the content of phenolics like flavonoids with ROS scavenger activity (Kocira, 2019). The increase in phenolics is also influenced by many other factors, especially drought and nutritional stresses: for example, the reduced levels of nitrogen found in the leaves compared to the control treatment would also have enhanced the accumulation of secondary metabolites (Downey et al., 2006; Król et al., 2014). On the contrary, the application of the plant extract did not reduce nitrogen concentration that is important for adequate fermentation (Bisson and Butzke, 2000). The reduction in Cu distribution likely induced the activation of a plant response also linked with the improvement of mechanical defenses at the leaf level, in particular based on the thickening of the collenchyma at the upper side of veins. Such a thickening was also stimulated by the application of the Trym®. The increased thickness of collenchyma layers and/or the thickening of the cell walls has been reported in grapevine as a leaf response to stress and is considered a strategy to protect the leaves not only from mechanical injuries, but also from fungal invasion (Nicole et al., 1992; Rhimi et al., 2016). The thickening of subepidermal layers of fruits also goes in this direction: subepidermal layers of cells of berries are characterized by elongated cells, parallel to the skin, and whose size and cell wall thickness are respectively much smaller and thicker compared to the cells of the inner layers of the flesh. Therefore, a thicker layer of subepidermal cells indicates a higher resistance of fruits to mechanical injury and pathogen attack (De Micco and Aronne, 2012). In the vines treated with half Cu dose plus Trym®, the occurrence of phenolics at the periphery of berries would also protect them from excessive solar radiation, which could be consequent to the reduced total leaf area, thus limiting radiation-induced oxidative damage by protecting the membranes (Lattanzio et al., 2008).
In conclusion, the foliar application of the tropical plant extract induced a differential response depending on the environmental factors and on the oxycloride copper dose distributed, with promising implications on Aglianico vegetative growth regulation, on improvement in leaf mechanical and berry antioxidant defenses, as well as on berry quality traits.
The applied multidisciplinary approach proved to be useful to achieve a comprehensive understanding of the vine behavior in the continuum soil-plant-atmosphere, thus providing information as valuable inputs to manage terroirs in the sight of climate change and chemical control restrictions.
Data Availability Statement
The raw data supporting the conclusions of this article will be made available by the authors, without undue reservation.
Author Contributions
All authors listed have made a substantial contribution to the work. CC, YR, AE, and VDM designed the study. CC, RC, ChA, FP, EV, AE, AB, and VDM contributed to fieldwork. CC, CA, RC, SDF, EV, AE, and VDM contributed to the laboratory analyses. CC, FP, SDF, and VDM performed statistical analyses. CC, CA, YR, AB, and VDM contributed to the interpretation of results. CC, CA, YR, and VDM wrote the main part of the manuscript. All authors contributed to specific parts of the text, supervised the final draft, and approved the final version of the manuscript.
Conflict of Interest
The authors declare that the research was conducted in the absence of any commercial or financial relationships that could be construed as a potential conflict of interest.
Acknowledgments
We wish to thank the Azienda Agricola Fonzone Caccese for logistic support. We also thank Antonio Pannico, Pasquale Scognamiglio, and Simone Iannella for technical support during fieldwork and laboratory activities. Giovanna Aronne and Stefania De Pascale for sharing the laboratory instruments and materials. Finally, we are grateful to Radicirpine di Canonico & Santoli for partial financial support to the field and laboratory activities.
References
Basile, B., Rouphael, Y., Colla, G., Soppelsa, S., and Andreotti, C. (2020). Appraisal of emerging crop management opportunities in fruit trees, grapevines and berry crops facilitated by the application of biostimulants. Sci. Hortic. 267:109330. doi: 10.1016/j.scienta.2020.109330
Bavaresco, L., Civardi, S., Pezzutto, S., Vezzulli, S., and Ferrari, F. (2005). Grape production, technological parameters, and stilbenic compounds as affected by lime-induced chlorosis. Vitis 44, 63–65.
Bergqvist, J., Dokoozlian, N., and Ebisuda, N. (2001). Sunlight exposure and temperature effects on berry growth and composition of cabernet sauvignon and grenache in the Central San Joaquin Valley of California. Am. J. Enol. Vitic. 52, 1–7. doi: 10.1130/spe234-p1
Bilger, W., and Björkman, O. (1990). Role of the xanthophyll cycle in photoprotection elucidated by measurements of light-induced absorbance changes, fluorescence and photosynthesis in leaves of Hedera canariensis. Photosyn. Res. 25, 173–185. doi: 10.1007/BF00033159
Bisson, L. F., and Butzke, C. E. (2000). Diagnosis and rectification of stuck and sluggish fermentations. Am. J. Enol. Vitic. 51, 168–177.
Bonfante, A., Monaco, E., Langella, G., Mercogliano, P., Bucchignani, E., Manna, P., et al. (2018). A dynamic viticultural zoning to explore the resilience of terroir concept under climate change. Sci. Total Environ. 624, 294–308. doi: 10.1016/j.scitotenv.2017.12.035
Bondada, B. K., and Keller, M. (2012). Not all shrivels are created equal—Morpho-anatomical and compositional characteristics differ among different shrivel types that develop during ripening of grape (Vitis vinifera L.) berries. Am. J. Plant Sci. 3, 879–898. doi: 10.4236/ajps.2012.37105
Brook, A., De Micco, V., Battipaglia, G., Erbaggio, A., Ludeno, G., Catapano, I., et al. (2020). A smart multiple spatial and temporal resolution system to support precision agriculture from satellite images: proof of concept on Aglianico vineyard. Remote Sens. Environ. 240:111679. doi: 10.1016/j.rse.2020.111679
Caccavello, G., Giaccone, M., Scognamiglio, P., Forlani, M., and Basile, B. (2017). Influence of intensity of post-veraison defoliation or shoot trimming on vine physiology, yield components, berry and wine composition in Aglianico grapevines. Aust. J. Grape Wine Res. 23, 226–239. doi: 10.1111/ajgw.12263
Calvo, P., Nelson, L., and Kloepper, J. W. (2014). Agricultural uses of plant biostimulants. Plant Soil 383, 3–41. doi: 10.1007/s11104-014-2131-8
Caradonia, F., Battaglia, V., Righi, L., Pascali, G., and La Torre, A. (2019). Plant biostimulant regulatory framework: prospects in europe and current situation at international level. J. Plant Growth Regul. 38, 438–448. doi: 10.1007/s00344-018-9853-4
Carillo, P., Cirillo, C., De Micco, V., Arena, C., De Pascale, S., and Rouphael Y. (2019). Morpho-anatomical, physiological and biochemical adaptive responses to saline water of Bougainvillea spectabilis Willd. trained to different canopy shapes. Agric. Water Manag. 212, 12–22. doi: 10.1016/j.agwat.2018.08.037
Chaves, M. M., Santos, T. P., Souza, C. D., Ortuño, M. F., Rodrigues, M. L., Lopes, C. M., et al. (2007). Deficit irrigation in grapevine improves water-use efficiency while controlling vigour and production quality. Ann. Appl. Biol. 150, 237–252. doi: 10.1111/j.1744-7348.2006.00123.x
Chaves, M. M., Zarrouk, O., Francisco, R., Costa, J. M., Santos, T., Regalado, T., et al. (2010). Grapevine under deficit irrigation: hints from physiological and molecular data. Ann. Bot. 105, 661–676. doi: 10.1093/aob/mcq030
Chuang, P. H., Lee, C. W., Chou, J. Y., Murugan, M., Shieh, B. J., and Chen, H. M. (2007). Anti-fungal activity of cruder extractsand essential oil of Moringa oleifera Lam. Bioresour. Technol. 98, 232–236. doi: 10.1016/j.biortech.2005.11.003
Cirillo, C., De Micco, V., Arena, C., Pannico, A., De Pascale, S., and Rouphael Y. (2019). Biochemical, Physiological and Anatomical Mechanisms of Adaptation of Callistemon citrinus and Viburnum lucidum to NaCl and CaCl2 Salinization. Front. Plant Sci. 10:742. doi: 10.3389/fpls.2019.00742
Cohen, Y., Wang, W. Q., Ben-Daniel, B. H., and Ben-Daniel, Y. (2006). Extracts of Inula viscosa control downy mildew of grapes caused by Plasmopara viticola. Phytopathology 96, 417–424. doi: 10.1094/phyto-96-0417
Colla, G., Hoagland, L., Ruzzi, M., Cardarelli, M., Bonini, P., Canaguier, R., et al. (2017). Biostimulant action of protein hydrolysates: unraveling their effects on plant physiology and microbiome. Front. Plant Sci. 8:2202. doi: 10.3389/fpls.2017.02202
Colla, G., Nardi, S., Cardarelli, M., Ertani, A., Lucini, L., Canaguier, R., et al. (2015). Protein hydrolysates as biostimulants in horticulture. Sci. Hortic. 196, 28–38. doi: 10.1016/j.scienta.2015.08.037
Conde, C., Silva, P., Fontes, N., Dias, A. C. P., Tavares, R. M., Sousa, M. J., et al. (2007). Biochemical changes throughout grape berry development and fruit and wine quality. Food 1, 1–22. doi: 10.1111/j.1755-0238.1996.tb00087.x
Coombe, B., and Iland, P. (2004). “Grape berry development and winegrape quality,” in Viticulture, Vol. 1, eds P. R. Dry and B. G. Coombe (Adelaide: Winetitles), 210–248.
Cornelissen, J. H. C., Lavorel, S., Garnier, E., Díaz, S., Buchmann, N., Gurvich, D. E., et al. (2003). A handbook of protocols for standardised and easy measurement of plant functional traits worldwide. Aust. J. Bot. 51, 335–380. doi: 10.1071/BT02124
Costa, M. H., Yanagi, S. N., Souza, P. J., Ribeiro, A., and Rocha, E. J. (2007). Climate change in Amazonia caused by soybean cropland expansion, as compared to caused by pastureland expansion. Geophys. Res. Lett. 34:L07706. doi: 10.1029/2007GL029271
Dagostin, S., Schärer, H.-J., Pertot, I., and Tamm, L. (2011). Are there alternative to copper for controlling grapevine downy mildew in organic viticulture? Crop Prot. 30, 776–788. doi: 10.1016/j.cropro.2011.02.031
De Micco, V., and Aronne, G. (2007). Combined histochemistry and autofluorescence for identifying lignin distribution in cell walls. Biotech. Histochem. 82, 209–216. doi: 10.1080/10520290701713981
De Micco, V., and Aronne, G. (2012). “Morpho-anatomical traits for plant adaptation to drought,” in Plant Responses to Drought Stress, ed. R. Aroca (Berlin: Springer), 37–61. doi: 10.1007/978-3-642-32653-0_2
Downey, M. O., Dokoozlian, N. K., and Krstic, M. (2006). Cultural practice and environmental impacts on the flavonoid composition of grapes and wine: a review of recent research. Am. J. Enol. Vitic. 3, 257–268.
du Jardin, P. (2012). The Science of Plant Biostimulants–A Bibliographic Analysis, Ad Hoc Study Report. Brussels: European Commission.
du Jardin, P. (2015). Plant biostimulants: definition, concept, main categories and regulation. Sci. Hortic. 196, 3–14. doi: 10.1016/j.scienta.2015.09.021
Ertani, A., Francioso, O., Tugnoli, V., Righi, V., and Nardi, S. (2011). Effect of commercial lignosulfonate-humate on Zea mays L. metabolism. J. Agric. Food Chem. 59, 11940–11948. doi: 10.1021/jf202473e
European Union [EU] (1990). Commission Regulation (EEC) No 2676/90 (REGULATION, H. A. T. 1990. Commission Regulation (EEC) No. 2676/90 Determining Community Methods for the Analysis of Wines Official Journal L 272, 3 October 1990. Brussels: European Union, 1–192.
European Union [EU] (2019). REGULATION OF THE EUROPEAN PARLIAMENT AND OF THE COUNCIL laying down rules on the making available on the market of EU fertilising products and amending Regulations (EC) No 1069/2009 and (EC) No 1107/2009 and repealing Regulation (EC) No 2003/2003. Brussels: European Union.
FAOSTAT (2020). Available online at: http://www.fao.org/faostat (accessed June 20, 2020).
Feder, N. E. D., and O’Brien, T. P. (1968). Plant microtechnique: some principles and new methods. Am. J. Bot. 55, 123–142. doi: 10.2307/2440500
Ferrara, G., and Brunetti, G. (2010). Effects of the times of application of a soil humic acid on berry quality of table grape (Vitis vinifera L.) cv Italia. Span J. Agric. Res. 8, 1–6. doi: 10.5424/1283
Franceschi, V. R., Krekling, T., Berryman, A. A., and Christiansen, E. (1998). Specialized phloem parenchyma cells in Norway spruce (Pinaceae) bark are an important site of defense reactions. Am. J. Bot. 85, 601–615. doi: 10.2307/2446529
Frioni, T., Sabbatini, P., Tombesi, S., Norrie, J., Poni, S., Gatti, M., et al. (2018). Effects of a biostimulant derived from the brown seaweed Ascophyllum nodosum on ripening dynamics and fruit quality of grapevines. Sci. Hortic. 232, 97–106. doi: 10.1016/j.scienta.2017.12.054
Fukazawa, K. (1992). Ultraviolet Microscopy. In Methods in Lignin Chemistry. Berlin: Springer, 110–121.
Genty, B., Briantais, J. M., and Baker, N. R. (1989). The relationship between the quantum yield of photosynthetic electron transport and quenching of chlorophyll fluorescence. BBA Gen. Subjects 990, 87–92. doi: 10.1016/S0304-4165(89)80016-9
Goode, J. (2012). Viticulture: fruity with a hint of drought. Nature 492, 351–352. doi: 10.1038/492351a
Graham, L. E., Kodner, R. B., Fisher, M. M., Graham, J. M., Wilcox, L. W., Hackney, J. M., et al. (2004). “Early land plant adaptations to terrestrial stress: a focus on phenolics,” in The Evolution of Plant Physiology, eds A. R. Hemsley and I. Poole (Boston, MA: Elsevier Academic Press), 155–169. doi: 10.1016/b978-012339552-8/50010-x
Gransee, A., and Führs, H. (2013). Magnesium mobility in soils as a challenge for soil and plant analysis, magnesium fertilization and root uptake under adverse growth conditions. Plant Soil 368, 5–21. doi: 10.1007/s11104-012-1567-y
Gutiérrez-Gamboa, G., Romanazzi, G., Garde-Cerdán, T., and Pérez-Álvarez, E. P. (2019). A review of the use of biostimulants in the vineyard for improved grape and wine quality: effects on prevention of grapevine diseases. J. Sci. Food Agric. 99, 1001–1009. doi: 10.1002/jsfa.9353
IPCC (2014). “2014: climate change 2014: synthesis report,” in Proceedings of the Contribution of Working Groups I. II and III to the Fifth Assessment Report of the Intergovernmental Panel on Climate Change, (Geneva: IPCC).
IUSS Working Group WRB (2015). International Soil Classification System for Naming Soils and Creating Legends for Soil Maps. World Reference Base for Soil Resources 2014, Update 2015, 106. Greenwich: IUSS Working Group WRB.
Jones, G. V., and Webb, L. B. (2010). Climate change, viticulture, and wine: challenges and opportunities. Int. J. Wine Res. 21, 103–106. doi: 10.1080/09571264.2010.530091
Kocira, S. (2019). Effect of amino acid biostimulant on the yield and nutraceutical potential of soybean. Chil. J. Agric. Res. 79, 17–25. doi: 10.4067/S0718-58392019000100017
Król, A., Amarowicz, R., and Weidner, S. (2014). Changes in the composition of phenolic compounds and antioxidant properties of grapevine roots and leaves (Vitis vinifera L.) under continuous of long-term drought stress. Acta Physiol. Plant. 36, 1491–1499. doi: 10.1007/s11738-014-1526-8
Kumar, P., Lucini, L., Rouphael, Y., Cardarelli, M., Kalunke, R. M., and Colla, G. (2015). Insight into the role of grafting and arbuscular mycorrhiza on cadmium stress tolerance in tomato. Front. Plant Sci. 6:477. doi: 10.3389/fpls.2015.00477
Lattanzio, V., Kroon, P. A., Quideau, S., and Treutter, D. (2008). Plant phenolics—secondary metabolites with diverse functions. Recent Adv. Polyphenol. Res. 1, 1–35. doi: 10.1002/9781444302400.ch1
Lereboullet, A. L., Bardsley, D., and Beltrando, G. (2013). Assessing vulnerability and framing adaptive options of two Mediterranean wine growing regions facing climate change: roussillon (France) and McLaren Vale (Australia). EchoGéo 23:13384. doi: 10.4000/echogeo.13384
Lereboullet, A. L., Beltrando, G., Bardsley, D. K., and Rouvellac, E. (2014). The viticultural system and climate change: coping with long-term trends in temperature and rainfall in Roussillon. France. Reg. Environ. Change 14, 1951–1966. doi: 10.1007/s10113-013-0446-2
Lichtenthaler, H. K. (1987). Chlorophylls and carotenoids, the pigments of photosynthetic biomembranes. Methods Enzymol. 148, 350–382. doi: 10.1016/0076-6879(87)48036-1
Lopes, L. D., Böger, B. R., Cavalli, K. F., Silveira-Júnior, J. F. D. S., Osório, D. V., de Oliveira, S., et al. (2014). Fatty acid profile, quality lipid index and bioactive compounds of flour from grape residues. Agric. Nat. Resour. 41, 225–234. doi: 10.4067/S0718-16202014000200009
Lorenz, D. H., Eichhorn, K. W., Bleiholder, H., Klose, R., Meier, U., and Weber, E. (1995). Growth stages of the grapevine: phenological growth stages of the grapevine (Vitis vinifera L. ssp. vinifera)—Codes and descriptions according to the extended BBCH scale. Aust. J. Grape Wine Res. 1, 100–103. doi: 10.1111/j.1755-0238.1995.tb00085.x
Lund, S. T., and Bohlmann, J. (2006). The molecular basis for wine grape quality-a volatile subject. Science 311, 804–805. doi: 10.1126/science.1118962
Mancuso, S., Azzarello, E., Mugnai, S., and Briand, X. (2006). Marine bioactive substances (IPA extract) improve foliar ion uptake and water stress tolerance in potted Vitis vinifera plants. Adv. Hortic. Sci. 20, 156–161. doi: 10.1400/53262
Martínez-Gil, A. M., Angenieux, M., Pardo-García, A. I., Alonso, G. L., Ojeda, H., and Salinas, M. R. (2013). Glycosidic aroma precursors of Syrah and Chardonnay grapes after an oak extract application to the grapevines. Food Chem. 138, 956–965. doi: 10.1016/j.foodchem.2012.11.032
Martínez-Gil, A. M., Garde-Cerdán, T., Zalacain, A., Pardo-García, A. I., and Salinas, M. R. (2012). Applications of an oak extract on Petit Verdot grapevines. Influence on grape and wine volatile compounds. Food Chem. 132, 1836–1845. doi: 10.1016/j.foodchem.2011.12.016
Medrano, H., Escalona, J. M., Cifre, J., Bota, J., and Flexas, J. (2003). A ten-year study on the physiology of two Spanish grapevine cultivars under field conditions: effects of water availability from leaf photosynthesis to grape yield and quality. Funct. Plant Biol. 30, 607–619. doi: 10.1071/FP02110
Mori, K., Goto-Yamamoto, N., Kitayama, M., and Hashizume, K. (2007). Loss of anthocyanins in red-wine grape under high temperature. J. Exp. Bot. 58, 1935–1945. doi: 10.1093/jxb/erm055
Nicole, M. R., Geiger, J. P., and Nandris, D. (1992). “Defense of angiosperm roots against fungal invasion,” in Defense Mechanisms of Woody Plants Against Fungi, eds R. A. Blanchette and A. R. Biggs (Berlin: Springer), 181–206. doi: 10.1007/978-3-662-01642-8_10
Palliotti, A., Tombesi, S., Silvestroni, O., Lanari, V., Gatti, M., and Poni, S. (2014). Changes in vineyard establishment and canopy management urged by earlier climate-related grape ripening: a review. Sci. Hortic. 178, 43–54. doi: 10.1016/j.scienta.2014.07.039
Parrado, J., Escudero-Gilete, M. L., Friaza, V., García-Martínez, A., González-Miret, M. L., Bautista, J. D., et al. (2007). Enzymatic vegetable extract with bio-active components: influence of fertiliser on the colour and anthocyanins of red grapes. J. Sci. Food Agric. 87, 2310–2318. doi: 10.1002/jsfa.2989
Poni, S., Gatti, M., Palliotti, A., Dai, Z., Duchêne, E., Truong, T. T., et al. (2018). Grapevine quality: a multiple choice issue. Sci. Hortic. 234, 445–462. doi: 10.1016/j.scienta.2017.12.035
Popescu, G. C., and Popescu, M. (2018). Yield, berry quality and physiological response of grapevine to foliar humic acid application. Bragantia 77, 273–282. doi: 10.1590/1678-4499.2017030
Rhimi, N., Ben Ahmed, C., Elloumi, N., Athar, H. R., Noreen, S., Ashraf, M., et al. (2016). Morpho-anatomical and physiological changes in grapevine leaves exposed to amospheric fluoride and sulfur dioxide pollution. Appl. Ecol. Environ. Res. 14, 77–89. doi: 10.15666/aeer/1405_077089
Rogiers, S. Y., and Clarke, S. J. (2013). Nocturnal and daytime stomatal conductance respond to root-zone temperature in ‘Shiraz’ grapevines. Ann. Bot. 111, 433–444. doi: 10.1093/aob/mcs298
Rouphael, Y., and Colla, G. (2018). Synergistic biostimulatory action: designing the next generation of plant biostimulants for sustainable agriculture. Front. Plant Sci. 9:1655. doi: 10.3389/fpls.2018.01655
Rouphael, Y., and Colla, G. (2020). Biostimulants in agriculture. Front. Plant Sci. 11:40. doi: 10.3389/fpls.2020.00040
Rusjan, D., Strlič, M., Pucko, D., and Korošec-Koruza, Z. (2007). Copper accumulation regarding the soil characteristics in Sub-Mediterranean vineyards of Slovenia. Geoderma 141, 111–118. doi: 10.1016/j.geoderma.2007.05.007
Ruzin, S. E. (1999). Plant Microtechnique and Microscopy, Vol. 198. New York, NY: Oxford University Press.
Salvi, L., Cataldo, E., Secco, S., and Mattii, G. B. (2016). Use of natural biostimulants to improve the quality of grapevine production: first results. Acta Hortic. 1148, 77–84. doi: 10.17660/ActaHortic.2016.1148.9
Sánchez-Gómez, R., Garde-Cerdán, T., Zalacain, A., Garcia, R., and Cabrita, M. J. (2016a). Vine-shoot waste aqueous extract applied as foliar fertilizer to grapevines: effect on amino acids and fermentative volatile content. Food Chem. 197, 132–140. doi: 10.1016/j.foodchem.2015.10.034
Sánchez-Gómez, R., Zalacain, A., Pardo, F., Alonso, G. L., and Salinas, M. R. (2016b). An innovative use of vine-shoots residues and their “feedback” effect on wine quality. Innov. Food Sci. Emerg. Technol. 37, 18–26. doi: 10.1016/j.ifset.2016.07.021
Schiavon, M., Pizzeghello, D., Muscolo, A., Vaccaro, S., Francioso, O., and Nardi, S. (2010). High molecular size humic substances enhance phenylpropanoid metabolism in maize (Zea mays L.). J. Chem. Ecol. 36, 662–669. doi: 10.1007/s10886-010-9790-6
Spayd, S. E., Tarara, J. M., Mee, D. L., and Ferguson, J. C. (2002). Separation of sunlight and temperature effects on the composition of Vitis vinifera cv. Merlot berries. Am. J. Enol. Vitic. 53, 171–182.
Teixeira, A., Eiras-Dias, J., Castellarin, S. D., and Gerós, H. (2013). Berry phenolics of grapevine under challenging environments. Int. J. Mol. Sci. 14, 18711–18739. doi: 10.3390/ijms140918711
Valverde, P., de Carvalho, M., Serralheiro, R., Maia, R., Ramos, V., and Oliveira, B. (2015). Climate change impacts on rainfed agriculture in the Guadiana river basin (Portugal). Agric. Water Manag. 150, 35–45. doi: 10.1016/j.agwat.2014.11.008
Webb, L. B., Watterson, I., Bhend, J., Whetton, P. H., and Barlow, E. W. R. (2013). Global climate analogues for winegrowing regions in future periods: projections of temperature and precipitation. Aust. J. Grape Wine Res. 19, 331–341. doi: 10.1111/ajgw.12045
Yakhin, O. I., Lubyanov, A. A., Yakhin, I. A., and Brown, P. H. (2017). Biostimulants in plant science: a global perspective. Front. Plant Sci. 7:2049. doi: 10.3389/fpls.2016.02049
Zarrouk, O., Brunetti, C., Egipto, R., Pinheiro, C., Genebra, T., Gori, A., et al. (2016). Grape ripening is regulated by deficit irrigation/elevated temperatures according to cluster position in the canopy. Front. Plant Sci. 7:1640. doi: 10.3389/fpls.2016.01640
Zhifeng, G., and Chengguang, F. (1994). Determination of organic acids and inorganic anions by single-column ion chromatography. J. Instrum. Anal. 5, 32–36.
Keywords: plant-based biostimulant, copper, eco-physiology, functional anatomical traits, soil-plant-atmosphere continuum, Vitis vinifera L.
Citation: Cirillo C, Arena C, Rouphael Y, Caputo R, Amitrano C, Petracca F, De Francesco S, Vitale E, Erbaggio A, Bonfante A and De Micco V (2021) Counteracting the Negative Effects of Copper Limitations Through the Biostimulatory Action of a Tropical Plant Extract in Grapevine Under Pedo-Climatic Constraints. Front. Environ. Sci. 9:587550. doi: 10.3389/fenvs.2021.587550
Received: 26 July 2020; Accepted: 26 February 2021;
Published: 25 March 2021.
Edited by:
Moritz Bigalke, University of Bern, SwitzerlandReviewed by:
Sahap Kaan Kurtural, University of California, Davis, United StatesMarkus Steffens, Research Institute of Organic Agriculture (FiBL), Switzerland
Copyright © 2021 Cirillo, Arena, Rouphael, Caputo, Amitrano, Petracca, De Francesco, Vitale, Erbaggio, Bonfante and De Micco. This is an open-access article distributed under the terms of the Creative Commons Attribution License (CC BY). The use, distribution or reproduction in other forums is permitted, provided the original author(s) and the copyright owner(s) are credited and that the original publication in this journal is cited, in accordance with accepted academic practice. No use, distribution or reproduction is permitted which does not comply with these terms.
*Correspondence: Antonello Bonfante, YW50b25lbGxvLmJvbmZhbnRlQGNuci5pdA==; YW50b25lbGxvLmJvbmZhbnRlQGdtYWlsLmNvbQ==