- College of Science and Engineering, Flinders University, Bedford Park, SA, Australia
Legionella is an opportunistic waterborne pathogen associated with Legionnaires' disease and Pontiac fever. Despite improved public awareness, the incidence of Legionella associated infections has been increasing. Aerosols generated from engineered potable water systems are a demonstrated cause of both nosocomial and community-acquired legionellosis. The ecology of Legionella in these systems is complex with multiple factors impacting their colonization and persistence. Flow dynamics has been identified as an important factor and stagnation in cooling towers is an accepted risk for increased Legionella growth; however, less is known about the impact of flow dynamic on Legionella in potable water systems. This is especially complex due to the inherent intermittent and variable usage observed within outlets of a potable water system. This systematic literature review examines the role of fluid dynamics and stagnation on the colonization and growth of Legionella in potable water systems. Twenty two of 24 identified studies show a positive association between stagnation zones and increased colonization of Legionella. These zones included dead legs, dead ends, storage tanks, and obstructed water flow (such as intermittent usage or flow restriction). Prolonged stagnation in building plumbing systems also deteriorates the quality of thermally or chemically treated potable water. This stimulates the colonization of Legionella established biofilms. Such biofilms are intrinsically resistant to disinfection procedures and accelerate the rate of decay of chemical disinfectants. Sub-lethal doses of disinfectants and the presence of protozoan hosts in stationary water promote generation of viable but non-culturable Legionella cells. This results in false negatives in surveillance methods that use culture methodology. In conclusion, elimination of temporal and permanent stagnation points can improve the quality of potable water, efficacy of disinfectants, and reduce the risk of legionellosis. Current guidelines and water safety plans recognize the risks associated with permanent stagnation point (dead ends and dead legs); however, there is a need for greater emphasis on controlling temporal stagnation arising from intermittent usage.
Introduction
The importance of Legionella as an opportunistic waterborne intracellular human pathogen is well-documented (Berjeaud et al., 2016). It is frequently associated with nosocomial and community-acquired legionellosis (Pontiac fever and Legionnaires' disease [LD]) in immunocompromised and immunosuppressed individuals (Fields et al., 2002). Legionella is ubiquitously present in constructed water systems. It is frequently detected in domestic and hospital water systems, cooling towers, humidifiers, fountains, and pools, (Bartram et al., 2007; Fitzhenry et al., 2017).
Despite recent advancements in disease surveillance and management systems, the legionellosis incidence and outbreak rate remains high. In the USA, there were 290 reported outbreaks of legionellosis from 2009 to 2017 (Centers for Disease Control Prevention, 2020) and in 2017, 28 outbreaks were reported in nine European Union countries. In Europe, the legionellosis notification rate also increased from 12 cases/million in 2013 to 18 cases/million in 2017 (European Centre for Disease Prevention and Control, 2019).
According to the USA Centers for Disease Control and Prevention, from 2013 to 2015 ~67% potable waterborne outbreaks were caused by Legionella pneumophila (Shah et al., 2018). Plumbing networks and potable water distribution systems of hotels, healthcare facilities, and residential buildings were identified as Legionella hotspots (Benedict et al., 2017). Clearly, understanding the environmental factors that affect the survival and growth of Legionella in potable water is essential to inform improved management and control strategies.
The growth of Legionella in potable water systems is primarily associated with biofilms and the free-living amoeba hosts feeding on biofilms (Ashbolt, 2015). This provides Legionella protection from severe physiochemical stresses (Thomas et al., 2004; Dupuy et al., 2011). The environmental conditions that influence the formation of biofilms are essential for the control of Legionella. These include stagnation and the presence of plumbing dead legs and dead ends that provide favorable conditions for microbial growth (Bartram et al., 2007). Dead legs (isolated pipes with limited or no water flow) and dead ends (redundant capped pipe which completely obstruct water flow) are well-established as factors contributing to stagnation (National Academies of Sciences Engineering Medicine, 2019). Stagnation or areas with inappropriate hydraulic dynamics are likely to result in the failure of disinfection procedures (Ling et al., 2018). Due to the complex relationship between different environmental variables it can be challenging to study role of stagnation in Legionella growth. This systematic literature review examines the specific role that water stagnation plays in Legionella colonization and growth within potable water systems. Studies on potable water systems including surveillance and laboratory-based studies that examine the relationship between stagnation and fluid dynamics on water quality and Legionella colonization are explored.
Materials and Methods
The protocol of this systematic literature review was designed according to the instructions of the PRISMA statement (Figure 1). The databases Scopus and Web of Science were searched for all articles written in English and published prior to April 2020. In the databases the search terms (“Legionella pneumophila” OR “L. pneumophila” OR Legionella OR legionellosis OR “Legionnaires' disease” OR “Pontiac fever”) AND (flow* OR “dead end*” OR “dead leg*” OR “water circulation” OR “water recirculation” OR “Reynolds number” OR stagnation OR stagnant OR “stationary water” OR turbid OR turbidity OR usage*) AND (plumbing OR pipework OR pipe OR artificial OR piping OR building OR manufactured OR engineered OR potable OR manmade* OR biofilm OR water) were applied. Initially, all identified records were combined and duplicates removed. Subsequently, articles were manually screened by reading the titles and abstracts and excluding those that discussed engineering works, bacterial colonization or biofilms without specifically referring to Legionella. Papers were also excluded that referred to stagnation in municipal water supplies as this is a different issue compared with building water supplies. Finally, the remaining articles were analyzed in full and excluded if they did not examine or describe the relationship between Legionella colonization, growth, survival, and water stagnation, or recirculation in building water systems.
Results
A total of 395 abstracts were obtained from the Web of Science and Scopus. After applying the described inclusion and exclusion criteria (as presented in Figure 1), 24 research articles describing a relationship between water stagnation and Legionella or L. pneumophila survival and colonization were identified as suitable for inclusion in this review (Table 1).
Study Sites
Seventeen of 24 studies (Table 1) investigated hot or cold water storage tanks and building water distribution systems; 7/24 studies were in vitro modeling experiments. The majority of the real-world studies were from investigations of hospital water systems (12/17) and almost half of these were conducted in response to a legionellosis outbreak (5/12). Half of the studies (14/24) discussed the relationship between Legionella contamination or colonization and permanent stagnation points (dead ends 6/24, dead legs 8/24). In the majority of studies, there was limited information provided describing fluid or water dynamics.
Building and Plumbing System Studies
According to Table 1, L. pneumophila serogroup (sg) 1 frequently contaminates water and plumbing system of hospitals and municipal buildings. L. pneumophila sg1 is the most common serogroup associated with legionellosis. Few studies reported contamination of other L. pneumophila serotypes (sg2–4, sg2–14, sg2–16, sg3, sg4, sg6, sg10, and sg10–14) and Legionella species (L. anisa, L. steigerwaltii, L. feelii, L. longbeachae, L. micdadei, and L. rubrilucens).
Sixteen out of 17 studies examining building plumbing systems showed a positive association between stagnation and increased Legionella colonization/persistence. Ten out of 17 studies of the building plumbing systems demonstrated that permanent stagnation points (dead ends 5/17, dead legs 5/17) were positively associated with increased Legionella contamination. Three studies presented in Table 1 specifically demonstrated that restricted
water circulation resulted in persistence of Legionella in hospital hot water storage tanks with capacities of ≈7,600–15,000 L (Ciesielski et al., 1984; Ezzeddine et al., 1989; Darelid et al., 2002).
Ten building water system studies reported a reduction in Legionella numbers after increasing water flow rates and removal of dead legs or dead ends. Some studies specifically used hot water recirculation (Darelid et al., 2002; Gavalda et al., 2019) or an increase in the flow rate of hot water (Ezzeddine et al., 1989; Boppe et al., 2016) to reduce Legionella contamination. In Italy (2018–2020), installation of time flow taps with 64–192 L/day flow rate in the vicinity of dead legs and dead ends effectively reduced L. pneumophila contamination from hot water supplies of hospitals (Totaro et al., 2018, 2020).
The one study that did not find any positive association between stagnation and Legionella growth was by Sidari et al. (2004). This study examined water storage and distribution in a 437 bed hospital in Pennsylvania after numerous cases of nosocomial LD over a 5 year period (1994–1999). Flushing of hot water temporarily reduced the concentration of Legionella; however, this study found that removal of dead legs and routine chlorination was not sufficient to reduce Legionella contamination. Finally, ClO2 (0.3–0.5 mg/L) treatment for 21 months resulted in complete removal of Legionella (culture negative).
In vitro Model Plumbing Studies
As with the real-world studies, in 6/7 in vitro model plumbing studies a positive relationship between water stagnation and Legionella colonization was identified (Table 1). Several model systems demonstrated that stagnation promoted genesis of stable Legionella–eukaryotic microbe biofilms (Vervaeren et al., 2006; Biyela et al., 2012). Farhat et al. (2010) constructed a pilot scale hot water system consisting of both dynamic water loops (flow rate: 15 L/min) and dead leg stagnation areas. They estimated the Legionella concentration (using culture, qPCR, and epifluorescent microscopic counts) was 1–2 log greater in stagnant points. Two studies also investigated the role of ClO2 treated water in elimination of Legionella contamination from dead legs (Thomas et al., 2004; Loret et al., 2005). It was found that 20% replacement of dead leg water with ClO2 treated water was not sufficient to completely eliminate culturable Legionella. However, regular complete replacement of ClO2 treated water in dead legs was able to eliminate culturable Legionella within 7 days (Loret et al., 2005). Another pilot scale domestic water model consisting of both dynamic and stagnant water channels demonstrated that continuous flow of ClO2 treated water for 7 days significantly reduced L. pneumophila from copper dead legs (Thomas et al., 2004).
The one in situ model study that did not find any positive association with stagnation and Legionella colonization was conducted by Liu et al. (2006). This model consisted of three parallel pipes within a partially open system (5% of water continuously flowed through the system while 95% of the water was recirculated). The water temperature was maintained at 24°C, one pipe had laminar flow (Re: 355–2,000), one turbulent flow (Re: 10,000–40,000), and one was stagnant. Significantly higher concentrations of culturable Legionella were recovered from the biofilm in the pipe with turbulent flow followed by laminar flow with the lowest Legionella concentrations observed in the biofilm in the pipe with stagnant flow. However, a significant difference in Legionella concentrations in biofilm was observed after 1 week. These concentrations remained static for the remaining 5 weeks of sampling. The authors attributed this result to the turbulent flow increasing the mass transfer of the nutrients and oxygen from the water in the seeding tank. They also noted that the turbulence created may have been insufficient to detach the biofilm. There was no significant difference in planktonic Legionella between any of the treatments. This study concluded that intermittently used pipe work (dead legs) sustained aerobic microbial populations than dead ends (where nutrient depletion is more likely).
Discussion
Legionella is a typical premise plumbing pathogen that can tolerate disinfectants, develop biofilms, survive in waterborne protozoa, and thrive in low levels of nutrients (Fields et al., 2002). Prolonged water stagnation can result in accumulation of nutrients and compromises disinfection, promoting colonization of premise plumbing pathogens in potable water systems (Gauthier et al., 1999; Rhoads et al., 2016; Ling et al., 2018; Xu et al., 2018).
Influence of Water Stagnation on Bacterial Colonization
Microbial ecology of potable water is very complex. From water source to point of use, the varying environmental conditions modulate growth and composition of microbial communities. In building plumbing systems, water can stagnate permanently or temporarily (Prévost et al., 1997). Dead ends permanently stagnate water (Tercelj-Zorman et al., 2004); whereas, water storage tanks and temporal water usage can result in intermittent stagnation (Peter and Routledge, 2018). Prior to consumption, water can stagnate from a few hours to weeks in a building piping system (Manuel et al., 2009). This may result in the deterioration of water quality, and promote accumulation of biomass thereby increasing the concentration of intact and cultivable cells.
Temporary Stagnation
Water in municipal distribution pipelines rarely gets obstructed. As it enters domestic building plumbing systems it stagnates and changes the diversity of microbial communities (Pepper et al., 2004). It has been observed that overnight stagnation resulted in a 4 to 580-fold increase in microbial load (using heterotrophic plate counts), a 2 to 3-fold increase in total cell concentration (using flow cytometry), and a 2 to 8-fold increase in microbial activity (ATP analysis) (Lautenschlager et al., 2010). This was supported by a study which showed that a 2 week stagnation of a domestic buildings plumbing system resulted in a 2 log increase in microbial load (using flow cytometry) (Lipphaus et al., 2014).
Building design also impacts on stagnation and microbial water quality. A recent study demonstrated that water from the plumbing system of a net-zero energy residential building contained 5 log higher amount of bacterial 16S rDNA (6.46 × 107 gene unit/mL) and Legionella 16S rDNA (8.91 × 104 gene copies/mL) compared with a typical residential building plumbing system (400 gene unit/mL and 100–2.3 × 103 gene copies/mL, respectively). This variation, due to building structure and design, could be attributed to increased stagnation prior to consumption as the average stagnation time for a net-zero energy residential building is 2.7 days compared with 1 day in a typical residential building (Rhoads et al., 2016). In vitro green building hot water plumbing model studies also suggested limited water flushing and inadequate temperature (51°C) support colonization of Legionella and host protozoa (Rhoads et al., 2015). In conclusion, stagnation and aging of water in plumbing system of green energy buildings resulted in persistence of microbial contamination.
Permanent Stagnation
Dead legs and dead ends have been implicated in several nosocomial outbreaks of LD (Patterson et al., 1994; Tercelj-Zorman et al., 2004; Bartley et al., 2016). These permanent stagnation points should be avoided or managed during building construction or modifications (Bartram et al., 2007). However, it is impractical to remove them all. Recently, two studies of Italian hospitals demonstrated that installation of time flow taps (flow rate 64–192 L/day) in the vicinity of dead legs was successful in reducing L. pneumophila contamination in the hot water system (Totaro et al., 2018, 2020). In the first study, L. pneumophila from various serogroups including sg3, sg2–4, sg6, and sg10–14, with concentrations ranging from 1 × 102 to 1.3 × 105 CFU/L, was present in hospital hot water (temperature: 38.2 ± 2.1–46.8 ± 2.1°C and free chlorine: 0.05 ± 0.007–0.30 ± 0.01 mg/L). Instead of removing dead legs, time flow taps were installed in the outlet closest to each identified dead leg. This strategy successfully eliminated all culturable L. pneumophila (Totaro et al., 2018). In the second study, it was found that within 15 days of installation of time flow taps (flow rates 192 L/day) in the proximity of dead ends, L. pneumophila sg1, sg2–14, and sg2–16 contamination in hospital hot water (temperature: 44.8–53.2°C and free chlorine: 0.23–0.36 mg/L) was reduced from 2 × 102 to 1.4 × 105 CFU/L to no culturability (Totaro et al., 2020). These studies demonstrate that reducing temporary stagnation and increasing flow, even in the presence of permanent stagnation points, reduces the risk of Legionella contamination.
Several model studies have explored the impact of permanent stagnation on Legionella colonization. In a pilot scale model, bacterial biofilms in dead legs (pre-treatment population density 107 CFU/L and 108 genomic unit/L) survived thermal shock treatment and promoted rapid recolonization within 48 h (Farhat et al., 2010). Thus, permanent stagnation sites act as a reservoir of Legionella biofilms that play an important role in re-contamination of water.
Water Stagnation and Failure of Disinfection Procedures
The accelerated decay of residual disinfectant significantly increases the risk of LD (Voss et al., 1985). Prolonged water stagnation, microbial communities, and organic nutrients accelerate the decay of disinfectants (Rhoads et al., 2016; Ling et al., 2018; Xu et al., 2018). According to the US CDC, from 2000 to 2014 70% of LD outbreaks were due to inadequate disinfectant concentrations in water supplies (Garrison et al., 2016). Currently in the USA, six disinfection procedures: Cu–Ag ionization, chlorine, chlorine dioxide, monochloramine, ozonisation, and ultraviolet disinfection, are used to control Legionella (Environmental Protection Agency, 2016b). In the USA, the concentration of chemical disinfectant is maintained in 95% potable water delivered to consumers (Environmental Protection Agency, 2016a). In studies from Austria, Germany, Netherlands, and Switzerland, the concentration of residual disinfectant in potable water (delivered to consumers) is not maintained (Rosario-Ortiz et al., 2016).
Decay of Disinfectant
Different chemical disinfectants are widely used to disinfectant potable water supplies (Pontius, 1990; Kim et al., 2002; World Health Organization, 2004). The residual disinfectant concentration in treated water does not remain constant and it gradually decreases within building plumbing systems. This continuous process of decay may result in complete degradation of chemical disinfectants, thereby increasing the chances of persistence of microbial contamination in building plumbing systems (Vieira et al., 2004). Rates of decay have been associated with temporary or permanent stagnation, aging of plumbing systems, nature of plumbing material, and microbial biomass (Vieira et al., 2004; Patrick et al., 2012; Ling et al., 2018). Goyal and Patel (2015) reported continuous temporal stagnation in storage tanks for 22 h decreased residual chlorine concentration (from 0.2 to ~0.12 mg/L) in domestic buildings. Similarly, Barbeau et al. (2005) reported that 24 h of temporary stagnation decreased chlorine content of water (from 0.6 to ~0.3 mg/L in cement line ductile and 0.4–0.05 mg/L in gray cast iron pipe dead end) and increased the microbial count. Galvin (2011) demonstrated that the lower flow velocity observed in dead ends can result in a decrease in concentration of both chlorine and chloramine within 200 h. Laboratory model based experiments have also demonstrated that an increase in residence time of water results in decreased concentrations of chlorine, chloramine, and ozone and increased microbial contamination (Clark et al., 1994). Another model system showed that complete renewal of ClO2 (0.5 mg/L) disinfected water in dead legs eliminated culturable Legionella (Loret et al., 2005).
Persistence of Intrinsically Disinfectant Resistant Legionella
Intrinsic resistance is a natural tolerance and resistance attributed to Legionella against physical and chemical water treatments (Steinert et al., 1998; Cooper and Hanlon, 2010). Biofilm in areas of stagnation within plumbing systems may harbor resistant populations and act as a continuous source of microbial contamination (Bartley et al., 2016). Studies have shown that currently available water disinfection methods (i.e., chlorine, chlorine dioxide, chloramines, hydrogen peroxide, ozone, copper, and silver ions) are only successful in reducing or eliminating Legionella populations transiently. Sidari et al. (2004) and Totaro et al. (2018) (see Table 1) demonstrated the inability of chlorine to eliminate all culturable Legionella. According to Totaro et al. (2018), circulation of hot chlorinated water in dead legs eliminated all chlorine sensitive culturable L. pneumophila sg2–4 and sg10–14 serotypes, though chlorine resistant and thermostable L. pneumophila sg3 and sg6 serotypes persisted in low concentrations.
A study conducted in a century old hospital in Italy found that continuous hyperchlorination (0.5–1 mg/L) for 5 years was not sufficient to completely eliminate Legionella contamination. Multiple factors including outdated piping, dead legs, improper water circulation and corrosion of plumbing materials were proposed as causes of Legionella persistence (Orsi et al., 2014). Similarly, a study conducted in another Italian hospital plumbing system demonstrated persistence of the same strain of L. pneumophila for a period of 15 years (1990–2004) despite thermal treatment, chlorination, and chlorine dioxide treatment (Scaturro et al., 2007). This demonstrates the role permanent stagnation (dead ends and dead legs) has in harboring and potentially selecting for more resistant strains through constant exposure to sub-lethal concentration of disinfectants (Cooper and Hanlon, 2010; Dupuy et al., 2011).
Stagnation and Microbial Biofilms
In water storage and distribution systems, 95% of the microbial population exists as biofilms attached to the inner surfaces and only 5% in the water (Flemming et al., 2002). According to an in vitro simulation experiment, plumbing coated with Legionella biofilms decayed free chlorine in stagnant water (48 h stagnation period) and increased the risk of legionellosis up to six times (Huang et al., 2020). Available literature shows that in potable water and building plumbing systems complex biofilms and amoebae hosts protect Legionella (Kilvington and Price, 1990; Thomas et al., 2004). Cargill et al. (1992) reported that in contrast to free living cells, L. pneumophila existing in complex biofilms can tolerate high doses of disinfectant. Specifically, the amoeba Acanthamoeba and Vermamoeba provide additional protection from prolonged and persistent water treatment processes (Kilvington and Price, 1990; Dupuy et al., 2011; Cervero-Arago et al., 2014; Dobrowsky et al., 2016). Donlan et al. (2005) observed that for L. pneumophila–amoebae complex biofilms, monochloramine (0.5 mg/L) was a more effective disinfectant compared to free chlorine (0.5 mg/L). Stagnant potable water allows formation of thick, dense, complex, and adherent biofilms, which accelerate decay of disinfectants (Tsagkari and Sloan, 2018). More importantly, chemical disinfectants are unable to penetrate such multispecies biofilms (Bridier et al., 2011).
Stagnation in Building Hot Water System
The studies presented in Table 1 demonstrate that due to stagnation points and low water consumption, building hot water systems will develop Legionella contamination. Unlike cold water, hot water systems contained diverse species and serotypes of Legionella (Voss et al., 1985; Trop Skaza et al., 2012; Totaro et al., 2018; Girolamini et al., 2019). An in vitro study demonstrated colonization of diverse species of Legionella i.e., L. pneumophila, L. anisa, L. taurinensis, and L. drancourtii, and protozoan hosts i.e., alveolata members, Bodonidae, Euglenozoa, Neobodo curvifilu, Thecamoebae, Vannella, and Vermamoeba vermiformis in a hot water plumbing model. Moreover, it was also noticed that pathogenic L. pneumophila and L. anisa developed stable biofilms with protozoa (Thecamoebae, Vannella, and V. vermiformis) in storage tanks and survived during thermal (70°C/30 min) and chemical (biodispersant: tensio-active Ferrofos® 5260 and biocide: H2O2-peracetic acid) treatments. After such treatments, these stable biofilms re-contaminated hot water within the entire plumbing model (Farhat et al., 2012). Saby et al. (2005) analyzed hot water plumbing system models (materials: steel, galvanized steel, and chlorinated polyvinyl chloride) and concluded that chemical disinfection procedures (H2O2, continuous chlorination, hyperchlorination, and peracetic acid treatment) temporarily eradicated established Legionella biofilm. The only solution to eradicate Legionella biofilms was to maintain water temperature at >55°C at all points, which required continuous circulation of hot water. In another pilot study (material: stainless steel), it was noticed that thermal treatment (70°C/30 min) of the plumbing system containing a well-established Legionella (103 CFU/cm2) biofilm decreased culturable Legionella. It was also observed that existence of dead legs in plumbing system promoted rapid recontamination of remaining water (Farhat et al., 2010). All studies discussing hot water supplies to buildings showed that stagnation of hot water in storage tanks and dead legs or dead ends led to a reduction in water temperature (<45°C), and rapid decay of disinfectants promoting colonization of Legionella. This was exacerbated in warm water systems as dissipation of the residual chemical disinfectant was further accelerated at high temperature (Vasconcelos et al., 1997; Ndiongue et al., 2005).
Nutrient and Oxygen Supply Hypothesis
One argument presented by Liu et al. (2006) that counters the positive association of stagnation with Legionella contamination of biofilms is the reduced oxygen and nutrient content present in areas of stagnation compared with areas of turbulent flow. However, L. pneumophila sg1 has been shown to survive in drinking water under low nutrient availability for more than 2 years (Paszko-Kolva et al., 1992). L. pneumophila can also proliferate efficiently at 6–6.7 mg/L concentration of dissolved oxygen. However, at oxygen concentration <2.2 mg/L it can survive but stops multiplying (Wadowsky et al., 1985). L. pneumophila (sg1, sg2, sg3, sg4, and sg6) has also been isolated from different water bodies (i.e., lakes and rivers) with dissolved oxygen concentrations of 0.3–9.6 mg/L (Fliermans et al., 1981). In water at temperatures of 20–45°C, the concentration of dissolved oxygen ranges from 9.06 to 5.94 mg/L (Environmental Protection Agency, 1989), which is sufficient for Legionella survival and growth. Furthermore, upon environmental stresses (nutrient starvation, low oxygen, osmolarity alterations, pH, and temperature fluctuations) bacteria in biofilms activate stress tolerance mechanisms, which can lead to genesis of viable but non-culturable (VBNC) cells (Yamamoto et al., 1996; Fux et al., 2005). Previous research has also shown replication within protozoan hosts (Buse et al., 2013) and presence of disinfectants (Allegra et al., 2008; Turetgen, 2008; Mustapha et al., 2015; Whiley et al., 2017; Casini et al., 2018) promotes Legionella transformation into VBNC cells. As such, studies that rely solely on culture methods of detection [such as the study by Liu et al. (2006)] will not detect VBNC Legionella and underestimate the numbers present (Whiley, 2016).
Current Methods of Legionella Screening
In stagnation areas, limited nutrient availability and sub-lethal doses of disinfectant promote generation of VBNC Legionella (Li et al., 2014). According to Farhat et al. (2010) thermal treatment of water decreased the numbers of culturable Legionella temporarily, but increased concentration of VBNC cells. A 1 year pilot study demonstrated that chemical disinfection (Ferrocid® 8591, Ferrofos® 5260, H2O2 and peracetic acid) decreased culturable Legionella and L. pneumophila from 0.5 to 2 log, however application of a PCR analysis showed existence of VBNC in high density (Farhat et al., 2011). As mentioned earlier, at stagnation points Legionella also exists in complex biofilms, and amoebae hosts dwell in such microbial communities as well. It is well-known that amoebae hosts (V. vermiformis) are capable to transform culturable Legionella into VBNC (Buse et al., 2013). VBNC are pathogenic in nature and infect amoebae and human cell lines (Cervero-Arago et al., 2019). Proteomic profiling suggests VBNC are able to synthesize some virulence factors and proteins involved in different metabolic pathways (Alleron et al., 2013). Acanthamoeba castellanii and A. polyphaga resuscitate VBNC synthesized by starvation (Steinert et al., 1997) and disinfectant treatment (Garcia et al., 2007), respectively. In vitro studies showed A. polyphaga resuscitated Legionella are infectious for alveolar epithelial and macrophage like cells (Epalle et al., 2015). So far the underlying mechanisms of VBNC biogenesis and resuscitation are not yet well-understood.
Multiple methods are available to screen and detect Legionella contamination in potable water and building plumbing systems (Table 2). Any method that can identify and estimate both culturable and VBNC Legionella is most appropriate to monitor building plumbing system and potable water. Microbiological culturing (International Organization for Standardization, 2017) and qPCR (International Organization for Standardization, 2019) are approaches recommended by ISO (international organization for standardization), however both methods are unable to provide information about VBNC Legionella. Fluorescence in situ hybridization (Delgado-Viscogliosi et al., 2005) and viability qPCR (Ditommaso et al., 2014) are two techniques that can be used to estimate populations of VBNC Legionella, however validity of both assays is challenged by high density of sample background bacteria other than Legionella. Differential live/dead stain flow cytometry is widely used for in vitro disinfectant efficacy and plumbing model experiments to estimate the population of VBNC Legionella (Allegra et al., 2008, 2011; Wang et al., 2010; Mustapha et al., 2015). Some researchers have tried to develop and use dye labeled Legionella specific antibodies for detection of VBNC, however these antibodies are highly specific and can only detect specific strain/serogroup/serotypes (Füchslin et al., 2010; Keserue et al., 2012). A universal probe, which can cover all members of genus Legionella, is required to be effective to detect and estimate VBNC contamination from building plumbing systems. Legionella–amoebae co–culturing is one of the best techniques to identify VBNC contamination. In this assay, suspected samples are cultivated on amoebae hosts (preferably Acanthamoeba) and VBNC resuscitation is monitored microscopically (Conza et al., 2013). Garcia et al. (2007) artificially generated VBNC and then resuscitated them back into culturable Legionella using an Acanthamoeba host. Using an Acanthamoeba co–culture assay, Conza et al. (2013) estimated the quantity of VBNC Legionella (102-106 cells/g) from a composting facility. A major drawback of Legionella–amoebae co–culturing is the requirement of incubation for prolonged time. However, to our knowledge available literature has not discussed the application of co–culture assays to estimate VBNC contamination from building plumbing system.
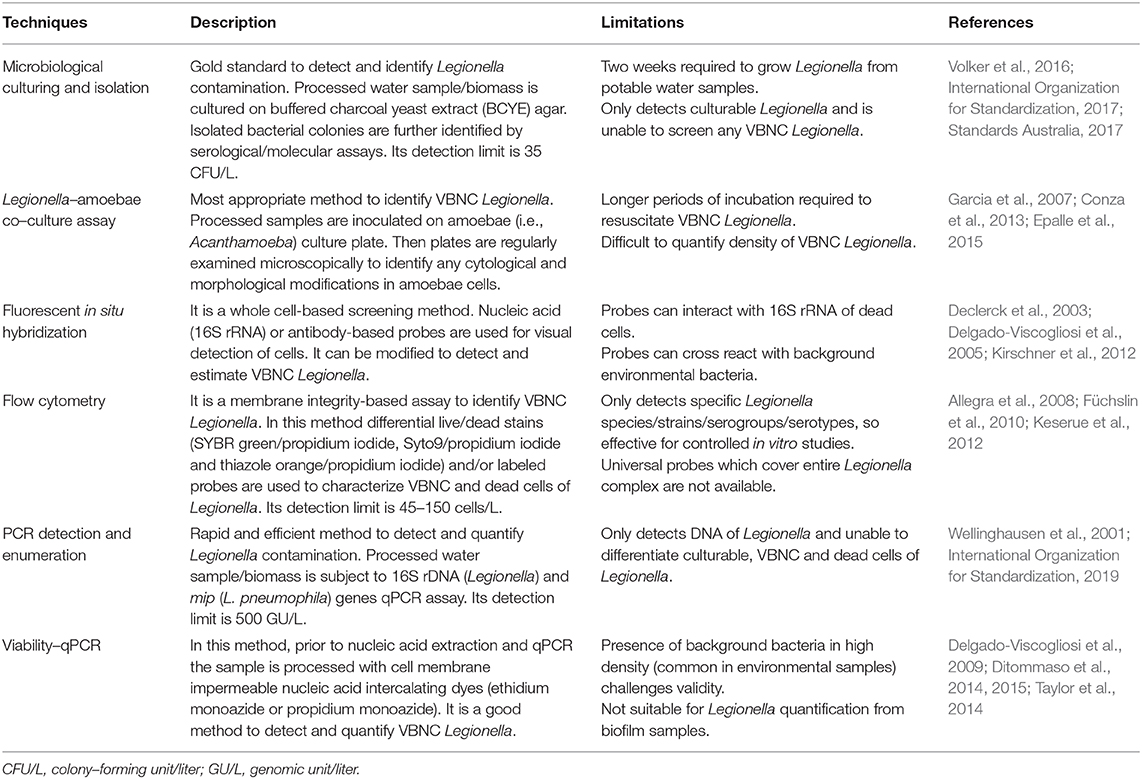
Table 2. Methods available for screening and detection of Legionella contamination from potable water and building plumbing system.
Conclusion
Restricted water circulation and temporary or permanent water stagnation allows Legionella to colonize in building plumbing systems and water storage facilities. Aging of water, stagnation, and microbial biofilms accelerate decay of residual disinfectants and deteriorates water quality in buildings. Further research is required to better understand role of complex Legionella–protozoa biofilms in degradation of disinfectant in stationary water. To achieve long term disinfection of potable water continuous circulation of thermally or chemically treated water in buildings is the only solution to prevent outbreaks of legionellosis.
Data Availability Statement
The original contributions presented in the study are included in the article/supplementary material, further inquiries can be directed to the corresponding author/s.
Author Contributions
MN and HW drafted and edited the manuscript, HW, KR, MB, and RB corrected and contributed to the manuscript. All authors approved of the final manuscript.
Funding
MN is the recipient of a doctoral scholarship by the Australian Government Research Training Program Scholarship (AGRTP).
Conflict of Interest
The authors declare that the research was conducted in the absence of any commercial or financial relationships that could be construed as a potential conflict of interest.
References
Ahmed, S. S., Hunter, C. M., Mercante, J. W., Garrison, L. E., Turabelidze, G., Kunz, J., et al. (2019). Legionnaires' disease at a hotel in Missouri, 2015: the importance of environmental health expertise in understanding water systems. J. Environ. Health 81, 8–13. Available online at: https://www.neha.org/sites/default/files/publications/jeh/JEH3.19-Feature-Legionnaires-Disease-at-a-Hotel.pdf
Allegra, S., Berger, F., Berthelot, P., Grattard, F., Pozzetto, B., and Riffard, S. (2008). Use of flow cytometry to monitor Legionella viability. Appl. Environ. Microbiol. 74, 7813–7816. doi: 10.1128/AEM.01364-08
Allegra, S., Grattard, F., Girardot, F., Riffard, S., Pozzetto, B., and Berthelot, P. (2011). Longitudinal evaluation of the efficacy of heat treatment procedures against Legionella spp. in hospital water systems by using a flow cytometric assay. Appl. Environ. Microbiol. 77, 1268–1275. doi: 10.1128/AEM.02225-10
Alleron, L., Khemiri, A., Koubar, M., Lacombe, C., Coquet, L., Cosette, P., et al. (2013). VBNC Legionella pneumophila cells are still able to produce virulence proteins. Water Res. 47, 6606–6617. doi: 10.1016/j.watres.2013.08.032
Ashbolt, N. (2015). Environmental (saprozoic) pathogens of engineered water systems: understanding their ecology for risk assessment and management. Pathogens 4:390. doi: 10.3390/pathogens4020390
Barbeau, B., Gauthier, V., Julienne, K., and Carriere, A. (2005). Dead-end flushing of a distribution system: short and long-term effects on water quality. J. Water Supply Res. Technol. Aqua 54, 371–383. doi: 10.2166/aqua.2005.0035
Bartley, P. B., Ben Zakour, N. L., Stanton-Cook, M., Muguli, R., Prado, L., Garnys, V., et al. (2016). Hospital-wide eradication of a nosocomial Legionella pneumophila serogroup 1 outbreak. Clin. Infect. Dis. 62, 273–279. doi: 10.1093/cid/civ870
Bartram, J., Chartier, Y., Lee, J. V., Pond, K., and Surman-Lee, S. (2007). Legionella and the Prevention of Legionellosis. Geneva: World Health Organization.
Benedict, K. M., Reses, H., Vigar, M., Roth, D. M., Roberts, V. A., Mattioli, M., et al. (2017). Surveillance for waterborne disease outbreaks associated with drinking water–United States, 2013–2014. Morb. Mortal. Wkly. Rep. 66, 1216–1221. doi: 10.15585/mmwr.mm6644a3
Berjeaud, J. M., Chevalier, S., Schlusselhuber, M., Portier, E., Loiseau, C., Aucher, W., et al. (2016). Legionella pneumophila: the paradox of a highly sensitive opportunistic waterborne pathogen able to persist in the environment. Front. Microbiol. 7:486. doi: 10.3389/fmicb.2016.00486
Biyela, P. T., Ryu, H., Brown, A., Alum, A., Abbaszadegan, M., and Rittmann, B. E. (2012). Distribution systems as reservoirs of Naegleria fowleri and other amoebae. J. Am. Water Works Assoc. 104, 49–50. doi: 10.5942/jawwa.2012.104.0007
Boppe, I., Bedard, E., Taillandier, C., Lecellier, D., Nantel-Gauvin, M. A., Villion, M., et al. (2016). Investigative approach to improve hot water system hydraulics through temperature monitoring to reduce building environmental quality hazard associated to Legionella. Build. Environ. 108, 230–239. doi: 10.1016/j.buildenv.2016.08.038
Bridier, A., Briandet, R., Thomas, V., and Dubois-Brissonnet, F. (2011). Resistance of bacterial biofilms to disinfectants: a review. Biofouling 27, 1017–1032. doi: 10.1080/08927014.2011.626899
Buse, H. Y., Donohue, M. J., and Ashbolt, N. J. (2013). Hartmannella vermiformis inhibition of Legionella pneumophila cultivability. Microb. Ecol. 66, 715–726. doi: 10.1007/s00248-013-0250-z
Cargill, K. L., Pyle, B. H., Sauer, R. L., and Mcfeters, G. A. (1992). Effects of culture conditions and biofilm formation on the iodine susceptibility of Legionella pneumophila. Can. J. Microbiol. 38, 423–429. doi: 10.1139/m92-071
Casini, B., Baggiani, A., Totaro, M., Mansi, A., Costa, A. L., Aquino, F., et al. (2018). Detection of viable but non-culturable Legionella in hospital water network following monochloramine disinfection. J. Hosp. Infect. 98, 46–52. doi: 10.1016/j.jhin.2017.09.006
Centers for Disease Control Prevention (2020). National Outbreak Reporting System. Available online at: https://wwwn.cdc.gov/norsdashboard/ (accessed April 17, 2020)
Cervero-Arago, S., Schrammel, B., Dietersdorfer, E., Sommer, R., Luck, C., Walochnik, J., et al. (2019). Viability and infectivity of viable but nonculturable Legionella pneumophila strains induced at high temperatures. Water Res. 158, 268–279. doi: 10.1016/j.watres.2019.04.009
Cervero-Arago, S., Sommer, R., and Araujo, R. M. (2014). Effect of UV irradiation (253.7 nm) on free Legionella and Legionella associated with its amoebae hosts. Water Res. 67, 299–309. doi: 10.1016/j.watres.2014.09.023
Ciesielski, C. A., Blaser, M. J., and Wang, W. L. (1984). Role of stagnation and obstruction of water flow in isolation of Legionella pneumophila from hospital plumbing. Appl. Environ. Microbiol. 48, 984–987. doi: 10.1128/AEM.48.5.984-987.1984
Clark, R., Lykins, B., Block, J., Wymer, L., and Reasoner, D. (1994). Water quality changes in a simulated distribution system. J. Water Supply Res. Technol. Aqua 43, 263–277.
Conza, L., Casati, S., and Gaia, V. (2013). Detection limits of Legionella pneumophila in environmental samples after co-culture with Acanthamoeba polyphaga. BMC Microbiol. 13:49. doi: 10.1186/1471-2180-13-49
Cooper, I. R., and Hanlon, G. W. (2010). Resistance of Legionella pneumophila serotype 1 biofilms to chlorine-based disinfection. J. Hosp. Infect. 74, 152–159. doi: 10.1016/j.jhin.2009.07.005
Darelid, J., Lofgren, S., and Malmvall, B. E. (2002). Control of nosocomial Legionnaires' disease by keeping the circulating hot water temperature above 55 degrees C: experience from a 10-year surveillance programme in a district general hospital. J. Hosp. Infect. 50, 213–219. doi: 10.1053/jhin.2002.1185
Declerck, P., Verelst, L., Duvivier, L., Van Damme, A., and Ollevier, F. (2003). A detection method for Legionella spp. in (cooling) water: fluorescent in situ hybridisation (FISH) on whole bacteria. Water Sci. Technol. 47, 143–146. doi: 10.2166/wst.2003.0184
Delgado-Viscogliosi, P., Simonart, T., Parent, V., Marchand, G., Dobbelaere, M., Pierlot, E., et al. (2005). Rapid method for enumeration of viable Legionella pneumophila and other Legionella spp. in water. Appl. Environ. Microbiol. 71, 4086–4096. doi: 10.1128/AEM.71.7.4086-4096.2005
Delgado-Viscogliosi, P., Solignac, L., and Delattre, J. M. (2009). Viability PCR, a culture-independent method for rapid and selective quantification of viable Legionella pneumophila cells in environmental water samples. Appl. Environ. Microbiol. 75, 3502–3512. doi: 10.1128/AEM.02878-08
Ditommaso, S., Ricciardi, E., Giacomuzzi, M., Rivera, S. R. A., Ceccarelli, A., and Zotti, C. M. (2014). Overestimation of the Legionella spp. load in environmental samples by quantitative real-time PCR: pretreatment with propidium monoazide as a tool for the assessment of an association between Legionella concentration and sanitary risk. Diagn. Microbiol. Infect. Dis. 80, 260–266. doi: 10.1016/j.diagmicrobio.2014.09.010
Ditommaso, S., Ricciardi, E., Giacomuzzi, M., Rivera, S. R. A., and Zotti, C. M. (2015). Legionella in water samples: how can you interpret the results obtained by quantitative PCR? Mol. Cell Probes 29, 7–12. doi: 10.1016/j.mcp.2014.09.002
Dobrowsky, P. H., Khan, S., Cloete, T. E., and Khan, W. (2016). Molecular detection of Acanthamoeba spp., Naegleria fowleri, and Vermamoeba (Hartmannella) vermiformis as vectors for Legionella spp. in untreated and solar pasteurized harvested rainwater. Parasit Vectors 9:539. doi: 10.1186/s13071-016-1829-2
Donlan, R. M., Forster, T., Murga, R., Brown, E., Lucas, C., Carpenter, J., et al. (2005). Legionella pneumophila associated with the protozoan Hartmannella vermiformis in a model multi-species biofilm has reduced susceptibility to disinfectants. Biofouling 21, 1–7. doi: 10.1080/08927010500044286
Dupuy, M., Mazoua, S., Berne, F., Bodet, C., Garrec, N., Herbelin, P., et al. (2011). Efficiency of water disinfectants against Legionella pneumophila and Acanthamoeba. Water Res. 45, 1087–1094. doi: 10.1016/j.watres.2010.10.025
Edagawa, A., Kimura, A., Doi, H., Tanaka, H., Tomioka, K., Sakabe, K., et al. (2008). Detection of culturable and nonculturable Legionella species from hot water systems of public buildings in Japan. J. Appl. Microbiol. 105, 2104–2114. doi: 10.1111/j.1365-2672.2008.03932.x
Environmental Protection Agency (1989). Handbook of Methods for Acid Deposition Studies Field Operations for Surface Water Chemistry (EPA/600/4-89/020). Washington, DC: US Environmental Protection Agency, Office of Research and Development.
Environmental Protection Agency (2016a). Six-Year Review 3 Technical Support Document for Microbial Contaminant Regulations. Available online at: https://www.epa.gov/sites/production/files/2016-12/documents/810r16010.pdf (accessed April 21, 2020)
Environmental Protection Agency (2016b). Technologies for Legionella Control in Premise Plumbing Systems: Scientific Literature Review. Available online at: https://www.epa.gov/sites/production/files/2016-09/documents/legionella_document_master_september_2016_final.pdf (accessed April 21, 2020)
Epalle, T., Girardot, F., Allegra, S., Maurice-Blanc, C., Garraud, O., and Riffard, S. (2015). Viable but not culturable forms of Legionella pneumophila generated after heat shock treatment are infectious for macrophage-like and alveolar epithelial cells after resuscitation on Acanthamoeba polyphaga. Microb. Ecol. 69, 215–224. doi: 10.1007/s00248-014-0470-x
European Centre for Disease Prevention and Control (2019). Surveillance Report: Legionnaires' Disease Annual Epidemiological Report for 2017. Stockholm: European Centre for Disease Prevention and Control.
Ezzeddine, H., Van Ossel, C., Delmee, M., and Wauters, G. (1989). Legionella spp. in a hospital hot water system: effect of control measures. J. Hosp. Infect. 13, 121–131. doi: 10.1016/0195-6701(89)90018-2
Farhat, M., Moletta-Denat, M., Frere, J., Onillon, S., Trouilhe, M. C., and Robine, E. (2012). Effects of disinfection on Legionella spp., eukarya, and biofilms in a hot water system. Appl. Environ. Microbiol. 78, 6850–6858. doi: 10.1128/AEM.00831-12
Farhat, M., Trouilhe, M. C., Briand, E., Moletta-Denat, M., Robine, E., and Frere, J. (2010). Development of a pilot-scale 1 for Legionella elimination in biofilm in hot water network: heat shock treatment evaluation. J. Appl. Microbiol. 108, 1073–1082. doi: 10.1111/j.1365-2672.2009.04541.x
Farhat, M., Trouilhé, M. C., Forêt, C., Hater, W., Moletta-Denat, M., Robine, E., et al. (2011). Chemical disinfection of Legionella in hot water systems biofilm: a pilot-scale 1 study. Water Sci. Technol. 64, 708–714. doi: 10.2166/wst.2011.696
Fields, B. S., Benson, R. F., and Besser, R. E. (2002). Legionella and Legionnaires' disease: 25 years of investigation. Clin. Microbiol. Rev. 15, 506–526. doi: 10.1128/CMR.15.3.506-526.2002
Fitzhenry, R., Weiss, D., Cimini, D., Balter, S., Boyd, C., Alleyne, L., et al. (2017). Legionnaires' disease outbreaks and cooling towers, New York city, New York, USA. Emerg. Infect. Dis. 23, 1769–1776. doi: 10.3201/eid2311.161584
Flemming, H.-C., Percival, S., and Walker, J. (2002). Contamination potential of biofilms in water distribution systems. Water Sci. Technol. Water Supply 2, 271–280. doi: 10.2166/ws.2002.0032
Fliermans, C., Cherry, W., Orrison, L., Smith, S., Tison, D., and Pope, D. (1981). Ecological distribution of Legionella pneumophila. Appl. Environ. Microbiol. 41, 9–16. doi: 10.1128/AEM.41.1.9-16.1981
Füchslin, H. P., Kötzsch, S., Keserue, H. A., and Egli, T. (2010). Rapid and quantitative detection of Legionella pneumophila applying immunomagnetic separation and flow cytometry. Cytometry A 77, 264–274. doi: 10.1002/cyto.a.20858
Fux, C. A., Costerton, J. W., Stewart, P. S., and Stoodley, P. (2005). Survival strategies of infectious biofilms. Trends Microbiol. 13, 34–40. doi: 10.1016/j.tim.2004.11.010
Galvin, R. (2011). Eliminate dead end water. Opflow 37, 20–21. doi: 10.1002/j.1551-8701.2011.tb03112.x
Garcia, M. T., Jones, S., Pelaz, C., Millar, R. D., and Abu Kwaik, Y. (2007). Acanthamoeba polyphaga resuscitates viable non-culturable Legionella pneumophila after disinfection. Environ. Microbiol. 9, 1267–1277. doi: 10.1111/j.1462-2920.2007.01245.x
Garrison, L. E., Kunz, J. M., Cooley, L. A., Moore, M. R., Lucas, C., Schrag, S., et al. (2016). Vital signs: deficiencies in environmental control identified in outbreaks of Legionnaires' disease–North America, 2000–2014. Am. J. Transplant. 16, 3049–3058. doi: 10.1111/ajt.14024
Gauthier, V., Gerard, B., Portal, J. M., Block, J. C., and Gatel, D. (1999). Organic matter as loose deposits in a drinking water distribution system. Water Res. 33, 1014–1026. doi: 10.1016/S0043-1354(98)00300-5
Gavalda, L., Garcia-Nunez, M., Quero, S., Gutierrez-Milla, C., and Sabria, M. (2019). Role of hot water temperature and water system use on Legionella control in a tertiary hospital: an 8-year longitudinal study. Water Res. 149, 460–466. doi: 10.1016/j.watres.2018.11.032
Girolamini, L., Dormi, A., Pellati, T., Somaroli, P., Montanari, D., Costa, A., et al. (2019). Advances in Legionella control by a new formulation of hydrogen peroxide and silver salts in a hospital hot water network. Pathogens 8:209. doi: 10.3390/pathogens8040209
Goyal, R. V., and Patel, H. M. (2015). Analysis of residual chlorine in simple drinking water distribution system with intermittent water supply. Appl. Water Sci. 5, 311–319. doi: 10.1007/s13201-014-0193-7
Huang, C., Shen, Y., Smith, R. L., Dong, S., and Nguyen, T. H. (2020). Effect of disinfectant residuals on infection risks from Legionella pneumophila released by biofilms grown under simulated premise plumbing conditions. Environ. Int. 137:105561. doi: 10.1016/j.envint.2020.105561
International Organization for Standardization (2017). ISO11731:2017-05 Water quality–Enumeration of Legionella. Geneva: ISO.
International Organization for Standardization (2019). ISO/TS12869:2019 Water Quality–Detection and Quantification of Legionella spp. and/or Legionella pneumophila by Concentration and Genic Amplification by Quantitative Polymerase Chain Reaction (qPCR). Geneva: ISO.
Keserue, H. A., Baumgartner, A., Felleisen, R., and Egli, T. (2012). Rapid detection of total and viable Legionella pneumophila in tap water by immunomagnetic separation, double fluorescent staining, and flow cytometry. Microb. Biotechnol. 5, 753–763. doi: 10.1111/j.1751-7915.2012.00366.x
Kilvington, S., and Price, J. (1990). Survival of Legionella pneumophila within cysts of Acanthamoeba polyphaga following chlorine exposure. J. Appl. Bacteriol. 68, 519–525. doi: 10.1111/j.1365-2672.1990.tb02904.x
Kim, B. R., Anderson, J. E., Mueller, S. A., Gaines, W. A., and Kendall, A. M. (2002). Literature review–efficacy of various disinfectants against Legionella in water systems. Water Res. 36, 4433–4444. doi: 10.1016/S0043-1354(02)00188-4
Kirschner, A. K., Rameder, A., Schrammel, B., Indra, A., Farnleitner, A. H., and Sommer, R. (2012). Development of a new CARD-FISH protocol for quantification of Legionella pneumophila and its application in two hospital cooling towers. J. Appl. Microbiol. 112, 1244–1256. doi: 10.1111/j.1365-2672.2012.05289.x
Lautenschlager, K., Boon, N., Wang, Y., Egli, T., and Hammes, F. (2010). Overnight stagnation of drinking water in household taps induces microbial growth and changes in community composition. Water Res. 44, 4868–4877. doi: 10.1016/j.watres.2010.07.032
Li, L., Mendis, N., Trigui, H., Oliver, J. D., and Faucher, S. P. (2014). The importance of the viable but non-culturable state in human bacterial pathogens. Front. Microbiol. 5:258. doi: 10.3389/fmicb.2014.00258
Ling, F., Whitaker, R., Lechevallier, M. W., and Liu, W. T. (2018). Drinking water microbiome assembly induced by water stagnation. ISME J. 12, 1520–1531. doi: 10.1038/s41396-018-0101-5
Lipphaus, P., Hammes, F., Kotzsch, S., Green, J., Gillespie, S., and Nocker, A. (2014). Microbiological tap water profile of a medium-sized building and effect of water stagnation. Environ. Technol. 35, 620–628. doi: 10.1080/09593330.2013.839748
Liu, Z., Lin, Y. E., Stout, J. E., Hwang, C. C., Vidic, R. D., and Yu, V. L. (2006). Effect of flow regimes on the presence of Legionella within the biofilm of a model plumbing system. J. Appl. Microbiol. 101, 437–442. doi: 10.1111/j.1365-2672.2006.02970.x
Loret, J. F., Robert, S., Thomas, V., Cooper, A. J., Mccoy, W. F., and Levi, Y. (2005). Comparison of disinfectants for biofilm, protozoa, and Legionella control. J. Water Health 3, 423–433. doi: 10.2166/wh.2005.047
Manuel, C., Nunes, O., and Melo, L. (2009). Unsteady state flow and stagnation in distribution systems affect the biological stability of drinking water. Biofouling 26, 129–139. doi: 10.1080/08927010903383448
Mustapha, P., Epalle, T., Allegra, S., Girardot, F., Garraud, O., and Riffard, S. (2015). Monitoring of Legionella pneumophila viability after chlorine dioxide treatment using flow cytometry. Res. Microbiol. 166, 215–219. doi: 10.1016/j.resmic.2015.01.004
National Academies of Sciences Engineering and Medicine (2019). “Strategies for Legionella control and their application in building water systems,” in Committee on Management of Legionella in Water Systems, eds J. B. Rose (Washington, DC: National Academies Press), 165–232.
Ndiongue, S., Huck, P., and Slawson, R. (2005). Effects of temperature and biodegradable organic matter on control of biofilms by free chlorine in a model drinking water distribution system. Water Res. 39, 953–964. doi: 10.1016/j.watres.2004.12.019
Orsi, G. B., Vitali, M., Marinelli, L., Ciorba, V., Tufi, D., Del Cimmuto, A., et al. (2014). Legionella control in the water system of antiquated hospital buildings by shock and continuous hyperchlorination: 5 years experience. BMC Infect. Dis. 14:394. doi: 10.1186/1471-2334-14-394
Paszko-Kolva, C., Shahamat, M., and Colwell, R. R. (1992). Long-term survival of Legionella pneumophila serogroup 1 under low-nutrient conditions and associated morphological changes. FEMS Microbio. Ecol. 11, 45–55. doi: 10.1111/j.1574-6968.1992.tb05794.x
Patrick, A. S., Roland, A., Chris, A., and Samuel, B. D. (2012). Nutrient-induced growth of coliform and HPC bacteria in drinking-water pipes. J. Environ. Prot. 3, 508–517. doi: 10.4236/jep.2012.36061
Patterson, W. J., Seal, D. V., Curran, E., Sinclair, T. M., and Mcluckie, J. C. (1994). Fatal nosocomial Legionnaires' disease: relevance of contamination of hospital water supply by temperature-dependent buoyancy-driven flow from spur pipes. Epidemiol. Infect. 112, 513–525. doi: 10.1017/S0950268800051219
Pepper, I. L., Rusin, P., Quintanar, D. R., Haney, C., Josephson, K. L., and Gerba, C. P. (2004). Tracking the concentration of heterotrophic plate count bacteria from the source to the consumer's tap. Int. J. Food Microbiol. 92, 289–295. doi: 10.1016/j.ijfoodmicro.2003.08.021
Peter, A., and Routledge, E. (2018). Present-day monitoring underestimates the risk of exposure to pathogenic bacteria from cold water storage tanks. PLoS ONE 13:e0195635. doi: 10.1371/journal.pone.0195635
Pontius, F. W., (ed.). (1990). “Water quality and treatment: a handbook of community water supplies, American water works association,” in Water Quality and Treatment: A Handbook of Community Water Supplies (New York, NY: McGraw-Hill).
Prévost, M., Rompré, A., Baribeau, H., Coallier, J., and Lafrance, P. (1997). Service lines: their effect on microbiological quality. J. Am. Water Works Assoc. 89, 78–91. doi: 10.1002/j.1551-8833.1997.tb08261.x
Rhoads, W. J., Ji, P., Pruden, A., and Edwards, M. A. (2015). Water heater temperature set point and water use patterns influence Legionella pneumophila and associated microorganisms at the tap. Microbiome 3:67. doi: 10.1186/s40168-015-0134-1
Rhoads, W. J., Pruden, A., and Edwards, M. A. (2016). Survey of green building water systems reveals elevated water age and water quality concerns. Environ. Sci. Wat. Res. Technol. 2, 164–173. doi: 10.1039/C5EW00221D
Rosario-Ortiz, F., Rose, J., Speight, V., Von Gunten, U., and Schnoor, J. (2016). How do you like your tap water? Science 351, 912–914. doi: 10.1126/science.aaf0953
Saby, S., Vidal, A., and Suty, H. (2005). Resistance of Legionella to disinfection in hot water distribution systems. Water Sci. Technol. 52, 15–28. doi: 10.2166/wst.2005.0216
Scaturro, M., Dell'eva, I., Helfer, F., and Ricci, M. L. (2007). Persistence of the same strain of Legionella pneumophila in the water system of an Italian hospital for 15 years. Infect. Control Hosp. Epidemiol. 28, 1089–1092. doi: 10.1086/519869
Shah, P. P., Barskey, A. E., Binder, A. M., Edens, C., Lee, S., Smith, J. C., et al. (2018). Legionnaires' Disease Surveillance Summary Report, United States: 2014-1015. Centers for Disease Control and Prevention.
Sidari, F. P., Stout, J. E., Vanbriesen, J. M., Bowman, A. M., Grubb, D., Neuner, A., et al. (2004). Keeping Legionella out of water systems. J. Am. Water Works Assoc. 96, 111–119. doi: 10.1002/j.1551-8833.2004.tb10538.x
Standards Australia (2017). AS5132:2017 Waters–Examination for Legionella spp. Including Legionella pneumophila–Using Concentration. Sydney: Australian Standard®.
Steinert, M., Emody, L., Amann, R., and Hacker, J. (1997). Resuscitation of viable but nonculturable Legionella pneumophila Philadelphia JR32 by Acanthamoeba castellanii. Appl. Environ. Microbiol. 63, 2047–2053. doi: 10.1128/AEM.63.5.2047-2053.1997
Steinert, M., Ockert, G., Luck, C., and Hacker, J. (1998). Regrowth of Legionella pneumophila in a heat-disinfected plumbing system. Zentralbl. Bakteriol. 288, 331–342. doi: 10.1016/S0934-8840(98)80005-4
Taylor, M. J., Bentham, R. H., and Ross, K. E. (2014). Limitations of using propidium monoazide with qPCR to discriminate between live and dead Legionella in biofilm samples. Microbiol. Insights 7:S17723. doi: 10.4137/MBI.S17723
Tercelj-Zorman, M., Seljak, M., Stare, J., Mencinger, J., Rakovec, J., Rylander, R., et al. (2004). A hospital outbreak of Legionella from a contaminated water supply. Arch. Environ. Health 59, 156–159. doi: 10.3200/AEOH.59.3.156-159
Tesauro, M., Bianchi, A., Consonni, M., Pregliasco, F., and Galli, M. G. (2010). Environmental surveillance of Legionella pneumophila in two Italian hospitals. Ann. Ist. Super. Sanita 46, 274–278. doi: 10.4415/ANN_10_03_08
Thomas, V., Bouchez, T., Nicolas, V., Robert, S., Loret, J. F., and Levi, Y. (2004). Amoebae in domestic water systems: resistance to disinfection treatments and implication in Legionella persistence. J. Appl. Microbiol. 97, 950–963. doi: 10.1111/j.1365-2672.2004.02391.x
Totaro, M., Mariotti, T., Bisordi, C., De Vita, E., Valentini, P., Costa, A. L., et al. (2020). Evaluation of Legionella pneumophila decrease in hot water network of four hospital buildings after installation of electron time flow taps. Water 12:210. doi: 10.3390/w12010210
Totaro, M., Valentini, P., Costa, A. L., Giorgi, S., Casini, B., and Baggiani, A. (2018). Rate of Legionella pneumophila colonization in hospital hot water network after time flow taps installation. J. Hosp. Infect. 98, 60–63. doi: 10.1016/j.jhin.2017.08.021
Trop Skaza, A., Beskovnik, L., Storman, A., Kese, D., and Ursic, S. (2012). Epidemiological investigation of a legionellosis outbreak in a Slovenian nursing home, August 2010. Scand. J. Infect. Dis. 44, 263–269. doi: 10.3109/00365548.2011.635313
Tsagkari, E., and Sloan, W. T. (2018). “Biofilm growth in drinking water systems under stagnant conditions,” in E-proceedings of Protection and Restoration of the Environment XIV (Thessaloniki), 707–717.
Turetgen, I. (2008). Induction of Viable but Non-culturable (VBNC) state and the effect of multiple subculturing on the survival of Legionella pneumophila strains in the presence of monochloramine. Ann. Microbiol. 58, 153–156. doi: 10.1007/BF03179460
Vasconcelos, J. J., Rossman, L. A., Grayman, W. M., Boulos, P. F., and Clark, R. M. (1997). Kinetics of chlorine decay. J. Am. Water Works Assoc. 89, 54–65. doi: 10.1002/j.1551-8833.1997.tb08259.x
Vervaeren, H., Temmerman, R., Devos, L., Boon, N., and Verstraete, W. (2006). Introduction of a boost of Legionella pneumophila into a stagnant-water model by heat treatment. FEMS Microbiol. Ecol. 58, 583–592. doi: 10.1111/j.1574-6941.2006.00181.x
Vieira, P., Coelho, S. T., and Loureiro, D. (2004). Accounting for the influence of initial chlorine concentration, TOC, iron, and temperature when modelling chlorine decay in water supply. J. Water Supply Res. Technol. Aqua 53, 453–467. doi: 10.2166/aqua.2004.0036
Volker, S., Schreiber, C., and Kistemann, T. (2016). Modelling characteristics to predict Legionella contamination risk–Surveillance of drinking water plumbing systems and identification of risk areas. Int. J. Hyg. Environ. Health 219, 101–109. doi: 10.1016/j.ijheh.2015.09.007
Voss, L., Button, K. S., and Tuovinen, O. H. (1985). Legioneilla pneumophila in a metropolitan water distribution system. Environ. Technol. 6, 429–438. doi: 10.1080/09593338509384361
Wadowsky, R. M., Wolford, R., Mcnamara, A., and Yee, R. B. (1985). Effect of temperature, pH, and oxygen level on the multiplication of naturally occurring Legionella pneumophila in potable water. Appl. Environ. Microbiol. 49, 1197–1205. doi: 10.1128/AEM.49.5.1197-1205.1985
Wang, Y., Claeys, L., Van Der Ha, D., Verstraete, W., and Boon, N. (2010). Effects of chemically and electrochemically dosed chlorine on Escherichia coli and Legionella beliardensis assessed by flow cytometry. Appl. Microbiol. Biotechnol. 87, 331–341. doi: 10.1007/s00253-010-2526-2
Wellinghausen, N., Frost, C., and Marre, R. (2001). Detection of Legionellae in hospital water samples by quantitative real-time LightCycler PCR. Appl. Environ. Microbiol. 67, 3985–3993. doi: 10.1128/AEM.67.9.3985-3993.2001
Whiley, H. (2016). Legionella risk management and control in potable water systems: argument for the abolishment of routine testing. Int. J. Environ. Res. Public Health 14:12. doi: 10.3390/ijerph14010012
Whiley, H., Bentham, R., and Brown, M. H. (2017). Legionella persistence in manufactured water systems: pasteurization potentially selecting for thermal tolerance. Front. Microbiol. 8:1330. doi: 10.3389/fmicb.2017.01330
World Health Organization (2004). Guidelines for Drinking-Water Quality. Geneva: World Health Organization.
Xu, J., Huang, C., Shi, X., Dong, S., Yuan, B., and Nguyen, T. H. (2018). Role of drinking water biofilms on residual chlorine decay and trihalomethane formation: an experimental and modeling study. Sci. Total Environ. 642, 516–525. doi: 10.1016/j.scitotenv.2018.05.363
Keywords: Legionnaires' disease, legionellosis, building, plumbing, water stagnation, flow dynamics, dead legs, dead ends
Citation: Nisar MA, Ross KE, Brown MH, Bentham R and Whiley H (2020) Water Stagnation and Flow Obstruction Reduces the Quality of Potable Water and Increases the Risk of Legionelloses. Front. Environ. Sci. 8:611611. doi: 10.3389/fenvs.2020.611611
Received: 30 September 2020; Accepted: 24 November 2020;
Published: 14 December 2020.
Edited by:
Efthalia Chatzisymeon, University of Edinburgh, United KingdomReviewed by:
Joseph Oliver Falkinham, Virginia Tech, United StatesAngelo Baggiani, University of Pisa, Italy
Copyright © 2020 Nisar, Ross, Brown, Bentham and Whiley. This is an open-access article distributed under the terms of the Creative Commons Attribution License (CC BY). The use, distribution or reproduction in other forums is permitted, provided the original author(s) and the copyright owner(s) are credited and that the original publication in this journal is cited, in accordance with accepted academic practice. No use, distribution or reproduction is permitted which does not comply with these terms.
*Correspondence: Harriet Whiley, aGFycmlldC53aGlsZXkmI3gwMDA0MDtmbGluZGVycy5lZHUuYXU=