- 1Institute of Grassland Science, Key Laboratory of Vegetation Ecology, Northeast Normal University, Changchun, China
- 2Peatland Ecology Research Group (PERG), Centre for Northern Studies, Department of Plant Sciences, Université Laval, Québec, QC, Canada
- 3Nicholas School of the Environment, Duke University Wetland Center, Durham, NC, United States
- 4School of Environment/Key Laboratory of Vegetation Ecology, Northeast Normal University, Changchun, China
Litter decomposition is a fundamental path for nutrient cycling in a natural ecosystem. However, it remains unclear how species diversity, including richness and evenness, affects the decomposition dynamics in the context of grassland degradation. Using a litter bag technique, we investigated the litter-mixing effects of two coexisting dominant species (Leymus chinensis Lc and Phragmites australis Pa), as monocultures and mixtures with evenness (Lc:Pa) from M1 (30:70%), M2 (50:50%), and M3 (70:30%), on decomposition processes over time (60 and 365 days). The litter bags were placed on the soil surface along a degradation gradient [near pristine (NP), lightly degraded (LD), and highly degraded (HD)]. We found that 1) mass loss in mixture compositions was significantly and positively correlated with initial nitrogen (N) and cellulose contents; 2) litter mixing (richness and evenness) influenced decomposition dynamics individually and in interaction with the incubation days and the degradation gradients; 3) in a general linear model (GLM), nonadditive antagonistic effects were more prominent than additive or neutral effects in final litter and nutrients except for carbon (C); and 4) in nutrients (C, N, lignin) and C/N ratio, additive effects shifted to nonadditive with incubation time. We speculated that the occurrence of nonadditive positive or negative effects varied with litter and nutrients mass remaining in each degraded gradient under the mechanism of initial litter quality of monoculture species, soil properties of experimental sites, and incubation time. Our study has important implications for grassland improvement and protection by considering species biodiversity richness, as well as species evenness.
Introduction
Grasslands cover approximately 40% of earth’s land surface (Huang et al., 2012) and are of critical importance to the maintenance of biogeochemical cycles in grassland ecosystems (Rumpel et al., 2015). However, most grasslands now suffer from unprecedented changes (i.e., degradation or desertification) in biodiversity, ecosystem functions, and structure across the globe (Maxwell et al., 2016). In grassland, litter decomposition is a pivotal process that controls the balances of nutrient cycling and energy flow (Zeng et al., 2018). Like other ecosystems with higher plant diversity, the litter on grassland floor naturally decomposes together (Mao and Zeng, 2012). Usually, biodiversity in an area is dependent on two dimensions, species richness (number of plant species) and evenness (relative abundance of plant species) (Munoz et al., 2018). Nonetheless, previous studies have focused on the contribution of species richness (Ball et al., 2008; Lecerf et al., 2011; Wu et al., 2013; Mao et al., 2015), and the contribution of species evenness in the decomposition process remains understudied.
Litter decomposition is a process in which 50–99% of the above-ground biomass becomes part of the detritus food chain and decompose into nutrients (Gessner et al., 2010; Jacobson et al., 2015). Plant litter decay is controlled by abiotic (prominently soil moisture, temperature, and soil C or N contents) and biotic (species diversity and composition, litter quality, and decomposers community) factors (Meier and Bowman, 2008; Gessner et al., 2010; García-Palacios et al., 2016). For instance, favorable humidity and temperature can enhance litter decomposition by reducing moisture and thermal limitations on the activities of decomposers, i.e., bacteria and fungi (Liski et al., 2003; Smith et al., 2010). Abiotic drivers often influence decomposition at a larger scale, while the impacts of biotic factors become more noticeable as the scale decreases (Zhang et al., 2013). Biotic factors, such as the quantity and quality of plant species, regulate the mass dynamics within a given climatic condition (Austin et al., 2014). In many grasslands, a litter with lower carbon to nitrogen (C/N) ratios tends to have an accelerated decomposition process, as N contents in plant tissues can improve litter decay (Penner and Frank, 2018). On the other hand, recalcitrant C and lignin in plant litter hinders the decay process (Gessner et al., 2010). Some studies showed that plant diversity impacted litter decomposition through changing microclimatic conditions, rhizosphere processes, and complimentary nutrient use (Hättenschwiler and Gasser, 2005; Santonja et al., 2018; Yang et al., 2019). The interactions between litter quality and ecological processes together explain approximately 70% of global litter decay (García-Palacios et al., 2013).
Litter mixing effects are usually calculated as the deviation of observed decomposition rates of mixed litter from the predicted values based on the arithmetic means of individual species decomposing alone (Liu et al., 2007). However, it has been pointed out that the decaying of plant species in the mixture is difficult to predict by a simple arithmetic mean of species decomposing in isolation (Lecerf et al., 2011; Zhang et al., 2019). The reasons of relative unpredictability may include variations in climatic conditions, experimental duration, and selection of litter component (Lecerf et al., 2007; Srivastava et al., 2009; Lecerf et al., 2011). Initial nutrient contents (litter quality) of the component species are the critical aspects affecting the decomposition (Penner and Frank, 2018; Luai et al., 2019). The litter that possesses high nutrient concentrations, low recalcitrant compounds, and low C/N ratios is considered as high-quality litter. When the litter of several plant species are mixed under natural conditions, C and other beneficiary nutrient contents can be transformed from high-to low-quality litter. This occurs because of the effects of secondary metabolites and microbial activities alteration (Pérez-Harguindeguy et al., 2007; Chen et al., 2019). As a consequence, the decomposition rate of low quality can be suppressed or enhanced depending upon the inhibitory or stimulating nature of the compounds transformed (Wu et al., 2013). Changes in species evenness can alter litter decomposition depending on the dominancy of plant species in the mixture (Li et al., 2013). A high proportion of nutrient-rich species in a mixture has been shown to intensify microbes’ activities and thereby enhance the decay rate (Chen et al., 2019). Ward et al. (2010) found that plant richness with varying ratios increased decomposition rates when dominant species identity was the prime factor, suggesting that the evenness effect could be important.
Generally, grassland has a variety of biomes ranging from near-pristine to degraded lands due to negative changes (e.g., degradation) in vegetation and soil due to overgrazing (Wang and Ba, 2008; Peng et al., 2020), resulting in widescale of soil salinization (Huang et al., 2012). The decomposition process varies with the degrees of degradation and how plant and soil interact with each other (López-Pujol et al., 2011). In the meadow steppe ecosystem, plant diversity at different sites should not to be neglected in litter decomposition experiments because richness and evenness both impact the magnitude and direction of litter mixing (Chen et al., 2019). Despite this, the relationship between plant diversity and degradation gradients has received insufficient attention in previous studies (Yi et al., 2012; An et al., 2019). Furthermore, a better understanding of their association may provide important information for effectively restoring or conserving the degraded grasslands (Yang et al., 2020).
Previous studies in both field and laboratory microcosms have reported positive or neutral effects of plant diversity on decomposition due to interactive effects of litter assemblage (King et al., 2002; Dickson and Wilsey, 2009; Paudel et al., 2015), but the underlying mechanisms are not well understood. Specifically, we considered the following questions: 1) the differences in initial nutrient contents and C/N ratio between species across degraded grasslands, 2) mass and nutrients dynamics in monoculture and mixture treatments among incubation time and degradation gradients, and 3) effects of litter quality and soil properties on decomposition along with degradation gradients. The main question we sought to address was whether litter mixing induces additive or nonadditive effects on litter and nutrients mass along degradation gradients. If nonadditive, will these effects be antagonistic or synergistic? On the basis of the literature reviewed (Pérez-Harguindeguy et al., 2007; Wu et al., 2013; Chen et al., 2019), we hypothesized that nonadditive, antagonistic effects would prevail and that additive effects would shift to nonadditive effects with incubation time.
Materials and Methods
Study Site
The litter decomposition experiment was conducted at a meadow steppe in the Grassland Ecological Research Station (44°31′–44°46′ N, 123°32′–123°57′ E, elevation of 130–160 m above sea level) of Northeast Normal University, Changling County, Jilin Province, PR China (Figure 1). The climate of the study site is semiarid monsoon with mean annual temperature ranging from 4.6 to 6.4°C. Mean annual precipitation ranges 280–400 mm, and 70–80% of precipitation occurs during June–August. Annual potential of evaporation is 840–1,200 mm, and about 225 days are frosty or snowy. Soil is saline, and alkaline, with pH ranging from 8.2 to 10.6 (Mei et al., 2019). The soil in the study area possesses low N, phosphorus, calcium, and magnesium but high C (Wang et al., 2018). The dominant grass is Leymus chinensis (Trin.) Tzvel followed by other subdominant plants including Phragmites australis (Cav.) Trin. ex Stued., Calamagrostis epigejos (Linn.) Roth., and Chloris virgata Sw. Forbs include Potentilla flagellaris Willd, Kalimeris integrifolia Turcz., and Carex duriuscula, while legumes include Lespedeza davurica (Laxm.) Schindler and Lathyrus quinquenervius (Miq.) Litv.
Experimental Design
A fractional factorial experiment was conducted for 1 year (July 2018–July 2019) on near-pristine, lightly or moderately degraded, and highly degraded (NP, LD, and HD, respectively) sites by following a litterbag technique. Soil degradation in the studied area is due to alkaline soil and overgrazing (Wang and Ba, 2008). The three sites had different soil properties (Supplementary Table S1). Although many grasslands have experienced harsh conditions like cold climate and poor soils, some species can still cope with such environmental stresses and thrive (Mei et al., 2019). At all microsites, dominant and codominant plants include perennial grasses L. chinensis and P. australis, which often co-occur (Wang et al., 2018). L. chinensis is a dominant grass species of meadow steppe, while P. australis tolerates a variety of climatic conditions (Zhang et al., 2018). Nine plots (25 × 25 m) in total, three for each site, were set up and fenced in summer, 2017. Each plot contains three randomly selected 5 × 5 m subplots separated by 50 × 250 cm wide buffers.
Soil and Plant Sampling
In July 2019, we collected topsoil (0–10 cm) using a soil corer (diameter = 2.5 cm) in the fenced plots. Samples were brought to a laboratory, powdered, sieved (mesh size = 2 mm), and divided into two halves. One half was oven dried (65°C for 48 h) to estimate total C (TC%), total organic C (TOC%), and total N (TN%) by an elemental analyzer (Vario EL cube, Elementar Analysensysteme GmbH, Hanau, Germany), while the other half was retained at 4°C for analyzing soil pH, electrical conductivity (EC µs/cm), particle density (PD g/cm3), and moisture content (MC%) later. For the assessment of soil pH and EC, the soil/water (1: 5) suspension technique was followed (Wang et al., 2015). Soil MC was determined by an ECH2O soil moisture sensor (EC-5, Decagon Ltd., Pullman, WA, United States). A picnometric method was used to estimate soil PD (Zygmunt et al., 2014). Soil total C/N was calculated on the basis of atomic mass as Eq. 1:
where TOC is total organic carbon, and TN is total nitrogen.
Freshly senesced leaves of both species were manually collected in early July 2018 from each site. The litter used in this study consisted only of leaves, while shoots and stems were removed carefully. Samples were brought to a laboratory, cleaned from debris, washed lightly with deionized water, oven dried (65°C for 48 h or until it reached to constant weight), and placed separately in an incubator. Leaf litter samples were divided into two halves. The first half was finely powdered in a ball mill grinder (MM 400, Retsch GmbH, Hanau, Germany) to estimate initial C and N concentrations (%) with the same elementar used for soil C and N analyses. The other half was ground with a mortar and pestle to make thread-like particles for the estimation of lignin and cellulose contents (%) by an FT 121 FibertecTM 8,000 analyzer (FOSS). We also calculated the C/N and lignin/N ratios by simply dividing C % or lignin % by N %.
Litter Bag Preparation
Senescent leaves after laboratory treatment were used for litter bag preparation. Nylon litter bags of 15 × 15 cm with a 1-mm mesh size were used. In each microsite, five compositions (two sets of monoculture species and three sets of their evenness mixtures) with triplicate were analyzed. Evenness mixtures included M1 with 30% L. chinensis and 70% P. australis (P. australis dominated or uneven mixture), M2 with 50% L. chinensis and 50% P. australis (even mixture), and M3 with 70% L. chinensis and 30% P. australis (L. chinensis dominated or uneven mixture). Each litterbag was filled with 5.00 ± 0.02 g oven-dried leaf litter of each composition. A total of 90 bags (5 compositions × 3 replications × 3 site types × 2 incubation dates) were prepared, of which 45 bags were collected after 60 days and the rest collected after 365 days. All the bags were deployed above the soil surface to give them natural conditions and on controlled sites to avoid any kind of disturbance. The litter was collected individually from each site, and their litter bags were also placed within each specific site.
Data Analyses
After collection, all litterbags were brought to a laboratory for cleaning and then transferred into paper bags. The litter was oven dried (65°C for 48 h) to measure mass and nutrient contents. Each bag sample was weighed to assess the final mass (MR) by percentage over the time of incubation in monocultures and mixtures:
where Mo and Mi are the observed and initial mass contents in grams. The final nutrient (NR) was determined based on (Bockheim et al., 1991):
where No and Ni are the observed and initial nutrient concentrations, while Mo and Mi are the same as in Eq. 2. The expected final mass (MRexp) of mixture compositions was measured as (Hoorens et al., 2003):
where Ma and Mb are initial mass of the species in the mixture litter bags, and Oa and Ob are the final mass of the mixed litter species in the single-species litter bag. The expected final nutrients (C, N, lignin, or cellulose) were calculated same as Eq. 4 by using appropriate substitutions. We also measured the expected C/N ratio by dividing CRexp with NRexp. Mass loss rate by 60 and 365 days were calculated by:
Statistical Analyses
Initial nutrient contents (%) and final mass or nutrients (%) in monoculture litter were statistically evaluated by t test. We used one-way ANOVA followed by Tukey’s honestly significant difference (HSD) test to determine 1) differences among degradation gradients in initial nutrient contents of each species; 2) final litter or nutrient mass between monoculture litters; 3) final mass (%) or nutrients concentration (%) among mixture compositions; and 4) difference among pooled monoculture litters (Lc + Pa), M1, M2, and M3. Paired t test was applied to compare final mass or nutrient concentration between incubation times of mixture compositions and also for the comparison of litter decomposition between monoculture and mixture within each site type. Redundancy analysis (RDA), a constrained multivariate model, was conducted to quantify how soil properties of each site responded to litter dynamics along degradation gradients over 60 and 365 days. Pearson correlation was used to assess the relationship between initial nutrient contents and mass loss or final nutrient contents in monoculture and mixture compositions by 365 days. Moreover, multiple regression analysis was applied to assess the relationship between soil properties and litter decomposition dynamics in mono and (even or uneven) mixture treatments. Significance was calculated in all tests at p < 0.05.
Litter mixing effects of evenness treatments were analyzed by using two procedures. First, we applied a general linear model (GLM) Type I sums of squares (SS) to test additive or nonadditive effects on litter dynamics. In model, final litter and nutrients mass were added as a response variable to estimate the incubation days, species mixing, evenness, and site effects. Incubation days (T, two levels: 60 and 365 days), mixing term (M, two levels: observed and predicted values), evenness term (E, three levels: M1, M2, and M3), and site term (S, three levels: NP, LD, and HD) were added in the GLM model as main and two-way interaction effects. Nonsignificant values indicate additive effects, while significant values represent nonadditive effects. Second, a traditional method [i.e., O-P (observed–predicted)] was used to specifically test the data in terms of synergistic or antagonistic nonadditive effects. Classifications of litter mixing effects in the O-P method followed Gartner and Cardon (2004): O-P > 0 indicates nonadditive synergistic, O-P < 0 indicates nonadditive antagonistic, and if there is no significant difference between O and p, this would indicate additive effects. The one-sample t test was used to assess the values differing from zero.
Redundancy and multiple regression analyses were conducted in R version 3.6.1, and the significance of RDA correlations was evaluated by Monte Carlo permutation test. All the remaining statistical analyses were evaluated by using SPSS version 23.0 (SPSS Inc., United States) and presented by GraphPad prism.
Results
Initial Nutrient Contents
Initial nutrient contents and C/N ratio varied between monoculture litters, L. chinensis and P. australis. Nutrient concentrations including C, N, lignin, and cellulose in L. chinensis litter were higher than in P. australis litter (p < 0.001) with few exceptions (Table 1). The C/N ratio was lower in L. chinensis than in P. australis. Lignin contents were ≤20% in both species, and cellulose contents were almost twice as much as lignin. Among sites, initial nutrient contents, except for C, were statistically different. N contents in L. chinensis and P. australis were highest in NP and HD with the order of NP > HD > LD and HD > LD = NP, respectively. In contrast, lignin and cellulose concentrations increased with the level of degradation and reached a maximum at HD site (Table 1). Lowest C/N contents were recorded at NP and HD in L. chinensis and P. australis, respectively (p < 0.05). Overall, L. chinensis litter was richer in nutrients than P. australis litter at almost all degraded sites (Table 1).
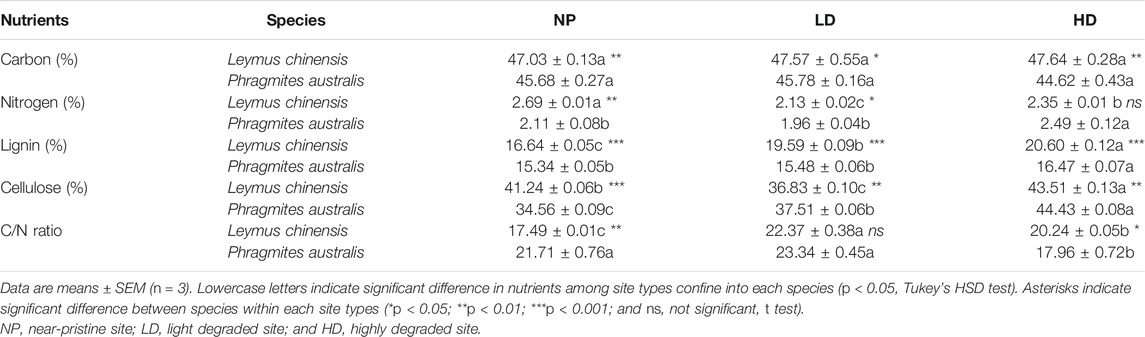
TABLE 1. Initial leaf litter nutrient contents and C/N ratio of plant species, Leymus chinensis and Phragmites australis.
Monoculture Species
At the end of the experiment, final litter and nutrients mass in monoculture litter showed differences with a few significant cases between species (Figure 2 and Supplementary Figure S1). Litter mass declined rapidly at first, and then, the decomposition slowed down toward the last incubation day. The final mass was 50.01, 51.50, and 49.13% for L. chinensis and 53.49, 58.17, and 48.46% for P. australis at NP, LD, and HD sites, respectively, while the only significance recorded between species was at LD by day 60 (p < 0.05). Final mass was consistently lower at the HD site (Figure 2). Litter C and N contents declined with decomposition time, and final C and N contents differed significantly at LD site by day 60 (Supplementary Figures S1A,B). Lignin mass decreased in the beginning and then accumulated through time in a few cases and differed statistically at LD by 365 days (p < 0.01) and also at HD by both incubation periods (Supplementary Figure S1C). However, cellulose mass decreased with time in both species and was different between species at NP by 60 days and HD by 365 days (Supplementary Figure S1D). Among the degraded gradients, final litter mass and nutrients contents did not show any discernible difference.
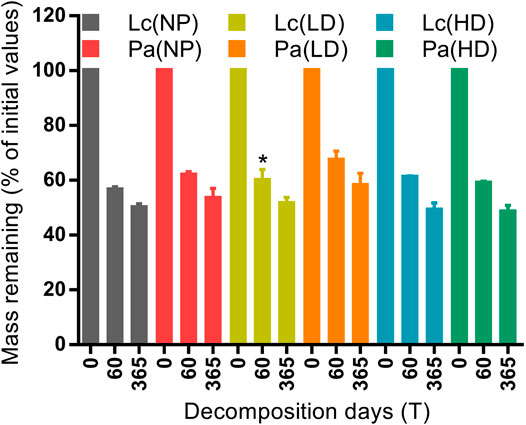
FIGURE 2. Final mass in monoculture litters of plant species, Leymus chinensis (Lc) and Phragmites australis (Pa), over 60 and 365 days of decomposition. NP, near-pristine site; LD, light-degraded site; HD, highly degraded site. Data are means ± SEM (n = 3). Asterisks indicate significant difference between species within each site and confine into each incubation date (*p < 0.05, t test).
Mixture Species
In line with final mass in monoculture litter, there was no significant difference recorded among mixture treatments of L. chinensis and P. australis species, except for final mass at HD by 60 days (Figure 3). In M1, M2, and M3 mixtures, the final mass averaged 48.61, 49.60, and 46.85% at NP; 54.68, 57.03, and 49.89% at LD; and 43.89, 41.47, and 41.23% at HD, respectively. The order of final mass across sites was NP = LD > HD (Figure 3). On the other hand, nutrient dynamics were statistically different by 365 days except for C concentrations at NP and lignin or cellulose concentrations at LD (Supplementary Figure S2). A linear downward pattern was shown in C contents at each site with incubation time, and N contents were significantly higher at NP followed by HD and LD sites (Supplementary Figures S2A,B). Unlike C, lignin contents showed linear upward trend at all sites, and cellulose contents showed irregular patterns (Supplementary Figures S2C,D). Among sites, C, N, and cellulose contents decrease with the degree of degradation (Supplementary Figures S2A,B,D); however, lignin contents were higher at LD site than at the other two sites (Supplementary Figure S2C). Additionally, final mass and nutrient concentrations (except cellulose) were highly significant between incubation days (Supplementary Figure S2).
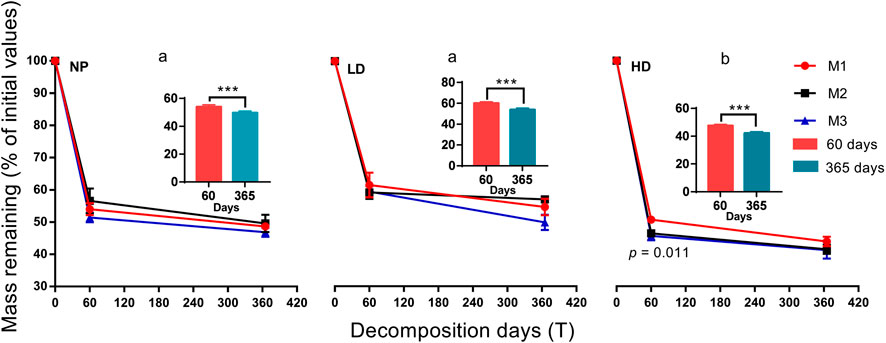
FIGURE 3. Final mass in evenness mixture litters (M1, M2, and M3) across site types over 60 and 365 days of decomposition. M1, 30% Lc:70% Pa; M2, 50% Lc:50% Pa; and M3, 70% Lc:30% Pa. Leymus chinensis (Lc) and Phragmites australis (Pa). NP, near-pristine site; LD, light-degraded site; and HD, highly degraded site. Data are means ± SEM (n = 3). Lowercase letters indicate significant differences in average final mass (M1 + M2 + M3) among sites [p < 0.05, Tukey’s honestly significant difference (HSD) test]. Significance difference is estimated among mixture litters within each site and confine into each incubation date (p < 0.05, Tukey’s HSD test). In the insets, the average final mass at 60 and 365 days of incubation within each evenness treatment has been shown (***p < 0.001, paired t test).
Overall mass loss was higher in M3, intermediate in M2, and lower in M1 with significant differences between monoculture species (L. chinensis + P. australis) and M3 mixture by day 60 (Supplementary Figure S3). Between the monoculture litters, we observed that mass loss in M3 mixture increased when mixed with L. chinensis monoculture litter in both periods, but the effect was not statistically significant (p > 0.05). In contrast, P. australis monoculture litter had great influence on the mass loss of M3 mixture by day 60 (Supplementary Figure S4). Along degraded sites, we found a significant difference only at HD site between monoculture and mixture final mass, C contents, and lignin contents (Supplementary Figures S5A,B,E). At the LD site, monoculture litter was different from mixture litter in N contents, lignin contents, and C/N ratio (Supplementary Figures S5C–E).
Influence of Litter Quality on Monospecific and Mixture Species Mass Loss
Mass losses after 365 days in the monoculture litter were not correlated with litter quality or initial nutrient contents (Table 2). For all nutrients, negative correlation was observed more frequently than positive correlation between initial and final concentrations. Initial lignin contents showed highly significant relationships with final C (positive), N (positive), lignin (negative), and cellulose (negative). Initial cellulose contents were positively correlated with final N and cellulose. Initial C was positively related with final C, while initial lignin/N was negatively correlated with final cellulose. Finally, initial C, C/N ratio, and lignin/N ratio were negatively related to final N (Table 2).
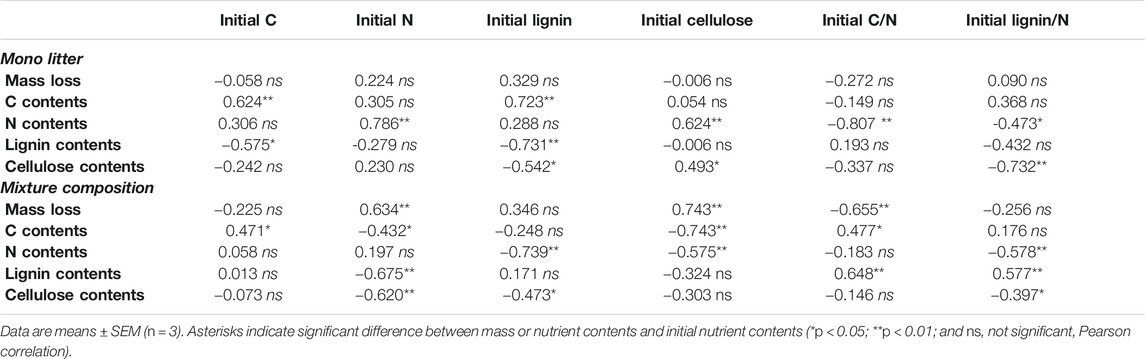
TABLE 2. Matrix of Pearson correlation between initial nutrient contents and mass loss or final nutrient contents observed in monoculture and mixture compositions after 365 days of decomposition.
Mass losses after 365 days in mixture compositions were positively correlated with initial nutrient contents of N or cellulose and negatively correlated with initial C/N ratio (Table 2). Initial lignin, cellulose, and lignin/N ratio were negatively related to final N and cellulose. Positive relationship was found between initial C/N and lignin/N ratios, on the one side, and final lignin on the other. Initial N showed negative relations with final N and lignin. Finally, significant relationships between initial cellulose (negative), C (positive), and C/N (positive), on the one side, and final C, on the other, were observed.
Influence of Soil Properties on Monospecific and Mixture Species Mass Loss
According to multiple regression and RDA analyses, soil properties significantly impacted the litter decomposition dynamics among grassland degraded degrees (Figure 4 and Supplementary Table S2). The soil attributes were constrained to the first and second axes, explaining 54.92 and 23.07% by 60 days and 71.49 and 22.53% by 365 days of the total variance in final mass and nutrient concentrations of all treatments on all sites. Litter decomposition dynamics of mixture treatments were positively correlated with EC, MC, TC/N, while pH and PD were minor factors affecting litter dynamics by 365 days only (Figure 4). Regarding degradation gradients, HD site was strongly regulated by pH, EC, and MC over 365 days. Multiple regression analysis showed that single-species litters were not significantly affected by soil properties (Supplementary Table S2). In uneven mixtures, positive correlations were found in M1 by MC and pH while in M3 by EC, MC, TOC, and TN. In even mixture, M2 was strongly affected by all soil properties except soil pH (Supplementary Table S2).
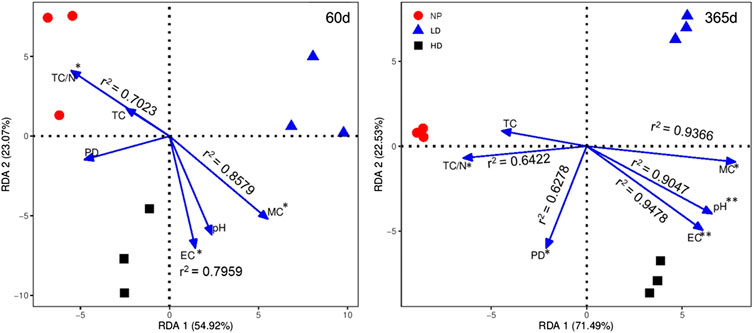
FIGURE 4. Redundancy analysis (RDA) for analyzing the effect of soil properties on final mass and nutrient concentrations in monoculture and mixture litters along degradation gradients over 60 and 365 days of decomposition. Final mass and nutrient contents coordinates are not displayed for clearer results. EC, electrical conductivity; TC, total carbon; MC, moisture contents; PD, particle density; and TC/N, total C/N. NP, near-pristine site; LD, light-degraded site; and HD, highly degraded site. Significance between compositions and soil properties variables has been shown (*p < 0.05 and **p < 0.01, Monte Carlo permutation test).
Influence of Litter Mixing on Litter and Nutrients Mass Remaining
Due to our nonfully factorial experiment design, there was a caution needed to unveil the results of GLM. Despite this, litter-mixing-induced nonadditive effects of mixing (M), evenness (E), site (S), and days (T) on final mass occurred in six out of 10 cases (Table 3). The two-way interaction as T × M and M × S strongly affected final mass, while remaining (two-way) interactions produce additive results. In final nutrient contents and C/N ratio, two-way ANOVA indicated that nonadditive effects prevailed over additive effects in 31 out of 50 cases of the mixture compositions. Nonadditive litter mixing effects were more prominent in lignin, cellulose, and C/N ratio as compared to C and N contents. Almost all the main or single terms showed significant nonadditive effects on litter and nutrients dynamics (Table 3).
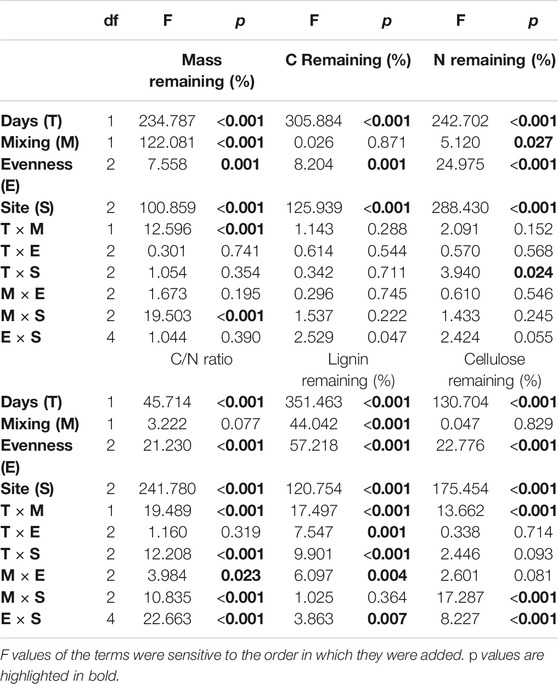
TABLE 3. Summary of the general linear model (GLM) using Type I sum of squares (SS) for testing the evenness and diversity effects of litter mixing on final litter mass and nutrients and C/N ratio at different degraded gradients during decomposition days.
Unlike GLM model results, additive effects were prevalent, pooling together with litter and nutrient dynamics, in 57% of all mixtures (62 out of 108 cases, Figure 5). Nonadditive antagonistic effects on final mass were observed in 22% of all mixture litters (4 out of 18 cases; three mixtures with two incubation dates, Figure 5A). In final nutrients, litter mixing induced both antagonistic and synergistic effects in evenness treatments along the decomposition period. In final C, 50% (9 out of 18 cases) showed more synergistic effects than antagonistic effects (6 vs. 3 out of 18 cases, Figure 5B). Overall, 56% of all mixtures in final N were nonadditive in which antagonistic effects were slightly more prominent than synergistic effects (6 vs. 4 out of 18 cases, Figure 5C). Concerning C/N ratio, nonadditive antagonistic and synergistic effects were found in 17 and 22% of all mixtures, respectively (3 vs. 4 out of 18 cases, Figure 5D). Nonadditive synergistic effects masked the antagonistic effects in 33 and 6% of all mixtures, respectively, in final lignin (6 vs. 1 out of 18 cases, Figure 5E). Regarding cellulose, antagonism and synergism were recorded at the same rate (22%, 4 vs. 4 out of 18, Figure 5F). Among mixtures, the highest nonadditive litter mixing effects were recorded in M3 mixture followed by M1 and M2 mixtures (17 vs. 16 vs. 13 out of 108 cases, respectively) on mass and nutrient dynamics throughout the decomposition process. Meanwhile, litter mixing effects were significant among degraded gradients in mixtures over incubation days with few exceptions. However, more cases were recorded at HD site followed by NP and LD sites (18 vs. 15 vs. 13 out of 108 cases, respectively, Figure 5).
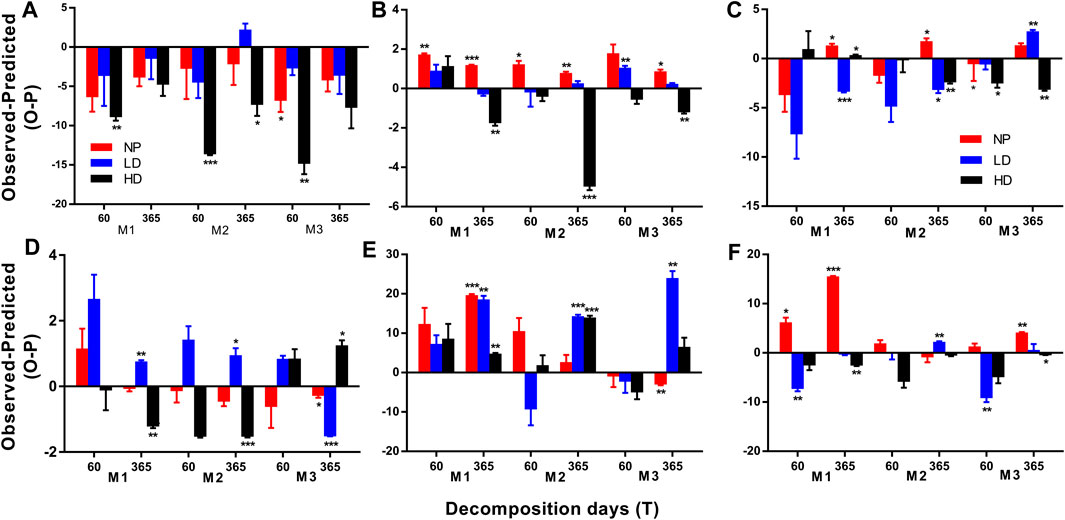
FIGURE 5. The relative mixture effect on final mass and nutrients concentrations and C/N ratio over 60 and 365 days of decomposition. M1, 30% Lc:70% Pa; M2, 50% Lc:50% Pa; and M3, 70% Lc:30% Pa. Leymus chinensis (Lc) and Phragmites australis (Pa). (A) Mass remaining; (B,C) remaining; (C) N remaining; (D) C/N ratio; (E) lignin remaining; and (F) cellulose remaining. NP, near-pristine site; LD, light-degraded site; and HD, highly degraded site. Data are means ± SEM (n = 3). Nonadditive effects are represented by significant difference between values and zero (*p < 0.05; **p < 0.01; and ***p < 0.001, one-sample t test).
Discussion
Some previous studies in temperate grasslands experimentally investigated litter mixing effects of different plant species with various proportions and found nonadditive effects being either antagonistic or synergistic. In the real world, however, it is complicated to explain these effects due to variations in vegetation properties, habitats, and their associated climatic conditions. In the current experiment, we tried to test the effect of litter mixing on monoculture and mixture species in degraded meadow steppe and also found antagonistic and synergistic effects in the same mixtures, but additive effects shifted to nonadditive effects in final nutrients concentrations with time.
The two contrasting species chosen in this study cover a wide range of nutrient contents that elucidate the differences detected for decomposition rates in single- and mixture-species litter bags (Figures 2, 3 and Table 1). There was a small difference in initial C and N contents and C/N ratios between L. chinensis and P. australis examined in the experiment (Table 1). Both plants are perennial, rhizomes, and resistant to alkaline stress (Zhang et al., 2018). Additionally, they belong to the same Gramineae family and coexist in the study area for a long time (home effect), but their richness varies with site conditions (Wang et al., 2018). Because of this reason, we found ample evidence for host advantage in our experiment with consumers decomposing litter more rapidly in their home plots (Luai et al., 2019). In the light of this, leaf litter of L. chinensis decomposed faster than that of P. australis (although not statistically different, Figure 2). However, the presence of L. chinensis litter did not affect mixture compositions significantly over both incubation days (Supplementary Figure S4), and final mass of pooled monoculture litter (L. chinensis + P. australis) was higher than final masses of mixture treatments (Supplementary Figure S3). Besides, soil properties, especially total organic C and N contents, were strongly correlated with mass loss in L. chinensis-dominant mixture (Supplementary Table S2). Soil chemical properties affect the activities of microbial communities and, in turn, stimulate mass loss (Frouz, 2018). We found rapid mass loss in monoculture and mixture litter compositions regardless of site effect ranging from 50 to 60% throughout the incubation process (Figures 2, 3), indicating that rapid mass decay is common in grasslands (Moore and Fairweather, 2006). Nearly 50% of the litter in monoculture and mixture decomposed in the first 60 days, which is comparable with other studies (Hector et al., 2000; Bengtsson et al., 2011; Berg and McClaugherty, 2014). The initial litter decay was due to the outcome of the more labile components in the litter (Table 1). In addition, our study began in July, a warm season with high precipitation, followed by November–May, a cool dry season. Given that microbial activities are higher during warm and humid seasons than during cold and dry seasons, litter decomposition was highly dependent on the intensity of microbial activities (Yang et al., 2011; Yao et al., 2011). Although the microbial abundance was not estimated, we noticed that mixture litters were more heavily fed upon by soil animals than monoculture litters.
We also found a wide range of nutrients dynamics in the leaf litter of monoculture and mixture specific species (Supplementary Figures S1, S2). In our study, the species with C/N < 20 showed continuous C and N contents release, while the litter with a C/N greater than the critical level accumulated these nutrients (Supplementary Figures S1A,B, S2A,B and Table 1). This result is aligned with that of Lu et al. (2011), who reported the same finding and stated that N is immobilized above the threshold level of C/N, and its dynamics can be explained based on the critical threshold level of the C/N ratio of the litter. The threshold level of C/N, in general, is 20–30 (Liu et al., 2012). Since fungi and bacteria are the main drivers of the decomposition process, at the beginning of the decay, the litter generally contains less nitrogen according to the needs of decomposers. Therefore, decomposer communities immobilize initial plus mineralized nitrogen from the neighboring environment and use litter carbon as the primary source of energy (Berglund and Ågren, 2012). On the other hand, lignin contents showed a constant increase in monoculture and mixture species at all sites (Supplementary Figures S1C, S2C); as a result, decomposition slowed down after the first incubation date (Figures 2, 3). Our findings support the fact that lignin is a resistant nutrient in litter compared to other chemical substrates (Taylor et al., 1989). The clear increase in lignin contents during litter nutrient dynamics is due to other labile contents as C or cellulose that have probably rapid loss rates (He et al., 2019). Loss in cellulose contents was twice as fast as loss in lignin contents during the last days of the experiment (Supplementary Figures S1C,D, S2C,D), although cellulose accumulation was detected by 60 days. Consistent with the hypotheses of a past study (Berg and McClaugherty, 2014), cellulose contents release from litter increased during later decomposition phase because the labile material of litter in plant cell walls was confined by recalcitrant C and lignin. These results are reasonable as litter is considered one of the main source of soil nutrient cycling. Furthermore, the analysis of two species mixtures of similar functional plant groups gives knowledge about the litter mixing effects on nutrient dynamics that can be helpful when scaling up the nutrient fluxes at grassland ecosystem levels.
According to the GLM model, litter mixing (in interaction with other terms) and species evenness (individually) induced nonadditive effects on mass and nutrients dynamics (Table 3). In two-way interactions with mixing, time, and site, the evenness factor had no effect on final mass, C, and N contents (Table 3). A few previous studies discovered similar findings (King et al., 2002; Li et al., 2013), but some also found positive relationships between evenness and decomposition (Dickson and Wilsey, 2009; Ward et al., 2010). This may explain the lack of relationship between litter evenness and decay process. Our results suggest that evenness effects are time dependent, and our study is 365 days long, thus unable to predict evenness effect in the advanced humus near stage. Our findings about litter mixing effects on final mass and nutrient contents are consistent with published results (Ball et al., 2008; Wu et al., 2013; Castro-Díez et al., 2019). The assumption that alteration is biogeochemical functioning is probably challenged by nonadditive effects on litter decay dynamics under climate change (Wu et al., 2013). Wardle et al. (1997) reported that nonadditive effects of litter mixing are dominant when monoculture litters vary greatly in functional properties, but strong evidence provides unambiguous support for this phenomenon (Quested et al., 2002; Hoorens et al., 2003; Mao et al., 2015). In conflict, it has been stated that mixture having functionally similar litter types may decompose faster than the predicted mass decay (Maisto et al., 2011). Likewise, our chosen species also shared the same functional group and pattern of decomposition. Moreover, litter quality of individual species in the mixture strongly affected the functioning of the decomposition process (Scowcroft, 1997; Zhang et al., 2013). We also found that initial nutrient contents, especially N and C/N ratio, were positively correlated with final mass of mixture species (Table 2). Species mixing and evenness can mediate the decay and most nutrient contents release, but plant species evenness per se may result in more stable decomposition processes (Mao et al., 2015). However, it is difficult to predict any directional effect of evenness on decay method as the number of species has been shown to be much less important than litter mixture’s taxonomic composition in controlling decomposition (Lecerf et al., 2011; Chen et al., 2019).
Based on general exploratory method, ∼57.4% additive, ∼21.3% antagonistic, and ∼21.3% synergistic effects were found in final mass and nutrients contents in a 1-year decomposition experiment (Figure 5). These results were different from reported results (∼30% additive, ∼50% antagonistic, and ∼20% synergistic effects) by Gartner and Cardon (2004) and other renowned studies (Bonanomi et al., 2010; Li et al., 2013; Chen et al., 2019). Some researchers suggested that litter mixing could have mixed effects on litter decomposition (Kominoski et al., 2007; Butenschoen et al., 2014). In line with our hypothesis, we found antagonistic effects of litter mixing on final mass in the mixture treatments but no relation with time (Figure 5A). Regarding nutrients, contrary to our hypothesis, synergistic effects prevailed over antagonistic effects, but additive effects shifted to nonadditive effects with incubation time (Figures 5B–F). Nonadditive effects are inconsistent during different decomposition stages of a litter cohort (Chen et al., 2019). This is because of deviations in the contents of water-soluble minerals and fiber components during incubation (Lecerf et al., 2011; Berg and McClaugherty, 2014). The conflicting results are likely due to the differences in the methodology used in previous studies by Ball et al. (2008), Bonanomi et al. (2010), and the current study, including the variant effects of species selection, environmental conditions, and most importantly incubation time i.e., 3 years, 90 days, and 1 year, respectively. Additionally, our experiment ended soon after cool dry season, so leaching and decay of inhibitory compounds in litter reduced microbial activities (Trevathan-Tackett et al., 2020). Moreover, there are more neutral effects recorded in the current study in agreement with a previous study by Srivastava et al. (2009). This happens because nonadditive effects mainly took place in labile carbon and most of the labile carbon lost by 60 days; therefore, additive effects become more obvious in the final mass. These outcomes could be because no correlation was found between mass loss and initial lignin or lignin/N ratio (Table 2). Second, the pattern of lignin and cellulose release (Supplementary Figures S1C,D) hinders litter decay in the later phase of incubation and hence results in antagonistic effects. Third, rapid leaching of nutrient (C and N) contents into soil occurred at the start of experiment (Supplementary Figures S1A,B, S2A,B) and increased in secondary metabolites due to high precipitation (Li et al., 2013). In our study, 70–80% precipitation was concentrated during June–August; thereby, the nutrient transfer among species in mixtures by leaching was high during the early phase of incubation. Our results showed that climatic conditions should be accounted for in the prediction of frequency and magnitude of litter mixing effects, different from some previous research findings (Duan et al., 2013). Shifts in litter mixing effects over time could have a substantial influence on biogeochemical functioning from a long-term perspective due to change in litter quality during the decomposition process.
Owing to the environmental stresses from livestock overgrazing and climate impacts like droughts, about one-third of the available grasslands have been degraded worldwide (Peng et al., 2020). Grassland degradation affects vegetation and soil properties and in turn affects litter decomposition. Our study found specific effects of degradation on litter and nutrient dynamics, as mass loss was high at the HD site in both monoculture and mixture litters (Figures 2, 3). Particularly, the significant nonadditive effects of litter mixing on final mass were more common at HD sites (Figure 5). Moreover, we found significant differences in final mass and C content at HD site between monoculture and mixture litters (Supplementary Figure S5). Cowan and Anderson (2019) did not discover any significant effect of site type on decomposition because of dense litter fall occurrence at degraded sites. Bengtsson et al. (2011) showed delay in the decomposition process in the degraded site compared with the pristine site. In contrast, another study reported an increase in mass with the degree of degradation in Chinese forest systems (Paudel et al., 2015). Such a conflict with previous studies is possibly due to that soil properties as pH, electrical conductivity, and moisture contents significantly affected mass and nutrient dynamics at HD site by 365 days (Figure 4). Moisture contents made the litter more favorable to microbial attack, leading to high decomposition (Brandt et al., 2010). Meanwhile, diverse litter fall can also increase litter decomposition at the degraded site (Naeem et al., 2017). Overall, the current study reveals a variable and peculiar response of plant litter decomposition dynamics to species diversity, especially for evenness.
Conclusion
In this study, litter mixing produced idiosyncratic response to mass and nutrients release in the decomposition process at variant degrees of degradation with incubation time. Although our experiment was limited to 1 year, we still found that decomposition was positively affected by L. chinensis and P. australis species, and almost every mixture showed significant results on litter dynamics in the meadow steppe. Nonadditive effects on litter and nutrients found in species mixing, evenness, site, and decomposition time are driven by litter quality. Our study suggests that the role of diversity, especially species evenness, is more important and critical to understanding the full scope of potential species loss and relative species composition in grasslands. Our study also provides insights into the quantification of nutrient cycling in the meadow steppe ecosystem.
Data Availability Statement
The original contributions presented in the study are included in the article/Supplementary Material, further inquiries can be directed to the corresponding author.
Author Contributions
LW, DW, and IN designed the study; IN, TA, XW, and LY performed the research; TA, XW, IN, and NH conducted statistical analyses; IN, TA, HW, and DW wrote the manuscript.
Funding
This project was funded by the National Key Research and Development Program of China (2016YFC0500602 and 2016YFC0500602), the National Natural Science Foundation of China (31770520 and 31700357), the National Key Technology Support Program (2013BAC09B03), and the Program for Introducing Talents to Universities (B16011).
Conflict of Interest
The authors declare that the research was conducted in the absence of any commercial or financial relationships that could be construed as a potential conflict of interest.
Supplementary Material
The Supplementary Material for this article can be found online at: https://www.frontiersin.org/articles/10.3389/fenvs.2021.582409/full#supplementary-material.
References
An, H., Tang, Z., Keesstra, S., and Shangguan, Z. (2019). Impact of desertification on soil and plant nutrient stoichiometry in a desert grassland. Sci. Rep. 9 (1), 9422. doi:10.1038/s41598-019-45927-0
Austin, A. T., Vivanco, L., González-Arzac, A., and Pérez, L. I. (2014). There’s no place like home? An exploration of the mechanisms behind plant litter-decomposer affinity in terrestrial ecosystems. New Phytol. 204 (2), 307–314. doi:10.1111/nph.12959
Ball, B. A., Hunter, M. D., Kominoski, J. S., Swan, C. M., and Bradford, M. A. (2008). Consequences of non-random species loss for decomposition dynamics: experimental evidence for additive and non-additive effects. J. Ecol. 96 (2), 303–313. doi:10.1111/j.1365-2745.2007.01346.x
Bengtsson, J., Janion, C., Chown, S. L., and Leinaas, H. P. (2011). Variation in decomposition rates in the fynbos biome, South Africa: the role of plant species and plant stoichiometry. Oecologia 165 (1), 225–235. doi:10.1007/s00442-010-1753-7
Berg, B., and McClaugherty, C. (2014). Plant litter: decomposition, humus formation, carbon sequestration. 3rd Edn. Berlin: Springer.
Berglund, S. L., and Ågren, G. I. (2012). When will litter mixtures decompose faster or slower than individual litters? A model for two litters. Oikos 121 (7), 1112–1120. doi:10.1111/j.1600-0706.2011.19787.x
Bockheim, J., Jepsen, E. A., and Heisey, D. M. (1991). Nutrient dynamics in decomposing leaf litter of four tree species on a sandy soil in northwestern Wisconsin. Can. J. For. Res. 21 (6), 803–812. doi:10.1139/x91-113
Bonanomi, G., Incerti, G., Antignani, V., Capodilupo, M., and Mazzoleni, S. (2010). Decomposition and nutrient dynamics in mixed litter of Mediterranean species. Plant Soil. 331 (1), 481–496. doi:10.1007/s11104-009-0269-6
Brandt, L. A., King, J. Y., Hobbie, S. E., Milchunas, D. G., and Sinsabaugh, R. L. (2010). The role of photodegradation in surface litter decomposition across a grassland ecosystem precipitation gradient. Ecosystems 13 (5), 765–781. doi:10.1007/s10021-010-9353-2
Butenschoen, O., Krashevska, V., Maraun, M., Marian, F., Sandmann, D., and Scheu, S. (2014). Litter mixture effects on decomposition in tropical montane rainforests vary strongly with time and turn negative at later stages of decay. Soil Biol. Biochem. 77, 121–128. doi:10.1016/j.soilbio.2014.06.019
Castro-Díez, P., Alonso, Á., and Romero-Blanco, A. (2019). Effects of litter mixing on litter decomposition and soil properties along simulated invasion gradients of non-native trees. Plant Soil. 442 (1-2), 79–96. doi:10.1007/s11104-019-04160-4
Chen, Y., Ma, S., Jiang, H., Yangzom, D., Cheng, G., and Lu, X. (2021). Decomposition time, chemical traits and climatic factors determine litter-mixing effects on decomposition in an alpine steppe ecosystem in Northern Tibet. Plant Soil. 459, 23–35. doi:10.1007/s11104-019-04131-9
Cowan, O. S., and Anderson, P. M. L. (2019). Litter decomposition variation across a degradation gradient and two seasons in a critically endangered vegetation type within the Fynbos biome, South Africa. S. Afr. J. Bot. 121, 200–209. doi:10.1016/j.sajb.2018.11.002
Dickson, T. L., and Wilsey, B. J. (2009). Biodiversity and tallgrass prairie decomposition: the relative importance of species identity, evenness, richness, and micro-topography. Plant Ecol. 201 (2), 639–649. doi:10.1007/s11258-008-9567-y
Duan, J., Wang, S., Zhang, Z., Xu, G., Luo, C., Chang, X., et al. (2013). Non-additive effect of species diversity and temperature sensitivity of mixed litter decomposition in the alpine meadow on Tibetan Plateau. Soil Biol. Biochem. 57, 841–847. doi:10.1016/j.soilbio.2012.08.009
Frouz, J. (2018). Effects of soil macro- and mesofauna on litter decomposition and soil organic matter stabilization. Geoderma 332, 161–172. doi:10.1016/j.geoderma.2017.08.039
García-Palacios, P., Maestre, F. T., Kattge, J., and Wall, D. H. (2013). Climate and litter quality differently modulate the effects of soil fauna on litter decomposition across biomes. Ecol. Lett. 16 (8), 1045–1053. doi:10.1111/ele.12137
García-Palacios, P., Shaw, E. A., Wall, D. H., and Hättenschwiler, S. (2016). Temporal dynamics of biotic and abiotic drivers of litter decomposition. Ecol. Lett. 19, 554–563. doi:10.1111/ele.12590
Gartner, T. B., and Cardon, Z. G. (2004). Decomposition dynamics in mixed-species leaf litter. Oikos 104 (2), 230–246. doi:10.1111/j.0030-1299.2004.12738.x
Gessner, M. O., Swan, C. M., Dang, C. K., McKie, B. G., Bardgett, R. D., Wall, D. H., et al. (2010). Diversity meets decomposition. Trends Ecol. Evol. 25 (6), 372–380. doi:10.1016/j.tree.2010.01.010
Hättenschwiler, S., and Gasser, P. (2005). Soil animals alter plant litter diversity effects on decomposition. Proc. Natl. Acad. Sci. U.S.A. 102 (5), 1519–1524. doi:10.1073/pnas.0404977102
He, W., Ma, Z., Pei, J., Teng, M., Zeng, L., Yan, Z., et al. (2019). Effects of predominant tree species mixing on lignin and cellulose degradation during leaf litter decomposition in the three Gorges reservoir, China. Forests 10 (4), 360. doi:10.3390/f10040360
Hector, A., Beale, A. J., Minns, A., Otway, S. J., and Lawton, J. H. (2000). Consequences of the reduction of plant diversity for litter decomposition: effects through litter quality and microenvironment. Oikos 90 (2), 357–371. doi:10.1034/j.1600-0706.2000.900217.x
Hoorens, B., Aerts, R., and Stroetenga, M. (2003). Does initial litter chemistry explain litter mixture effects on decomposition? Oecologia 137 (4), 578–586. doi:10.1007/s00442-003-1365-6
Huang, F., Wang, P., and Zhang, J. (2012). Grasslands changes in the northern songnen plain, China during 1954-2000. Environ. Monit. Assess. 184 (4), 2161–2175. doi:10.1007/s10661-011-2107-6
Jacobson, K., van Diepeningen, A., Evans, S., Fritts, R., Gemmel, P., Marsho, C., et al. (2015). Non-rainfall moisture activates fungal decomposition of surface litter in the Namib Sand Sea. PLoS One. 10 (5), e0126977. doi:10.1371/journal.pone.0126977
King, R. F., Dromph, K. M., and Bardgett, R. D. (2002). Changes in species evenness of litter have no effect on decomposition processes. Soil Biol. Biochem. 34 (12), 1959–1963. doi:10.1016/S0038-0717(02)00204-3
Kominoski, J. S., Pringle, C. M., Ball, B. A., Bradford, M. A., Coleman, D. C., Hall, D. B., et al. (2007). Nonadditive effects of leaf litter species diversity on breakdown dynamics in a detritus-based stream. Ecology 88 (5), 1167–1176. doi:10.1890/06-0674
López-Pujol, J., Zhang, F.-M., Sun, H.-Q., Ying, T.-S., and Ge, S. (2011). Centres of plant endemism in China: places for survival or for speciation? J. Biogeogr. 38 (7), 1267–1280. doi:10.1111/j.1365-2699.2011.02504.x
Lecerf, A., Marie, G., Kominoski, J. S., LeRoy, C. J., Bernadet, C., and Swan, C. M. (2011). Incubation time, functional litter diversity, and habitat characteristics predict litter-mixing effects on decomposition. Ecology 92 (1), 160–169. doi:10.1890/10-0315.1
Lecerf, A., Risnoveanu, G., Popescu, C., Gessner, M. O., and Chauvet, E. (2007). Decomposition of diverse litter mixtures in streams. Ecology 88 (1), 219–227. doi:10.1890/0012-9658(2007)88[219:DODLMI]2.0.CO;2
Li, D., Peng, S., and Chen, B. (2013). The effects of leaf litter evenness on decomposition depend on which plant functional group is dominant. Plant Soil. 365 (1-2), 255–266. doi:10.1007/s11104-012-1337-x
Liski, J., Nissinen, A. R. I., Erhard, M., and Taskinen, O. (2003). Climatic effects on litter decomposition from arctic tundra to tropical rainforest. Glob. Change Biol. 9 (4), 575–584. doi:10.1046/j.1365-2486.2003.00605.x
Liu, J., Wu, J., Liu, F., and Han, X., 2012. Quantitative assessment of bioenergy from crop stalk resources in Inner Mongolia, China. Appl. Energ. 93, 305–318. doi:10.1016/j.apenergy.2011.12.059
Liu, P., Sun, O. J., Huang, J., Li, L., and Han, X. (2007). Non-additive effects of litter mixtures on decomposition and correlation with initial litter N and P concentrations in grassland plant species of northern China. Biol. Fertil. Soils. 44 (1), 211–216. doi:10.1007/s00374-007-0195-9
Lu, M., Zhou, X., Luo, Y., Yang, Y., Fang, C., Chen, J., et al. (2011). Minor stimulation of soil carbon storage by nitrogen addition: a meta-analysis. Agric. Ecosyst. Environ. 140 (1-2), 234–244. doi:10.1016/j.agee.2010.12.010
Luai, V. B., Ding, S., and Wang, D. (2019). The effects of litter quality and living plants on the home-field advantage of aquatic macrophyte decomposition in a eutrophic urban lake, China. Sci. Total Environ. 650, 1529–1536. doi:10.1016/j.scitotenv.2018.09.104
Maisto, G., De Marco, A., Meola, A., Sessa, L., and De Santo, A. V. (2011). Nutrient dynamics in litter mixtures of four Mediterranean maquis species decomposing in situ. Soil Biol. Biochem. 43 (3):520–530. doi:10.1016/j.soilbio.2010.11.017
Mao, B., Yu, Z.-Y., and Zeng, D.-H. (2015). Non-additive effects of species mixing on litter mass loss and chemical properties in a Mongolian pine plantation of Northeast China. Plant Soil. 396 (1), 339–351. doi:10.1007/s11104-015-2593-3
Mao, R., and Zeng, D. H. (2012). Non-additive effects vary with the number of component residues and their mixing proportions during residue mixture decomposition: a microcosm study’ Geoderma 170, 112–117. doi:10.1016/j.geoderma.2011.11.008
Maxwell, S. L., Fuller, R. A., Brooks, T. M., and Watson, J. E. (2016). Biodiversity: the ravages of guns, nets and bulldozers. Nature 536 (7615), 143–145. doi:10.1038/536143a
Mei, L., Yang, X., Zhang, S., Zhang, T., and Guo, J. (2019). Arbuscular mycorrhizal fungi alleviate phosphorus limitation by reducing plant N:P ratios under warming and nitrogen addition in a temperate meadow ecosystem. Sci. Total Environ. 686, 1129–1139. doi:10.1016/j.scitotenv.2019.06.035
Meier, C. L., and Bowman, W. D. (2008). Links between plant litter chemistry, species diversity, and below-ground ecosystem function. Proc. Natl. Acad. Sci. U.S.A. 105, 19780–19785. doi:10.1073/pnas.0805600105
Moore, T. N., and Fairweather, P. G. (2006). Decay of multiple species of seagrass detritus is dominated by species identity, with an important influence of mixing litters. Oikos 114 (2), 329–337. doi:10.1111/j.2006.0030-1299.14576.x
Munoz, B., Savage, R., and Baker, V. (2018). “Chapter 3.10 – general issues in statistical analysis of RAMs,” in Wetland and stream rapid assessments. Editors J. Dorney, R. Savage, R. W. Tiner, and P. Adamus (Academic Press: San Diego).
Naeem, I., Ansari, L., Nizami, S. M., Asif, T., Tariq, H., and Mahmood, T. (2017). Investigating the rate of litterfall and decomposition in Phulai dominated forest of Pakistan: a nutrient cycling perspective. Int. J. Biosci. 10 (1), 42–51. doi:10.12692/ijb/10.1.42-51
Pérez-Harguindeguy, N., Blundo, C. M., Gurvich, D. E., Díaz, S., and Cuevas, E. (2007). More than the sum of its parts? Assessing litter heterogeneity effects on the decomposition of litter mixtures through leaf chemistry. Plant Soil. 303 (1), 151–159. doi:10.1007/s11104-007-9495-y
Paudel, E., Dossa, G. G. O., de Blécourt, M., Beckschäfer, P., Xu, J., and Harrison, R. D. (2015). Quantifying the factors affecting leaf litter decomposition across a tropical forest disturbance gradient. Ecosphere 6 (12), 1–20. doi:10.1890/es15-00112.1
Peng, F., Xue, X., Li, C., Lai, C., Sun, J., Tsubo, M., et al. (2020). Plant community of alpine steppe shows stronger association with soil properties than alpine meadow alongside degradation. Sci. Total Environ. 733, 139048. doi:10.1016/j.scitotenv.2020.139048
Penner, J. F., and Frank, D. A. (2018). Litter decomposition in Yellowstone grasslands: the roles of large herbivores, litter quality, and climate. Ecosystems 22, 929–937. doi:10.1007/s10021-018-0310-9
Quested, H. M., Press, M. C., Callaghan, T. V., and Cornelissen, H. J. (2002). The hemiparasitic angiosperm Bartsia alpina has the potential to accelerate decomposition in sub-arctic communities. Oecologia 130 (1), 88–95. doi:10.1007/s004420100780
Rumpel, C., Crème, A., Ngo, P. T., Velásquez, G., Mora, M. L., and Chabbi, A. (2015). The impact of grassland management on biogeochemical cycles involving carbon, nitrogen and phosphorus. J. Soil Sci. Plant Nutr. 15 (2), 353–371. doi:10.4067/S0718-95162015005000034
Santonja, M., Milcu, A., Fromin, N., Rancon, A., Shihan, A., Fernandez, C., et al. (2018). Temporal shifts in plant diversity effects on carbon and nitrogen dynamics during litter decomposition in a Mediterranean shrubland exposed to reduced precipitation. Ecosystems 22, 939–954. doi:10.1007/s10021-018-0315-4
Scowcroft, P. G. (1997). Mass and nutrient dynamics of decaying litter from Passiflora mollissima and selected native species in a Hawaiian montane rain forest. J. Trop. Ecol. 13 (3), 407–426. doi:10.1017/S0266467400010592
Smith, W. K., Gao, W. E. I., Steltzer, H., Wallenstein, M. D., and Tree, R. (2010). Moisture availability influences the effect of ultraviolet-B radiation on leaf litter decomposition. Glob. Change Biol. 16 (1), 484–495. doi:10.1111/j.1365-2486.2009.01973.x
Srivastava, D. S., Cardinale, B. J., Downing, A. L., Duffy, J. E., Jouseau, C., Sankaran, M., et al. (2009). Diversity has stronger top-down than bottom-up effects on decomposition. Ecology 90 (4), 1073–1083. doi:10.1890/08-0439.1
Taylor, B. R., Parkinson, D., and Parsons, W. F. J. (1989). Nitrogen and lignin content as predictors of litter decay rates: a microcosm test. Ecology 70 (1), 97–104. doi:10.2307/1938416
Trevathan-Tackett, S. M., Jeffries, T. C., Macreadie, P. I., Manojlovic, B., and Ralph, P. (2020). Long-term decomposition captures key steps in microbial breakdown of seagrass litter. Sci. Total Environ. 705, 135806. doi:10.1016/j.scitotenv.2019.135806
Wang, D., and Ba, L. (2008). Ecology of meadow steppe in northeast China. Rangeland J. 30 (2), 247–254. doi:10.1071/RJ08005
Wang, Y., Jiang, Q., Yang, Z., Sun, W., and Wang, D. (2015). Effects of water and nitrogen addition on ecosystem carbon exchange in a meadow steppe. PLoS One. 10 (5), e0127695. doi:10.1371/journal.pone.0127695
Wang, Z., Yuan, X., Wang, D., Zhang, Y., Zhong, Z., Guo, Q., et al. (2018). Large herbivores influence plant litter decomposition by altering soil properties and plant quality in a meadow steppe. Sci. Rep. 8, 9089. doi:10.1038/s41598-018-26835-1
Ward, S. E., Ostle, N. J., McNamara, N. P., and Bardgett, R. D. (2010). Litter evenness influences short-term peatland decomposition processes. Oecologia 164 (2), 511–520. doi:10.1007/s00442-010-1636-y
Wardle, D. A., Bonner, K. I., and Nicholson, K. S. (1997). Biodiversity and plant litter: experimental evidence which does not support the view that enhanced species richness improves ecosystem function. Oikos 79 (2), 247–258. doi:10.2307/3546010
Wu, D., Li, T., and Wan, S. (2013). Time and litter species composition affect litter-mixing effects on decomposition rates. Plant Soil. 371 (1), 355–366. doi:10.1007/s11104-013-1697-x
Yang, H., Wu, M., Liu, W., Zhang, Z. H. E., Zhang, N., and Wan, S. (2011). Community structure and composition in response to climate change in a temperate steppe. Glob. Change Biol. 17 (1), 452–465. doi:10.1111/j.1365-2486.2010.02253.x
Yang, X., Qu, Y., Yang, N., Zhao, H., Wang, J., Zhao, N., et al. (2019). Litter species diversity is more important than genotypic diversity of dominant grass species Stipa grandis in influencing litter decomposition in a bare field. Sci. Total Environ. 666 (1), 490–498. doi:10.1016/j.scitotenv.2019.02.247
Yang, Y., Hobbie, S. E., Hernandez, R. R., Fargione, J., Grodsky, S. M., Tilman, D., et al. (2020). Restoring abandoned farmland to mitigate climate change on a full earth. One Earth. 3, 176–186. doi:10.1016/j.oneear.2020.07.019
Yao, H., Bowman, D., and Shi, W. (2011). Seasonal variations of soil microbial biomass and activity in warm- and cool-season turfgrass systems. Soil Biol. Biochem. 43 (7), 1536–1543. doi:10.1016/j.soilbio.2011.03.031
Yi, X. S., Li, G. S., and Yin, Y. Y. (2012). The impacts of grassland vegetation degradation on soil hydrological and ecological effects in the source region of the Yellow River-A case study in Junmuchang region of Maqin country. Proced. Environ. Sci. 13, 967–981. doi:10.1016/j.proenv.2012.01.090
Zeng, L., He, W., Teng, M., Luo, X., Yan, Z., Huang, Z., et al. (2018). Effects of mixed leaf litter from predominant afforestation tree species on decomposition rates in the Three Gorges Reservoir, China. Sci. Total Environ. 639, 679–686. doi:10.1016/j.scitotenv.2018.05.208
Zhang, N., Guo, R., Song, P., Guo, J., and Gao, Y. (2013). Effects of warming and nitrogen deposition on the coupling mechanism between soil nitrogen and phosphorus in Songnen Meadow Steppe, northeastern China. Soil Biol. Biochem. 65, 96–104. doi:10.1016/j.soilbio.2013.05.015
Zhang, N., Zhang, J., Li, Z., Chen, J., Zhang, Z., and Mu, C. (2018). Resistance strategies of Phragmites australis (common reed) to Pb pollution in flood and drought conditions. PeerJ 6 (2-3), e4188. doi:10.7717/peerj.4188
Zhang, X., Wang, X., Finnegan, P. M., Tan, W., and Mao, R. (2019). Effects of litter mixtures on aerobic decomposition rate and its temperature sensitivity in a boreal peatland. Geoderma 354, 113890. doi:10.1016/j.geoderma.2019.113890
Keywords: litter mixing, nutrient cycling, litter quality, evenness, non-additive effects, incubation time, grassland
Citation: Naeem I, Asif T, Wu X, Hassan N, Yiming L, Wang H, Wang L and Wang D (2021) Species Diversity Induces Idiosyncratic Effects on Litter Decomposition in a Degraded Meadow Steppe. Front. Environ. Sci. 9:582409. doi: 10.3389/fenvs.2021.582409
Received: 21 August 2020; Accepted: 11 January 2021;
Published: 03 March 2021.
Edited by:
Fereidoun Rezanezhad, University of Waterloo, CanadaReviewed by:
Saraswati Saraswati, University of Waterloo, CanadaBijendra Man Bajracharya, University of Waterloo, Canada
Copyright © 2021 Naeem, Asif, Wu, Hassan, Yiming, Wang, Wang and Wang. This is an open-access article distributed under the terms of the Creative Commons Attribution License (CC BY). The use, distribution or reproduction in other forums is permitted, provided the original author(s) and the copyright owner(s) are credited and that the original publication in this journal is cited, in accordance with accepted academic practice. No use, distribution or reproduction is permitted which does not comply with these terms.
*Correspondence: Deli Wang, d2FuZ2RAbmVudS5lZHUuY24=